- Section of Cell Biology and Functional Genomics, Department of Metabolism, Digestion and Reproduction, Faculty of Medicine, Imperial College London, London, United Kingdom
Pancreatic β-cells within the islets of Langerhans respond to rising blood glucose levels by secreting insulin that stimulates glucose uptake by peripheral tissues to maintain whole body energy homeostasis. To different extents, failure of β-cell function and/or β-cell loss contribute to the development of Type 1 and Type 2 diabetes. Chronically elevated glycaemia and high circulating free fatty acids, as often seen in obese diabetics, accelerate β-cell failure and the development of the disease. MiRNAs are essential for endocrine development and for mature pancreatic β-cell function and are dysregulated in diabetes. In this review, we summarize the different molecular mechanisms that control miRNA expression and function, including transcription, stability, posttranscriptional modifications, and interaction with RNA binding proteins and other non-coding RNAs. We also discuss which of these mechanisms are responsible for the nutrient-mediated regulation of the activity of β-cell miRNAs and identify some of the more important knowledge gaps in the field.
Introduction
Over 8.5% of the world’s adult population suffer diabetes, which causes annually more than 1.5 million deaths (1). Type 1 diabetes (T1D) is a chronic autoimmune disease that usually leads to a severe loss of insulin-secreting pancreatic β-cells, whereas type 2 diabetes (T2D) is generally characterized by a reduced capability of peripheral tissues to respond to insulin and the incapacity of β-cells to secrete enough hormone to compensate for the higher demand. Both forms of the disease, if untreated, result in chronic hyperglycaemia that strongly contributes to the development of cardiovascular, neurological, kidney, and other complications. These result in significant morbidity, a strong reduction in life quality and expectancy, and colossal costs to health services (1).
Besides the relevance of loss in β-cell mass for the development of both T1D and, to a lesser extent, T2D (2), recent studies have suggested that impaired β-cell function plays a key role in early stages of both diseases (3). Whilst glucose metabolism in the β-cell is an important positive regulator of β-cell survival and regeneration (4), chronic hyperglycaemia impacts both β-cell function and survival (5). Adipose tissue secretes adipokines and sequesters fatty acids (FA) such as triglycerides to maintain glucose homeostasis (6). Obesity can cause adipose tissue dysfunction that contributes to peripheral insulin resistance (6). Furthermore, β-cell failure can result from the deleterious effects of high circulating free fatty acid levels, inflammation, and as recently proposed, pancreatic lipid deposition (7, 8).
Since their discovery less than 30 years ago, microRNAs (miRNAs) have been studied under each conceivable experimental condition and cellular context and are now well established as essential regulators of cellular function and important contributors to human disease (9). It was almost 20 years ago that the first islet-specific miRNA, miR-375, was identified and characterized by the Stoffel lab (10) and that Lynn et al. demonstrated that miRNAs are essential for the development of pancreatic islet cells (11). Islets contain hundreds of different miRNAs, and great efforts have been made to identify those dysregulated in diabetes and to understand their function in these cells. This has been extensively reviewed by others and us during the past few years (12–14). Nevertheless, the molecular and cellular mechanisms leading to the regulation of miRNA expression and action in these cells remain elusive. In this review, we aim to discuss the different molecular mechanisms that control miRNA function and their contribution to the regulation of miRNA expression and action in β-cells and islets. Given the central role that glucose and fatty acids play in β-cell survival and function, we will pay special attention to those mechanisms involved in nutrient-mediated regulation of miRNAs.
Canonical MiRNA Biogenesis and Mechanism of Action
Genomic organization of miRNA genes occurs in multiple configurations (Figure 1). On one hand, animal miRNAs can be encoded by individual genes or in clusters with other miRNAs. While clustered, miRNAs are often transcribed as a common polycistronic transcript though miRNAs inside the cluster can additionally contain independent transcription start sites (TSS). On the other hand, individual and clustered miRNA genes can be intergenic or intragenic, as defined by their location within other protein coding genes, often within their introns. Adding further complexity, intragenic miRNAs can be expressed with and processed from the host gene or be generated independently from independent TSS. Alternative splicing of the host primary transcript can also lead to additional layers of regulation (15).
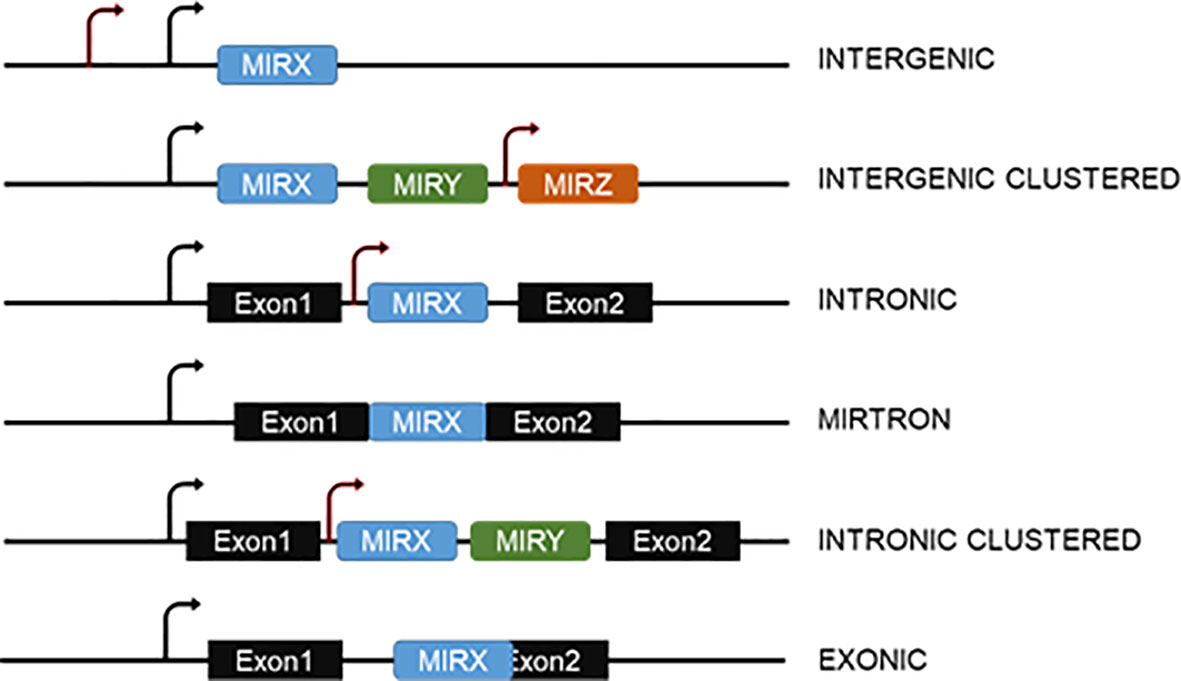
Figure 1 Genomic organization of miRNA genes. MiRNAs can be intergenic or be encoded within introns or overlapping exons of other genes. In both cases, they can be in clusters with other miRNAs. Mirtrons are generated from spliced-out introns and do not require the action of the Microprocessor. The arrows indicate the possibility of more than one transcription start site for both intergenic or intragenic miRNAs.
Most mammalian miRNAs are transcribed by RNA polymerase II as capped and polyadenylated primary transcripts (pri-miRNAs) (16–18). Pri-miRNAs contain at least one hairpin with the mature miRNA sequence inside the stem and are often up to hundreds of kilobases long (16, 18, 19). Pri-miRNAs are recognized by the microprocessor complex, consisting of the RNAse III enzyme DROSHA and the double-stranded RNA binding protein DGCR8, between other proteins (20, 21). The microprocessor complex very rapidly cleaves the base of the hairpin to generate ~70 nucleotide miRNA precursors with a 2-nt 3’ overhang (pre-miRNAs) (22, 23). Pre-miRNAs are exported to the cytoplasm via exportin-5 (24, 25) where they are recognized and processed by the RNase III enzyme DICER into 20–25 nucleotides RNA duplex with ~2-nt 3’ overhang at each end, containing the mature miRNA (26, 27). The short miRNA duplex will bind a protein of the Argonaute (AGO) family in an ATP-dependent manner (28) that will promote the expulsion of one of the miRNA strands [the “passenger” or “star (*)”] (29). Which one of the strands is degraded depends on the orientation of the duplex within Argonaute as determined by the most 5’ nt sequence and the stability of the 5’ terminal pairing (30, 31). In some cases, both strands can work as mature miRNAs (32) (Figure 2). Consequently, DICER is dissociated from this complex known as the miRNA-induced silencing complex (miRISC). MiRNAs guide miRISC to partially complementary sequences in the 3’ untranslated region (3’UTR) of target mRNAs or, less frequently, their coding region (CDS) and, rarely, the 5’UTR (33). It is now well established that most miRNA binding sites contain extensive complementarity to nucleotides 2–8 of the miRNA (the “seed” region) and that an adenine opposite to position 1 of the miRNA enforces target recognition (34, 35). Nevertheless, non-canonical binding sites, with little or no seed-complementarity, can also mediate effective mRNA target recognition (33, 36, 37). MiRNA action results in silencing of gene expression through mainly two mechanisms in mammals: inhibition of translation and/or mRNA destabilization. MiRNAs recruit TNRC6 (orthologue to Drosophila’s GW182 and nematodes’ AIN-1/2), which interacts with the poly(A)-associated protein PABPC that recruits deadenylase complexes PAN2-PAN3 and CCR4-NOT resulting in poly(A) tail shortening, often followed by DCP1/DCP2-mediated decapping and 5’-to-3’ exonucleolysis by XRN1 of the target mRNA. TNRC6-CCR4-NOT can also recruit the helicase DDX6 resulting in inhibition of mRNA translation. Additional mechanisms contributing to inhibition of translation include the interference with initiation factor eIF4E and ribosome recruitment and scanning (Figure 3). The specific molecular mechanisms of repression and their relative contribution to gene silencing remain debated and can be cell- and miRNA-specific (9, 38). It is worth noting that while mRNA decay has been proposed to be the dominant mechanism for biologically meaningful gene silencing in mammalian cells (39), translational repression is the main mechanism regulating zebrafish development (40). Moreover, there are multiple examples of differentiated mammalian cells in which translational repression regulates the expression of key miRNA targets (41–45).
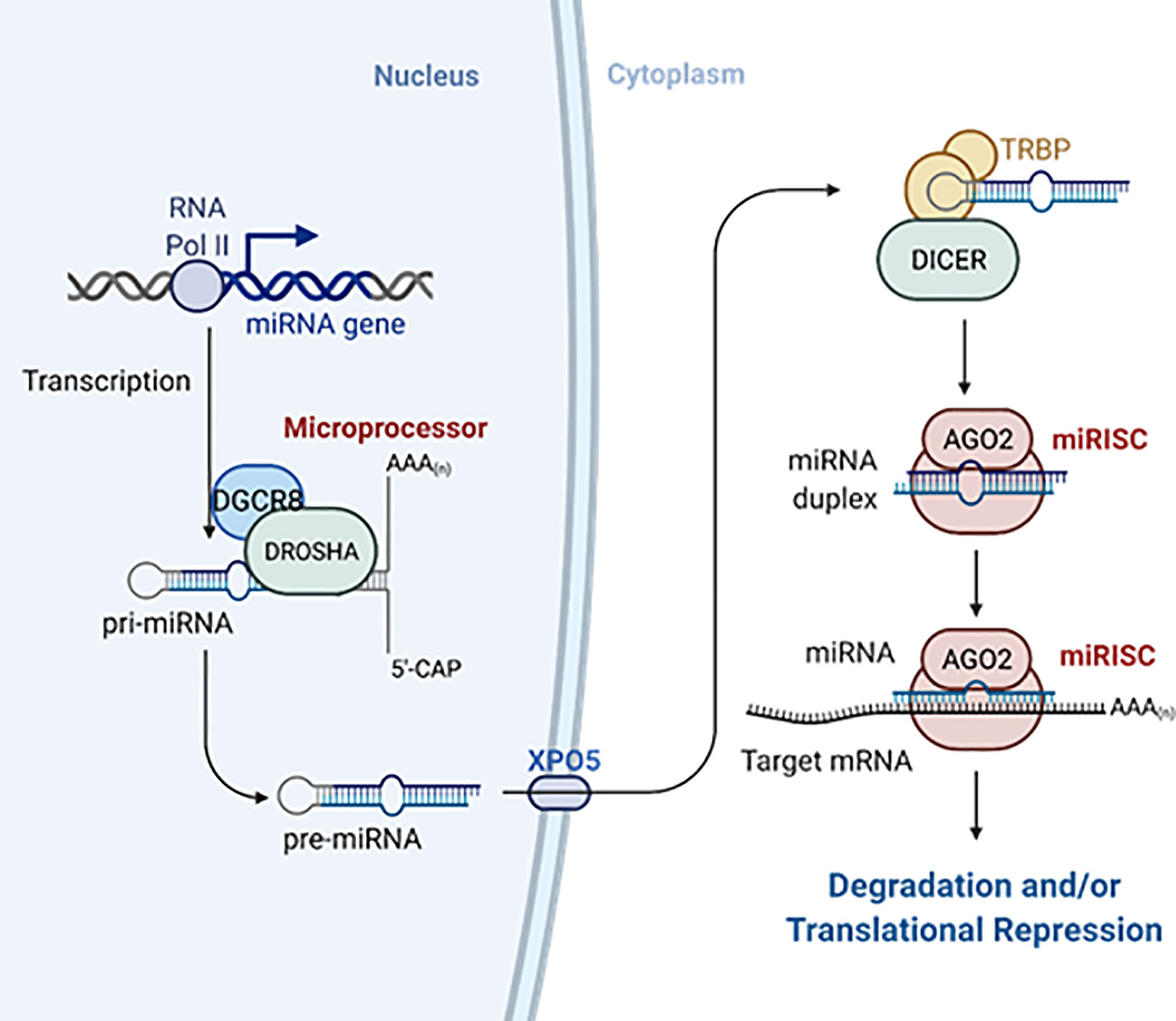
Figure 2 MiRNAs biogenesis. MiRNAs are transcribed as longer primary transcripts (pri-miRNAs) that are first processed in the nucleus by the Microprocessor complex to generate ~70-nucleotides pre-miRNAs that are exported to the cytosol by exportin-5 (XPO5). Pre-miRNAs are further processed by Dicer and TRBP into small RNA duplex. The mature miRNA is loaded with Argonaute proteins into the miRISC. Created with Biorender.com. See the main text for further details.
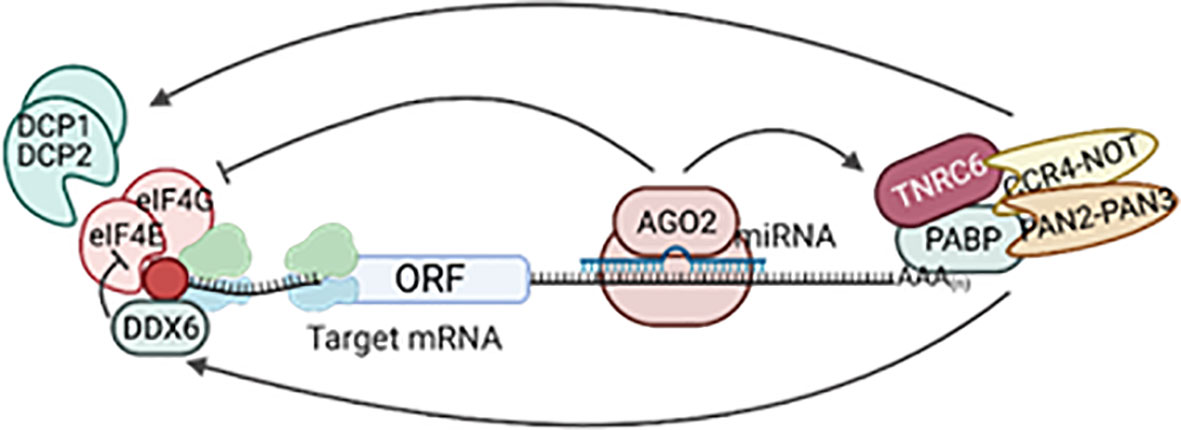
Figure 3 MiRNA mechanism of gene silencing. Following loading into miRISC, the miRNA guides the complex to partially complementary target mRNAs. MiRISC recruits TNRC6, PABPC, and deadenylase complexes CCR4-NOT and PAN2-PAN3, shortening the poly(A) tail. This can be followed by decapping and 5’-to-3’ exonucleolytic degradation and/or recruitment of the helicase DDX6 to inhibit translation. Other mechanisms of translational repression include inhibition of the recruitment of initiation factors (eIF4) and ribosome scanning. Created with Biorender.com.
A given mRNA can be simultaneously targeted by several different miRNAs, and conversely, miRNAs usually target several mRNAs at the same time. This often results in small effects in the expression of several genes on the same biological pathway and contributes to fine-tune and reinforce cellular identity and states (46–48).
In addition, non-canonical mechanisms of miRNA biogenesis have been described that bypass one or more of the canonical steps mentioned above (49). For excellent comprehensive reviews on miRNA biogenesis and mechanisms of action, we refer the reader to Treiber et al. (50), Bartel (9), and Gebert and MacRae (38).
