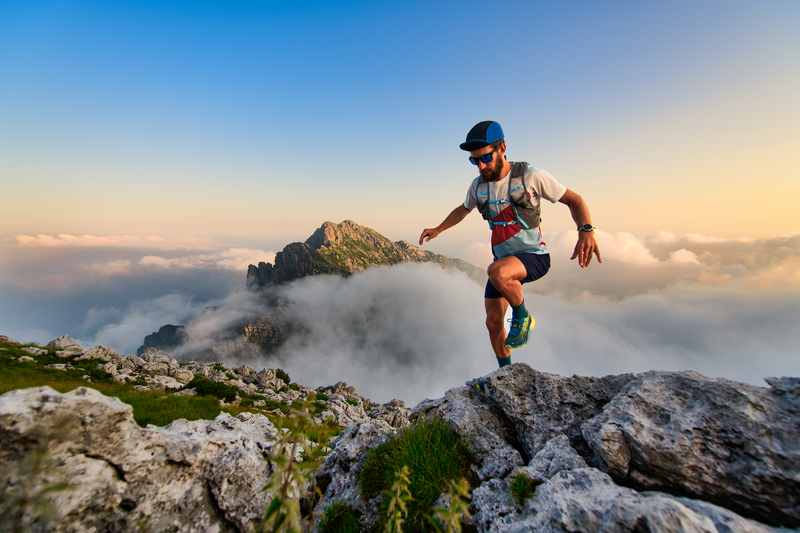
94% of researchers rate our articles as excellent or good
Learn more about the work of our research integrity team to safeguard the quality of each article we publish.
Find out more
REVIEW article
Front. Endocrinol. , 10 August 2021
Sec. Diabetes: Molecular Mechanisms
Volume 12 - 2021 | https://doi.org/10.3389/fendo.2021.716625
This article is part of the Research Topic Pancreatic Islets Cell Therapy: Immune protection and Advanced Technology View all 8 articles
Insulin injection is currently the main therapy for type 1 diabetes (T1D) or late stage of severe type 2 diabetes (T2D). Human pancreatic islet transplantation confers a significant improvement in glycemic control and prevents life-threatening severe hypoglycemia in T1D patients. However, the shortage of cadaveric human islets limits their therapeutic potential. In addition, chronic immunosuppression, which is required to avoid rejection of transplanted islets, is associated with severe complications, such as an increased risk of malignancies and infections. Thus, there is a significant need for novel approaches to the large-scale generation of functional human islets protected from autoimmune rejection in order to ensure durable graft acceptance without immunosuppression. An important step in addressing this need is to strengthen our understanding of transplant immune tolerance mechanisms for both graft rejection and autoimmune rejection. Engineering of functional human pancreatic islets that can avoid attacks from host immune cells would provide an alternative safe resource for transplantation therapy. Human pluripotent stem cells (hPSCs) offer a potentially limitless supply of cells because of their self-renewal ability and pluripotency. Therefore, studying immune tolerance induction in hPSC-derived human pancreatic islets will directly contribute toward the goal of generating a functional cure for insulin-dependent diabetes. In this review, we will discuss the current progress in the immune protection of stem cell-derived islet cell therapy for treating diabetes.
Diabetes is a complex disease that affects more than 30 million people in the US alone and over 463 million people worldwide. Individuals with diabetes are subject to an increased risk for mortality due to cancers, infectious diseases, and other complications (1–6). The current COVID-19 pandemic has additionally highlighted that diabetes is a major risk factor for severe bouts of disease (7–18). In particular, type 1 diabetes (T1D) is a significant burden that typically appears during adolescence and requires life-long insulin administration and blood glucose monitoring (19). T1D is a chronic disease characterized by the autoimmune destruction of pancreatic islet β cells (20). This irreversible loss of insulin-producing β cells impairs crucial glucose uptake in peripheral tissues, resulting in hyperglycemia and subsequent life-threatening microvascular and macrovascular complications (21). Though the etiology of T1D remains unclear, it is known that both environmental risk factors and genetic susceptibility contribute to disease pathogenesis (22). The multifactorial nature of the disease precludes the discovery of a cure. However, the increasing global T1D prevalence, as well as the significant economic and social burdens, demands a solution (23). Currently, T1D and late-stage type 2 diabetes (T2D), a condition triggered by severe peripheral insulin resistance and β cell dysfunction, comes in the form of exogenous insulin administration. However, this method of insulin delivery is not an accurate substitute for normal pancreatic islet function, largely due to the lack of precise temporal glucose control (24). In comparison, allogeneic pancreatic islet transplantation offers a minimally invasive treatment option for T1D patients, which significantly improves glycemic control while preventing severe hypoglycemia (25). Although clinical trials indicate that pancreatic islet transplantation is a promising β cell replacement therapy (26, 27), there are two key issues that prevent its widespread therapeutic utilization. The first issue is a chronic shortage of human islets; the shortage of cadaveric pancreatic islets and the low yield of pancreatic islet purification directly limits transplantation use, whose efficacy depends on the number of functional islets that survive engraftment (28). Additionally, the cumulative cost of islet transplantation is exceedingly high, amounting to more than $120,000 in 2009 per surgical treatment (29), limiting the use of this therapy to only wealthy patients in high-income countries. The second issue is the immune rejection and loss of function of transplanted grafts; patients who have undergone pancreatic islet transplantation require immunosuppressive agents, such as thymoglobulin and basiliximab, to avoid graft rejection of transplanted islets (30–32). Chronic immunosuppression can lead to increased risks of infection, autoreactivity, and the induction of malignancies (33–35).
The shortage of human cadaveric islets, the high cost of islet transplantation, and the complications associated with life-long immunosuppression collectively demonstrate the crucial need for novel approaches to pancreatic islet transplantation. One promising avenue of research is the generation of functional pancreatic islets from human pluripotent stem cells (hPSCs) such as embryonic pluripotent stem cells (ESCs) (36) and induced pluripotent stem cells (iPSCs) (37), which possess indefinite self-renewal and pluripotency.
Chemically defined stepwise differentiation by using small molecules and recombinant proteins enables us to induce glucose-responsive, functional insulin-producing cells from hPSCs in a reproducible manner. hPSC-derived pancreatic islet β cells hold great promise as an alternative source of primary islets for treating diabetes, although there remain major limitations in maturity and graft survival due to immune rejection. In vivo, β cells become functionally mature via a long-term of postnatal maturation process. This process has not yet been duplicated in vitro to transform hiPSCs into fully functional β cells equivalent to primary β cells, indicating that further improvements in the hPSC-derived β cell maturation process are required. In addition, even though islet cells delivered from hiPSCs are by definition autologous (and thus MHC-matched), life-long immune suppression may still be required to protect against autoimmune rejection of transplanted islet cells due to a hyperactive immune reaction in T1D patients.
In this review, we will outline the current progress in generating functional human pancreatic islets as well as novel approaches to protect stem-cell derived islets from immune rejection. Lastly, we will discuss limitations that presently hinder the utilization of hPSC-derived β cells as a common diabetes therapy.
Stem cell biology is a rapidly developing field with immense implications for regenerative medicine, including the treatment of diabetes. hPSCs successfully differentiate in vitro into pancreatic progenitors (PPs) and, more recently β-like cells, via differentiation protocols that rely on developmental paradigms and the introduction of small molecules that regulate stage-specific pathways (38) (Figure 1).
Figure 1 Step by step differentiation and maturation to generate functional human islet-like organoids. By using chemically defined recombinant proteins and small molecules, hPSCs are introduced pancreatic lineage specification. Islet-like organoids contains insulin producing β-cells, glucagon producing α-cells, somatostatin producing δ-cells, and pancreatic polypeptide producing γ-cells. The representative duration and small compounds/recombinant proteins used for β-cells differentiation are listed in the Figure.
In 2006, D’Amour et al. developed a five-stage differentiation protocol capable of producing endocrine hormone–expressing cells from hESCs which is a step forward from their protocol for hESC-derived definitive endoderm differentiation (39); however, these insulin-expressing cells largely resemble immature fetal β cells in their glucose responsiveness, despite their promising insulin content (40). Differentiation efficacy has been improved from a few percent to over 10% by identifying significant pathways that regulate human pancreas development such as FGFs, WNTs, BMP, PKC and TGFβ signaling (41–47). However, several key differences between primary adult human β cells and stem cell-derived human β cells have been observed, such as aberrant populations of polyhormonal cells (48), functionality (49), transcriptome (50) and epigenetic profiling (51). In 2014, Pagliuca et al. and Rezania et al. reported a protocol to generate functional glucose-responsive human β cells (52, 53); a simplified protocol has also been reported (54). Notably, these insulin-producing cells reverse diabetes in vivo after transplantation into rodents (52–54). Although these pioneering protocols recaptured well-differentiated mono-hormonal β cell features, such as co-expression of PDX1, NKX6-1 and insulin/c-peptide and high glucose-stimulated insulin secretion, cells exhibited slow insulin secretion speeds in response to glucose and aberrant Ca2+ signaling, which are critical for insulin exocytosis (52, 53). The maturity of β cells can be characterized by two separate components. The first component of maturity is β cell lineage specification: how β cells express specific functional genes such as INS, IAPP, UCN3, MAFA, MAFB, G6PC2 and SIX2, and coordinate the proper β cell identity network (55–63). MAFA regulates INS and G6PC2 gene expression, which are required for enhancing insulin production, and suppressing insulin secretion at lower glucose concentrations to enhance the amplitude of glucose-stimulated insulin secretion (GSIS), respectively (64–66). Another component of β cell maturity is physiological metabolic regulation: how β cells regulate physiological metabolic activity which links to the amplification of GSIS. β cells exhibit unique features of glucose metabolism (67, 68). High expression of glucose transporters, such as GLUT1 in humans and Glut2 in rodents, rapidly equalizes extra- and intracellular glucose concentrations. Low glucose affinity glucokinase (GK) expression, instead of hexokinase (HK) expression, and low lactate dehydorogenese (LDH) and monocarbohydorate transporter (MCT) expression accumulates intracellular pyruvate, which is the primary fuel source of the citric acid cycle and mitochondrial oxidative phosphorylation to produce ATP (67, 69). The increased ATP/ADP ratio by glucose-stimulated fuel metabolic utilities triggers Ca2+ influx and insulin secretion. Fetal and neonatal immature β cells show poor glucose responsiveness and transition to postnatal functional maturation with enhanced mitochondrial metabolic function (70–80). Previously, we have identified a critical role for the nuclear receptor estrogen related receptor gamma (ERRγ) in the postnatal maturation of β cells (77). Coincident with weaning, neonatal β cells become glucose-responsive, acquiring the ability to secrete insulin in response to a high glucose challenge. We have previously reported that the postnatal increase in ERRγ expression orchestrates the metabolic maturation of β cells. Indeed, mice lacking ERRγ in β cells are glucose intolerant and fail to appropriately secrete insulin in response to a high glucose challenge (77). Furthermore, forced ERRγ expression in hiPSC-derived β-like cells (ERRγ-β-like cells) enhances the capacity for oxidative phosphorylation, resulting in significantly improved GSIS function in human β-like cells, both in vitro and in vivo (77). These findings support the notion that a metabolic maturation step driven by ERRγ provides the energetic capacity necessary for GSIS in hiPSC-derived β-like cells. Acknowledging the importance of 3-dimensional (3D) organization and cell-cell communications in organ function and the terminal differentiation of organ-specific cell types (81, 82), we also adapted our technology to generate 3D structured human pancreatic islet tissues called human islet-like organoids (HILOs) from pluripotent stem cells (83). HILOs similar in size to human islets (100-500 μm) have been generated in 5 weeks with newly identified maturation factors such as non-canonical Wnt family member 4 (WNT4), which enhances mitochondrial function to enhance GSIS function. Nair et al. have found that facilitating endocrine cell clustering in vitro promotes human stem cell-derived β cell maturation as demonstrated by increased mitochondrial oxidative respiration and robust insulin secretion (84). Additionally, circadian regulation (85), aberrant expression of glycolytic enzymes (86), and transforming growth factor β (TGF-β) modulation (87) during hPSC differentiation into β cells have been reported as key components in achieving GSIS with dynamic insulin secretion. Recent advances in the single-cell transcriptome approach have enabled the fine tuning of genomic maps in human islet maturation, while further studies to identify the effects of peripheral nerve innervation (88), mesenchymal stromal cell contributions (89) and other microenvironmental cues in human islet maturation are required to overcome the current limitations regarding the efficacy of differentiation and maturation of stem cell-derived islets. Instead of fully functional primary islets or functionally mature stem cell derived islets, pancreatic progenitors from hPSCs have been acknowledged as an alternative source for islet cell therapy. In 2008, Kroon et al. showed that hESC-derived PPs undergo further differentiation and maturation in vivo a few months post-transplantation (90), suggesting that transplantation of hPSCs derived PPs can recapitulate functional organogenesis in vivo. The idea to use hPSC derived PPs for transplantation to ameliorate T1D became popular and it remains open for discussion whether hPSC derived PPs or fully mature β cells are the better source for transplantation (91). A clinical trial using hPSC derived PP for treating diabetes is ongoing (ClinicalTrials.gov: NCT03163511, NCT02239354). Although Chromatin remodeling (51) and systemic gene regulation (92) provides the mechanistic insights, the particular cues which lead to in vivo functional maturation of hPSC derived PP has not been elucidated yet. Sex differences (93) as well as species differences (94) in functional maturation have been observed, suggesting that the efficacy of hPSC derived PP for treating preexisting diabetes may rely on the condition of patients. Advances in the generation of more functionally matured hPSC derived β cell enriched clusters or islets may offer less variation in efficacy for diabetic patients. However, the effort for generating fully mature bona fide human islets has not been ended yet. A better grasp on the complex mechanism of postnatal maturation, include unveiling precise collaboration of transcriptional factors (95) is crucial to optimize in vitro differentiation protocols.