Beta-Cell MicroRNAs and Their Regulation by Nutrients
Studies based on Dicer deletion at different stages during pancreas development and in the adult β-cell have extensively demonstrated that miRNAs are essential for endocrine cell differentiation (11, 51–53) and mature β-cell function and survival (54, 55). In the adult β-cell, miRNAs play a plethora of roles that range from control of insulin production (54, 56–59), insulin exocytosis (60, 61), apoptosis (62, 63), growth (64), repression of disallowed genes (55, 65, 66), and maintenance of β-cell identity (54, 67, 68). Others and we have comprehensively reviewed this work before (12, 13, 69), so here we will focus on the influence that nutrients have in islet miRNA expression and function and, more specifically, the molecular mechanisms underlying this regulation.
Hyperglycaemia/Lipidaemia
Chronic hyperglycaemia impacts both β-cell function and survival (5) and is a critical contributor to the development of over diabetes (70). Hyperglycaemia exerts a strong effect on gene expression, which contributes to loss of β-cell identity and function in diabetes (71–73). Very recently, Ebrahimi et al. (74) performed RNAseq in islets from partially pancreatectomized rats subjected to different levels of hyperglycaemia and demonstrated that whereas changes in gene expression correlated with diabetes severity, even a very mild hyperglycaemia was sufficient to alter the expression of genes important for β-cell identity, insulin secretion, and mitochondrial function (74).
Obesity is strongly associated with insulin resistance and increased risk of T2D (75), and chronic exposure to free fatty acids (FFA), specially saturated fatty acids, leads to lipotoxicity that affects β-cell functionality and survival and contributes to the development of hyperglycaemia (76).
Whereas Goto-Kakizaki (GK) rats represent a non-obese model of T2D, db/db and ob/ob mice spontaneously develop severe hyperphagia leading to obesity and T2D (77). These models have been widely used to determine, both by high-throughput and targeted experiments, whether miRNAs contribute to glucolipotoxicity. Other studies have assessed the expression of miRNAs in islets of mice fed a high-fat diet or in cell lines and murine or human islets cultured under lipotoxic and/or glucotoxic conditions. Table 1 contains a comprehensive list of glucose and fatty acid-regulated miRNAs and a summary of their key functions in pancreatic β-cells/islets. Nutrient-regulated miRNAs, such as for example miR-130a/d and miR-152 (81, 95), are also often dysregulated in diabetes in humans (Table 1, “Human T2D” column), supporting their contribution to the development of the disease. On the other hand, it remains contested whether the expression of other glucose-regulated miRNAs with important roles for β-cell function is dysregulated in T2D. For example, β-cell-specific miR-375 is an important regulator of β-cell mass and insulin secretion (153, 156, 168–170) upregulated by glucose in murine cell lines and islets, as well as in islets from ob/ob mice (64, 156). Nevertheless, only one study reported increased miR-375 in pancreatic tissue from T2D human donors (154) with most studies finding identical expression in islets from T2D and healthy human donors (95, 122, 123) and in other murine models of diabetes (81, 97). Another example is miR-184, an important mediator of compensatory β-cell secretion and expansion during insulin resistance in the face of obesity and pregnancy (64, 119). MiR-184 is strongly downregulated by glucose in MIN6 cells and mouse, rat and human islets (64, 97, 118), reduced in islets of prediabetic, db/db mice and mice fed a HFD (97) and strongly upregulated in islets of mice starved for 24 h or kept on a (low-sugar) ketogenic diet (64). Nevertheless, although Tattikota et al. observed downregulation of miR-184 in islets from human T2D donors, our group failed to confirm these differences, as so did others, perhaps concealed by the sex-dimorphism observed in miR-184 expression (118, 122, 123).
Low-Protein Diet
Feeding a low-protein (LP) diet during gestation represents a model of intrauterine growth restriction (IUGR) characterized by decreased placental leucine transport and reductions in essential amino acids in dams (171). Alejandro et al. (172)found that several miRNAs, including miR-7, -199a-3p, miR-152 and miR-342-5p were increased and others, such as miR-30b, miR-487b and miR-224, decreased in adult islets from mice born to LP-fed dams. Although the function of all these miRNAs was not interrogated, the authors demonstrated that miR-199a-3p and -342 regulated mTOR protein levels and insulin secretion in β-cells and their inhibition was able to rescue the secretory defects observed in these islets.
In another study, altered miR-375 levels were increased in neoformed islets from foetuses and in adult islets from the progeny of female dams fed a LP diet during pregnancy. These islets had impaired β-cell mass and function that was rescued by restoration of miR-375. Of note, an additional 43 miRNAs were upregulated while four were downregulated in the foetal pancreas of LP-fed mothers (173). In a similar study, Su et al. identified upregulation of miR-15 in offspring of LP-fed dams, and the inhibition of this miRNA corrected the defects in beta-cell proliferation observed in the islets (174). More recently, Alejandro and colleagues assessed the effect of the LP diet administered only during the last week of gestation. This was sufficient to induce glucose intolerance in offspring at age older than 12.5 weeks and to increase the sensitivity of HFD-mediated diabetes. On the contrary to what they had previously observed when LP diet was administered during the whole pregnancy, these defects occurred without changes in mTOR and resulted in dysregulation of a completely different subset of miRNAs, including miR-342, miR-143, miR-338, miR-335, and miR-184 (175). It is worth noting that even though very little is known on the effects of LP diet in maternal islet miRNAs, de Siqueira et al. observed a reduction in miR-124a that may contribute to defects in insulin release, possibly via regulation of the exocytotic machinery (176). Nevertheless, these studies were performed in murine models, and therefore further investigation is required to determine whether these effects are sustained in humans.
Weaning
It has been demonstrated that the nutritional changes associated with weaning are essential to trigger complete β-cell maturation, develop β-cell capacity of compensatory proliferation, and improve glucose-stimulated insulin secretion (177). Interestingly, the expression of several miRNAs such as miR-25, miR-17, and miR-29b changes during this process and may contribute to the improvement in glucose-induced secretory response (117).
It is important to acknowledge that there is often little overlap in the differentially expressed miRNAs under diabetic/glucolipotoxic conditions between studies. Underlying these discrepancies are the use of low number of samples (specially for human islets), heterogeneity of the samples (i.e., origin, purity, sex, age, and other characteristics of the donors), experimental conditions (i.e., concentration and duration of the glucolipotoxic insult, or diet composition), or the use of different detections systems (i.e., qPCR, arrays, high-throughput sequencing). Moreover, β-cell lines represent a de-differentiated system that only partially preserves the original characteristics of primary β-cells. Additionally, cell lines are removed from the natural tissue architecture and therefore lack cell-cell interactions with other endocrine, and non-endocrine, cells. Many studies have on the other hand been performed in full islets, where β-cells only represent a proportion (50–80%, humans-mice) of the cellular population. Islets might also respond differently to treatments in isolation or to diets administration in vivo. Finally, even though mature miRNA sequences are generally highly conserved amongst mammals, different levels of expression often occur between homologous tissues of mice and humans, especially for late-emerging miRNAs (178). Rodent and primate islets not only differ in innervation and architecture, but the β-cells contain significant transcriptome differences that involve species-specific transcription factors and long non-coding RNAs (lncRNAs) (179). It is therefore imperative that nutrient-dependent regulation of miRNA expression is validated in human islets, facilitated by the significant improvement of human islets availability during the past decade. Moreover, “humanized” mouse models for the study of human β-cell function in vivo are now well established in several laboratories around the globe. In these models, human islets are transplanted into mice, often following drug-mediated elimination of their own islets. This approach has been previously used to identify human islet miRNAs associated with β-cell destruction in mouse models of T1D. The effect of experimental diets fed to the recipient mice could also be investigated in the transplanted human islets. Whereas standard protocols involve transplantation under the renal capsule and the effects on transplanted-islet miRNAs can only be measured at specific end-points, recent developments use transplantation in the anterior chamber of the eye, which allow in vivo, longitudinal imaging of the implanted islets, often exploited to interrogate functionality and engraftment (180). Recent advances in miRNA monitoring in vivo, such as the use of fluorescently labelled miRNA activity reporters or molecular beacons which activity depends on the presence of the miRNA (181), could be incorporated to allow the interrogation of miRNA function in the transplanted beta cells.
Molecular Mechanisms Underlying Nutrient-Mediated Regulation of miRNA Action
Despite the great effort dedicated to identify glucose- and diabetes-regulated miRNAs, little information is available regarding the cellular and molecular mechanisms underlying changes in islet miRNA expression. Cellular expression of mature miRNA is determined by the rates of miRNA transcription, processing, and decay, each of which can be globally or specifically regulated. Moreover, miRNAs’ capacity to bind and silence their target can be modulated by additional factors such as post-transcriptional modifications and interaction with proteins and other non-coding RNAs (Figure 4).
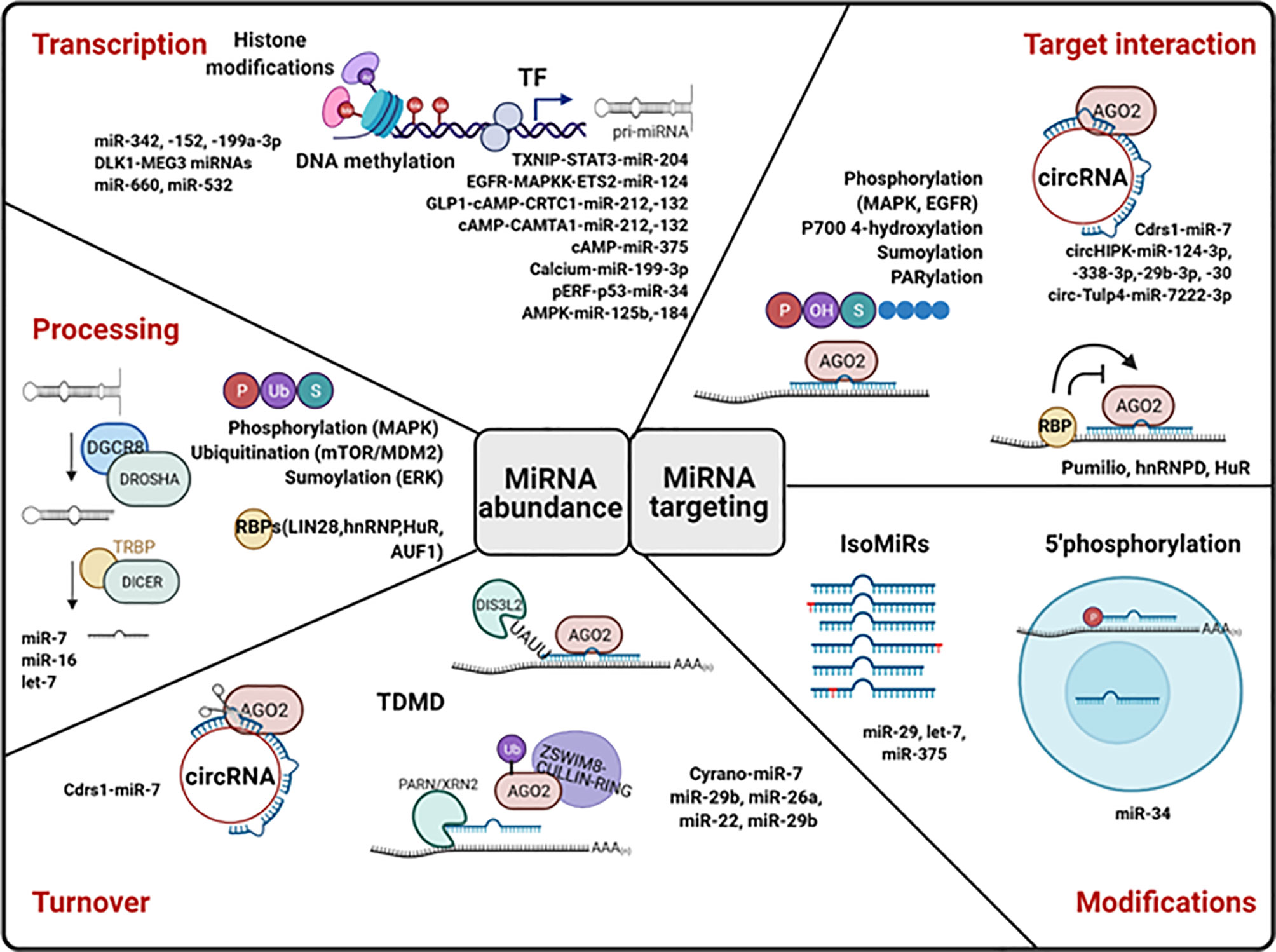
Figure 4 Molecular mechanisms of miRNA regulation. MiRNA levels can be control at the level of transcription, processing, and stability. MiRNA transcription is regulated by epigenetic modifications (histone modifications and DNA methylation) and by the action of transcription factors (TF). The action of proteins important for RNA processing is regulated by post-translational modifications (P, phosphorylation; U, Ubiquitination; S, Sumoylation) and by RNA-binding proteins (RBPs). Circular RNAs (cirRNAs) can promote miRNA degradation. TDMD, target-directed miRNA degradation, can occur dependently or independently of miRNA tailing and trimming. The capacity of miRNAs to bind and repress their targets can be modulated by miRNA modifications and by RBPs, lncRNAs, and circRNAs. Examples of islet miRNAs regulated at each level are included in the figure. Created with Biorender.com. See text for more details.
MiRNAs Transcription
As is the case for messenger RNAs, the transcription of each pri-miRNA is tightly controlled by epigenetic and transcription factors. Epigenetic regulation of miRNA expression has been widely studied in tumours and involves DNA methylation and histone modifications that often contribute to miRNA dysregulation during tumour progression (182). It is well established that miRNAs form tightly coordinated regulatory networks with transcription factors, which contribute to reinforce cellular states (47).
Several examples of nutrient-dependent regulation of miRNA transcription have been described in pancreatic islets. MiR-204 is an intronic miRNA located within and sharing a common promoter with Trpm3 (183) and upregulated in islets of ob/ob (127) and db/db mice (128). In pancreatic islets, TXNIP stimulates the expression of both miR-204 and TRMP3 through, at least in part, inhibition of STAT3 (signal transduction and activator of transcription 3) activity (127). MiR-204 blocks insulin production via direct targeting of Mafa, contributing to the development of diabetes (127), and its inhibition improves glycaemic control in db/db mice (128). Another interesting example is miR-124 (miR-124-3p/miR-124a), which is a negative regulator of insulin secretion (82) upregulated by glucose in MIN6 cells (78), islets of GK rats (81), and T2D human islets (82). In a recent study, Yang and colleagues demonstrated that β-cell miR-124a expression is negatively regulated by EGFR signalling through MEK/ERK and PI3K/AKT downstream signalling cascades leading to ETS2 (E26 transformation-specific 2) activation and association with miR-124a promoters on chromosomes 2,3 and 14 (184). Glucose (185), HFD, and pregnancy (186) have been previously shown to modulate EGFR signalling, and it is therefore tempting to hypothesize that this EGFR-MEK/PI3K-ETS2 pathway contributes to the regulation of miR-124 by nutrients. Interestingly, miR-124 targets ionotropic glutamate receptors iGluR2 and iGluR3 in islet α-cells, and the expression of this miRNA and iGluR2/3 was strongly inhibited and upregulated, respectively, following fasting. Thus, miR-124 might additionally contribute to glucotoxicity by impairing glucagon release by α-cells (187).
Recently, Werneck-de-Castro et al. explored the mechanisms underlying the regulation of miR-199-5p and miR-199-3p in murine β-cells. As previously mentioned and summarized in Table 1, these miRNAs are upregulated in islets from mouse models of diabetes and in the progeny of LP-fed dams and have been implicated in impaired β-cell function and survival (81, 97, 172). Werneck-de-Castro et al. found that pri-miR-199 abundance is also regulated by glucose in MIN6 cells and mouse islets and that membrane depolarization-induced calcium release modulates Mir199a-2 promoter activity and mature miR-199 expression acutely, though the exact molecular mechanisms remain to be elucidated (124).
Additionally, miR-34a is increased in INS-1 cells cultured with palmitate and stearic acid, as well as in islets from mice fed a high-stearic acid or a high-palmitic acid diet and db/db mice. Inhibition of this miRNA reduced lipotoxicity-induced islet β-cell death and rescued insulin content (152). These authors also demonstrated that pERK-p53 mediated miR-34a upregulation. They suggested that this occurs at the level of miR-34a transcription since p53 is a well-established transcriptional activator of this miRNA in cancer and other cell types (188, 189).
Other interesting examples of transcriptional regulation are miR-132 and miR-212. Both miRNAs are upregulated in GK rats (81) and induced by glucagon-like peptide 1 (GLP-1) in mouse and human islets (134). GLP-1 is an incretin hormone produced by the L-cells in the gastrointestinal track upon oral nutrient administration and a potent insulin secretagogue (190). GLP-1 activates GLP-1R triggering the activation of adenylate cyclases and the generation of cAMP that promotes granules exocytosis (191). Malm and colleagues demonstrated that the CREB (cAMP-responsive element binding protein) transcriptional co-activator CRTC1 (cAMP-regulated transcriptional Co-activator-1) and the CRTC inhibitors SIK1-3 (salt-inducible kinases) mediate cAMP-co-regulation of miR-212/miR-132 in rat β-cells (192). In a separate study (193), the same group showed that hyperglycaemia increased the expression of both mature and primary miR-212/-132. Interestingly, knockdown of calmodulin binding transcription activator 1 (Camta1) increased the expression of primary and mature miR-212/132 in rat islets and clonal β-cell and reduced the increase in pri-miR-212/132 levels mediated by the adenylate cyclase activator foskolin (193). Intriguingly, pri-miR-212/132 remained unchanged following stimulation with GLP-1, perhaps due to the complex kinetics of cAMP signalling in these cells (194). To the best of our knowledge a high-throughput screen of miRNAs regulated by GLP-1 (and/or cAMP) in islets has not been performed. Nevertheless, mTOR upregulation and concomitant decrease of miR-7, miR-375, and miR-9 was also observed in islets of HFD-fed mice treated with the GLP-1 analogue exendin-4 (195, 196). Additionally, Keller et al. found that miR-375 promoter occupancy by Pol II was reduced with cAMP agonists and concluded that cAMP represses miR-375 at the transcriptional level. Of note, this regulation occurred via PKA and was not modulated by glucose. Interestingly, miR-375 expression is downregulated in islets from GK rats, in INS1 cells cultured at high-glucose concentrations during 1 h–4 days and in rat islets cultured during a short period of time (2 h) at high-glucose concentration (81, 153). Nevertheless, rat islet miR-375 was upregulated upon 72 h of exposure to high glucose (153). Similar differences were observed for other miRNAs involved in the control of insulin secretion and content such as miR-132, miR-335, and miR-15 (81, 116) and might reflect the dual role of short- and long-term glucose in pancreatic β-cell function and survival. In an earlier study, Keller et al. found that β-cell specific transcription factors, namely, Pdx1 and NeuroD1, bind the proximal promoter and distal enhancer of MIR375. Glucose stimulates Pdx1 activation by the PI-3 kinase pathway (197), whereas miR-375 represses PI 3-kinase signalling to limit glucose stimulation of insulin gene expression (153), resulting in a negative-feedback loop that may contribute to the effects of glucose in miR-375, Pdx-1, and insulin expression.
Finally, our group showed that islets with β-cell selective deletion of AMP-activated protein kinase (βAMPKdKO) contain decreased and increased levels of both mature and primary miR-184 (118) and miR-125b miRNAs (83), respectively, suggesting regulation at the transcriptional level. Nevertheless, further studies are needed to fully elucidate the transcription factors involved in this regulation and to interrogate whether additional mechanisms are also involved. Several transcription start sites (TSS) in two different loci have been annotated in other cell types for miR-125, while miR-184 TSS remains to be fully annotated, which complicates these studies.
Of note, the majority of these studies have been performed in rodent islets/β-cells, so it is yet to be determined whether the transcription of these miRNAs is regulated in a similar manner in humans.
DNA methylation and histone modifications have been extensively demonstrated to control islet gene expression and secretory function (198), and both are altered during lipoglucotoxicity in β-cells (199, 200) and in T2D [reviewed in (201)]. Nevertheless, there is limited knowledge on their contribution to regulation of islet miRNA expression. Alejandro et al. (172) noted that the majority of miRNAs upregulated in islets from mice born to LP-fed mice are regulated by methylation of their promoters (miR-342, -152, and -199a-3p-a1) and that miR-199a-3p-a2 and -7a contain E-boxes, so could potentially be transcriptionally targeted by Ngn3 and NeuroD/β2. Kameswaran et al. (122) detected a cluster containing 54 miRNAs (DLK1-MEG3) highly expressed in β-cells and strongly downregulated in islets from T2D human donors. This cluster is maternally imprinted, and these authors observed that DNA methylation in a differentially methylated region responsible for the maternal imprinting was increasingly methylated in the T2D islets. Differential methylation between male and female human pancreatic islets was also associated with the levels of miR-660 and miR-532 (202). More studies are required to elucidate the contribution of these mechanisms to nutrient-mediated regulation of islet miRNA expression.
The Challenge of Studying miRNA Transcription
Although transcriptional regulation of a few specific islet miRNAs has been studied to some extent, as exemplified above, nutrient-mediated effect on β-cell miRNA transcription remains to be investigated in a high-throughput manner.
During the past few years, several methods have been developed to measure transcriptional rates genome-wide, many of which rely in enrichment in nascent mRNAs. Some examples are Nascent Elongating Transcript Sequencing (NET-seq), which uses immunoprecipitation to capture RNAs associated with PolII (203), and run-on-based assays such as Coordinated Precision Run-On and sequencing (CoPRO) (204), that use radio-labelled NTPs to tag nascent RNAs, which can be identified by high-throughput sequencing and provide active site location of PolII and cap status from single nascent transcripts.
Nevertheless, the use of these approaches to study miRNA transcription is subject to the availability of accurate pri-miRNA annotations. MiRNA transcription start sites (TSS) are often tissue-specific and conventional techniques for miRNA TSS identification such as 5’RACE, and RNAseq has been somewhat restricted by the quick processing of pri-miRNAs into mature miRNAs and by the fact that miRNA TSS can be located thousands of nucleotides away from the mature miRNA sequence. The quick improvement in the resolution of high-throughput sequencing technologies and the computational analysis (including deep machine learning) has more recently allowed the integration of epigenetic marks (H3K4me3, H3K9/14Ac, PolII) and cap analysis of gene expression (CAGE-seq) to more precisely map miRNA transcription start sites in a cell-specific manner (205–207). More recently, Liu et al. (206) used global nuclear run-on sequencing [GRO-seq (208)] and precision nuclear run-on sequencing (PRO-seq [209)] that provide sharp peaks around transcription start sites and continuous signal over active transcription regions to map the TSS of 480 intergenic miRNAs in 27 different human cell lines. Moreover, they integrated this information with ChIP-seq data from matched ENCODE tissues and generated a transcriptional circuitry that further demonstrated the complex interplay between TF and miRNA. Importantly, these authors generated a computational tool, mirSTP (http://bioinfo.vanderbilt.edu/mirSTP/), which can be utilized to map miRNA TSS in any cell type with available GRO/PRO-seq data. Although big consortium studies such as ENCODE do not normally generate data on pancreatic islets, which comprise a small proportion of the pancreas, the increased accessibility to protocols and systems for genome-wide profiling of epigenetic marks is allowing biologists worldwide to rapidly generate and share islet-specific data. These data will hopefully be used in the near future to precisely map β-cell/islet miRNA TSS and to shed more light into the transcriptional mechanisms by which nutrients control pri-miRNA expression in these cells. Additionally, imaging methods that use in vivo labelling of the sites of nascent transcripts [reviewed in (210)] can also be adapted for their use to study transcription of specific miRNAs in β-cells in response to nutrients in vivo.
It has been long established that miRNAs act cooperatively with other miRNAs and transcription factors that are themselves targeted by miRNAs, typically acting as hubs in regulatory networks. Over the past decade, fed by the increased availability of expression data and high-throughput miRNA target determination, important efforts have been made to develop in silico approaches that integrate this information to identify key regulatory networks (211). Nevertheless, most available networks are built based on gene-wide miRNA targeting and gene expression correlations, with very little input on regulation of miRNA gene expression. For example, miRNet (212) is a web-accessible tool originally generated to illustrate miRNA target interactions in which a given miRNA can target several genes and a gene can be targeted by several miRNAs. More recently, MiRNet 2.0 attempts to integrate transcription factor–miRNA interactions obtained from TransmiR (213) that incorporates publication-curated miRNA-TF as well as ChIP-seq identified interactions. MiRNet has also implemented a tissue-specific filter that restricts the cellular context based on miRNA expression profile, but profiles in pancreatic islets and/or beta cells have not been included. Very recently, Wong et al. (214) used miRNA profiling data of insulin-negative human tissue samples and (insulin-expressing) pancreatic islets and applied an unbiased, machine learning discovery approach to identify miRNAs associated with insulin mRNA that regulated insulin transcription. A similar networking method could be applied in the future to identify transcriptional regulators of the expression of specific islets miRNAs by integrating tissue-specific mRNA expression data.
MiRNA Processing
As mentioned above, the abundance of mature miRNAs can strongly depend on their processing from pri- and pre-miRNAs. Both Drosha and Dicer were downregulated at the mRNA level in hyperglycaemic rat islets, though the impact on miRNA expression remained to be determined (74). On the contrary, proteomic analysis of isolated human islets cultured at high glucose concentration identified increased levels of DICER (215). On the other hand, peripheral blood mononuclear cells (PBMCs) from mice treated with metformin or caloric restriction contained higher levels of Dicer1 mRNA due to increased binding of the RNA Binding Protein (RBP) AU-rich element-binding factor 1 (AUF1) (216). Of note, no changes in Drosha or Dicer expression were observed in βAMPKdKO islets (217), suggesting a glucose effect independent of AMPK and the need of further studies to clarify whether glucose regulate the miRNA processing machinery.
Both Microprocessor proteins and Dicer can suffer post-transcriptional modifications that modulate their activity. For example, DGCR8, DROSHA, and TRBP can be phosphorylated by mitogen-activated protein kinases (MAPKs), DROSHA can be ubiquitinated via mTOR/MDM2 activation, and DGCR8 sumoylation and stabilization can be stimulated by ERK [reviewed in (218)]. MAPK pathways play central roles in β-cells response to stress, including hyperglycaemia and lipotoxicity. Whether this results in post-translational modifications of the miRNA biogenesis machinery, though likely, remains to be investigated.
RNA binding proteins (RBPs) can bind to precursor miRNAs to alter their processing by the Microprocessor and Dicer, providing a sequence-specific level of regulation [see (219] for a comprehensive review). Some examples include the well-characterized protein LIN28, which can act both at the level of DROSHA in the nucleus or by impairing DICER-mediated processing in the cytosol (220, 221) and hnRNP A1 that can enhance pri-miRNA processing (222) but has a dual effect on pre-miRNA maturation (223, 224). Unfortunately, it is not currently known whether processing of specific miRNAs contributes to nutrients’ action in islets. As a proof-of-concept in HeLa cells, oleic acid induced miR-7 processing through remodelling of pri-miR-7 and inhibition of RBPs HuR and MSI2 binding and reduced miR-16 processing by an unknown mechanism (225). Also, it has been recently shown that Lin28a protects against β-cell destruction induced by streptozotocin and may enhance insulin secretion in a whole-body model of overexpression. Interestingly, MIN6 cells overexpressing this protein contained reduced levels of let-7 family members, though other miRNAs were not investigated (226).
MiRNA Turnover
MiRNA half-life differs considerably between miRNAs, cell types, and importantly, cellular context, ranging from minutes to weeks (227–229). For example, neuronal miRNAs present faster turnover rates in comparison with other tissues, which reflects how crucial dynamic miRNA regulation is for the nervous system (183). It has been proposed that the base composition of the miRNA can itself impact degradation rate (183) and that miRNA stability can vary according to their binding to specific members of the Ago family (228). Nevertheless, the mechanisms responsible for miRNA turnover remain poorly understood. Addition or removal of nucleotides in the 3’ end of miRNAs (see MiRNA Modifications below), a process known as tailing and trimming, alters the stability of some, but not all, miRNAs (230). MiRNA degradation can be triggered by extensive (albeit incomplete) complementarity to target mRNA in a process known as target-directed miRNA-degradation (TDMD) (231). TDMD often involves 3’ terminal tailing and trimming of the miRNA, and it is a relevant mechanism contributing to neuronal miRNA regulation (232). Nevertheless, recent work by Mendell and Bartel labs have uncovered a tailing- and trimming-independent TDMD mechanism mediated by the ZSWIM8 Cullin-RING E3 ubiquitin ligase, which occurs in multiple cell types (233, 234). ZSWIM8-CRL binds to and targets for degradation AGO proteins exposing miRNAs for degradation by cytosolic RNAses, such as PARN and XRN2. XRN2 has already been demonstrated to mediate miRNA degradation in C elengans (235), although its role in mammalian cells might be more complex and leads to both miRNA degradation but also stabilization (236). On the other hand, the Perlman syndrome exonuclease DIS3L2 has been implicated in the degradation of oligouridylated miRNAs (237). Many of these proteins are components of the no-go and non-sense-mediated RNA decay pathway that were upregulated by pro-inflammatory cytokines in INS-1 cells to affect stability of Ins1/2 and other mRNAs, though miRNA expression was not interrogated (238).
Long non-coding RNAs can induce TDMD of miRNAs. This has been observed for miR-29b in Zebrafish (239) and for miR-7 in brain in zebrafish and mice (240). Interestingly, neuronal miR-7 was regulated not only by the lncRNA Cyrano but also, to a lesser extent, by Cdr1as, a circular RNA. In this model, miR-7 repressed Cdr1as via binding to multiple regions, forming a post-transcriptionally regulatory network important for neuronal function (240). Cyrano is poorly expressed in islets, and its deletion does not affect miR-7 expression (240). Nevertheless Cdrs1 has been shown to regulate insulin secretion and transcription in MIN6 cells and islets, allegedly by acting as a miR-7 sponge de-repressing its targets (59). Interestingly, Cdrs1 is downregulated in islet from db/db and ob/ob mice (241).
To the best of our knowledge, the effect of nutrients on islet miRNA stability hasn’t been investigated. Stefan-Lifshitz et al. found that human islets exposed to cytokines showed changes in DNA methylation patterns and gene expression, including that of the exoribonuclease PNPase old-35 (PNTP1) that caused degradation of miR-26a and, to a lesser extent, miR-22 and miR-29b (242). This in turn increased 5-hydorxymethylcytosine levels via upregulation of ten-eleven-translocation 2 (TET2). It would be interesting to know whether this mechanism contributes to reduced miR-26a in db/db and HFD-fed mice (143).
As mentioned above, miR-204 and its host gene Trmp3 are transcriptionally repressed by TNXIP/STAT3 in islets. Interestingly, miR-204 and Trpm3 are also co-regulated in retina where not only rapid transcription but also fast decay allow for miR-204 rapid turnover during light-dark adaptation (183). Many of the studies on miRNA stability have focused on neuronal miRNAs, and it has been indeed suggested that neuronal mechanisms regulating miRNA degradation are unique compared to other tissues. Given that neurons and β-cells share multiple physiological and organizational characteristics as well as gene expression patterns (243), this represents a promising future area of study for islet biologists.
Some of the molecular approaches to estimate RNA synthesis mentioned above have been further engineered to measure RNA degradation rates. An example is transient transcriptome sequencing (TT-seq), which is based on sequencing following the short exposure of cells to the nucleoside analogue 4-thiouridine (4sU-seq) that is incorporated during transcription and allows isolation of labelled RNAs (244). The Ameres lab developed an optimized TT-seq protocol called Time-resolved RNA sequencing (SLAM-seq) to determine pri-miRNA transcription rates that, importantly, they combined with the rates of mature miRNA biogenesis (assessed by SLAM-small-seq) to determine miRNA processing in a high-throughput manner in a Drosophila cell line (228). Even though these approaches are normally limited to their use in cultured cells, 4-thiouracil can be administered by intraperitoneal or subcutaneous injection (245), opening the doors to its application to the study of miRNA kinetics in β-cells/islets in vivo.
MiRNA Modifications
Any changes affecting miRNA sequence will have an impact on their capacity to recognize their targets, especially if those changes rely within the seed region. IsomiRs were first described by Morin et al. (246) and are microRNA isoforms originated from the same gene that differ in length and/or sequence. IsomiRs are usually generated by alternative Drosha/Dicer cleavage (247) but also by non-templated nucleotide addition (NTA) or RNA editing, and the proportion of IsomiRs expressed is cell- and tissue-specific (38, 248, 249). Alterations in the 5’ end of the miRNA tend to result in changes in their targeting properties, including potency and identity of the targets (248, 250). On the other hand, 3’ isomiRs usually present altered guide/passenger strand selection and stability and, to a lesser extent, targeting properties (247, 251). MiRNA precursors can be subject of deamination by ADAR1/2 that converts adenosine to inosine (249). Inosine shows guanosine characteristics, and thus A-to-I editing can alter miRNA biogenesis and stability, as well as target recognition (252, 253). Although unfrequently observed so far, A-to-I editing of miRNAs has already been shown to be biologically relevant for tumour progression (254, 255). The 3’ ends of mature miRNAs can also be subjected to adenylation or uridylation (256). Although not much data are currently available, the mechanism regulating adenylation involves, at least in some cases (i.e., liver miR-122, or miR-21 in tumours), the poly(A) RNA polymerases GLD2 and/or PAPD5 and the ribonuclease PARN (257, 258). Uridylation of miRNAs by TUT4 and TUT7 can both limit and expand target repression (259, 260) and can reposition and shift Dicer processing when occurring in the pre-miRNA (261).
Baran-Gale et al. undertook a computational effort to profile isomiRs in small RNA sequencing data from MIN6 cells and human β-cells and islets and identified multiple 5’isomiRs. Some of the isomiRs with shifted 5’ positions (5’-shifted isomiRs), such as miR-375+1 and miR-375-1, were as abundant as the reference miRNAs (Figure 5). Further analysis suggested that some of these isomiRs, including miR-29, let-7, and miR-375, were predicted to target multiple alternative genes dysregulated in T2D. Experimental validation of the physiological relevance of these isomiRs remains to be provided, as well as further insights into the mechanisms leading to the generation of these and other miRNAs. HFD feeding, fasting, and glucose regulate the expression of ADAR2 by MAPK signalling in mouse islets and β-cell lines. ADAR2 is essential for adequate exocytosis, but its contribution to islet miRNA editing is unknown (262–264).