Pancreatic islet transplants are subject to both alloimmune and autoimmune reactivities, which can cause graft rejection or the progressive loss of islet function. To combat graft rejection, previous immunosuppression regimens for allogeneic islet recipients consisted of an induction phase targeting T lymphocytes, followed by the delivery of immunosuppressive maintenance agents such as calcineurin inhibitors, DNA antimetabolites, and corticosteroids (96). Although these drugs bolstered pancreatic islet allogeneic graft survival, they also displayed diabetogenic effects and direct toxicity toward pancreatic β cells (96–101). Moreover, whole body immune suppression increases the risk of cancer development, infectious diseases and other complications, limiting the enthusiasm for islet cell therapy (102). The publication of the Edmonton Protocol in 2000 illustrated that islet transplantation from multiple donors accompanied by a glucocorticoid-free immunosuppressive regimen could achieve graft survival and insulin independence in patients with T1D (103). This steroid-free protocol included an IL-2 receptor antagonist (daclizumab), sirolimus, and low-dose tacrolimus (103). However, researchers at the University of Chicago who assessed the short- and long-term outcomes of the Edmonton protocol concluded that although the patients remained insulin-free for five or more years after the initial transplantation, they suffered recurrent side effects as a result of immunosuppression (104). Recent strategies for immunosuppression have shown clinical improvements derived from modifications made to the Edmonton protocol. Hering et al. employed antithymocyte globulin, daclizumab, and etanercept as an induction protocols, along with a mycophenolate mofetil, sirolimus, and no or low-dose tacrolimus maintenance regimen (30). All eight study participants became insulin- independent, with five out of the eight achieving insulin independence for over a year; importantly, none of the study participants experienced immunosuppression-related adverse events (30). A follow-up phase 3 clinical trial examining human islet transplantation in T1D patients utilized a similar induction immunosuppression regimen of antithymocyte globulin (eventually replaced by basiliximab) and etanercept in addition to sirolimus and low-dose tacrolimus maintenance agents (31). At the 1-year mark, 87.5% of patients achieved an HbA1c < 53 mmol/mol, with HbA1c > 50 mmol/mol indicating diabetes (31, 105). An alternate immunosuppression regimen combined Fc receptor non-binding humanized anti-CD3 monoclonal antibody hOKT3γ 1 (Ala-Ala) and sirolimus induction agents followed by sirolimus and reduced‐dose tacrolimus for maintenance immunosuppression, resulted in a prolonged reduction in CD4+ T-cell expression in islet transplant recipients (106). Bellin et al. illustrated the efficacy of the anti-CD3 monoclonal antibody alone, as well as antithymocyte globulin combined with tumor necrosis factor-alpha (TNF-α) inhibition, over the previously used interleukin-2 receptor antibodies (IL-2RAb) (107). Several studies showed that an effective calcineurin inhibitor-free immunosuppression protocol using the co-stimulation blocker belatacept, achieved insulin independence in transplanted patients (108, 109). Kim et al. found that replacement of tacrolimus with the JAK3 inhibitor tofacitinib effectively suppresses immune responses in diabetic monkeys transplanted with MHC-mismatched allogeneic islets (110). All these efforts contribute to our understanding of immunosuppression strategies to avoid acute immune rejection of transplanted islets, although chronic rejection still remains a major issue (111). Therefore, modern studies on islet transplantation have focused on the development of protective encapsulation biomaterials and immune tolerance induction, particularly with regard to stem cell-derived β cells to overcome both the shortage of functional islet supply and immune rejection.
Many studies have examined the efficacy of transplanting encapsulated pancreatic islets to treat or cure T1D. Cell encapsulation in biomaterials with low immunogenic profiles directly protects grafts from rejection by the host immune system. Encapsulation systems such as the TheraCyte macroencapsulation device system, enable protection of the grafts from host immune cell infiltration via a physical barrier (112–116). A gas exchangeable enhanced O2 supply device has been developed to aim for enhancing the graft survival (117–119). This macroencapsulation device system is being utilized in an ongoing clinical trial of stem cell-derived pancreatic progenitor cells or primary human islets in T1D patients (116, 118). Alternatively, the naturally derived hydrogel alginate is the most common biomaterial employed in microencapsulation (120–129). When combined with divalent ions at physiological concentrations, alginate develops into a gel matrix suitable for cellular encapsulation. This biomaterial provides a selectively permeable membrane, which allows for the diffusion of oxygen, nutrients, and insulin as well as protects transplanted islets from intrinsic immune cell infiltration. This technology was first employed over 40 years ago and demonstrated that implanted alginate-microencapsulated islets could ameliorate diabetes for 2 to 3 weeks in a streptozotocin-induced diabetic rat model (120). In 1994, Soon-Shiong et al. reported that the intraperitoneal injection of alginate-microencapsulated human islets with low-dose cyclosporine normalized blood glucose over 9 months in a patient with T1D without affecting the function of a previously transplanted kidney (123). However, the capsules are prone to destruction by the foreign body response, which leads to fibrosis and loss of islet function, and therefore short-lived glycemic correction (122, 125, 128, 130, 131). Innovations in encapsulation technology have enabled major improvements in transplant function longevity. Altering the spherical dimensions of capsules, reducing transplant volume, and chemically modifying alginate biomaterials have improved biocompatibility and sustained graft function for long-term periods (124, 126, 132–135). Incorporating stem cell technology, Vegas et al. demonstrated that implanting hPSC-derived β cells encapsulated with triazole-thiomorpholine dioxide alginate corrects diabetes in immunocompetent mice, without any immunosuppression (134). The implanted islets exhibited appropriate glucose responsiveness and, upon removal at 174 days, still contained functional β cells (134). Bochenek et al. showed that chemically modified alginate derivatives reduce foreign body immune responses and improve graft survival and GSIS in allogeneic islets of non-human primates (NHP) for 4 months without the need for immunosuppression (135). Alagpulinsa et al. reported that CXCL12-containing sodium alginate-encapsulated hPSC-derived β cells transplanted into immunocompetent mice enhance insulin secretion, normalize hyperglycemia, and remain fully functional for more than 150 days without immunosuppression (136). The preliminary success of hPSC-derived β cell encapsulation and transplantation into diabetic mouse models and NHP provides a proof-of concept for treating T1D in humans using the same strategy. Additionally, in some cases of optimized pancreatic islet encapsulation technology, continuous immunosuppression in transplant recipients, including patients with T1D, is unnecessary (126, 134, 137–139). Further refinement of O2 and nutritional supplies with or without functional vascularization for long-term stability, as well as inquiries into human auto- and alloreactivity toward these alginate compositions, including newly developed nanofiber technologies (140, 141) will be necessary steps toward future clinical significance.
Transplant preconditioning has been investigated to improve islet transplantation outcomes. Early graft failure in pancreatic islet transplant recipients led to inquiries into the complex mechanisms underlying this process. While T-cell recognition of mismatched human leukocyte antigens (HLA) is a major cause of allogeneic graft rejection in T1D patients, hypoxia and inhospitable transplant microenvironments are additional barriers to transplant success. A key trigger of early graft failure, islet embolism during intraportal pancreatic islet transplantation is known to cause liver ischemia and subsequent apoptosis of transplanted pancreatic β cells via induction of pro-inflammatory cytokines (142). Paradoxically, exposing the liver to ischemic conditions prior to transplantation was found to significantly reduce early graft failure in mice by decreasing the mRNA levels of the pro-inflammatory cytokine interleukin-1β (IL-1β) as well as tumor necrosis factor-α (TNF-α) and by increasing the plasma levels of Interleukin-10 (IL-10), an anti-inflammatory cytokine (142–146). This stress-induced resistance induction may not only be beneficial by direct exposure, but also beneficial by indirect exposure to co-cultured cells. Hypoxia-preconditioned mesenchymal stem cells or primary hepatocytes were shown to significantly reduce reactive oxygen species (ROS) production and induce pro-apoptotic proteins, such as Bcl-2, to improve islet survival when co-cultured with human islets (147, 148). Alternatively, pancreatic islets can be directly preconditioned via strategic drug delivery. Rat and human pancreatic islets preconditioned with diazoxide, a potassium channel activator, and subsequently exposed to hypoxic conditions are protected from hypoxia-induced necrosis by an unknown mechanism and ameliorate hyperglycemia in STZ-induced diabetic rats and immune deficient mice, respectively (149). Transplanted mouse pancreatic islet allografts preconditioned with mitomycin c, an antitumor antibiotic, experience a median survival duration of 28 days without immunosuppression compared to 13 days in the control group (150). In an in vitro model, mitomycin c treatment suppressed allograft proinflammatory cytokine expression, decreased inflammatory cell infiltration, and upregulation of CD4+-suppressing regulatory T cells (150). A green tea polyphenol and anti-inflammatory agent, epigallocatechin 3-gallate (EGCG), also improves pancreatic islet transplant viability (151). Mouse pancreatic islets cultured in 100 µM EGCG-containing medium and transplanted under the kidney capsules of STZ-induced diabetic mice demonstrated preserved insulin secretion and decreased ROS production as a function of the Nrf2 pathway (151). Taken together, these findings indicate multiple avenues for further studies focusing on pancreatic islet protection against inflammatory immune responses, hypoxia, and the transplant microenvironment. Additionally, regulatory T-cell (Treg) therapies offer a personalized approach to transplant immune tolerance. Tregs play an important role in preventing autoimmunity, while their antigen specificity ensures that tumor and pathogen immunosurveillance is not hampered (152). After identifying and isolating graft-specific patient Tregs, these cells have been cultured in vitro to generate a clinically useful population (152). An alternate therapy utilizes chimeric antigen receptor (CAR) T-cells, patient-derived T-cells engineered to express a synthetic receptor targeted against a specific antigen (153). CAR-T cells also develop into Tregs with graft-specific antigen receptors (154). Importantly they can be utilized to combat HLA mismatches in transplant graft recipients. Since HLA-A2 population expression is significant and develops a substantial number of HLA-A2-mismatched transplants, HLA-A2-specific CAR-T cells have been developed in a mouse transplant model, which has successfully prevented HLA-A2+ T-cell-initiated graft versus host disease (155). However, challenges remain with CAR-T cell-related toxicity, which must be addressed before application in human transplant cases (156, 157). Nevertheless, these preconditioning approaches may be promising in the context of hPSC-derived β cells, whose culturing methodology can be adapted to optimize graft survival.