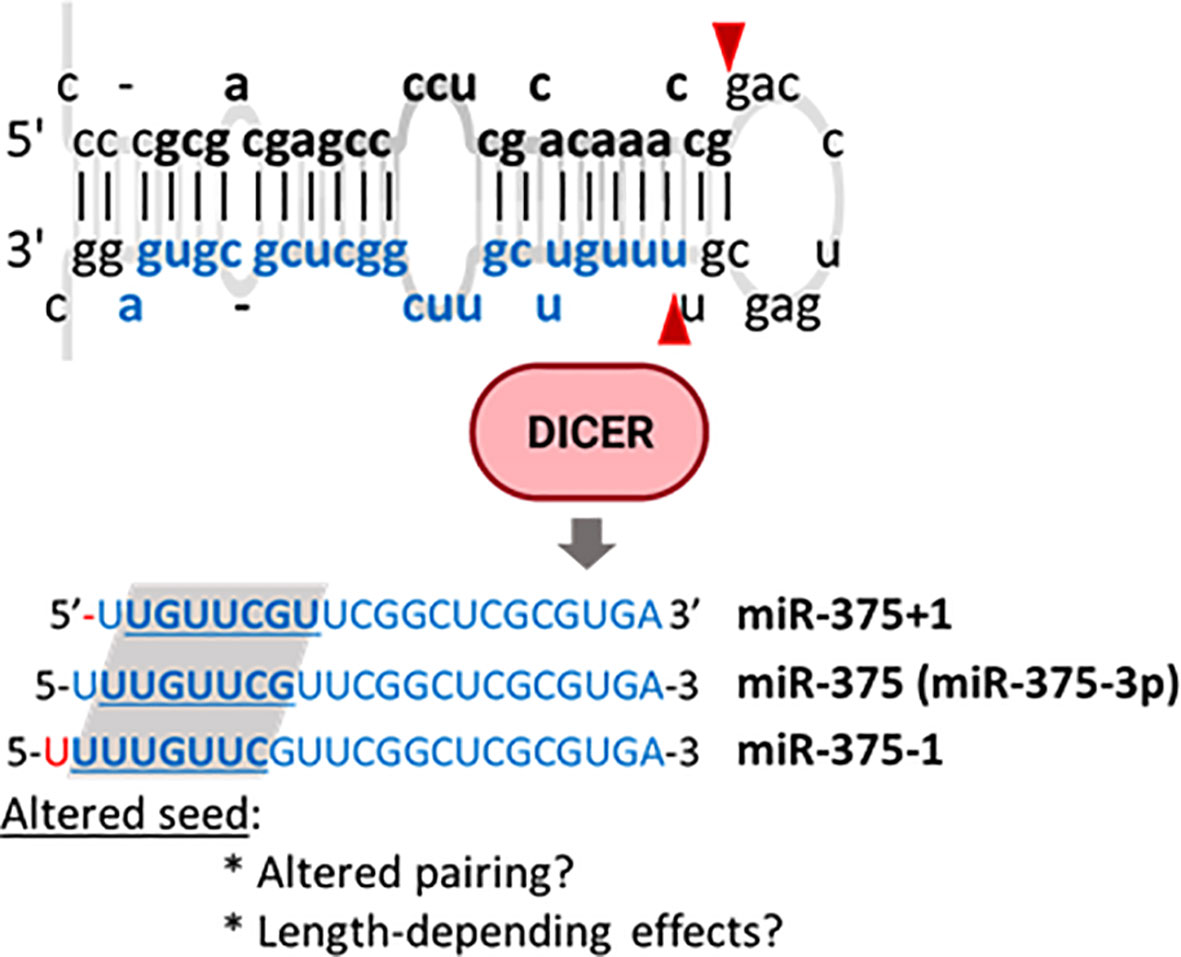
Figure 5 MiR-375 isomiRs identified in pancreatic islets. Pre-miR-375 may be alternatively processed by Dicer to generate isoMiRs with one additional (miR-375-1) or one less (miR-375+1) nucleotide in the miRNA 5’. These modifications have the potential to alter target recognition.
The 5’ miRNA phosphorylation/de-phosphorylation has emerged as an additional mechanism contributing to regulation of miRNA, though, to the best of our knowledge, it has so far only been observed for miR-34. In the absence of DNA damage, a pool of miR-34 lacking 5’phosphate remains inactive and not loaded into Ago. Nevertheless, ionizing radiation activates miR-34 by phosphorylation dependent of ATM (ataxia telangiectasia) and the RNA 5’kinase Clp1 (cleavage factor polyribonucleotide kinase subunit 1) (265). Of note, both ATM and miR-34 regulate β-cell damage response and survival (152, 266, 267).
Regulation of miRNA-Target Accessibility
Target accessibility is a critical factor in microRNA function and can be regulated at different levels, including the interaction with proteins, non-coding RNAs, and subcellular localization.
Regulation of Protein Activity in miRISC
Ago proteins consist in four domains: the amino (N)-terminal domain, the PAZ (Piwi-Argonaute-Zwille) domain, the MID (middle) domain, and the PIWI (P-element induced wimpy testes) domain, as well as two interconnecting, linker domains (L1 and L2). The 5’ end of the miRNA is hold by the MID and PIWI domains, whereas the PAZ domain binds the 3’ of the miRNA (268). There are four Ago proteins (1–4) present in mammals, of which Ago2 is the most abundant and the only one retaining catalytic activity (269). Ago proteins can undergo multiple types of post-translational modifications. Phosphorylation of the L2 linker or the MID and PIWI domains have been widely observed and modulate the activity of the protein by altering their miRISC assembly capabilities, stability, and/or trafficking to exosomes [reviewed in (38)]. Other post-translational modifications include ubiquitination and Lys402 sumoylation to destabilize Ago2 (270, 271), Pro700 hydroxylation to increase Ago2 stability (272), and PARylation [poly(ADP-ribosylation)] that reduces target accessibility (273, 274).
Tattikota et al. demonstrated that reduction of AGO2 during pregnancy and obesity-mediated β-cell compensation is required for compensatory proliferation. They also showed that loss of Ago2 in an ob/ob background results in the upregulation of mRNA targets of the abundant islet miRNA miR-375 (64). As previously mentioned, these authors found that Ago2 downregulation occurred as a consequence of direct targeting by the glucose-regulated miRNA miR-184. Other mechanisms contributing to glucose-mediated regulation of Ago2 content or post-transcriptional modifications have not been investigated in pancreatic β-cells.
RNA Binding Proteins (RBP) Can Modulate miRNA Targeting
Following the demonstration by Kedde et al. in 2007 that dead end 1 (Dnd1) counteracts the function of several miRNAs in human and zebrafish cells by binding to U-rich regions in the mRNAs and impeding miRNA accessibility (275), many other RBPs have been demonstrated to play essential roles in controlling miRNA-target binding. Pumilio represents another example of an RBP that limits miRNA accessibility to the target mRNAs (276), whereas HNRNPD (AUF1) enhances miRISC interactions in HeLa (277) and HuR can both antagonize or promote miRNA function (278, 279). HuD is a member of the Hu family highly expressed in β-cells regulated by glucose and insulin receptor signalling. In β-cells, HuD binds to the Ins 5’UTR and promotes its translational repression (280). On the contrary, HuD regulates β-cell death and mitochondrial function by binding to the 3’UTRs and increasing the translation of ATG5 (autophagy-related gene 5) and Mfn2 (Mitofusin 2), respectively (281, 282). It would be very interesting to determine whether HuD also modulates miRNA-target interactions in β-cells.
Competition With Other Non-Coding RNAs
Under specific conditions, endogenous long non-coding RNAs (lncRNAs), pseudogenes, mRNAs, and more recently, circular RNAs (circRNAs) are all capable of interfering with miRNA function by competing with the targets for miRNA binding [reviewed in (283)].
CircRNAs are single-stranded RNAs that form covalently closed loops that arise during splicing (284). Thousands of circRNAs are expressed in pancreatic islets, though the function of only a few has been characterized (241, 285). The effect of a circRNA in target miRNA stability may depend on the cellular context. As briefly mentioned above, Cdr1as (antisense of Cerebellar Degeneration-Related Protein 1, also known as ciRS-7, Circular RNA sponge for miR-7) has been proposed to regulate the expression of miR-7 targets in islets and MIN6 cells. Another circRNA present in human islets, circHIPK3 acts as a sponge of miR-124-3p, miR-338-3p, miR-29b-3p, and miR-30 and is downregulated in islets from db/db mice. Deletion of this circRNA in primary rat β-cells impaired proliferation and promoted apoptosis (241). More recently, high-throughput RNA sequencing was used to identify circRNAs dysregulated in db/db islets. One of the downregulated circRNAs, Circ-Tulp4, was also downregulated by HFD and palmitate, and it has been proposed to control cell proliferation by the interaction with miR-7222-3p (285).
The expression of lncRNAs is also regulated by high glucose, palmitate, and HFD (286). For example, the lnRNA LEGLTBC was downregulated by glucolipotoxicity and functions as an endogenous sponge for miR-34a and a regulator of apoptosis (286). Other examples of miRNAs regulated by lncRNA-sponges are miR-181a-5p, regulated by the plasmacytoma variant translocation 1 (PVT1) lncRNA in INS-1 cells (287) and miR-17, regulated by metastasis-associated lung adenocarcinoma transcript 1 (MALAT1).
Importantly, both lncRNAs and circRNAs often regulate gene expression in a miRNA-independent manner. Regazzi’s lab has made important contributions to the understanding of the function of both lncRNAs and circRNAs in β-cells and have recently generated comprehensive reviews on the subject (288, 289).
MiRNA Localization
MiRNA activity can also be modulated by the location and therefore accessibility of the miRNA in and within the cell. Both miRNAs and proteins from the miRISC complex have been found in the nucleus (274, 290), although the functional significance of these miRNAs remains poorly understood. In the nucleus, miRNAs can regulate transcription by promoting chromatin remodelling (291, 292) and have also been suggested to interact with nuclear lncRNAs to modulate their function (293, 294) and to even interact with pri-miRNAs to regulate their processing to mature miRNAs (295). MiRNAs can also be localized into exosomes and represent an important proportion of circulating miRNAs. It is debated whether circulating miRNAs can be merely considered as biomarkers or whether they play regulatory functions. Supporting the latter, increasing numbers of studies demonstrate that cellular-derived exosomal miRNAs can control cellular function and target gene expression in recipient cells, potentially contributing to cross-talk between metabolic organs (296).
The mechanism regulating miRNA localization into exosomes may involve the association with lncRNAs (297), RBPs such as hnRNPA2/B1 or the major vault protein (MVB) (298, 299) and/or Ago proteins (300). Importantly, extracellular miRNAs can also be found free from vesicles, often associated with RBPs or lipoproteins (296, 301, 302). For example, it has been recently shown that β-cells export miR-375 to high-density lipoproteins (HDL) in an specific process tightly regulated by glucose availability, extracellular calcium, and cAMP (303). Several groups have studied changes in the levels of circulating miRNAs in association with hyperglycaemia and/or diabetes. These studies uncovered, for example, a strong association between glycaemia (HbA1c) and elevated circulating miR-125b levels in pre-diabetic (304), T2D (304), and T1D (305) subjects. Moreover, gestational diabetes led to increased serum miR-125b in early pregnancy (306). Our most recent research shows that miR-125b is an abundant islet miRNA regulated by glucose and AMPK (83). Circulating miRNAs may increase as a consequence of damage or alteration of the cells of origin, which in most cases remain to be identified. On the other hand, circulating miRNAs could potentially target β-cells to modulate their function and contribute to the progression of the disease. Pancreatic islets take up and secrete exosomes that affect their own function and that of neighbouring cells (125, 307, 308). For example, lymphocytes infiltrating mouse islets during the development of T1D release exosomal miR-155 that is taken up by β-cells to trigger apoptosis (308). Also, β-cells release miR-29b in response to HFD, which has been proposed to target insulin signalling in the liver (309). On the other hand, the contribution of β-cells to circulating miRNAs (ci-miRNAs) levels has been challenged. For example, only ~1% of the circulating miR-375 originates from β-cells (168), despite being strongly enriched in these cells. Exciting research published in the past few years (310, 311) has identified adipose tissue as an important source of circulating exosomal miRNAs. The liver, skeletal muscle, and perhaps, the gut have also been suggested to release and intake miRNA-containing extracellular vesicles (312). Even though little is known about the destiny and function of ci-miRNAs derived from each of these tissues, considerable effort has been put into identifying the cellular origin of ci-miRNAs. We anticipate that similar studies to those performed by Thomou and colleagues (310), who assessed changes in circulating miRNAs following the elimination of Dicer in adipocytes using an adipocyte-specific Cre deleter strain, may be useful to ascertain the contribution of pancreatic β-cells, and perhaps other islet cells, to circulating miRNAs.
Conclusions and Perspectives
Research during the past 15 years has clearly demonstrated that β-cell miRNAs play an essential role in glucose-stimulated insulin secretion and cell survival. It has also been widely demonstrated that diet modulates the expression of islet miRNAs. These nutrient-regulated miRNAs contribute not only to β-cell compensation but also to the β-cell functional failure and death mediated by hyperglycaemia and excess of fatty acids. Nevertheless, the molecular mechanisms leading to these changes in miRNA expression remain mostly unexplored. For example, only a few individual miRNA-transcription factor connections have been studied to some detail. As discussed in detail above, one of the constraints is the lack of accurate annotations of miRNAs transcription start sites. Hopefully, state-of-the-art approaches such as those described above be applied to identify β-cell/islet-specific miRNAs TSS and to study their transcriptional regulation in a high-throughput manner. Moreover, a precise mapping of islet miRNA TSS will also allow us to determine whether Single-Nucleotide Polymorphisms (SNPs) associated with diabetes traits rely within pri-miRNAs or regulatory regions of miRNA genes. This information can be leveraged to identify miRNAs with an important role in the maintenance of glucose homeostasis.
Pancreatic beta cells within the islets have been shown to be heterogeneous at multiple levels (313) and to present a functional and transcriptional heterogeneous response to high-fat diet (314, 315). Whereas single-cell RNA sequencing (scRNA-seq) techniques have evolved considerably during the past few years and have helped to understand β-cell functional heterogeneity, single-cell small RNA-seq has only started to be developed (316). Single-cell miRNA profiling can be used to further our understanding of the molecular mechanisms underlying cellular heterogeneity (317) and to determine whether β-cell changes in miRNA expression in response to nutrients are also heterogeneous.
It is now well established that miRNAs processing and turnover are tightly regulated processes that can have a substantial impact on steady-state cellular miRNA abundance. Moreover, it is now clear that miRNA activity depends not only on miRNA abundance but also on target accessibility. Research in the past recent years has provided novel mechanistic insights into the processes that govern miRNA-target interactions, uncovering important roles for RBPs and other ncRNAs. These regulatory mechanisms play an important part in controlling the function of miRNAs in neurons, which display multiple functional similarities and common regulatory mechanisms of gene expression to β-cells. Nevertheless, the contribution of these pathways to nutrient-mediated changes in β-cell miRNA expression and action remains chiefly unexplored and constitute an exciting new research avenue in the field
Future studies will hopefully fill all these gaps and provide important new findings that will allow us to better understand the contribution of miRNAs to β-cell dysfunction.
Author Contributions
Both AS and AM-S contributed to the critical review of the literature and drafting of the manuscript. Both authors contributed to the article and approved the submitted version.
Funding
This work was supported by a New Investigator Research Grant from the Medical Research Council (MRC, MR/P023223/1).
Conflict of Interest
The authors declare that the research was conducted in the absence of any commercial or financial relationships that could be construed as a potential conflict of interest.
Publisher’s Note
All claims expressed in this article are solely those of the authors and do not necessarily represent those of their affiliated organizations, or those of the publisher, the editors and the reviewers. Any product that may be evaluated in this article, or claim that may be made by its manufacturer, is not guaranteed or endorsed by the publisher.
References
2. Rutter GA, Pullen TJ, Hodson DJ, Martinez-Sanchez A. Pancreatic Beta Cell Identity, Glucose Sensing and the Control of Insulin Secretion. Biochem J (2015), 202–18.