The emergence of hiPSC technology, which is derived from various somatic cells, has popularized the idea of personalized treatments in regenerative medicine. One such idea is autologous transplantation by using patients’ self-derived hiPSCs. There are several immunological barriers for transplant technologies, particularly in patients with T1D who intrinsically possess hyperactive immune responses targeted against pancreatic β cells. However, because hiPSCs can be derived from adult tissues, they unlock a powerful form of personalized medicine: fibroblast cells taken from patients, converted into hiPSCs, and differentiated into pancreatic islet β cells could be transplanted autologously. This practice reduces the risk of graft versus host disease by accounting for immune identity (158, 159). Unfortunately, autologous hiPSC production is expensive and time-consuming. To attempt to overcome these disadvantages, there has been the generation of a bank of stringently selected HLA-homozygous hiPSC lines, which can be differentiated and transplanted to a broad group of patients (160–163). Using this strategy, healthy donors with homozygous HLA-A, HLA-B, and HLA-DR have been selected and, according to the frequent HLA haplotypes in the population, hiPSCs have been selectively generated and preserved via cryopreservation (163). Although, this approach may be effective in certain countries with less diversity, it is anticipated that a large-scale bank will be needed to cover the entire global population. Moreover, even with autologous delivery strategies, transplanted hiPSC-derived insulin-producing cells would eventually be rejected due to the hyperactive immune reaction in which activated T-cells are presented insulin as antigens. This autoimmunity is, in some cases, connected to the HLA haplotypes HLA-DR4-DQ8 and HLA-DR3-DQ2, which have been identified as two major genetic risk factors for T1D development (21). Naturally, introducing pancreatic islets with mismatched HLA to transplant recipients compounds this innate immune response by activating T-cells (111). Therefore, in addition to MHC matching strategies, protecting transplanted insulin-producing cells from hyperactive T-cells in T1D may be required. Ultimately, the development of a universal hPSC population that evades immune detection is a major goal of T1D translational research.
Generating universal hPSCs that resist both allogenic and autoimmune rejection would constitute a clear advance in regenerative medicine. The self-renewal function of hPSCs provides a superior opportunity for genomic modification in vitro. Genome engineering technologies such as TALEN (164, 165) or CRISPR (166–171)-based genetic modification offer more flexible HLA design and immune evasive function in both hESCs and hiPSCs. HLA-A and HLA-B deletion reduces antigen presentation, while sustaining HLA-C or HLA-E suppresses NK cell lysis, which reduces allogeneic responses in hPSCs (172–174). In addition to MHC matching, enhancing the ability of transplanted β cells to evade immune detection could be an alternative strategy for reducing the risk of autoimmune rejection. The major question is how β-cells can attain immune tolerance and thus evade autoimmune rejection by T-cells. Anecdotally, one T1D patient who successfully survived for 50 years with intensive insulin injection therapy still retained at least some functional glucose-responsive β-cells, which were surrounded by T-cells because of the presence of antigenic insulin but retained their function (175). Although the limited number of these cells was insufficient to rescue this T1D patient, the mechanism through which these β-cells evade T cell-mediated immune rejection remain unknown. β-cells manifest slow turnover, undergo limited proliferation, and thus lack regenerative capacity (176–178). Cancer cells evade T-cell recognition and autoimmune rejection by expressing the immune checkpoint molecule programmed death 1 ligand 1 (PD-L1/CD274), the ligand of the T-cell inhibitory receptor programmed death 1 (PD-1/CD273) ligand (179). Similarly, a correlation between immune tolerance and PD-1/PD-L1 has been observed in diabetic rodents (180). PD-L1 is expressed in pancreatic islet cells, where it suppresses self-antigen-reactive CD8+ T cells (181–183). In vitro PD-L1 expression in human islet cells and EndoC-βH1 cells is upregulated in response to stimulation with the interferon-γ (IFN-γ), and interferon-α (IFN-α) stimulation (184, 185). Interestingly, when islets isolated from PD-L1-deficient C57BL/6j mice are transplanted into STZ-induced diabetic BALB/c mice, there is increased allograft rejection, inflammatory cell tissue infiltration, and T cell alloreactivity (186). It was also shown that PD-L1 + murine β cells have also been found during immune tolerance in murine β cells in the non-obese spontaneous type 1 diabetic (NOD) mice model (180), however they evidently dedifferentiated and, hence, were non-functional. We have previously shown that forced PD-L1 expression significantly reduced immune cell infiltration into transplanted HILOs, ameliorated diabetes in a xenograft, and humanized allogenic immunocompetent environment for more than ~50 days, significantly longer than their PD-L1-lacking counterparts (83). Notably, PD-L1-expressing grafts contain less CD45+ immune cells, such as T-cells (83). Similarly, it has been shown that PD-L1 overexpression by a CRISPR knock-in system enabled partial protection from autoreactive T-cells modeled with CD19-expressing stem cell-derived functional human β like cells with CAR-T cells (187). Using an alternate approach, pancreatic islet grafts have been engineered with chimeric PD-L1/streptavidin oligomers (SA-PD-L1) (188, 189). More than 90% of these SA-PD-L1 grafts have been retained for over a 100-day observation period after transplantation into STZ-induced diabetic C57BL/6 mice undergoing a 15-day rapamycin course as transient immune suppression (188). Immune cell phenotyping has revealed that SA-PD-L1 induces peri-islet infiltration of FOXP3+ regulatory T-cells, serving as a practical local immune modulation to support long-term graft survival without chronic immune suppression (189). Thus, induction of PD-L1 and other immune-tolerance-aiding protein-induction presents an innovative approach to improving pancreatic islet graft longevity.
PD-L1 + β-cells possess immune tolerance in non-obese diabetic (NOD) mice (180), a common mouse model for T1D. However, these immune tolerant murine β-cells exhibit defective GSIS function, and even their β-cell identity is unclear (180). Our recent findings revealed that constitutive PD-L1 expression in HILOs rapidly ameliorates diabetes in an immune-competent environment (83). In healthy primary human islet β-cells, only a few β-cells express PD-L1. However, IFNγ induces PD-L1 expression in both cadaveric human islets and β-cells or non-β-cell clusters (GFP+ or -) in mature HILOs. However, in agreement with previous reports of cytokine-induced β-cell dysfunction (190–192), stimulation with >10 ng IFNγ for 24 hours significantly reduces gene expression governing GSIS function and β-cell identity such as INS-1, UCN3 and STY4 in HILOs. This suggests that IFNγ signaling paradoxically regulates β-cell dysfunction (defects in GSIS and β-cell identity gene expression) and protection (enhanced PD-L1 expression). Therefore, we hypothesized that short-term IFNγ stimulation is sufficient to induce PD-L1 expression while preserving β-cell function. Interestingly, short term (2 hours) exposure of low dose IFNγ (<10ng/ml) is sufficient to induce PD-L1 expression in HILOs, but the expression of PD-L1 was not sustained for more than 3 days (83). Innate immune mechanisms have evolved into a type of built-in memory (193). This memory is important for maintaining cellular adaptability, which in turn helps with preparedness for similar future events. For example, multiple LPS injections induce differential LPS responsiveness and modify the pathological features after stroke (194). Recent studies have also revealed that innate immune memory is induced not only immune cells but also other differentiated cells such as skin fibroblasts and neuronal cells to alter the transcriptional and physiological immune responses against second or third similar stimulation (194–196). To achieve stable PD-L1 expression without long-term IFNγ exposure, we hypothesized that multiple short-term (2 hours) IFNγ stimulation (MPS) causes transcriptional memory for sustainable PD-L1 expression, ideally without affecting functionality. We found that MPS by IFNγ induces sustainable PD-L1 expression in HILOs (MPS-HILOs) without adversely affecting insulin secretion (83). Furthermore, transcriptome analyses in MPS-HILOs revealed “de novo” anti-inflammatory gene induction by MPS (83). Indeed, MPS-HILOs showed resistance to IL1β-induced β cell dedifferentiation (83). We propose this phenomenon as a novel transcriptional memory system of β-cells for adaptive immune tolerance and evasion. Elucidating the specific mechanisms underlying transcriptional memory in pancreatic islets may provide a novel and effective strategy for inducing immune tolerance in transplant recipients. These findings raise the possibility of generating immune-tolerant mature functional human islets from hPSCs in vitro, which in turn may offer a novel therapeutic approach to avoid graft and autoimmune rejection without immune-suppressive drugs in T1D patients.
Immune-evasive human islets derived from hPSCs represent a promising and renewable cell source with a reduced risk of chronic immune suppression to treat insulin-dependent diabetes. The manufacturing scalability of hPSC-derived β cells or islet organoids must also be addressed before its widespread clinical application can become a reality. Although, there has been success in generating α-like cells from hPSCs (197, 198), no protocol has been successful in generating each endocrine hormone cell independently. Understanding the fine-tuning lineage specification as well as the spatial information of intracellular communication in islet organoids may further improve the efficacy of differentiation and maturation in stem cell derived islets for clinical use. Additionally, improvements in immune protection, particularly with respect to encapsulation biomaterials, T cell or hPSC engineering, preconditioning, and immune tolerance induction, will strongly impact the long-term efficacy of islet cell therapy in both T1D and T2D (Figure 2 and Table 1). Although creating immune evasive human islets is a major goal in achieving standardized islet cell therapy in diabetes, there are potential concerns to develop unwilling driverless cells, such as teratomas, with future events of infection or health complications. A suicide system represented by induced caspase 9 with chimeric dimers eliminates the hPSC-derived cells and may therefore be a potential safeguard system against this concern (199). However, the current suicide system induces approximately 95% apoptosis in hPSCs and hPSC-derived cells, suggesting that further improvements of the system to ensure the 100% elimination of transplanted cells are required (200). The challenges for the long-term survival of hPSC-derived immune evasive derived β cells or islet organoids with improved nutrition and oxygen supply as well as controlled graft microenvironment will make the safe harbor of stem-cell derived islets transplanted into patients with diabetes one step closer to a reality.
Figure 2 Strategies for immune protection of hPSC derived islets. To protect the transplanted hPSC derived islets graft: 1. Immunosuppressants, 2. Macro encapsulation devices (e.g. Theracyte Device), 3. Micro encapsulation gels (e.g. Alginate-coating), 4. MHC-matching, 5. Genome engineering of immune checkpoint molecules and/or HLAs, 6. Induction of immune tolerance by preconditioning or trained immunity.
MT and EY wrote and edited the manuscript. EY conceptualized and obtained funding for this study. All authors contributed to the article and approved the submitted version.
This work was supported by the funding from CTSI-UCLA awards, California Institute for Regenerative Medicine (CIRM)-DISC2 discovery award, Integrated Islet Distribution Program (IIDP) Pilot award, Allen foundation grant, Mishima Kaiun Memorial Foundation Research award and Lundquist Institute Voucher award.
EY is inventor on licensed patents and patent applications related to the HILOs technology described in this manuscript.
The remaining author declares that the research was conducted in the absence of any commercial or financial relationships that could be construed as a potential conflict of interest.
All claims expressed in this article are solely those of the authors and do not necessarily represent those of their affiliated organizations, or those of the publisher, the editors and the reviewers. Any product that may be evaluated in this article, or claim that may be made by its manufacturer, is not guaranteed or endorsed by the publisher.