3. Chen C, Cohrs CM, Stertmann J, Bozsak R, Speier S. Human Beta Cell Mass and Function in Diabetes: Recent Advances in Knowledge and Technologies to Understand Disease Pathogenesis. Mol Metab (2017) 6(9):943–57. doi: 10.1016/j.molmet.2017.06.019
4. Porat S, Weinberg-Corem N, Tornovsky-Babaey S, Schyr-Ben-Haroush R, Hija A, Stolovich-Rain M, et al. Control of Pancreatic β Cell Regeneration by Glucose Metabolism. Cell Metab (2011) 13(4):440–9. doi: 10.1016/j.cmet.2011.02.012
5. Swisa A, Glaser B, Dor Y. Metabolic Stress and Compromised Identity of Pancreatic Beta Cells. Front Genet (2017) 8:21. doi: 10.3389/fgene.2017.00021
6. Guilherme A, Virbasius JV, Puri V, Czech MP. Adipocyte Dysfunctions Linking Obesity to Insulin Resistance and Type 2 Diabetes. Nat Rev Mol Cell Biol (2008) 9(5):367–77. doi: 10.1038/nrm2391
7. Cerf ME. Beta Cell Dysfunction and Insulin Resistance. Front Endocrinol (Lausanne) (2013) 4:37. doi: 10.3389/fendo.2013.00037
8. Garcia TS, Rech TH, Leitão CB. Pancreatic Size and Fat Content in Diabetes: A Systematic Review and Meta-Analysis of Imaging Studies. PLoS One (2017) 12(7):e0180911. doi: 10.1371/journal.pone.0180911
10. Poy MN, Eliasson L, Krutzfeldt J, Kuwajima S, Ma X, Macdonald PE, et al. A Pancreatic Islet-Specific microRNA Regulates Insulin Secretion. Nature (2004) 432(7014):226–30. doi: 10.1038/nature03076
11. Lynn FC, Skewes-Cox P, Kosaka Y, McManus MT, Harfe BD, German MS. MicroRNA Expression Is Required for Pancreatic Islet Cell Genesis in the Mouse. Diabetes (2007) 56(12):2938–45. doi: 10.2337/db07-0175
12. Martinez-Sanchez A, Rutter GA, Latreille M. MiRNAs in β-Cell Development, Identity, and Disease. Front Genet (2016) 7:226. doi: 10.3389/fgene.2016.00226
13. LaPierre MP, Stoffel M. MicroRNAs as Stress Regulators in Pancreatic Beta Cells and Diabetes. Mol Metab (2017) 6(9):1010–23. doi: 10.1016/j.molmet.2017.06.020
14. Grieco GE, Brusco N, Licata G, Fignani D, Formichi C, Nigi L, et al. The Landscape of microRNAs in βcell: Between Phenotype Maintenance and Protection. Int J Mol Sci (2021) 22(2). doi: 10.3390/ijms22020803
15. Ramalingam P, Palanichamy JK, Singh A, Das P, Bhagat M, Kassab MA, et al. Biogenesis of Intronic miRNAs Located in Clusters by Independent Transcription and Alternative Splicing. RNA (2014) 20(1):76–87. doi: 10.1261/rna.041814.113
16. Cai X, Hagedorn CH, Cullen BR. Human microRNAs Are Processed From Capped, Polyadenylated Transcripts That Can Also Function as mRNAs. RNA (2004) 10(12):1957–66. doi: 10.1261/rna.7135204
17. Lee Y, Kim M, Han J, Yeom KH, Lee S, Baek SH, et al. MicroRNA Genes Are Transcribed by RNA Polymerase II. EMBO J (2004) 23(20):4051–60. doi: 10.1038/sj.emboj.7600385
18. Denli AM, Tops BB, Plasterk RH, Ketting RF, Hannon GJ. Processing of Primary MicroRNAs by the Microprocessor Complex. Nature (2004) 432(7014):231–5. doi: 10.1038/nature03049
19. Chang TC, Pertea M, Lee S, Salzberg SL, Mendell JT. Genome-Wide Annotation of microRNA Primary Transcript Structures Reveals Novel Regulatory Mechanisms. Genome Res (2015) 25(9):1401–9. doi: 10.1101/gr.193607.115
20. Gregory RI, Yan KP, Amuthan G, Chendrimada T, Doratotaj B, Cooch N, et al. The Microprocessor Complex Mediates the Genesis of microRNAs. Nature (2004) 432(7014):235–40. doi: 10.1038/nature03120
21. Lee Y, Ahn C, Han J, Choi H, Kim J, Yim J, et al. The Nuclear RNase III Drosha Initiates microRNA Processing. Nature (2003) 425(6956):415–9. doi: 10.1038/nature01957
22. Han J, Lee Y, Yeom KH, Kim YK, Jin H, Kim VN. The Drosha-DGCR8 Complex in Primary microRNA Processing. Genes Dev (2004) 18(24):3016–27. doi: 10.1101/gad.1262504
23. Nguyen TA, Jo MH, Choi YG, Park J, Kwon SC, Hohng S, et al. Functional Anatomy of the Human Microprocessor. Cell (2015) 161(6):1374–87. doi: 10.1016/j.cell.2015.05.010
24. Yi R, Qin Y, Macara IG, Cullen BR. Exportin-5 Mediates the Nuclear Export of Pre-microRNAs and Short Hairpin RNAs. Genes Dev (2003) 17(24):3011–6. doi: 10.1101/gad.1158803
25. Lund E, Güttinger S, Calado A, Dahlberg JE, Kutay U. Nuclear Export of microRNA Precursors. Science (2004) 303(5654):95–8. doi: 10.1126/science.1090599
26. Ketting RF, Fischer SE, Bernstein E, Sijen T, Hannon GJ, Plasterk RH. Dicer Functions in RNA Interference and in Synthesis of Small RNA Involved in Developmental Timing in C. Elegans. Genes Dev (2001) 15(20):2654–9. doi: 10.1101/gad.927801
27. Hutvágner G, McLachlan J, Pasquinelli AE, Bálint E, Tuschl T, Zamore PD. A Cellular Function for the RNA-Interference Enzyme Dicer in the Maturation of the Let-7 Small Temporal RNA. Science (2001) 293(5531):834–8. doi: 10.1126/science.1062961
28. Iwasaki S, Kobayashi M, Yoda M, Sakaguchi Y, Katsuma S, Suzuki T, et al. Hsc70/Hsp90 Chaperone Machinery Mediates ATP-Dependent RISC Loading of Small RNA Duplexes. Mol Cell (2010) 39(2):292–9. doi: 10.1016/j.molcel.2010.05.015
29. Kawamata T, Tomari Y. Making RISC. Trends Biochem Sci (2010) 35(7):368–76. doi: 10.1016/j.tibs.2010.03.009
30. Frank F, Sonenberg N, Nagar B. Structural Basis for 5’-Nucleotide Base-Specific Recognition of Guide RNA by Human AGO2. Nature (2010) 465(7299):818–22. doi: 10.1038/nature09039
31. Khvorova A, Reynolds A, Jayasena SD. Functional siRNAs and miRNAs Exhibit Strand Bias. Cell (2003) 115(2):209–16. doi: 10.1016/S0092-8674(03)00801-8
32. Yoda M, Kawamata T, Paroo Z, Ye X, Iwasaki S, Liu Q, et al. ATP-Dependent Human RISC Assembly Pathways. Nat Struct Mol Biol (2010) 17(1):17–23. doi: 10.1038/nsmb.1733
33. Broughton JP, Lovci MT, Huang JL, Yeo GW, Pasquinelli AE. Pairing Beyond the Seed Supports MicroRNA Targeting Specificity. Mol Cell (2016) 64(2):320–33. doi: 10.1016/j.molcel.2016.09.004
34. Bartel DP. MicroRNAs: Target Recognition and Regulatory Functions. Cell (2009) 136(2):215–33. doi: 10.1016/j.cell.2009.01.002
35. Agarwal V, Bell GW, Nam JW, Bartel DP. Predicting Effective microRNA Target Sites in Mammalian mRNAs. Elife (2015) 4.
36. Helwak A, Kudla G, Dudnakova T, Tollervey D. Mapping the Human miRNA Interactome by CLASH Reveals Frequent Noncanonical Binding. Cell (2013) 153(3):654–65. doi: 10.1016/j.cell.2013.03.043
37. Moore MJ, Scheel TK, Luna JM, Park CY, Fak JJ, Nishiuchi E, et al. miRNA-Target Chimeras Reveal miRNA 3’-End Pairing as a Major Determinant of Argonaute Target Specificity. Nat Commun (2015) 6:8864. doi: 10.1038/ncomms9864
38. Gebert LFR, MacRae IJ. Regulation of microRNA Function in Animals. Nat Rev Mol Cell Biol (2019) 20(1):21–37. doi: 10.1038/s41580-018-0045-7
39. Eichhorn SW, Guo H, McGeary SE, Rodriguez-Mias RA, Shin C, Baek D, et al. mRNA Destabilization Is the Dominant Effect of Mammalian microRNAs by the Time Substantial Repression Ensues. Mol Cell (2014) 56(1):104–15. doi: 10.1016/j.molcel.2014.08.028
40. Subtelny AO, Eichhorn SW, Chen GR, Sive H, Bartel DP. Poly(A)-Tail Profiling Reveals an Embryonic Switch in Translational Control. Nature (2014) 508(7494):66–71. doi: 10.1038/nature13007
41. Martinez-Sanchez A, Lazzarano S, Sharma E, Lockstone H, Murphy CL. High-Throughput Identification of MiR-145 Targets in Human Articular Chondrocytes. Life (Basel) (2020) 10(5). doi: 10.3390/life10050058
42. Martinez-Sanchez A, Dudek KA, Murphy CL. Regulation of Human Chondrocyte Function Through Direct Inhibition of Cartilage Master Regulator SOX9 by microRNA-145 (miRNA-145). J Biol Chem (2012) 287(2):916–24. doi: 10.1074/jbc.M111.302430
43. Cuesta R, Martínez-Sánchez A, Gebauer F. miR-181a Regulates Cap-Dependent Translation of P27(Kip1) mRNA in Myeloid Cells. Mol Cell Biol (2009) 29(10):2841–51. doi: 10.1128/MCB.01971-08
44. van Rooij E, Sutherland LB, Qi X, Richardson JA, Hill J, Olson EN. Control of Stress-Dependent Cardiac Growth and Gene Expression by a microRNA. Science (2007) 316(5824):575–9. doi: 10.1126/science.1139089
45. Wu S, Huang S, Ding J, Zhao Y, Liang L, Liu T, et al. Multiple microRNAs Modulate p21Cip1/Waf1 Expression by Directly Targeting its 3’ Untranslated Region. Oncogene (2010) 29(15):2302–8. doi: 10.1038/onc.2010.34
46. Selbach M, Schwanhausser B, Thierfelder N, Fang Z, Khanin R, Rajewsky N. Widespread Changes in Protein Synthesis Induced by microRNAs. Nature (2008) 455(7209):58–63. doi: 10.1038/nature07228
47. Ebert MS, Sharp PA. Roles for microRNAs in Conferring Robustness to Biological Processes. Cell (2012) 149(3):515–24. doi: 10.1016/j.cell.2012.04.005
48. Gurtan AM, Sharp PA. The Role of miRNAs in Regulating Gene Expression Networks. J Mol Biol (2013) 425(19):3582–600. doi: 10.1016/j.jmb.2013.03.007
49. Yang JS, Lai EC. Alternative miRNA Biogenesis Pathways and the Interpretation of Core miRNA Pathway Mutants. Mol Cell (2011) 43(6):892–903. doi: 10.1016/j.molcel.2011.07.024
50. Dexheimer PJ, Cochella L. MicroRNAs: From Mechanism to Organism. Front Cell Dev Biol (2020) 8:409. doi: 10.3389/fcell.2020.00409
51. Kanji MS, Martin MG, Bhushan A. Dicer1 Is Required to Repress Neuronal Fate During Endocrine Cell Maturation. Diabetes (2013) 62(5):1602–11. doi: 10.2337/db12-0841
52. Kalis M, Bolmeson C, Esguerra JL, Gupta S, Edlund A, Tormo-Badia N, et al. Beta-Cell Specific Deletion of Dicer1 Leads to Defective Insulin Secretion and Diabetes Mellitus. PLoS One (2011) 6(12):e29166. doi: 10.1371/journal.pone.0029166
53. Mandelbaum AD, Melkman-Zehavi T, Oren R, Kredo-Russo S, Nir T, Dor Y, et al. Dysregulation of Dicer1 in Beta Cells Impairs Islet Architecture and Glucose Metabolism. Exp Diabetes Res 2012 (2012) p:470302. doi: 10.1155/2012/470302
54. Melkman-Zehavi T, Oren R, Kredo-Russo S, Shapira T, Mandelbaum AD, Rivkin N, et al. miRNAs Control Insulin Content in Pancreatic Beta-Cells via Downregulation of Transcriptional Repressors. EMBO J (2011) 30(5):835–45. doi: 10.1038/emboj.2010.361
55. Martinez-Sanchez A, Nguyen-Tu MS, Rutter GA. DICER Inactivation Identifies Pancreatic Beta-Cell “Disallowed” Genes Targeted by MicroRNAs. Mol Endocrinol (2015) 29(7):1067–79. doi: 10.1210/me.2015-1059
56. Zhang ZW, Zhang LQ, Ding L, Wang F, Sun YJ, An Y, et al. MicroRNA-19b Downregulates Insulin 1 Through Targeting Transcription Factor Neurod1. FEBS Lett (2011) 585(16):2592–8. doi: 10.1016/j.febslet.2011.06.039
57. Nieto M, Hevia P, Garcia E, Klein D, Alvarez-Cubela S, Bravo-Egana V, et al. Antisense miR-7 Impairs Insulin Expression in Developing Pancreas and in Cultured Pancreatic Buds. Cell Transplant (2012) 21(8):1761–74. doi: 10.3727/096368911X612521
58. Setyowati Karolina D, Sepramaniam S, Tan HZ, Armugam A, Jeyaseelan K. miR-25 and miR-92a Regulate Insulin I Biosynthesis in Rats. RNA Biol (2013) 10(8):1365–78. doi: 10.4161/rna.25557
59. Xu H, Guo S, Li W, Yu P. The Circular RNA Cdr1as, via miR-7 and Its Targets, Regulates Insulin Transcription and Secretion in Islet Cells. Sci Rep (2015) 5:12453. doi: 10.1038/srep12453
60. Plaisance V, Abderrahmani A, Perret-Menoud V, Jacquemin P, Lemaigre F, Regazzi R. MicroRNA-9 Controls the Expression of Granuphilin/Slp4 and the Secretory Response of Insulin-Producing Cells. J Biol Chem (2006) 281(37):26932–42. doi: 10.1074/jbc.M601225200
61. Lovis P, Gattesco S, Regazzi R. Regulation of the Expression of Components of the Exocytotic Machinery of Insulin-Secreting Cells by microRNAs. Biol Chem (2008) 389(3):305–12. doi: 10.1515/BC.2008.026
62. Ruan Q, Wang T, Kameswaran V, Wei Q, Johnson DS, Matschinsky F, et al. The microRNA-21-PDCD4 Axis Prevents Type 1 Diabetes by Blocking Pancreatic Beta Cell Death. Proc Natl Acad Sci USA (2011) 108(29):12030–5. doi: 10.1073/pnas.1101450108
63. Lovis P, Roggli E, Laybutt DR, Gattesco S, Yang JY, Widmann C, et al. Alterations in microRNA Expression Contribute to Fatty Acid-Induced Pancreatic Beta-Cell Dysfunction. Diabetes (2008) 57(10):2728–36. doi: 10.2337/db07-1252
64. Tattikota SG, Rathjen T, McAnulty SJ, Wessels HH, Akerman I, van de Bunt M, et al. Argonaute2 Mediates Compensatory Expansion of the Pancreatic Beta Cell. Cell Metab (2014) 19(1):122–34. doi: 10.1016/j.cmet.2013.11.015
65. Pullen TJ, da Silva Xavier G, Kelsey G, Rutter GA. miR-29a and miR-29b Contribute to Pancreatic Beta-Cell-Specific Silencing of Monocarboxylate Transporter 1 (Mct1). Mol Cell Biol (2011) 31(15):3182–94. doi: 10.1128/MCB.01433-10
66. Tugay K, Guay C, Marques AC, Allagnat F, Locke JM, Harries LW, et al. Role of microRNAs in the Age-Associated Decline of Pancreatic Beta Cell Function in Rat Islets. Diabetologia (2016) 59(1):161–9. doi: 10.1007/s00125-015-3783-5
67. Kredo-Russo S, Mandelbaum AD, Ness A, Alon I, Lennox KA, Behlke MA, et al. Pancreas-Enriched miRNA Refines Endocrine Cell Differentiation. Development (2012) 139(16):3021–31. doi: 10.1242/dev.080127
68. Latreille M, Hausser J, Stutzer I, Zhang Q, Hastoy B, Gargani S, et al. MicroRNA-7a Regulates Pancreatic Beta Cell Function. J Clin Invest (2014) 124(6):2722–35. doi: 10.1172/JCI73066
69. Guay C, Regazzi R. New Emerging Tasks for microRNAs in the Control of β-Cell Activities. Biochim Biophys Acta (2016). doi: 10.1016/j.bbalip.2016.05.003
70. Jonas JC, Sharma A, Hasenkamp W, Ilkova H, Patanè G, Laybutt R, et al. Chronic Hyperglycemia Triggers Loss of Pancreatic Beta Cell Differentiation in an Animal Model of Diabetes. J Biol Chem (1999) 274(20):14112–21. doi: 10.1074/jbc.274.20.14112
71. Talchai C, Xuan S, Lin HV, Sussel L, Accili D. Pancreatic Beta Cell Dedifferentiation as a Mechanism of Diabetic Beta Cell Failure. Cell (2012) 150(6):1223–34. doi: 10.1016/j.cell.2012.07.029
72. Jeffery N, Harries LW. β-Cell Differentiation Status in Type 2 Diabetes. Diabetes Obes Metab (2016). doi: 10.1111/dom.12778
73. Schuit F, Flamez D, De Vos A, Pipeleers D. Glucose-Regulated Gene Expression Maintaining the Glucose-Responsive State of Beta-Cells. Diabetes (2002) 51 Suppl 3:S326–32.
74. Ebrahimi AG, Hollister-Lock J, Sullivan BA, Tsuchida R, Bonner-Weir S, Weir GC. Beta Cell Identity Changes With Mild Hyperglycemia: Implications for Function, Growth, and Vulnerability. Mol Metab (2020) 35:100959. doi: 10.1016/j.molmet.2020.02.002
75. Kahn SE, Hull RL, Utzschneider KM. Mechanisms Linking Obesity to Insulin Resistance and Type 2 Diabetes. Nature (2006) 444(7121):840–6. doi: 10.1038/nature05482
76. Sharma RB, Alonso LC. Lipotoxicity in the Pancreatic Beta Cell: Not Just Survival and Function, But Proliferation as Well? Curr Diabetes Rep (2014) 14(6):492. doi: 10.1007/s11892-014-0492-2
77. Wang YW, Sun GD, Sun J, Liu SJ, Wang J, Xu XH, et al. Spontaneous Type 2 Diabetic Rodent Models. J Diabetes Res (2013) 2013:401723. doi: 10.1155/2013/401723
78. Tang X, Muniappan L, Tang G, Ozcan S. Identification of Glucose-Regulated miRNAs From Pancreatic {Beta} Cells Reveals a Role for miR-30d in Insulin Transcription. RNA (2009) 15(2):287–93. doi: 10.1261/rna.1211209
79. Herrera BM, Lockstone HE, Taylor JM, Ria M, Barrett A, Collins S, et al. Global microRNA Expression Profiles in Insulin Target Tissues in a Spontaneous Rat Model of Type 2 Diabetes. Diabetologia (2010) 53(6):1099–109. doi: 10.1007/s00125-010-1667-2
80. Zhu H, Leung SW. Identification of microRNA Biomarkers in Type 2 Diabetes: A Meta-Analysis of Controlled Profiling Studies. Diabetologia (2015) 58(5):900–11. doi: 10.1007/s00125-015-3510-2
81. Esguerra JL, Bolmeson C, Cilio CM, Eliasson L. Differential Glucose-Regulation of microRNAs in Pancreatic Islets of Non-Obese Type 2 Diabetes Model Goto-Kakizaki Rat. PLoS One (2011) 6(4):e18613. doi: 10.1371/journal.pone.0018613
82. Sebastiani G, Po A, Miele E, Ventriglia G, Ceccarelli E, Bugliani M, et al. MicroRNA-124a Is Hyperexpressed in Type 2 Diabetic Human Pancreatic Islets and Negatively Regulates Insulin Secretion. Acta Diabetol (2015) 52(3):523–30. doi: 10.1007/s00592-014-0675-y
83. Cheung RWS, Pizza G, Chabosseau P, Rolando D, Tomas A, Burgoyne T, et al. Glucose-Dependent miR-125b Is a Negative Regulator of β-Cell Function. bioRxiv (2021), 2021.05.17.444559.
84. Shen Y, Xu H, Pan X, Wu W, Wang H, Yan L, et al. miR-34a and miR-125b Are Upregulated in Peripheral Blood Mononuclear Cells From Patients With Type 2 Diabetes Mellitus. Exp Ther Med (2017) 14(6):5589–96. doi: 10.3892/etm.2017.5254
85. Klein D, Misawa R, Bravo-Egana V, Vargas N, Rosero S, Piroso J, et al. MicroRNA Expression in Alpha and Beta Cells of Human Pancreatic Islets. PLoS One (2013) 8(1):e55064. doi: 10.1371/journal.pone.0055064
86. Yu CY, Yang CY, Rui ZL. MicroRNA-125b-5p Improves Pancreatic Beta-Cell Function Through Inhibiting JNK Signaling Pathway by Targeting DACT1 in Mice With Type 2 Diabetes Mellitus. Life Sci (2019) 224:67–75. doi: 10.1016/j.lfs.2019.01.031
87. Zhao E, Keller MP, Rabaglia ME, Oler AT, Stapleton DS, Schueler KL, et al. Obesity and Genetics Regulate microRNAs in Islets, Liver, and Adipose of Diabetic Mice. Mamm Genome (2009) 20(8):476–85. doi: 10.1007/s00335-009-9217-2
88. Jimenez-Lucena R, Rangel-Zuniga OA, Alcala-Diaz JF, Lopez-Moreno J, Roncero-Ramos I, Molina-Abril H, et al. Circulating miRNAs as Predictive Biomarkers of Type 2 Diabetes Mellitus Development in Coronary Heart Disease Patients From the CORDIOPREV Study. Mol Ther Nucleic Acids (2018) 12:146–57. doi: 10.1016/j.omtn.2018.05.002
89. Zhang T, Lv C, Li L, Chen S, Liu S, Wang C, et al. Plasma miR-126 Is a Potential Biomarker for Early Prediction of Type 2 Diabetes Mellitus in Susceptible Individuals. BioMed Res Int (2013) 2013:761617. doi: 10.1155/2013/761617
90. Kim M, Zhang X. The Profiling and Role of miRNAs in Diabetes Mellitus. J Diabetes Clin Res (2019) 1(1):5–23.
91. Seyhan AA, Nunez Lopez YO, Xie H, Yi F, Mathews C, Pasarica M, et al. Pancreas-Enriched miRNAs Are Altered in the Circulation of Subjects With Diabetes: A Pilot Cross-Sectional Study. Sci Rep (2016) 6:31479. doi: 10.1038/srep31479
92. Olivieri F, Spazzafumo L, Bonafe M, Recchioni R, Prattichizzo F, Marcheselli F, et al. MiR-21-5p and miR-126a-3p Levels in Plasma and Circulating Angiogenic Cells: Relationship With Type 2 Diabetes Complications. Oncotarget (2015) 6(34):35372–82. doi: 10.18632/oncotarget.6164
93. Shen Z, Jiang H, Hsu HT, Qian L, Fu Q, Shen M, et al. MicroRNA-127 Inhibits Cell Proliferation via Targeting Kif3b in Pancreatic Beta Cells. Aging (Albany NY) (2019) 11(5):1342–55. doi: 10.18632/aging.101835
94. Bolmeson C, Esguerra JL, Salehi A, Speidel D, Eliasson L, Cilio CM. Differences in Islet-Enriched miRNAs in Healthy and Glucose Intolerant Human Subjects. Biochem Biophys Res Commun (2011) 404(1):16–22. doi: 10.1016/j.bbrc.2010.11.024
95. Ofori JK, Salunkhe VA, Bagge A, Vishnu N, Nagao M, Mulder H, et al. Elevated miR-130a/Mir130b/miR-152 Expression Reduces Intracellular ATP Levels in the Pancreatic Beta Cell. Sci Rep (2017) 7:44986. doi: 10.1038/srep44986
96. Yerlikaya FH, Öz M. Aberrant Expression of miRNA Profiles in High-Fat and High-Sucrose Fed Rats. Clin Nutr Exp (2019) 27:1–8. doi: 10.1016/j.yclnex.2019.07.001
97. Nesca V, Guay C, Jacovetti C, Menoud V, Peyot ML, Laybutt DR, et al. Identification of Particular Groups of microRNAs That Positively or Negatively Impact on Beta Cell Function in Obese Models of Type 2 Diabetes. Diabetologia (2013) 56(10):2203–12. doi: 10.1007/s00125-013-2993-y
98. Bijkerk R, Esguerra JLS, Ellenbroek JH, Au YW, Hanegraaf MAJ, de Koning EJ, et al. In Vivo Silencing of MicroRNA-132 Reduces Blood Glucose and Improves Insulin Secretion. Nucleic Acid Ther (2019) 29(2):67–72. doi: 10.1089/nat.2018.0763
99. Fred RG, Bang-Berthelsen CH, Mandrup-Poulsen T, Grunnet LG, Welsh N. High Glucose Suppresses Human Islet Insulin Biosynthesis by Inducing miR-133a Leading to Decreased Polypyrimidine Tract Binding Protein-Expression. PLoS One (2010) 5(5):e10843. doi: 10.1371/journal.pone.0010843
100. Delic D, Eisele C, Schmid R, Luippold G, Mayoux E, Grempler R. Characterization of Micro-RNA Changes During the Progression of Type 2 Diabetes in Zucker Diabetic Fatty Rats. Int J Mol Sci (2016) 17(5).
101. de Gonzalo-Calvo D, van der Meer RW, Rijzewijk LJ, Smit JW, Revuelta-Lopez E, Nasarre L, et al. Serum microRNA-1 and microRNA-133a Levels Reflect Myocardial Steatosis in Uncomplicated Type 2 Diabetes. Sci Rep (2017) 7(1):47. doi: 10.1038/s41598-017-00070-6
102. Belgardt BF, Ahmed K, Spranger M, Latreille M, Denzler R, Kondratiuk N, et al. The microRNA-200 Family Regulates Pancreatic Beta Cell Survival in Type 2 Diabetes. Nat Med (2015) 21(6):619–27. doi: 10.1038/nm.3862
103. Zhang T, Ji C, Shi R. miR-142-3p Promotes Pancreatic Beta Cell Survival Through Targeting FOXO1 in Gestational Diabetes Mellitus. Int J Clin Exp Pathol (2019) 12(5):1529–38.
104. Jordan SD, Kruger M, Willmes DM, Redemann N, Wunderlich FT, Bronneke HS, et al. Obesity-Induced Overexpression of miRNA-143 Inhibits Insulin-Stimulated AKT Activation and Impairs Glucose Metabolism. Nat Cell Biol (2011) 13(4):434–46. doi: 10.1038/ncb2211
105. Kang MH, Zhang LH, Wijesekara N, de Haan W, Butland S, Bhattacharjee A, et al. Regulation of ABCA1 Protein Expression and Function in Hepatic and Pancreatic Islet Cells by miR-145. Arterioscler Thromb Vasc Biol (2013) 33(12):2724–32. doi: 10.1161/ATVBAHA.113.302004
106. Karolina DS, Armugam A, Tavintharan S, Wong MT, Lim SC, Sum CF, et al. MicroRNA 144 Impairs Insulin Signaling by Inhibiting the Expression of Insulin Receptor Substrate 1 in Type 2 Diabetes Mellitus. PLoS One (2011) 6(8):e22839. doi: 10.1371/journal.pone.0022839
107. Cui X, You L, Zhu L, Wang X, Zhou Y, Li Y, et al. Change in Circulating microRNA Profile of Obese Children Indicates Future Risk of Adult Diabetes. Metabolism (2018) 78:95–105. doi: 10.1016/j.metabol.2017.09.006
108. Kong L, Zhu J, Han W, Jiang X, Xu M, Zhao Y, et al. Significance of Serum microRNAs in Pre-Diabetes and Newly Diagnosed Type 2 Diabetes: A Clinical Study. Acta Diabetol (2011) 48(1):61–9. doi: 10.1007/s00592-010-0226-0
109. Rong Y, Bao W, Shan Z, Liu J, Yu X, Xia S, et al. Increased microRNA-146a Levels in Plasma of Patients With Newly Diagnosed Type 2 Diabetes Mellitus. PLoS One (2013) 8(9):e73272. doi: 10.1371/journal.pone.0073272
110. Baldeon RL, Weigelt K, de Wit H, Ozcan B, van Oudenaren A, Sempertegui F, et al. Decreased Serum Level of miR-146a as Sign of Chronic Inflammation in Type 2 Diabetic Patients. PLoS One (2014) 9(12):e115209. doi: 10.1371/journal.pone.0115209
111. Balasubramanyam M, Aravind S, Gokulakrishnan K, Prabu P, Sathishkumar C, Ranjani H, et al. Impaired miR-146a Expression Links Subclinical Inflammation and Insulin Resistance in Type 2 Diabetes. Mol Cell Biochem (2011) 351(1-2):197–205. doi: 10.1007/s11010-011-0727-3
112. Chen L, Qian H, Xue J, Zhang J, Chen H. MicroRNA152 Regulates Insulin Secretion and Pancreatic Beta Cell Proliferation by Targeting PI3Kalpha. Mol Med Rep (2018) 18(4):4113–21. doi: 10.3892/mmr.2018.9359
113. Mandemakers W, Abuhatzira L, Xu H, Caromile LA, Hébert SS, Snellinx A, et al. Co-Regulation of Intragenic microRNA miR-153 and Its Host Gene Ia-2β: Identification of miR-153 Target Genes With Functions Related to IA-2β in Pancreas and Brain. Diabetologia (2013) 56(7):1547–56. doi: 10.1007/s00125-013-2901-5
114. Sun Y, Zhou S, Shi Y, Zhou Y, Zhang Y, Liu K, et al. Inhibition of miR-153, an IL-1beta-Responsive miRNA, Prevents Beta Cell Failure and Inflammation-Associated Diabetes. Metabolism (2020) 111:154335. doi: 10.1016/j.metabol.2020.154335
115. Xu H, Abuhatzira L, Carmona GN, Vadrevu S, Satin LS, Notkins AL. The Ia-2beta Intronic miRNA, miR-153, Is a Negative Regulator of Insulin and Dopamine Secretion Through Its Effect on the Cacna1c Gene in Mice. Diabetologia (2015) 58(10):2298–306. doi: 10.1007/s00125-015-3683-8
116. Sun LL, Jiang BG, Li WT, Zou JJ, Shi YQ, Liu ZM. MicroRNA-15a Positively Regulates Insulin Synthesis by Inhibiting Uncoupling Protein-2 Expression. Diabetes Res Clin Pract (2011) 91(1):94–100. doi: 10.1016/j.diabres.2010.11.006
117. Jacovetti C, Matkovich SJ, Rodriguez-Trejo A, Guay C, Regazzi R. Postnatal Beta-Cell Maturation Is Associated With Islet-Specific microRNA Changes Induced by Nutrient Shifts at Weaning. Nat Commun (2015) 6:8084. doi: 10.1038/ncomms9084
118. Martinez-Sanchez A, Nguyen-Tu MS, Cebola I, Yavari A, Marchetti P, Piemonti L, et al. MiR-184 Expression Is Regulated by AMPK in Pancreatic Islets. FASEB J (2018) 32(5):2587–600. doi: 10.1096/fj.201701100R
119. Tattikota SG, Rathjen T, Hausser J, Khedkar A, Kabra UD, Pandey V, et al. miR-184 Regulates Pancreatic Beta-Cell Function According to Glucose Metabolism. J Biol Chem (2015) 290(33):20284–94. doi: 10.1074/jbc.M115.658625
120. Tattikota SG, Rathjen T, McAnulty SJ, Wessels H-H, Akerman I, van de Bunt M, et al. Argonaute2 Mediates Compensatory Expansion of the Pancreatic β Cell. Cell Metab (2014) 19(1):122–34. doi: 10.1016/j.cmet.2013.11.015
121. Bao L, Fu X, Si M, Wang Y, Ma R, Ren X, et al. MicroRNA-185 Targets SOCS3 to Inhibit Beta-Cell Dysfunction in Diabetes. PLoS One (2015) 10(2):e0116067. doi: 10.1371/journal.pone.0116067
122. Kameswaran V, Bramswig NC, McKenna LB, Penn M, Schug J, Hand NJ, et al. Epigenetic Regulation of the DLK1-MEG3 microRNA Cluster in Human Type 2 Diabetic Islets. Cell Metab (2014) 19(1):135–45. doi: 10.1016/j.cmet.2013.11.016
123. Locke JM, da Silva Xavier G, Dawe HR, Rutter GA, Harries LW. Increased Expression of miR-187 in Human Islets From Individuals With Type 2 Diabetes Is Associated With Reduced Glucose-Stimulated Insulin Secretion. Diabetologia (2014) 57(1):122–8. doi: 10.1007/s00125-013-3089-4
124. Werneck-de-Castro JP, Blandino-Rosano M, Hilfiker-Kleiner D, Bernal-Mizrachi E. Glucose Stimulates microRNA-199 Expression in Murine Pancreatic β-Cells. J Biol Chem (2020) 295(5):1261–70. doi: 10.1016/S0021-9258(17)49884-X
125. Zhang A, Li D, Liu Y, Li J, Zhang Y, Zhang CY. Islet Beta Cell: An Endocrine Cell Secreting miRNAs. Biochem Biophys Res Commun (2018) 495(2):1648–54. doi: 10.1016/j.bbrc.2017.12.028
126. Dangwal S, Stratmann B, Bang C, Lorenzen JM, Kumarswamy R, Fiedler J, et al. Impairment of Wound Healing in Patients With Type 2 Diabetes Mellitus Influences Circulating MicroRNA Patterns via Inflammatory Cytokines. Arterioscler Thromb Vasc Biol (2015) 35(6):1480–8. doi: 10.1161/ATVBAHA.114.305048
127. Xu G, Chen J, Jing G, Shalev A. Thioredoxin-Interacting Protein Regulates Insulin Transcription Through microRNA-204. Nat Med (2013) 19(9):1141–6. doi: 10.1038/nm.3287
128. Gaddam RR, Kim YR, Li Q, Jacobs JS, Gabani M, Mishra A, et al. Genetic Deletion of miR-204 Improves Glycemic Control Despite Obesity in Db/Db Mice. Biochem Biophys Res Commun (2020) 532(2):167–72. doi: 10.1016/j.bbrc.2020.08.077
129. Xu G, Chen J, Jing G, Grayson TB, Shalev A. miR-204 Targets PERK and Regulates UPR Signaling and Beta-Cell Apoptosis. Mol Endocrinol (2016) 30(8):917–24. doi: 10.1210/me.2016-1056
130. Jo S, Chen J, Xu G, Grayson TB, Thielen LA, Shalev A. miR-204 Controls Glucagon-Like Peptide 1 Receptor Expression and Agonist Function. Diabetes (2018) 67(2):256–64. doi: 10.2337/db17-0506
131. Grieco FA, Schiavo AA, Brozzi F, Juan-Mateu J, Bugliani M, Marchetti P, et al. The miRNAs miR-211-5p and miR-204-5p Modulate ER Stress in Human Beta Cells. J Mol Endocrinol (2019) 63(2):139–49. doi: 10.1530/JME-19-0066
132. Vinod M, Patankar JV, Sachdev V, Frank S, Graier WF, Kratky D, et al. MiR-206 is Expressed in Pancreatic Islets and Regulates Glucokinase Activity. Am J Physiol Endocrinol Metab (2016) 311(1):E175–85. doi: 10.1152/ajpendo.00510.2015
133. Joglekar MV, Wong WKM, Maynard CL, Umrani MR, Martin D, Loudovaris T, et al. Expression of miR-206 in Human Islets and Its Role in Glucokinase Regulation. Am J Physiol Endocrinol Metab (2018) 315(4):E634–7. doi: 10.1152/ajpendo.00152.2018
134. Shang J, Li J, Keller MP, Hohmeier HE, Wang Y, Feng Y, et al. Induction of miR-132 and miR-212 Expression by Glucagon-Like Peptide 1 (GLP-1) in Rodent and Human Pancreatic Beta-Cells. Mol Endocrinol (2015) 29(9):1243–53. doi: 10.1210/me.2014-1335
135. Backe MB, Novotny GW, Christensen DP, Grunnet LG, Mandrup-Poulsen T. Altering Beta-Cell Number Through Stable Alteration of miR-21 and miR-34a Expression. Islets (2014) 6(1):e27754.