1. Huang Y, Karuranga S, Malanda B, Williams DRR. Call for Data Contribution to the IDF Diabetes Atlas 9th Edition 2019. Diabetes Res Clin Pract (2018) 140:351–2. doi: 10.1016/j.diabres.2018.05.033
2. Tan X, Lee LK, Huynh S, Pawaskar M, Rajpathak S. Sociodemographic Disparities in the Management of Type 2 Diabetes in the United States. Curr Med Res Opin (2020) 36(6):967–76. doi: 10.1080/03007995.2020.1756764
3. Smalls BL, Ritchwood TD, Bishu KG, Egede LE. Racial/Ethnic Differences in Glycemic Control in Older Adults With Type 2 Diabetes: United States 2003-2014. Int J Environ Res Public Health (2020) 17(3):950. doi: 10.3390/ijerph17030950
4. Shah VN, Grimsmann JM, Foster NC, Dost A, Miller KM, Pavel M, et al. Undertreatment of Cardiovascular Risk Factors in the Type 1 Diabetes Exchange Clinic Network (United States) and the Prospective Diabetes Follow-Up (Germany/Austria) Registries. Diabetes Obes Metab (2020) 22(9):1577–85. doi: 10.1111/dom.14069
5. Rogers MAM, Wei MY, Kim C, Lee JM. Sex Differences in Autoimmune Multimorbidity in Type 1 Diabetes Mellitus and the Risk of Cardiovascular and Renal Disease: A Longitudinal Study in the United States, 2001-2017. J Womens Health (Larchmt) (2020) 29(4):511–9. doi: 10.1089/jwh.2019.7935
6. Rogers MAM, Kim C. Congenital Infections as Contributors to the Onset of Diabetes in Children: A Longitudinal Study in the United States, 2001-2017. Pediatr Diabetes (2020) 21(3):456–9. doi: 10.1111/pedi.12957
7. Cefalu WT, Rodgers GP. COVID-19 and Metabolic Diseases: A Heightened Awareness of Health Inequities and a Renewed Focus for Research Priorities. Cell Metab (2021) 33(3):473–8. doi: 10.1016/j.cmet.2021.02.006
8. Bleich SN, Ard JD. COVID-19, Obesity, and Structural Racism: Understanding the Past and Identifying Solutions for the Future. Cell Metab (2021) 33(2):234–41. doi: 10.1016/j.cmet.2021.01.010
9. Cai J, Li H, Zhang C, Chen Z, Liu H, Lei F, et al. The Neutrophil-to-Lymphocyte Ratio Determines Clinical Efficacy of Corticosteroid Therapy in Patients With COVID-19. Cell Metab (2021) 33(2):258–69.e3. doi: 10.1016/j.cmet.2021.01.002
10. Yu B, Li C, Sun Y, Wang DW. Insulin Treatment is Associated With Increased Mortality in Patients With COVID-19 and Type 2 Diabetes. Cell Metab (2021) 33(1):65–77.e2. doi: 10.1016/j.cmet.2020.11.014
11. Donath MY. Glucose or Insulin, Which Is the Culprit in Patients With COVID-19 and Diabetes? Cell Metab (2021) 33(1):2–4. doi: 10.1016/j.cmet.2020.11.015
12. Kusmartseva I, Wu W, Syed F, van der Heide V, Jorgensen M, Joseph P, et al. Expression of SARS-Cov-2 Entry Factors in the Pancreas of Normal Organ Donors and Individuals With COVID-19. Cell Metab (2020) 32(6):1041–1051 e6. doi: 10.1016/j.cmet.2020.11.005
13. Evans RM, Lippman SM. Shining Light on the COVID-19 Pandemic: A Vitamin D Receptor Checkpoint in Defense of Unregulated Wound Healing. Cell Metab (2020) 32(5):704–9. doi: 10.1016/j.cmet.2020.09.007
14. Cheng X, Liu YM, Li H, Zhang X, Lei F, Qin JJ, et al. Metformin is Associated With Higher Incidence of Acidosis, But Not Mortality, in Individuals With COVID-19 and Pre-Existing Type 2 Diabetes. Cell Metab (2020) 32(4):537–47.e3. doi: 10.1016/j.cmet.2020.08.013
15. Fajgenbaum DC, Rader DJ. Teaching Old Drugs New Tricks: Statins for COVID-19? Cell Metab (2020) 32(2):145–7. doi: 10.1016/j.cmet.2020.07.006
16. Song JW, Lam SM, Fan X, Cao WJ, Wang SY, Tian H, et al. Omics-Driven Systems Interrogation of Metabolic Dysregulation in COVID-19 Pathogenesis. Cell Metab (2020) 32(2):188–202.e5. doi: 10.1016/j.cmet.2020.06.016
17. Zhang XJ, Qin JJ, Cheng X, Shen L, Zhao YC, Yuan Y, et al. In-Hospital Use of Statins Is Associated With a Reduced Risk of Mortality Among Individuals With COVID-19. Cell Metab (2020) 32(2):176–87.e4. doi: 10.1016/j.cmet.2020.06.015
18. Zhu L, She ZG, Cheng X, Qin JJ, Zhang XJ, Cai J, et al. Association of Blood Glucose Control and Outcomes in Patients With COVID-19 and Pre-Existing Type 2 Diabetes. Cell Metab (2020) 31(6):1068–77.e3. doi: 10.1016/j.cmet.2020.04.021
19. Joish VN, Zhou FL, Preblick R, Lin D, Deshpande M, Verma S, et al. Estimation of Annual Health Care Costs for Adults With Type 1 Diabetes in the United States. J Manag Care Spec Pharm (2020) 26(3):311–8. doi: 10.18553/jmcp.2020.26.3.311
20. Katsarou A, Gudbjornsdottir S, Rawshani A, Dabelea D, Bonifacio E, Anderson BJ, et al. Type 1 Diabetes Mellitus. Nat Rev Dis Primers (2017) 3:17016. doi: 10.1038/nrdp.2017.16
21. Soleimanpour SA, Stoffers DA. The Pancreatic Beta Cell and Type 1 Diabetes: Innocent Bystander or Active Participant? Trends Endocrinol Metab (2013) 24(7):324–31. doi: 10.1016/j.tem.2013.03.005
22. DiMeglio LA, Evans-Molina C, Oram RA. Type 1 Diabetes. Lancet (2018) 391(10138):2449–62. doi: 10.1016/S0140-6736(18)31320-5
23. A. American Diabetes. Economic Costs of Diabetes in the US In 2017. Diabetes Care (2018) 41(5):917–28. doi: 10.2337/dci18-0007
24. Garyu JW, Meffre E, Cotsapas C, Herold KC. Progress and Challenges for Treating Type 1 Diabetes. J Autoimmun (2016) 71:1–9. doi: 10.1016/j.jaut.2016.04.004
25. Rickels MR, Robertson RP. Pancreatic Islet Transplantation in Humans: Recent Progress and Future Directions. Endocr Rev (2019) 40(2):631–68. doi: 10.1210/er.2018-00154
26. Nakamura T, Fujikura J, Anazawa T, Ito R, Ogura M, Okajima H, et al. Long-Term Outcome of Islet Transplantation on Insulin-Dependent Diabetes Mellitus: An Observational Cohort Study. J Diabetes Investig (2020) 11(2):363–72. doi: 10.1111/jdi.13128
27. Ricordi C, Tzakis AG, Carroll PB, Zeng YJ, Rilo HL, Alejandro R, et al. Human Islet Isolation and Allotransplantation in 22 Consecutive Cases. Transplantation (1992) 53(2):407–14. doi: 10.1097/00007890-199202010-00027
28. Marzorati S, Pileggi A, Ricordi C. Allogeneic Islet Transplantation. Expert Opin Biol Ther (2007) 7(11):1627–45. doi: 10.1517/14712598.7.11.1627
29. Vrochides D, Paraskevas S, Papanikolaou V. Transplantation for Type 1 Diabetes Mellitus. Whole Organ or Islets? Hippokratia (2009) 13(1):6–8.
30. Hering BJ, Kandaswamy R, Ansite JD, Eckman PM, Nakano M, Sawada T, et al. Single-Donor, Marginal-Dose Islet Transplantation in Patients With Type 1 Diabetes. JAMA (2005) 293(7):830–5. doi: 10.1001/jama.293.7.830
31. Hering BJ, Clarke WR, Bridges ND, Eggerman TL, Alejandro R, Bellin MD, et al. Phase 3 Trial of Transplantation of Human Islets in Type 1 Diabetes Complicated by Severe Hypoglycemia. Diabetes Care (2016) 39(7):1230–40. doi: 10.2337/dc15-1988
32. Bottino R, Knoll MF, Knoll CA, Bertera S, Trucco MM. The Future of Islet Transplantation Is Now. Front Med (Lausanne) (2018) 5:202. doi: 10.3389/fmed.2018.00202
33. Chang CA, Lawrence MC, Naziruddin B. Current Issues in Allogeneic Islet Transplantation. Curr Opin Organ Transplant (2017) 22(5):437–43. doi: 10.1097/MOT.0000000000000448
34. Hilbrands R, Huurman VA, Gillard P, Velthuis JH, De Waele M, Mathieu C, et al. Differences in Baseline Lymphocyte Counts and Autoreactivity are Associated With Differences in Outcome of Islet Cell Transplantation in Type 1 Diabetic Patients. Diabetes (2009) 58(10):2267–76. doi: 10.2337/db09-0160
35. Moberg L, Johansson H, Lukinius A, Berne C, Foss A, Kallen R, et al. Production of Tissue Factor by Pancreatic Islet Cells as a Trigger of Detrimental Thrombotic Reactions in Clinical Islet Transplantation. Lancet (2002) 360(9350):2039–45. doi: 10.1016/s0140-6736(02)12020-4
36. Thomson JA, Itskovitz-Eldor J, Shapiro SS, Waknitz MA, Swiergiel JJ, Marshall VS, et al. Embryonic Stem Cell Lines Derived From Human Blastocysts. Science (1998) 282(5391):1145–7. doi: 10.1126/science.282.5391.1145
37. Takahashi K, Tanabe K, Ohnuki M, Narita M, Ichisaka T, Tomoda K, et al. Induction of Pluripotent Stem Cells From Adult Human Fibroblasts by Defined Factors. Cell (2007) 131(5):861–72. doi: 10.1016/j.cell.2007.11.019
38. Melton D. The Promise of Stem Cell-Derived Islet Replacement Therapy. Diabetologia (2021) 64(5):1030–6. doi: 10.1007/s00125-020-05367-2
39. D’Amour KA, Agulnick AD, Eliazer S, Kelly OG, Kroon E, Baetge EE. Efficient Differentiation of Human Embryonic Stem Cells to Definitive Endoderm. Nat Biotechnol (2005) 23(12):1534–41. doi: 10.1038/nbt1163
40. D’Amour KA, Bang AG, Eliazer S, Kelly OG, Agulnick AD, Smart NG, et al. Production of Pancreatic Hormone-Expressing Endocrine Cells From Human Embryonic Stem Cells. Nat Biotechnol (2006) 24(11):1392–401. doi: 10.1038/nbt1259
41. Cho YM, Lim JM, Yoo DH, Kim JH, Chung SS, Park SG, et al. Betacellulin and Nicotinamide Sustain PDX1 Expression and Induce Pancreatic Beta-Cell Differentiation in Human Embryonic Stem Cells. Biochem Biophys Res Commun (2008) 366(1):129–34. doi: 10.1016/j.bbrc.2007.11.112
42. Zhang D, Jiang W, Liu M, Sui X, Yin X, Chen S, et al. Highly Efficient Differentiation of Human ES Cells and Ips Cells Into Mature Pancreatic Insulin-Producing Cells. Cell Res (2009) 19(4):429–38. doi: 10.1038/cr.2009.28
43. Chen S, Borowiak M, Fox JL, Maehr R, Osafune K, Davidow L, et al. A Small Molecule That Directs Differentiation of Human Escs Into the Pancreatic Lineage. Nat Chem Biol (2009) 5(4):258–65. doi: 10.1038/nchembio.154
44. Mfopou JK, Chen B, Mateizel I, Sermon K, Bouwens L. Noggin, Retinoids, and Fibroblast Growth Factor Regulate Hepatic or Pancreatic Fate of Human Embryonic Stem Cells. Gastroenterology (2010) 138(7):2233–45, 2245 e1-14. doi: 10.1053/j.gastro.2010.02.056
45. Kunisada Y, Tsubooka-Yamazoe N, Shoji M, Hosoya M. Small Molecules Induce Efficient Differentiation Into Insulin-Producing Cells From Human Induced Pluripotent Stem Cells. Stem Cell Res (2012) 8(2):274–84. doi: 10.1016/j.scr.2011.10.002
46. Rezania A, Bruin JE, Riedel MJ, Mojibian M, Asadi A, Xu J, et al. Maturation of Human Embryonic Stem Cell-Derived Pancreatic Progenitors Into Functional Islets Capable of Treating Pre-Existing Diabetes in Mice. Diabetes (2012) 61(8):2016–29. doi: 10.2337/db11-1711
47. Rezania A, Bruin JE, Xu J, Narayan K, Fox JK, O’Neil JJ, et al. Enrichment of Human Embryonic Stem Cell-Derived NKX6.1-Expressing Pancreatic Progenitor Cells Accelerates the Maturation of Insulin-Secreting Cells in Vivo. Stem Cells (2013) 31(11):2432–42. doi: 10.1002/stem.1489
48. Bruin JE, Erener S, Vela J, Hu X, Johnson JD, Kurata HT, et al. Characterization of Polyhormonal Insulin-Producing Cells Derived in Vitro From Human Embryonic Stem Cells. Stem Cell Res (2014) 12(1):194–208. doi: 10.1016/j.scr.2013.10.003
49. Rajagopal J, Anderson WJ, Kume S, Martinez OI, Melton DA. Insulin Staining of ES Cell Progeny From Insulin Uptake. Science (2003) 299(5605):363. doi: 10.1126/science.1077838