136. Roggli E, Britan A, Gattesco S, Lin-Marq N, Abderrahmani A, Meda P, et al. Involvement of microRNAs in the Cytotoxic Effects Exerted by Proinflammatory Cytokines on Pancreatic Beta-Cells. Diabetes (2010) 59(4):978–86. doi: 10.2337/db09-0881
137. Li Y, Deng S, Peng J, Wang X, Essandoh K, Mu X, et al. MicroRNA-223 Is Essential for Maintaining Functional Beta-Cell Mass During Diabetes Through Inhibiting Both FOXO1 and SOX6 Pathways. J Biol Chem (2019) 294(27):10438–48. doi: 10.1074/jbc.RA119.007755
138. Zampetaki A, Kiechl S, Drozdov I, Willeit P, Mayr U, Prokopi M, et al. Plasma microRNA Profiling Reveals Loss of Endothelial miR-126 and Other microRNAs in Type 2 Diabetes. Circ Res (2010) 107(6):810–7. doi: 10.1161/CIRCRESAHA.110.226357
139. Nielsen LB, Wang C, Sorensen K, Bang-Berthelsen CH, Hansen L, Andersen ML, et al. Circulating Levels of microRNA From Children With Newly Diagnosed Type 1 Diabetes and Healthy Controls: Evidence That miR-25 Associates to Residual Beta-Cell Function and Glycaemic Control During Disease Progression. Exp Diabetes Res 2012 (2012) 2012:896362. doi: 10.1155/2012/896362
140. Erener S, Marwaha A, Tan R, Panagiotopoulos C, Kieffer TJ. Profiling of Circulating microRNAs in Children With Recent Onset of Type 1 Diabetes. JCI Insight (2017) 2(4):e89656. doi: 10.1172/jci.insight.89656
141. Tan W, Li Y, Lim SG, Tan TM. miR-106b-25/miR-17-92 Clusters: Polycistrons With Oncogenic Roles in Hepatocellular Carcinoma. World J Gastroenterol (2014) 20(20):5962–72. doi: 10.3748/wjg.v20.i20.5962
142. Wang B, Li W, Guo K, Xiao Y, Wang Y, Fan J. miR-181b Promotes Hepatic Stellate Cells Proliferation by Targeting P27 and Is Elevated in the Serum of Cirrhosis Patients. Biochem Biophys Res Commun (2012) 421(1):4–8. doi: 10.1016/j.bbrc.2012.03.025
143. Song Y, Jin D, Jiang X, Lv C, Zhu H. Overexpression of microRNA-26a Protects Against Deficient Beta-Cell Function via Targeting Phosphatase With Tensin Homology in Mouse Models of Type 2 Diabetes. Biochem Biophys Res Commun (2018) 495(1):1312–6. doi: 10.1016/j.bbrc.2017.11.170
144. Fu X, Jin L, Wang X, Luo A, Hu J, Zheng X, et al. MicroRNA-26a Targets Ten Eleven Translocation Enzymes and is Regulated During Pancreatic Cell Differentiation. Proc Natl Acad Sci USA (2013) 110(44):17892–7. doi: 10.1073/pnas.1317397110
145. Guo R, Yu Y, Zhang Y, Li Y, Chu X, Lu H, et al. Overexpression of miR-297b-5p Protects Against Stearic Acid-Induced Pancreatic Beta-Cell Apoptosis by Targeting LATS2. Am J Physiol Endocrinol Metab (2020) 318(3):E430–9. doi: 10.1152/ajpendo.00302.2019
146. Bagge A, Clausen TR, Larsen S, Ladefoged M, Rosenstierne MW, Larsen L, et al. MicroRNA-29a Is Up-Regulated in Beta-Cells by Glucose and Decreases Glucose-Stimulated Insulin Secretion. Biochem Biophys Res Commun (2012) 426(2):266–72. doi: 10.1016/j.bbrc.2012.08.082
147. Pandey AK, Verma G, Vig S, Srivastava S, Srivastava AK, Datta M. miR-29a Levels Are Elevated in the Db/Db Mice Liver and Its Overexpression Leads to Attenuation of Insulin Action on PEPCK Gene Expression in HepG2 Cells. Mol Cell Endocrinol (2011) 332(1-2):125–33. doi: 10.1016/j.mce.2010.10.004
148. Kim JW, You YH, Jung S, Suh-Kim H, Lee IK, Cho JH, et al. miRNA-30a-5p-Mediated Silencing of Beta2/NeuroD Expression Is an Important Initial Event of Glucotoxicity-Induced Beta Cell Dysfunction in Rodent Models. Diabetologia (2013) 56(4):847–55. doi: 10.1007/s00125-012-2812-x
149. Zhao X, Mohan R, Ozcan S, Tang X. MicroRNA-30d Induces Insulin Transcription Factor MafA and Insulin Production by Targeting Mitogen-Activated Protein 4 Kinase 4 (MAP4K4) in Pancreatic Beta-Cells. J Biol Chem (2012) 287(37):31155–64. doi: 10.1074/jbc.M112.362632
150. Salunkhe VA, Ofori JK, Gandasi NR, Salo SA, Hansson S, Andersson ME, et al. MiR-335 Overexpression Impairs Insulin Secretion Through Defective Priming of Insulin Vesicles. Physiol Rep (2017) 5(21). doi: 10.14814/phy2.13493
151. Jacovetti C, Abderrahmani A, Parnaud G, Jonas JC, Peyot ML, Cornu M, et al. MicroRNAs Contribute to Compensatory Beta Cell Expansion During Pregnancy and Obesity. J Clin Invest (2012) 122(10):3541–51. doi: 10.1172/JCI64151
152. Lu H, Hao L, Li S, Lin S, Lv L, Chen Y, et al. Elevated Circulating Stearic Acid Leads to a Major Lipotoxic Effect on Mouse Pancreatic Beta Cells in Hyperlipidaemia via a miR-34a-5p-Mediated PERK/p53-Dependent Pathway. Diabetologia (2016) 59(6):1247–57. doi: 10.1007/s00125-016-3900-0
153. El Ouaamari A, Baroukh N, Martens GA, Lebrun P, Pipeleers D, van Obberghen E. miR-375 Targets 3’-Phosphoinositide-Dependent Protein Kinase-1 and Regulates Glucose-Induced Biological Responses in Pancreatic Beta-Cells. Diabetes (2008) 57(10):2708–17. doi: 10.2337/db07-1614
154. Zhao H, Guan J, Lee HM, Sui Y, He L, Siu JJ, et al. Up-Regulated Pancreatic Tissue microRNA-375 Associates With Human Type 2 Diabetes Through Beta-Cell Deficit and Islet Amyloid Deposition. Pancreas (2010) 39(6):843–6. doi: 10.1097/MPA.0b013e3181d12613
155. He Y, Ding Y, Liang B, Lin J, Kim TK, Yu H, et al. A Systematic Study of Dysregulated MicroRNA in Type 2 Diabetes Mellitus. Int J Mol Sci (2017) 18(3). doi: 10.3390/ijms18030456
156. Poy MN, Hausser J, Trajkovski M, Braun M, Collins S, Rorsman P, et al. miR-375 Maintains Normal Pancreatic Alpha- and Beta-Cell Mass. Proc Natl Acad Sci USA (2009) 106(14):5813–8. doi: 10.1073/pnas.0810550106
157. Cheng X, Huang Y, Yang P, Bu L. miR-383 Ameliorates High Glucose-Induced Beta-Cells Apoptosis and Hyperglycemia in High-Fat Induced Diabetic Mice. Life Sci (2020) 263:118571. doi: 10.1016/j.lfs.2020.118571
158. Tu C, Wang L, Tao H, Gu L, Zhu S, Chen X. Expression of miR-409-5p in Gestational Diabetes Mellitus and Its Relationship With Insulin Resistance. Exp Ther Med (2020) 20(4):3324–9. doi: 10.3892/etm.2020.9049
159. Sun R, Xue W, Zhao J. Hsa_circ_0054633 Mediates Apoptosis and Insulin Secretion in Human Pancreatic Beta Cells Through miR-409-3p/Caspase-8 Axis. Diabetes Res Clin Pract (2021) p:108837.
160. Wang M. Mir433 Protects Pancreatic Beta Cell Growth in Highglucose Conditions. Mol Med Rep (2017) 16(3):2604–10. doi: 10.3892/mmr.2017.6925
161. Xu Y, Huang Y, Guo Y, Xiong Y, Zhu S, Xu L, et al. microRNA-690 Regulates Induced Pluripotent Stem Cells (iPSCs) Differentiation Into Insulin-Producing Cells by Targeting Sox9. Stem Cell Res Ther (2019) 10(1):59. doi: 10.1186/s13287-019-1154-8
162. Rodriguez-Comas J, Moreno-Asso A, Moreno-Vedia J, Martin M, Castano C, Marza-Florensa A, et al. Stress-Induced MicroRNA-708 Impairs Beta-Cell Function and Growth. Diabetes (2017) 66(12):3029–40. doi: 10.2337/db16-1569
163. Kornfeld JW, Baitzel C, Konner AC, Nicholls HT, Vogt MC, Herrmanns K, et al. Obesity-Induced Overexpression of miR-802 Impairs Glucose Metabolism Through Silencing of Hnf1b. Nature (2013) 494(7435):111–5. doi: 10.1038/nature11793
164. Zhang F, Ma D, Zhao W, Wang D, Liu T, Liu Y, et al. Obesity-Induced Overexpression of miR-802 Impairs Insulin Transcription and Secretion. Nat Commun (2020) 11(1):1822. doi: 10.1038/s41467-020-15529-w
165. Ramachandran D, Roy U, Garg S, Ghosh S, Pathak S, Kolthur-Seetharam U. Sirt1 and Mir-9 Expression Is Regulated During Glucose-Stimulated Insulin Secretion in Pancreatic Beta-Islets. FEBS J (2011) 278(7):1167–74. doi: 10.1111/j.1742-4658.2011.08042.x
166. Al-Muhtaresh HA, Al-Kafaji G. Evaluation of Two-Diabetes Related microRNAs Suitability as Earlier Blood Biomarkers for Detecting Prediabetes and Type 2 Diabetes Mellitus. J Clin Med (2018) 7(2). doi: 10.3390/jcm7020012
167. Hu D, Wang Y, Zhang H, Kong D. Identification of miR-9 as a Negative Factor of Insulin Secretion From Beta Cells. J Physiol Biochem (2018) 74(2):291–9. doi: 10.1007/s13105-018-0615-3
168. Latreille M, Herrmanns K, Renwick N, Tuschl T, Malecki MT, McCarthy MI, et al. miR-375 Gene Dosage in Pancreatic Beta-Cells: Implications for Regulation of Beta-Cell Mass and Biomarker Development. J Mol Med (Berlin Germany) (2015) 93(10):1159–69. doi: 10.1007/s00109-015-1296-9
169. Nathan G, Kredo-Russo S, Geiger T, Lenz A, Kaspi H, Hornstein E, et al. MiR-375 Promotes Redifferentiation of Adult Human Beta Cells Expanded In Vitro. PLoS One (2015) 10(4):e0122108. doi: 10.1371/journal.pone.0122108
170. Salunkhe VA, Esguerra JL, Ofori JK, Mollet IG, Braun M, Stoffel M, et al. Modulation of microRNA-375 Expression Alters Voltage-Gated Na(+) Channel Properties and Exocytosis in Insulin-Secreting Cells. Acta Physiol (Oxf) (2015) 213(4):882–92. doi: 10.1111/apha.12460
171. Bhasin KK, van Nas A, Martin LJ, Davis RC, Devaskar SU, Lusis AJ. Maternal Low-Protein Diet or Hypercholesterolemia Reduces Circulating Essential Amino Acids and Leads to Intrauterine Growth Restriction. Diabetes (2009) 58(3):559–66. doi: 10.2337/db07-1530
172. Alejandro EU, Gregg B, Wallen T, Kumusoglu D, Meister D, Chen A, et al. Maternal Diet-Induced microRNAs and mTOR Underlie β Cell Dysfunction in Offspring. J Clin Invest (2014) 124(10):4395–410. doi: 10.1172/JCI74237
173. Dumortier O, Hinault C, Gautier N, Patouraux S, Casamento V, Van Obberghen E. Maternal Protein Restriction Leads to Pancreatic Failure in Offspring: Role of Misexpressed microRNA-375. Diabetes (2014) 63(10):3416–27. doi: 10.2337/db13-1431
174. Su Y, Jiang X, Li Y, Li F, Cheng Y, Peng Y, et al. Maternal Low Protein Isocaloric Diet Suppresses Pancreatic β-Cell Proliferation in Mouse Offspring via miR-15b. Endocrinology (2016) 157(12):4782–93. doi: 10.1210/en.2016-1167
175. Alejandro EU, Jo S, Akhaphong B, Llacer PR, Gianchandani M, Gregg B, et al. Maternal Low-Protein Diet on the Last Week of Pregnancy Contributes to Insulin Resistance and β-Cell Dysfunction in the Mouse Offspring. Am J Physiol Regul Integr Comp Physiol (2020) 319(4):R485–96. doi: 10.1152/ajpregu.00284.2019
176. de Siqueira KC, de Lima FM, Lma FS, Taki MS, da Cunha CF, de Lima Reis SR, et al. miR-124a Expression Contributes to the Monophasic Pattern of Insulin Secretion in Islets From Pregnant Rats Submitted to a Low-Protein Diet. Eur J Nutr (2018) 57(4):1471–83. doi: 10.1007/s00394-017-1425-z
177. Stolovich-Rain M, Enk J, Vikesa J, Nielsen FC, Saada A, Glaser B, et al. Weaning Triggers a Maturation Step of Pancreatic β Cells. Dev Cell (2015) 32(5):535–45. doi: 10.1016/j.devcel.2015.01.002
178. Roux J, Gonzàlez-Porta M, Robinson-Rechavi M. Comparative Analysis of Human and Mouse Expression Data Illuminates Tissue-Specific Evolutionary Patterns of miRNAs. Nucleic Acids Res (2012) 40(13):5890–900. doi: 10.1093/nar/gks279
179. Benner C, van der Meulen T, Cacéres E, Tigyi K, Donaldson CJ, Huising MO, et al. The Transcriptional Landscape of Mouse Beta Cells Compared to Human Beta Cells Reveals Notable Species Differences in Long Non-Coding RNA and Protein-Coding Gene Expression. BMC Genomics (2014) 15:620. doi: 10.1186/1471-2164-15-620
180. Ilegems E, Berggren PO. The Eye as a Transplantation Site to Monitor Pancreatic Islet Cell Plasticity. Front Endocrinol (Lausanne) (2021) 12:652853. doi: 10.3389/fendo.2021.652853
181. Siddika T, Heinemann IU. Bringing MicroRNAs to Light: Methods for MicroRNA Quantification and Visualization in Live Cells. Front Bioeng Biotechnol (2020) 8:619583. doi: 10.3389/fbioe.2020.619583
182. Gregorova J, Vychytilova-Faltejskova P, Sevcikova S. Epigenetic Regulation of MicroRNA Clusters and Families During Tumor Development. Cancers (Basel) (2021) 13(6). doi: 10.3390/cancers13061333
183. Krol J, Busskamp V, Markiewicz I, Stadler MB, Ribi S, Richter J, et al. Characterizing Light-Regulated Retinal microRNAs Reveals Rapid Turnover as a Common Property of Neuronal microRNAs. Cell (2010) 141(4):618–31. doi: 10.1016/j.cell.2010.03.039
184. Yang L, Zhu Y, Kong D, Gong J, Yu W, Liang Y, et al. EGF Suppresses the Expression of miR-124a in Pancreatic β Cell Lines via ETS2 Activation Through the MEK and PI3K Signaling Pathways. Int J Biol Sci (2019) 15(12):2561–75. doi: 10.7150/ijbs.34985
185. Maachi H, Fergusson G, Ethier M, Brill GN, Katz LS, Honig LB, et al. HB-EGF Signaling Is Required for Glucose-Induced Pancreatic Beta-Cell Proliferation in Rats. Diabetes (2020) 69(3):369–80. doi: 10.2337/db19-0643
186. Hakonen E, Ustinov J, Mathijs I, Palgi J, Bouwens L, Miettinen PJ, et al. Epidermal Growth Factor (EGF)-Receptor Signalling Is Needed for Murine Beta Cell Mass Expansion in Response to High-Fat Diet and Pregnancy But Not After Pancreatic Duct Ligation. Diabetologia (2011) 54(7):1735–43. doi: 10.1007/s00125-011-2153-1
187. Zhang H, Liu R, Deng T, Wang X, Lang H, Qu Y, et al. The microRNA-124-Iglur2/3 Pathway Regulates Glucagon Release From Alpha Cells. Oncotarget (2016) 7(17):24734–43. doi: 10.18632/oncotarget.8270
188. Taheri F, Ebrahimi SO, Shareef S, Reiisi S. Regulatory and Immunomodulatory Role of miR-34a in T Cell Immunity. Life Sci (2020) 262:118209. doi: 10.1016/j.lfs.2020.118209
189. Raver-Shapira N, Marciano E, Meiri E, Spector Y, Rosenfeld N, Moskovits N, et al. Transcriptional Activation of miR-34a Contributes to P53-Mediated Apoptosis. Mol Cell (2007) 26(5):731–43. doi: 10.1016/j.molcel.2007.05.017