50. Hrvatin S, O’Donnell CW, Deng F, Millman JR, Pagliuca FW, DiIorio P, et al. Differentiated Human Stem Cells Resemble Fetal, Not Adult, Beta Cells. Proc Natl Acad Sci USA (2014) 111(8):3038–43. doi: 10.1073/pnas.1400709111
51. Xie R, Everett LJ, Lim HW, Patel NA, Schug J, Kroon E, et al. Dynamic Chromatin Remodeling Mediated by Polycomb Proteins Orchestrates Pancreatic Differentiation of Human Embryonic Stem Cells. Cell Stem Cell (2013) 12(2):224–37. doi: 10.1016/j.stem.2012.11.023
52. Pagliuca FW, Millman JR, Gurtler M, Segel M, Van Dervort A, Ryu JH, et al. Generation of Functional Human Pancreatic Beta Cells in Vitro. Cell (2014) 159(2):428–39. doi: 10.1016/j.cell.2014.09.040
53. Rezania A, Bruin JE, Arora P, Rubin A, Batushansky I, Asadi A, et al. Reversal of Diabetes With Insulin-Producing Cells Derived in Vitro From Human Pluripotent Stem Cells. Nat Biotechnol (2014) 32(11):1121–33. doi: 10.1038/nbt.3033
54. Russ HA, Parent AV, Ringler JJ, Hennings TG, Nair GG, Shveygert M, et al. Controlled Induction of Human Pancreatic Progenitors Produces Functional Beta-Like Cells in Vitro. EMBO J (2015) 34(13):1759–72. doi: 10.15252/embj.201591058
55. Hang Y, Stein R. Mafa and Mafb Activity in Pancreatic Beta Cells. Trends Endocrinol Metab (2011) 22(9):364–73. doi: 10.1016/j.tem.2011.05.003
56. Aguayo-Mazzucato C, Koh A, El Khattabi I, Li WC, Toschi E, Jermendy A, et al. Mafa Expression Enhances Glucose-Responsive Insulin Secretion in Neonatal Rat Beta Cells. Diabetologia (2011) 54(3):583–93. doi: 10.1007/s00125-010-2026-z
57. Blum B, Hrvatin S, Schuetz C, Bonal C, Rezania A, Melton DA. Functional Beta-Cell Maturation Is Marked by an Increased Glucose Threshold and by Expression of Urocortin 3. Nat Biotechnol (2012) 30(3):261–4. doi: 10.1038/nbt.2141
58. van der Meulen T, Xie R, Kelly OG, Vale WW, Sander M, Huising MO. Urocortin 3 Marks Mature Human Primary and Embryonic Stem Cell-Derived Pancreatic Alpha and Beta Cells. PloS One (2012) 7(12):e52181. doi: 10.1371/journal.pone.0052181
59. Arda HE, Li L, Tsai J, Torre EA, Rosli Y, Peiris H, et al. Age-Dependent Pancreatic Gene Regulation Reveals Mechanisms Governing Human Beta Cell Function. Cell Metab (2016) 23(5):909–20. doi: 10.1016/j.cmet.2016.04.002
60. Veres A, Faust AL, Bushnell HL, Engquist EN, Kenty JH, Harb G, et al. Charting Cellular Identity During Human in Vitro Beta-Cell Differentiation. Nature (2019) 569(7756):368–73. doi: 10.1038/s41586-019-1168-5
61. Velazco-Cruz L, Goedegebuure MM, Maxwell KG, Augsornworawat P, Hogrebe NJ, Millman JR. SIX2 Regulates Human Beta Cell Differentiation From Stem Cells and Functional Maturation in Vitro. Cell Rep (2020) 31(8):107687. doi: 10.1016/j.celrep.2020.107687
62. Augsornworawat P, Maxwell KG, Velazco-Cruz L, Millman JR. Single-Cell Transcriptome Profiling Reveals Beta Cell Maturation in Stem Cell-Derived Islets After Transplantation. Cell Rep (2020) 32(8):108067. doi: 10.1016/j.celrep.2020.108067
63. Bevacqua RJ, Lam JY, Peiris H, Whitener RL, Kim S, Gu X, et al. SIX2 and SIX3 Coordinately Regulate Functional Maturity and Fate of Human Pancreatic Beta Cells. Genes Dev (2021) 35(3-4):234–49. doi: 10.1101/gad.342378.120
64. Martin CC, Flemming BP, Wang Y, Oeser JK, O’Brien RM. Foxa2 and MafA Regulate Islet-Specific Glucose-6-Phosphatase Catalytic Subunit-Related Protein Gene Expression. J Mol Endocrinol (2008) 41(5):315–28. doi: 10.1677/JME-08-0062
65. Artner I, Hang Y, Mazur M, Yamamoto T, Guo M, Lindner J, et al. MafA and MafB Regulate Genes Critical to Beta-Cells in a Unique Temporal Manner. Diabetes (2010) 59(10):2530–9. doi: 10.2337/db10-0190
66. Boortz KA, Syring KE, Lee RA, Dai C, Oeser JK, McGuinness OP, et al. G6PC2 Modulates the Effects of Dexamethasone on Fasting Blood Glucose and Glucose Tolerance. Endocrinology (2016) 157(11):4133–45. doi: 10.1210/en.2016-1678
67. Prentki M, Matschinsky FM, Madiraju SR. Metabolic Signaling in Fuel-Induced Insulin Secretion. Cell Metab (2013) 18(2):162–85. doi: 10.1016/j.cmet.2013.05.018
68. Campbell JE, Newgard CB. Mechanisms Controlling Pancreatic Islet Cell Function in Insulin Secretion. Nat Rev Mol Cell Biol (2021) 22(2):142–58. doi: 10.1038/s41580-020-00317-7
69. Schuit F, De Vos A, Farfari S, Moens K, Pipeleers D, Brun T, et al. Metabolic Fate of Glucose in Purified Islet Cells. Glucose-Regulated Anaplerosis in Beta Cells. J Biol Chem (1997) 272(30):18572–9. doi: 10.1074/jbc.272.30.18572
70. Espinosa de los M, Driscoll SG, Steinke J. Insulin Release From Isolated Human Fetal Pancreatic Islets. Science (1970) 168(3935):1111–2. doi: 10.1126/science.168.3935.1111
71. Rorsman P, Arkhammar P, Bokvist K, Hellerstrom C, Nilsson T, Welsh M, et al. Failure of Glucose to Elicit a Normal Secretory Response in Fetal Pancreatic Beta Cells Results From Glucose Insensitivity of the ATP-Regulated K+ Channels. Proc Natl Acad Sci USA (1989) 86(12):4505–9. doi: 10.1073/pnas.86.12.4505
72. Hellerstrom C, Swenne I. Functional Maturation and Proliferation of Fetal Pancreatic Beta-Cells. Diabetes (1991) 40(Suppl 2):89–93. doi: 10.2337/diab.40.2.s89
73. Carvalho CP, Barbosa HC, Britan A, Santos-Silva JC, Boschero AC, Meda P, et al. Beta Cell Coupling and Connexin Expression Change During the Functional Maturation of Rat Pancreatic Islets. Diabetologia (2010) 53(7):1428–37. doi: 10.1007/s00125-010-1726-8
74. Dhawan S, Tschen SI, Zeng C, Guo T, Hebrok M, Matveyenko A, et al. DNA Methylation Directs Functional Maturation of Pancreatic Beta Cells. J Clin Invest (2015) 125(7):2851–60. doi: 10.1172/JCI79956
75. Jacovetti C, Matkovich SJ, Rodriguez-Trejo A, Guay C, Regazzi R. Postnatal Beta-Cell Maturation is Associated With Islet-Specific Microrna Changes Induced by Nutrient Shifts at Weaning. Nat Commun (2015) 6:8084. doi: 10.1038/ncomms9084
76. Stolovich-Rain M, Enk J, Vikesa J, Nielsen FC, Saada A, Glaser B, et al. Weaning Triggers a Maturation Step of Pancreatic Beta Cells. Dev Cell (2015) 32(5):535–45. doi: 10.1016/j.devcel.2015.01.002
77. Yoshihara E, Wei Z, Lin CS, Fang S, Ahmadian M, Kida Y, et al. Errgamma is Required for the Metabolic Maturation of Therapeutically Functional Glucose-Responsive Beta Cells. Cell Metab (2016) 23(4):622–34. doi: 10.1016/j.cmet.2016.03.005
78. Bader E, Migliorini A, Gegg M, Moruzzi N, Gerdes J, Roscioni SS, et al. Identification of Proliferative and Mature Beta-Cells in the Islets of Langerhans. Nature (2016) 535(7612):430–4. doi: 10.1038/nature18624
79. Wortham M, Benthuysen JR, Wallace M, Savas JN, Mulas F, Divakaruni AS, et al. Integrated In Vivo Quantitative Proteomics and Nutrient Tracing Reveals Age-Related Metabolic Rewiring of Pancreatic Beta Cell Function. Cell Rep (2018) 25(10):2904–18.e8. doi: 10.1016/j.celrep.2018.11.031
80. Helman A, Cangelosi AL, Davis JC, Pham Q, Rothman A, Faust AL, et al. A Nutrient-Sensing Transition at Birth Triggers Glucose-Responsive Insulin Secretion. Cell Metab (2020) 31(5):1004–16.e5. doi: 10.1016/j.cmet.2020.04.004
81. Takebe T, Sekine K, Enomura M, Koike H, Kimura M, Ogaeri T, et al. Vascularized and Functional Human Liver From an Ipsc-Derived Organ Bud Transplant. Nature (2013) 499(7459):481–4. doi: 10.1038/nature12271
82. Camp JG, Sekine K, Gerber T, Loeffler-Wirth H, Binder H, Gac M, et al. Multilineage Communication Regulates Human Liver Bud Development From Pluripotency. Nature (2017) 546(7659):533–8. doi: 10.1038/nature22796
83. Yoshihara E, O’Connor C, Gasser E, Wei Z, Oh TG, Tseng TW, et al. Immune-Evasive Human Islet-Like Organoids Ameliorate Diabetes. Nature (2020) 586(7830):606–11. doi: 10.1038/s41586-020-2631-z
84. Nair GG, Liu JS, Russ HA, Tran S, Saxton MS, Chen R, et al. Recapitulating Endocrine Cell Clustering in Culture Promotes Maturation of Human Stem-Cell-Derived Beta Cells. Nat Cell Biol (2019) 21(2):263–74. doi: 10.1038/s41556-018-0271-4
85. Alvarez-Dominguez JR, Donaghey J, Rasouli N, Kenty JHR, Helman A, Charlton J, et al. Circadian Entrainment Triggers Maturation of Human In Vitro Islets. Cell Stem Cell (2020) 26(1):108–22.e10. doi: 10.1016/j.stem.2019.11.011
86. Davis JC, Alves TC, Helman A, Chen JC, Kenty JH, Cardone RL, et al. Glucose Response by Stem Cell-Derived Beta Cells In Vitro Is Inhibited by a Bottleneck in Glycolysis. Cell Rep (2020) 31(6):107623. doi: 10.1016/j.celrep.2020.107623
87. Velazco-Cruz L, Song J, Maxwell KG, Goedegebuure MM, Augsornworawat P, Hogrebe NJ, et al. Acquisition of Dynamic Function in Human Stem Cell-Derived Beta Cells. Stem Cell Rep (2019) 12(2):351–65. doi: 10.1016/j.stemcr.2018.12.012
88. Borden P, Houtz J, Leach SD, Kuruvilla R. Sympathetic Innervation During Development Is Necessary for Pancreatic Islet Architecture and Functional Maturation. Cell Rep (2013) 4(2):287–301. doi: 10.1016/j.celrep.2013.06.019
89. Sneddon JB, Borowiak M, Melton DA. Self-Renewal of Embryonic-Stem-Cell-Derived Progenitors by Organ-Matched Mesenchyme. Nature (2012) 491(7426):765–8. doi: 10.1038/nature11463
90. Kroon E, Martinson LA, Kadoya K, Bang AG, Kelly OG, Eliazer S, et al. Pancreatic Endoderm Derived From Human Embryonic Stem Cells Generates Glucose-Responsive Insulin-Secreting Cells in Vivo. Nat Biotechnol (2008) 26(4):443–52. doi: 10.1038/nbt1393
91. Memon B, Abdelalim EM. Stem Cell Therapy for Diabetes: Beta Cells Versus Pancreatic Progenitors. Cells (2020) 9(2):283. doi: 10.3390/cells9020283
92. Augsornworawat P, Maxwell KG, Velazco-Cruz L, Millman JR. Single-Cell Transcriptome Profiling Reveals Beta Cell Maturation in Stem Cell-Derived Islets After Transplantation. Cell Rep (2021) 34(10):108850. doi: 10.1016/j.celrep.2021.108850
93. Saber N, Bruin JE, O’Dwyer S, Schuster H, Rezania A, Kieffer TJ. Sex Differences in Maturation of Human Embryonic Stem Cell-Derived Beta Cells in Mice. Endocrinology (2018) 159(4):1827–41. doi: 10.1210/en.2018-00048
94. Bruin JE, Asadi A, Fox JK, Erener S, Rezania A, Kieffer TJ. Accelerated Maturation of Human Stem Cell-Derived Pancreatic Progenitor Cells Into Insulin-Secreting Cells in Immunodeficient Rats Relative to Mice. Stem Cell Rep (2015) 5(6):1081–96. doi: 10.1016/j.stemcr.2015.10.013
95. Wortham M, Sander M. Transcriptional Mechanisms of Pancreatic β-Cell Maturation and Functional Adaptation. Trends Endocrinol Metab (2021) 32(7):474–487. doi: 10.1016/j.tem.2021.04.011
96. Farney AC, Sutherland DE, Opara EC. Evolution of Islet Transplantation for the Last 30 Years. Pancreas (2016) 45(1):8–20. doi: 10.1097/MPA.0000000000000391
97. Gruessner RW. Tacrolimus in Pancreas Transplantation: A Multicenter Analysis. Tacrolimus Pancreas Transplant Study Group. Clin Transplant (1997) 11(4):299–312.
98. Nieto Y, Russ P, Everson G, Bearman SI, Cagnoni PJ, Jones RB, et al. Acute Pancreatitis During Immunosuppression With Tacrolimus Following an Allogeneic Umbilical Cord Blood Transplantation. Bone Marrow Transplant (2000) 26(1):109–11. doi: 10.1038/sj.bmt.1702471
99. Rangel EB. The Metabolic and Toxicological Considerations for Immunosuppressive Drugs Used During Pancreas Transplantation. Expert Opin Drug Metab Toxicol (2012) 8(12):1531–48. doi: 10.1517/17425255.2012.724058
100. Krautz C, Wolk S, Steffen A, Knoch KP, Ceglarek U, Thiery J, et al. Effects of Immunosuppression on Alpha and Beta Cell Renewal in Transplanted Mouse Islets. Diabetologia (2013) 56(7):1596–604. doi: 10.1007/s00125-013-2895-z
101. Bonaventura A, Montecucco F. Steroid-Induced Hyperglycemia: An Underdiagnosed Problem or Clinical Inertia? A Narrative Review. Diabetes Res Clin Pract (2018) 139:203–20. doi: 10.1016/j.diabres.2018.03.006
102. Srinivas TR, Meier-Kriesche HU. Minimizing Immunosuppression, an Alternative Approach to Reducing Side Effects: Objectives and Interim Result. Clin J Am Soc Nephrol (2008) 3(Suppl 2):S101–16. doi: 10.2215/CJN.03510807
103. Shapiro AM, Lakey JR, Ryan EA, Korbutt GS, Toth E, Warnock GL, et al. Islet Transplantation in Seven Patients With Type 1 Diabetes Mellitus Using a Glucocorticoid-Free Immunosuppressive Regimen. N Engl J Med (2000) 343(4):230–8. doi: 10.1056/NEJM200007273430401
104. Tekin Z, Garfinkel MR, Chon WJ, Schenck L, Golab K, Savari O, et al. Outcomes of Pancreatic Islet Allotransplantation Using the Edmonton Protocol at the University of Chicago. Transplant Direct (2016) 2(10):e105. doi: 10.1097/TXD.0000000000000609
105. Florkowski C. Hba1c as a Diagnostic Test for Diabetes Mellitus - Reviewing the Evidence. Clin Biochem Rev (2013) 34(2):75–83.
106. Hering BJ, Kandaswamy R, Harmon JV, Ansite JD, Clemmings SM, Sakai T, et al. Transplantation of Cultured Islets From Two-Layer Preserved Pancreases in Type 1 Diabetes With Anti-CD3 Antibody. Am J Transplant (2004) 4(3):390–401. doi: 10.1046/j.1600-6143.2003.00351.x
107. Bellin MD, Barton FB, Heitman A, Harmon JV, Kandaswamy R, Balamurugan AN, et al. Potent Induction Immunotherapy Promotes Long-Term Insulin Independence After Islet Transplantation in Type 1 Diabetes. Am J Transplant (2012) 12(6):1576–83. doi: 10.1111/j.1600-6143.2011.03977.x
108. Froud T, Baidal DA, Faradji R, Cure P, Mineo D, Selvaggi G, et al. Islet Transplantation With Alemtuzumab Induction and Calcineurin-Free Maintenance Immunosuppression Results in Improved Short- and Long-Term Outcomes. Transplantation (2008) 86(12):1695–701. doi: 10.1097/TP.0b013e31819025e5
109. Posselt AM, Szot GL, Frassetto LA, Masharani U, Tavakol M, Amin R, et al. Islet Transplantation in Type 1 Diabetic Patients Using Calcineurin Inhibitor-Free Immunosuppressive Protocols Based on T-Cell Adhesion or Costimulation Blockade. Transplantation (2010) 90(12):1595–601. doi: 10.1097/TP.0b013e3181fe1377
110. Kim JM, Shin JS, Min BH, Kang SJ, Yoon IH, Chung H, et al. JAK3 Inhibitor-Based Immunosuppression in Allogeneic Islet Transplantation in Cynomolgus Monkeys. Islets (2019) 11(5):119–28. doi: 10.1080/19382014.2019.1650580
111. Ingulli E. Mechanism of Cellular Rejection in Transplantation. Pediatr Nephrol (2010) 25(1):61–74. doi: 10.1007/s00467-008-1020-x
112. Josephs SF, Loudovaris T, Dixit A, Young SK, Johnson RC. In Vivo Delivery of Recombinant Human Growth Hormone From Genetically Engineered Human Fibroblasts Implanted Within Baxter Immunoisolation Devices. J Mol Med (Berl) (1999) 77(1):211–4. doi: 10.1007/s001090050338
113. Kumagai-Braesch M, Jacobson S, Mori H, Jia X, Takahashi T, Wernerson A, et al. The Theracyte Device Protects Against Islet Allograft Rejection in Immunized Hosts. Cell Transplant (2013) 22(7):1137–46. doi: 10.3727/096368912X657486
114. Motte E, Szepessy E, Suenens K, Stange G, Bomans M, Jacobs-Tulleneers-Thevissen D, et al. Composition and Function of Macroencapsulated Human Embryonic Stem Cell-Derived Implants: Comparison With Clinical Human Islet Cell Grafts. Am J Physiol Endocrinol Metab (2014) 307(9):E838–46. doi: 10.1152/ajpendo.00219.2014
115. Boettler T, Schneider D, Cheng Y, Kadoya K, Brandon EP, Martinson L, et al. Pancreatic Tissue Transplanted in Theracyte Encapsulation Devices Is Protected and Prevents Hyperglycemia in a Mouse Model of Immune-Mediated Diabetes. Cell Transplant (2016) 25(3):609–14. doi: 10.3727/096368915X688939
116. Henry RR PJ, Wilensky JON, Shapiro AMJ, Senior PA, Roep B, Wang R, et al. Initial Clinical Evaluation of VC-01TM Combination Product-a Stem Cell-Derived Islet Replacement for Type 1 Diabetes (T1D). Diabetes (2018) 67(Supplement 1). doi: 10.2337/db18-138-OR
117. Ludwig B, Rotem A, Schmid J, Weir GC, Colton CK, Brendel MD, et al. Improvement of Islet Function in a Bioartificial Pancreas by Enhanced Oxygen Supply and Growth Hormone Releasing Hormone Agonist. Proc Natl Acad Sci USA (2012) 109(13):5022–7. doi: 10.1073/pnas.1201868109
118. Carlsson PO, Espes D, Sedigh A, Rotem A, Zimerman B, Grinberg H, et al. Transplantation of Macroencapsulated Human Islets Within the Bioartificial Pancreas Betaair to Patients With Type 1 Diabetes Mellitus. Am J Transplant (2018) 18(7):1735–44. doi: 10.1111/ajt.14642
119. Wang LH, Ernst AU, Flanders JA, Liu W, Wang X, Datta AK, et al. An Inverse-Breathing Encapsulation System for Cell Delivery. Sci Adv (2021) 7(20):eabd5835. doi: 10.1126/sciadv.abd5835
120. Lim F, Sun AM. Microencapsulated Islets as Bioartificial Endocrine Pancreas. Science (1980) 210(4472):908–10. doi: 10.1126/science.6776628
121. Smidsrod O, Skjak-Braek G. Alginate as Immobilization Matrix for Cells. Trends Biotechnol (1990) 8(3):71–8. doi: 10.1016/0167-7799(90)90139-o
122. Zekorn T, Siebers U, Horcher A, Schnettler R, Zimmermann U, Bretzel RG, et al. Alginate Coating of Islets of Langerhans: In Vitro Studies on a New Method for Microencapsulation for Immuno-Isolated Transplantation. Acta Diabetol (1992) 29(1):41–5. doi: 10.1007/BF00572829
123. Soon-Shiong P, Heintz RE, Merideth N, Yao QX, Yao Z, Zheng T, et al. Insulin Independence in a Type 1 Diabetic Patient After Encapsulated Islet Transplantation. Lancet (1994) 343(8903):950–1. doi: 10.1016/s0140-6736(94)90067-1
124. Duvivier-Kali VF, Omer A, Parent RJ, O’Neil JJ, Weir GC. Complete Protection of Islets Against Allorejection and Autoimmunity by a Simple Barium-Alginate Membrane. Diabetes (2001) 50(8):1698–705. doi: 10.2337/diabetes.50.8.1698
125. de Groot M, Schuurs TA, van Schilfgaarde R. Causes of Limited Survival of Microencapsulated Pancreatic Islet Grafts. J Surg Res (2004) 121(1):141–50. doi: 10.1016/j.jss.2004.02.018
126. Omer A, Duvivier-Kali V, Fernandes J, Tchipashvili V, Colton CK, Weir GC. Long-Term Normoglycemia in Rats Receiving Transplants With Encapsulated Islets. Transplantation (2005) 79(1):52–8. doi: 10.1097/01.tp.0000149340.37865.46
127. Krol S, del Guerra S, Grupillo M, Diaspro A, Gliozzi A, Marchetti P. Multilayer Nanoencapsulation. New Approach for Immune Protection of Human Pancreatic Islets. Nano Lett (2006) 6(9):1933–9. doi: 10.1021/nl061049r
128. Tuch BE, Keogh GW, Williams LJ, Wu W, Foster JL, Vaithilingam V, et al. Safety and Viability of Microencapsulated Human Islets Transplanted Into Diabetic Humans. Diabetes Care (2009) 32(10):1887–9. doi: 10.2337/dc09-0744
129. Lee KY, Mooney DJ. Alginate: Properties and Biomedical Applications. Prog Polym Sci (2012) 37(1):106–26. doi: 10.1016/j.progpolymsci.2011.06.003
130. Jacobs-Tulleneers-Thevissen D, Chintinne M, Ling Z, Gillard P, Schoonjans L, Delvaux G, et al. Sustained Function of Alginate-Encapsulated Human Islet Cell Implants in the Peritoneal Cavity of Mice Leading to a Pilot Study in a Type 1 Diabetic Patient. Diabetologia (2013) 56(7):1605–14. doi: 10.1007/s00125-013-2906-0
131. Scharp DW, Marchetti P. Encapsulated Islets for Diabetes Therapy: History, Current Progress, and Critical Issues Requiring Solution. Adv Drug Delivery Rev (2014) 67-68:35–73. doi: 10.1016/j.addr.2013.07.018
132. Ludwig B, Reichel A, Steffen A, Zimerman B, Schally AV, Block NL, et al. Transplantation of Human Islets Without Immunosuppression. Proc Natl Acad Sci USA (2013) 110(47):19054–8. doi: 10.1073/pnas.1317561110
133. Veiseh O, Doloff JC, Ma M, Vegas AJ, Tam HH, Bader AR, et al. Size- and Shape-Dependent Foreign Body Immune Response to Materials Implanted in Rodents and Non-Human Primates. Nat Mater (2015) 14(6):643–51. doi: 10.1038/nmat4290
134. Vegas AJ, Veiseh O, Gurtler M, Millman JR, Pagliuca FW, Bader AR, et al. Long-Term Glycemic Control Using Polymer-Encapsulated Human Stem Cell-Derived Beta Cells in Immune-Competent Mice. Nat Med (2016) 22(3):306–11. doi: 10.1038/nm.4030
135. Bochenek MA, Veiseh O, Vegas AJ, McGarrigle JJ, Qi M, Marchese E, et al. Alginate Encapsulation as Long-Term Immune Protection of Allogeneic Pancreatic Islet Cells Transplanted Into the Omental Bursa of Macaques. Nat BioMed Eng (2018) 2(11):810–21. doi: 10.1038/s41551-018-0275-1
136. Alagpulinsa DA, Cao JJL, Driscoll RK, Sirbulescu RF, Penson MFE, Sremac M, et al. Alginate-Microencapsulation of Human Stem Cell-Derived Beta Cells With CXCL12 Prolongs Their Survival and Function in Immunocompetent Mice Without Systemic Immunosuppression. Am J Transplant (2019) 19(7):1930–40. doi: 10.1111/ajt.15308
137. Calafiore R, Basta G, Luca G, Lemmi A, Montanucci MP, Calabrese G, et al. Microencapsulated Pancreatic Islet Allografts Into Nonimmunosuppressed Patients With Type 1 Diabetes: First Two Cases. Diabetes Care (2006) 29(1):137–8. doi: 10.2337/diacare.29.1.137
138. Wang T, Adcock J, Kuhtreiber W, Qiang D, Salleng KJ, Trenary I, et al. Successful Allotransplantation of Encapsulated Islets in Pancreatectomized Canines for Diabetic Management Without the Use of Immunosuppression. Transplantation (2008) 85(3):331–7. doi: 10.1097/TP.0b013e3181629c25
139. Basta G, Montanucci P, Luca G, Boselli C, Noya G, Barbaro B, et al. Long-Term Metabolic and Immunological Follow-Up of Nonimmunosuppressed Patients With Type 1 Diabetes Treated With Microencapsulated Islet Allografts: Four Cases. Diabetes Care (2011) 34(11):2406–9. doi: 10.2337/dc11-0731
140. An D, Chiu A, Flanders JA, Song W, Shou D, Lu YC, et al. Designing a Retrievable and Scalable Cell Encapsulation Device for Potential Treatment of Type 1 Diabetes. Proc Natl Acad Sci USA (2018) 115(2):E263–72. doi: 10.1073/pnas.1708806115
141. Wang X, Maxwell KG, Wang K, Bowers DT, Flanders JA, Liu W, et al. A Nanofibrous Encapsulation Device for Safe Delivery of Insulin-Producing Cells to Treat Type 1 Diabetes. Sci Transl Med (2021) 13(596):eabb4601. doi: 10.1126/scitranslmed.abb4601
142. Yin D, Ding JW, Shen J, Ma L, Hara M, Chong AS. Liver Ischemia Contributes to Early Islet Failure Following Intraportal Transplantation: Benefits of Liver Ischemic-Preconditioning. Am J Transplant (2006) 6(1):60–8. doi: 10.1111/j.1600-6143.2005.01157.x
143. Dembinski A, Warzecha Z, Ceranowicz P, Dembinski M, Cieszkowski J, Pawlik WW, et al. Effect of Ischemic Preconditioning on Pancreatic Regeneration and Pancreatic Expression of Vascular Endothelial Growth Factor and Platelet-Derived Growth Factor-a in Ischemia/Reperfusion-Induced Pancreatitis. J Physiol Pharmacol (2006) 58(2):303–19.
144. Tapuria N, Kumar Y, Habib MM, Abu Amara M, Seifalian AM, Davidson BR. Remote Ischemic Preconditioning: A Novel Protective Method From Ischemia Reperfusion Injury–a Review. J Surg Res (2008) 150(2):304–30. doi: 10.1016/j.jss.2007.12.747
145. Saraiva M, O’Garra A. The Regulation of IL-10 Production by Immune Cells. Nat Rev Immunol (2010) 10(3):170–81. doi: 10.1038/nri2711
146. Delaune V, Lacotte S, Gex Q, Slits F, Kahler-Quesada A, Lavallard V, et al. Effects of Remote Ischaemic Preconditioning on Intraportal Islet Transplantation in a Rat Model. Transpl Int (2019) 32(3):323–33. doi: 10.1111/tri.13360
147. Lee JG, Lee JI, Kim JY, Cho Y, Huh KH, Kim BS, et al. Coculture With Ischemia/Reperfusion-Preconditioned Hepatocytes Improves Islet Function and Survival. Transplant Proc (2018) 50(10):3887–94. doi: 10.1016/j.transproceed.2018.07.007
148. Keshtkar S, Kaviani M, Jabbarpour Z, Sabet Sarvestani F, Ghahremani MH, Esfandiari E, et al. Hypoxia-Preconditioned Wharton’s Jelly-Derived Mesenchymal Stem Cells Mitigate Stress-Induced Apoptosis and Ameliorate Human Islet Survival and Function in Direct Contact Coculture System. Stem Cells Int (2020) 2020:8857457. doi: 10.1155/2020/8857457
149. Ma Z, Moruzzi N, Catrina SB, Hals I, Oberholzer J, Grill V, et al. Preconditioning With Associated Blocking of Ca2+ Inflow Alleviates Hypoxia-Induced Damage to Pancreatic Beta-Cells. PloS One (2013) 8(7):e67498. doi: 10.1371/journal.pone.0067498
150. Yamane K, Anazawa T, Tada S, Fujimoto N, Inoguchi K, Emoto N, et al. Mitomycin C Treatment Improves Pancreatic Islet Graft Longevity in Intraportal Islet Transplantation by Suppressing Proinflammatory Response. Sci Rep (2020) 10(1):12086. doi: 10.1038/s41598-020-69009-8
151. Wada Y, Takata A, Ikemoto T, Morine Y, Imura S, Iwahashi S, et al. The Protective Effect of Epigallocatechin 3-Gallate on Mouse Pancreatic Islets via the Nrf2 Pathway. Surg Today (2019) 49(6):536–45. doi: 10.1007/s00595-019-1761-0
152. Tang Q, Bluestone JA. Regulatory T-Cell Therapy in Transplantation: Moving to the Clinic. Cold Spring Harb Perspect Med (2013) 3(11):a015552. doi: 10.1101/cshperspect.a015552
153. Feins S, Kong W, Williams EF, Milone MC, Fraietta JA. An Introduction to Chimeric Antigen Receptor (CAR) T-Cell Immunotherapy for Human Cancer. Am J Hematol (2019) 94(S1):S3–9. doi: 10.1002/ajh.25418
154. Romano M, Fanelli G, Albany CJ, Giganti G, Lombardi G. Past, Present, and Future of Regulatory T Cell Therapy in Transplantation and Autoimmunity. Front Immunol (2019) 10:43. doi: 10.3389/fimmu.2019.00043
155. MacDonald KG, Hoeppli RE, Huang Q, Gillies J, Luciani DS, Orban PC, et al. Alloantigen-Specific Regulatory T Cells Generated With a Chimeric Antigen Receptor. J Clin Invest (2016) 126(4):1413–24. doi: 10.1172/JCI82771
156. Chavez JC, Bachmeier C, Kharfan-Dabaja MA. CAR T-Cell Therapy for B-Cell Lymphomas: Clinical Trial Results of Available Products. Ther Adv Hematol (2019) 10:2040620719841581. doi: 10.1177/2040620719841581
157. Larson RC, Maus MV. Recent Advances and Discoveries in the Mechanisms and Functions of CAR T Cells. Nat Rev Cancer (2021) 21(3):145–61. doi: 10.1038/s41568-020-00323-z
158. Morizane A, Kikuchi T, Hayashi T, Mizuma H, Takara S, Doi H, et al. MHC Matching Improves Engraftment of Ipsc-Derived Neurons in Non-Human Primates. Nat Commun (2017) 8(1):385. doi: 10.1038/s41467-017-00926-5
159. Bloor AJC, Patel A, Griffin JE, Gilleece MH, Radia R, Yeung DT, et al. Production, Safety and Efficacy of Ipsc-Derived Mesenchymal Stromal Cells in Acute Steroid-Resistant Graft Versus Host Disease: A Phase I, Multicenter, Open-Label, Dose-Escalation Study. Nat Med (2020) 26(11):1720–5. doi: 10.1038/s41591-020-1050-x
160. Taylor CJ, Bolton EM, Pocock S, Sharples LD, Pedersen RA, Bradley JA. Banking on Human Embryonic Stem Cells: Estimating the Number of Donor Cell Lines Needed for HLA Matching. Lancet (2005) 366(9502):2019–25. doi: 10.1016/S0140-6736(05)67813-0
161. Nakatsuji N, Nakajima F, Tokunaga K. HLA-Haplotype Banking and Ips Cells. Nat Biotechnol (2008) 26(7):739–40. doi: 10.1038/nbt0708-739
162. Taylor CJ, Peacock S, Chaudhry AN, Bradley JA, Bolton EM. Generating an Ipsc Bank for HLA-Matched Tissue Transplantation Based on Known Donor and Recipient HLA Types. Cell Stem Cell (2012) 11(2):147–52. doi: 10.1016/j.stem.2012.07.014
163. Umekage M, Sato Y, Takasu N. Overview: An iPS Cell Stock at CiRA. Inflamm Regener (2019) 39:17. doi: 10.1186/s41232-019-0106-0
164. Cermak T, Doyle EL, Christian M, Wang L, Zhang Y, Schmidt C, et al. Efficient Design and Assembly of Custom TALEN and Other TAL Effector-Based Constructs for DNA Targeting. Nucleic Acids Res (2011) 39(12):e82. doi: 10.1093/nar/gkr218
165. Zhao H, He L, Li S, Huang H, Tang F, Han X, et al. Generation of Corrected-Hipsc (Ustci001-a-1) From Epilepsy Patient Ipscs Using TALEN-Mediated Editing of the SCN1A Gene. Stem Cell Res (2020) 46:101864. doi: 10.1016/j.scr.2020.101864
166. Wiedenheft B, Zhou K, Jinek M, Coyle SM, Ma W, Doudna JA. Structural Basis for Dnase Activity of a Conserved Protein Implicated in CRISPR-Mediated Genome Defense. Structure (2009) 17(6):904–12. doi: 10.1016/j.str.2009.03.019
167. Haurwitz RE, Jinek M, Wiedenheft B, Zhou K, Doudna JA. Sequence- and Structure-Specific RNA Processing by a CRISPR Endonuclease. Science (2010) 329(5997):1355–8. doi: 10.1126/science.1192272
168. Gilbert LA, Larson MH, Morsut L, Liu Z, Brar GA, Torres SE, et al. CRISPR-Mediated Modular RNA-Guided Regulation of Transcription in Eukaryotes. Cell (2013) 154(2):442–51. doi: 10.1016/j.cell.2013.06.044
169. Cong L, Ran FA, Cox D, Lin S, Barretto R, Habib N, et al. Multiplex Genome Engineering Using CRISPR/Cas Systems. Science (2013) 339(6121):819–23. doi: 10.1126/science.1231143
170. Ran FA, Hsu PD, Wright J, Agarwala V, Scott DA, Zhang F. Genome Engineering Using the CRISPR-Cas9 System. Nat Protoc (2013) 8(11):2281–308. doi: 10.1038/nprot.2013.143
171. Doudna JA, Charpentier E. Genome Editing. The New Frontier of Genome Engineering With CRISPR-Cas9. Science (2014) 346(6213):1258096. doi: 10.1126/science.1258096
172. Gornalusse GG, Hirata RK, Funk SE, Riolobos L, Lopes VS, Manske G, et al. HLA-E-Expressing Pluripotent Stem Cells Escape Allogeneic Responses and Lysis by NK Cells. Nat Biotechnol (2017) 35(8):765–72. doi: 10.1038/nbt.3860
173. Xu H, Wang B, Ono M, Kagita A, Fujii K, Sasakawa N, et al. Targeted Disruption of HLA Genes via CRISPR-Cas9 Generates Ipscs With Enhanced Immune Compatibility. Cell Stem Cell (2019) 24(4):566–78.e7. doi: 10.1016/j.stem.2019.02.005
174. Han X, Wang M, Duan S, Franco PJ, Kenty JH, Hedrick P, et al. Generation of Hypoimmunogenic Human Pluripotent Stem Cells. Proc Natl Acad Sci USA (2019) 116(21):10441–6. doi: 10.1073/pnas.1902566116
175. Keenan HA, Sun JK, Levine J, Doria A, Aiello LP, Eisenbarth G, et al. Residual Insulin Production and Pancreatic Ss-Cell Turnover After 50 Years of Diabetes: Joslin Medalist Study. Diabetes (2010) 59(11):2846–53. doi: 10.2337/db10-0676
176. Teta M, Long SY, Wartschow LM, Rankin MM, Kushner JA. Very Slow Turnover of Beta-Cells in Aged Adult Mice. Diabetes (2005) 54(9):2557–67. doi: 10.2337/diabetes.54.9.2557
177. Cnop M, Hughes SJ, Igoillo-Esteve M, Hoppa MB, Sayyed F, van de Laar L, et al. The Long Lifespan and Low Turnover of Human Islet Beta Cells Estimated by Mathematical Modelling of Lipofuscin Accumulation. Diabetologia (2010) 53(2):321–30. doi: 10.1007/s00125-009-1562-x
178. Perl S, Kushner JA, Buchholz BA, Meeker AK, Stein GM, Hsieh M, et al. Significant Human Beta-Cell Turnover is Limited to the First Three Decades of Life as Determined by in Vivo Thymidine Analog Incorporation and Radiocarbon Dating. J Clin Endocrinol Metab (2010) 95(10):E234–9. doi: 10.1210/jc.2010-0932
179. Iwai Y, Ishida M, Tanaka Y, Okazaki T, Honjo T, Minato N. Involvement of PD-L1 on Tumor Cells in the Escape From Host Immune System and Tumor Immunotherapy by PD-L1 Blockade. Proc Natl Acad Sci USA (2002) 99(19):12293–7. doi: 10.1073/pnas.192461099
180. Rui J, Deng S, Arazi A, Perdigoto AL, Liu Z, Herold KC. Beta Cells That Resist Immunological Attack Develop During Progression of Autoimmune Diabetes in NOD Mice. Cell Metab (2017) 25(3):727–38. doi: 10.1016/j.cmet.2017.01.005
181. Freeman GJ, Long AJ, Iwai Y, Bourque K, Chernova T, Nishimura H, et al. Engagement of the PD-1 Immunoinhibitory Receptor by a Novel B7 Family Member Leads to Negative Regulation of Lymphocyte Activation. J Exp Med (2000) 192(7):1027–34. doi: 10.1084/jem.192.7.1027
182. Ansari MJ, Salama AD, Chitnis T, Smith RN, Yagita H, Akiba H, et al. The Programmed Death-1 (PD-1) Pathway Regulates Autoimmune Diabetes in Nonobese Diabetic (NOD) Mice. J Exp Med (2003) 198(1):63–9. doi: 10.1084/jem.20022125
183. Keir ME, Liang SC, Guleria I, Latchman YE, Qipo A, Albacker LA, et al. Tissue Expression of PD-L1 Mediates Peripheral T Cell Tolerance. J Exp Med (2006) 203(4):883–95. doi: 10.1084/jem.20051776
184. Colli ML, Hill JLE, Marroqui L, Chaffey J, Dos Santos RS, Leete P, et al. PDL1 is Expressed in the Islets of People With Type 1 Diabetes and Is Up-Regulated by Interferons-Alpha and-Gamma via IRF1 Induction. EBioMedicine (2018) 36:367–75. doi: 10.1016/j.ebiom.2018.09.040
185. Osum KC, Burrack AL, Martinov T, Sahli NL, Mitchell JS, Tucker CG, et al. Interferon-Gamma Drives Programmed Death-Ligand 1 Expression on Islet Beta Cells to Limit T Cell Function During Autoimmune Diabetes. Sci Rep (2018) 8(1):8295. doi: 10.1038/s41598-018-26471-9
186. Ma D, Duan W, Li Y, Wang Z, Li S, Gong N, et al. PD-L1 Deficiency Within Islets Reduces Allograft Survival in Mice. PloS One (2016) 11(3):e0152087. doi: 10.1371/journal.pone.0152087
187. Ma H, Jeppesen JF, Jaenisch R. Human T Cells Expressing a CD19 CAR-T Receptor Provide Insights Into Mechanisms of Human CD19-Positive Beta Cell Destruction. Cell Rep Med (2020) 1(6):100097. doi: 10.1016/j.xcrm.2020.100097
188. Batra L, Shrestha P, Zhao H, Woodward KB, Togay A, Tan M, et al. Localized Immunomodulation With PD-L1 Results in Sustained Survival and Function of Allogeneic Islets Without Chronic Immunosuppression. J Immunol (2020) 204(10):2840–51. doi: 10.4049/jimmunol.2000055
189. Coronel MM, Martin KE, Hunckler MD, Barber G, O’Neill EB, Medina JD, et al. Immunotherapy via PD-L1-Presenting Biomaterials Leads to Long-Term Islet Graft Survival. Sci Adv (2020) 6(35):eaba5573. doi: 10.1126/sciadv.aba5573
190. Donath MY, Dalmas E, Sauter NS, Boni-Schnetzler M. Inflammation in Obesity and Diabetes: Islet Dysfunction and Therapeutic Opportunity. Cell Metab (2013) 17(6):860–72. doi: 10.1016/j.cmet.2013.05.001
191. Berchtold LA, Prause M, Storling J, Mandrup-Poulsen T. Cytokines and Pancreatic Beta-Cell Apoptosis. Adv Clin Chem (2016) 75:99–158. doi: 10.1016/bs.acc.2016.02.001
192. Qaisar N, Jurczyk A, Wang JP. Potential Role of Type I Interferon in the Pathogenic Process Leading to Type 1 Diabetes. Curr Opin Endocrinol Diabetes Obes (2018) 25(2):94–100. doi: 10.1097/MED.0000000000000399
193. Lau CM, Adams NM, Geary CD, Weizman OE, Rapp M, Pritykin Y, et al. Epigenetic Control of Innate and Adaptive Immune Memory. Nat Immunol (2018) 19(9):963–72. doi: 10.1038/s41590-018-0176-1
194. Wendeln AC, Degenhardt K, Kaurani L, Gertig M, Ulas T, Jain G, et al. Innate Immune Memory in the Brain Shapes Neurological Disease Hallmarks. Nature (2018) 556(7701):332–8. doi: 10.1038/s41586-018-0023-4
195. Naik S, Larsen SB, Gomez NC, Alaverdyan K, Sendoel A, Yuan S, et al. Inflammatory Memory Sensitizes Skin Epithelial Stem Cells to Tissue Damage. Nature (2017) 550(7677):475–80. doi: 10.1038/nature24271
196. Ordovas-Montanes J, Dwyer DF, Nyquist SK, Buchheit KM, Vukovic M, Deb C, et al. Allergic Inflammatory Memory in Human Respiratory Epithelial Progenitor Cells. Nature (2018) 560(7720):649–54. doi: 10.1038/s41586-018-0449-8
197. Rezania A, Riedel MJ, Wideman RD, Karanu F, Ao Z, Warnock GL, et al. Production of Functional Glucagon-Secreting Alpha-Cells From Human Embryonic Stem Cells. Diabetes (2011) 60(1):239–47. doi: 10.2337/db10-0573
198. Peterson QP, Veres A, Chen L, Slama MQ, Kenty JHR, Hassoun S, et al. A Method for the Generation of Human Stem Cell-Derived Alpha Cells. Nat Commun (2020) 11(1):2241. doi: 10.1038/s41467-020-16049-3
199. Ando M, Nishimura T, Yamazaki S, Yamaguchi T, Kawana-Tachikawa A, Hayama T, et al. A Safeguard System for Induced Pluripotent Stem Cell-Derived Rejuvenated T Cell Therapy. Stem Cell Rep (2015) 5(4):597–608. doi: 10.1016/j.stemcr.2015.07.011
Keywords: diabetes, stem cells, human islet-like organoids, immune evasion, transcriptional memory
Citation: Tahbaz M and Yoshihara E (2021) Immune Protection of Stem Cell-Derived Islet Cell Therapy for Treating Diabetes. Front. Endocrinol. 12:716625. doi: 10.3389/fendo.2021.716625
Received: 28 May 2021; Accepted: 19 July 2021;
Published: 10 August 2021.
Edited by:
Anca Dana Dobrian, Eastern Virginia Medical School, United StatesReviewed by:
Essam M Abdelalim, Qatar Biomedical Research Institute, QatarCopyright © 2021 Tahbaz and Yoshihara. This is an open-access article distributed under the terms of the Creative Commons Attribution License (CC BY). The use, distribution or reproduction in other forums is permitted, provided the original author(s) and the copyright owner(s) are credited and that the original publication in this journal is cited, in accordance with accepted academic practice. No use, distribution or reproduction is permitted which does not comply with these terms.
*Correspondence: Eiji Yoshihara, ZWlqaS55b3NoaWhhcmFAbHVuZHF1aXN0Lm9yZw==
Disclaimer: All claims expressed in this article are solely those of the authors and do not necessarily represent those of their affiliated organizations, or those of the publisher, the editors and the reviewers. Any product that may be evaluated in this article or claim that may be made by its manufacturer is not guaranteed or endorsed by the publisher.
Research integrity at Frontiers
Learn more about the work of our research integrity team to safeguard the quality of each article we publish.