190. Kuna RS, Girada SB, Asalla S, Vallentyne J, Maddika S, Patterson JT, et al. Glucagon-Like Peptide-1 Receptor-Mediated Endosomal cAMP Generation Promotes Glucose-Stimulated Insulin Secretion in Pancreatic β-Cells. Am J Physiol Endocrinol Metab (2013) 305(2):E161–70. doi: 10.1152/ajpendo.00551.2012
191. Ammälä C, Ashcroft FM, Rorsman P. Calcium-Independent Potentiation of Insulin Release by Cyclic AMP in Single Beta-Cells. Nature (1993) 363(6427):356–8. doi: 10.1038/363356a0
192. Malm HA, Mollet IG, Berggreen C, Orho-Melander M, Esguerra JL, Göransson O, et al. Transcriptional Regulation of the miR-212/miR-132 Cluster in Insulin-Secreting β-Cells by cAMP-Regulated Transcriptional Co-Activator 1 and Salt-Inducible Kinases. Mol Cell Endocrinol (2016) 424:23–33. doi: 10.1016/j.mce.2016.01.010
193. Mollet IG, Malm HA, Wendt A, Orho-Melander M, Eliasson L. Integrator of Stress Responses Calmodulin Binding Transcription Activator 1 (Camta1) Regulates miR-212/miR-132 Expression and Insulin Secretion. J Biol Chem (2016) 291(35):18440–52. doi: 10.1074/jbc.M116.716860
194. Dyachok O, Isakov Y, Sågetorp J, Tengholm A. Oscillations of Cyclic AMP in Hormone-Stimulated Insulin-Secreting Beta-Cells. Nature (2006) 439(7074):349–52. doi: 10.1038/nature04410
195. Keller DM, Clark EA, Goodman RH. Regulation of microRNA-375 by cAMP in Pancreatic Beta-Cells. Mol Endocrinol (2012) 26(6):989–99. doi: 10.1210/me.2011-1205
196. Guo C, Sun YQ, Li Q, Zhang JC. MiR-7, miR-9 and miR-375 Contribute to Effect of Exendin-4 on Pancreatic β-Cells in High-Fat-Diet-Fed Mice. Clin Invest Med (2018) 41(1):E16–24. doi: 10.25011/cim.v41i1.29459
197. Rafiq I, da Silva Xavier G, Hooper S, Rutter GA. Glucose-Stimulated Preproinsulin Gene Expression and Nuclear Trans-Location of Pancreatic Duodenum Homeobox-1 Require Activation of Phosphatidylinositol 3-Kinase But Not P38 MAPK/Sapk2. J Biol Chem (2000) 275(21):15977–84. doi: 10.1074/jbc.275.21.15977
198. Kim H, Kulkarni RN. Epigenetics in β-Cell Adaptation and Type 2 Diabetes. Curr Opin Pharmacol (2020) 55:125–31. doi: 10.1016/j.coph.2020.10.008
199. Malmgren S, Spégel P, Danielsson AP, Nagorny CL, Andersson LE, Nitert MD, et al. Coordinate Changes in Histone Modifications, mRNA Levels, and Metabolite Profiles in Clonal INS-1 832/13 β-Cells Accompany Functional Adaptations to Lipotoxicity. J Biol Chem (2013) 288(17):11973–87. doi: 10.1074/jbc.M112.422527
200. Hall E, Jönsson J, Ofori JK, Volkov P, Perfilyev A, Dekker Nitert M, et al. Glucolipotoxicity Alters Insulin Secretion via Epigenetic Changes in Human Islets. Diabetes (2019) 68(10):1965–74. doi: 10.2337/db18-0900
201. Ling C, Rönn T. Epigenetics in Human Obesity and Type 2 Diabetes. Cell Metab (2019) 29(5):1028–44. doi: 10.1016/j.cmet.2019.03.009
202. Hall E, Volkov P, Dayeh T, Esguerra JL, Salö S, Eliasson L, et al. Sex Differences in the Genome-Wide DNA Methylation Pattern and Impact on Gene Expression, microRNA Levels and Insulin Secretion in Human Pancreatic Islets. Genome Biol (2014) 15(12):522. doi: 10.1186/s13059-014-0522-z
203. Nojima T, Gomes T, Grosso ARF, Kimura H, Dye MJ, Dhir S, et al. Mammalian NET-Seq Reveals Genome-Wide Nascent Transcription Coupled to RNA Processing. Cell (2015) 161(3):526–40. doi: 10.1016/j.cell.2015.03.027
204. Tome JM, Tippens ND, Lis JT. Single-Molecule Nascent RNA Sequencing Identifies Regulatory Domain Architecture at Promoters and Enhancers. Nat Genet (2018) 50(11):1533–41. doi: 10.1038/s41588-018-0234-5
205. Hua X, Chen L, Wang J, Li J, Wingender E. Identifying Cell-Specific microRNA Transcriptional Start Sites. Bioinformatics (2016) 32(16):2403–10. doi: 10.1093/bioinformatics/btw171
206. Liu Q, Wang J, Zhao Y, Li CI, Stengel KR, Acharya P, et al. Identification of Active miRNA Promoters From Nuclear Run-on RNA Sequencing. Nucleic Acids Res (2017) 45(13):e121. doi: 10.1093/nar/gkx318
207. Cha M, Zheng H, Talukder A, Barham C, Li X, Hu H. A Two-Stream Convolutional Neural Network for microRNA Transcription Start Site Feature Integration and Identification. Sci Rep (2021) 11(1):5625. doi: 10.1038/s41598-021-85173-x
208. Core LJ, Waterfall JJ, Lis JT. Nascent RNA Sequencing Reveals Widespread Pausing and Divergent Initiation at Human Promoters. Science (2008) 322(5909):1845–8. doi: 10.1126/science.1162228
209. Mahat DB, Kwak H, Booth GT, Jonkers IH, Danko CG, Patel RK, et al. Base-Pair-Resolution Genome-Wide Mapping of Active RNA Polymerases Using Precision Nuclear Run-on (PRO-Seq). Nat Protoc (2016) 11(8):1455–76. doi: 10.1038/nprot.2016.086
210. Wissink EM, Vihervaara A, Tippens ND, Lis JT. Nascent RNA Analyses: Tracking Transcription and Its Regulation. Nat Rev Genet (2019) 20(12):705–23. doi: 10.1038/s41576-019-0159-6
211. Nazarov PV, Kreis S. Integrative Approaches for Analysis of mRNA and microRNA High-Throughput Data. Comput Struct Biotechnol J (2021) 19:1154–62. doi: 10.1016/j.csbj.2021.01.029
212. Chang L, Zhou G, Soufan O, Xia J. Mirnet 2.0: Network-Based Visual Analytics for miRNA Functional Analysis and Systems Biology. Nucleic Acids Res (2020) 48(W1):W244–51. doi: 10.1093/nar/gkaa467
213. Tong Z, Cui Q, Wang J, Zhou Y. TransmiR V2.0: An Updated Transcription factor-microRNA Regulation Database. Nucleic Acids Res (2019) 47(D1):D253–8. doi: 10.1093/nar/gky1023
214. Wong WKM, Joglekar MV, Saini V, Jiang G, Dong CX, Chaitarvornkit A, et al. Machine Learning Workflows Identify a microRNA Signature of Insulin Transcription in Human Tissues. iScience (2021) 24(4):102379. doi: 10.1016/j.isci.2021.102379
215. Schrimpe-Rutledge AC, Fontès G, Gritsenko MA, Norbeck AD, Anderson DJ, Waters KM, et al. Discovery of Novel Glucose-Regulated Proteins in Isolated Human Pancreatic Islets Using LC-MS/MS-Based Proteomics. J Proteome Res (2012) 11(7):3520–32. doi: 10.1021/pr3002996
216. Noren Hooten N, Martin-Montalvo A, Dluzen DF, Zhang Y, Bernier M, Zonderman AB, et al. Metformin-Mediated Increase in DICER1 Regulates microRNA Expression and Cellular Senescence. Aging Cell (2016) 15(3):572–81. doi: 10.1111/acel.12469
217. Kone M, Pullen TJ, Sun G, Ibberson M, Martinez-Sanchez A, Sayers S, et al. LKB1 and AMPK Differentially Regulate Pancreatic Beta-Cell Identity. FASEB J (2014) 28(11):4972–85. doi: 10.1096/fj.14-257667
218. Treiber T, Treiber N, Meister G. Regulation of microRNA Biogenesis and Its Crosstalk With Other Cellular Pathways. Nat Rev Mol Cell Biol (2019) 20(1):5–20. doi: 10.1038/s41580-018-0059-1
219. Michlewski G, Cáceres JF. Post-Transcriptional Control of miRNA Biogenesis. RNA (2019) 25(1):1–16. doi: 10.1261/rna.068692.118
220. Newman MA, Thomson JM, Hammond SM. Lin-28 Interaction With the Let-7 Precursor Loop Mediates Regulated microRNA Processing. RNA (2008) 14(8):1539–49. doi: 10.1261/rna.1155108
221. Heo I, Joo C, Kim YK, Ha M, Yoon MJ, Cho J, et al. TUT4 in Concert With Lin28 Suppresses microRNA Biogenesis Through pre-microRNA Uridylation. Cell (2009) 138(4):696–708. doi: 10.1016/j.cell.2009.08.002
222. Michlewski G, Guil S, Cáceres JF. Stimulation of pri-miR-18a Processing by hnRNP A1. Adv Exp Med Biol (2010) 700:28–35. doi: 10.1007/978-1-4419-7823-3_3
223. Michlewski G, Cáceres JF. Antagonistic Role of hnRNP A1 and KSRP in the Regulation of Let-7a Biogenesis. Nat Struct Mol Biol (2010) 17(8):1011–8. doi: 10.1038/nsmb.1874
224. Kooshapur H, Choudhury NR, Simon B, Mühlbauer M, Jussupow A, Fernandez N, et al. Structural Basis for Terminal Loop Recognition and Stimulation of pri-miRNA-18a Processing by hnRNP A1. Nat Commun (2018) 9(1):2479. doi: 10.1038/s41467-018-04871-9
225. Kumar S, Downie Ruiz Velasco A, Michlewski G. Oleic Acid Induces MiR-7 Processing Through Remodeling of Pri-MiR-7/Protein Complex. J Mol Biol (2017) 429(11):1638–49. doi: 10.1016/j.jmb.2017.05.001
226. Sung Y, Jeong J, Kang RJ, Choi M, Park S, Kwon W, et al. Lin28a Expression Protects Against Streptozotocin-Induced β-Cell Destruction and Prevents Diabetes in Mice. Cell Biochem Funct (2019) 37(3):139–47. doi: 10.1002/cbf.3376
227. Rüegger S, Großhans H. MicroRNA Turnover: When, How, and Why. Trends Biochem Sci (2012) 37(10):436–46. doi: 10.1016/j.tibs.2012.07.002
228. Reichholf B, Herzog VA, Fasching N, Manzenreither RA, Sowemimo I, Ameres SL. Time-Resolved Small RNA Sequencing Unravels the Molecular Principles of MicroRNA Homeostasis. Mol Cell (2019) 75(4):756–68.e7. doi: 10.1016/j.molcel.2019.06.018
229. Guo Y, Liu J, Elfenbein SJ, Ma Y, Zhong M, Qiu C, et al. Characterization of the Mammalian miRNA Turnover Landscape. Nucleic Acids Res (2015) 43(4):2326–41. doi: 10.1093/nar/gkv057
230. Kingston ER, Bartel DP. Global Analyses of the Dynamics of Mammalian microRNA Metabolism. Genome Res (2019) 29(11):1777–90. doi: 10.1101/gr.251421.119
231. Ameres SL, Horwich MD, Hung JH, Xu J, Ghildiyal M, Weng Z, et al. Target RNA-Directed Trimming and Tailing of Small Silencing RNAs. Science (2010) 328(5985):1534–9. doi: 10.1126/science.1187058
232. de la Mata M, Gaidatzis D, Vitanescu M, Stadler MB, Wentzel C, Scheiffele P, et al. Potent Degradation of Neuronal miRNAs Induced by Highly Complementary Targets. EMBO Rep (2015) 16(4):500–11. doi: 10.15252/embr.201540078
233. Han J, LaVigne CA, Jones BT, Zhang H, Gillett F, Mendell JT. A Ubiquitin Ligase Mediates Target-Directed microRNA Decay Independently of Tailing and Trimming. Science (2020) 370(6523). doi: 10.3390/cancers13061333
234. Shi CY, Kingston ER, Kleaveland B, Lin DH, Stubna MW, Bartel DP. The ZSWIM8 Ubiquitin Ligase Mediates Target-Directed microRNA Degradation. Science (2020) 370(6523). doi: 10.1126/science.abc9359
235. Chatterjee S, Grosshans H. Active Turnover Modulates Mature microRNA Activity in Caenorhabditis Elegans. Nature (2009) 461(7263):546–9. doi: 10.1038/nature08349
236. Zhang H, Lu Y, Chen E, Li X, Lv B, Vikis HG, et al. XRN2 Promotes EMT and Metastasis Through Regulating Maturation of miR-10a. Oncogene (2017) 36(27):3925–33. doi: 10.1038/onc.2017.39
237. Chang HM, Triboulet R, Thornton JE, Gregory RI. A Role for the Perlman Syndrome Exonuclease Dis3l2 in the Lin28-Let-7 Pathway. Nature (2013) 497(7448):244–8. doi: 10.1038/nature12119
238. Ghiasi SM, Krogh N, Tyrberg B, Mandrup-Poulsen T. The No-Go and Nonsense-Mediated RNA Decay Pathways Are Regulated by Inflammatory Cytokines in Insulin-Producing Cells and Human Islets and Determine β-Cell Insulin Biosynthesis and Survival. Diabetes (2018) 67(10):2019–37. doi: 10.2337/db18-0073
239. Bitetti A, Mallory AC, Golini E, Carrieri C, Carreño Gutiérrez H, Perlas E, et al. MicroRNA Degradation by a Conserved Target RNA Regulates Animal Behavior. Nat Struct Mol Biol (2018) 25(3):244–51. doi: 10.1038/s41594-018-0032-x
240. Kleaveland B, Shi CY, Stefano J, Bartel DP. A Network of Noncoding Regulatory RNAs Acts in the Mammalian Brain. Cell (2018) 174(2):350–62.e17. doi: 10.1016/j.cell.2018.05.022
241. Stoll L, Sobel J, Rodriguez-Trejo A, Guay C, Lee K, Venø MT, et al. Circular RNAs as Novel Regulators of β-Cell Functions in Normal and Disease Conditions. Mol Metab (2018) 9:69–83. doi: 10.1016/j.molmet.2018.01.010
242. Stefan-Lifshitz M, Karakose E, Cui L, Ettela A, Yi Z, Zhang W, et al. Epigenetic Modulation of β Cells by Interferon-α via PNPT1/mir-26a/TET2 Triggers Autoimmune Diabetes. JCI Insight (2019) 4(5). doi: 10.1172/jci.insight.126663
243. Arntfield ME, van der Kooy D. β-Cell Evolution: How the Pancreas Borrowed From the Brain: The Shared Toolbox of Genes Expressed by Neural and Pancreatic Endocrine Cells May Reflect Their Evolutionary Relationship. Bioessays (2011) 33(8):582–7. doi: 10.1002/bies.201100015
244. Schwalb B, Michel M, Zacher B, Frühauf K, Demel C, Tresch A, et al. TT-Seq Maps the Human Transient Transcriptome. Science (2016) 352(6290):1225–8. doi: 10.1126/science.aad9841
245. Matsushima W, Herzog VA, Neumann T, Gapp K, Zuber J, Ameres SL, et al. Sequencing Cell-Type-Specific Transcriptomes With SLAM-ITseq. Nat Protoc (2019) 14(8):2261–78. doi: 10.1038/s41596-019-0179-x
246. Morin RD, O'Connor MD, Griffith M, Kuchenbauer F, Delaney A, Prabhu AL, et al. Application of Massively Parallel Sequencing to microRNA Profiling and Discovery in Human Embryonic Stem Cells. Genome Res (2008) 18(4):610–21. doi: 10.1101/gr.7179508
247. Wilson RC, Tambe A, Kidwell MA, Noland CL, Schneider CP, Doudna JA. Dicer-TRBP Complex Formation Ensures Accurate Mammalian microRNA Biogenesis. Mol Cell (2015) 57(3):397–407. doi: 10.1016/j.molcel.2014.11.030
248. Tan GC, Chan E, Molnar A, Sarkar R, Alexieva D, Isa IM, et al. 5’ isomiR Variation Is of Functional and Evolutionary Importance. Nucleic Acids Res (2014) 42(14):9424–35. doi: 10.1093/nar/gku656
249. Nishikura K. A-To-I Editing of Coding and Non-Coding RNAs by ADARs. Nat Rev Mol Cell Biol (2016) 17(2):83–96. doi: 10.1038/nrm.2015.4
250. Llorens F, Bañez-Coronel M, Pantano L, del Río JA, Ferrer I, Estivill X, et al. A Highly Expressed miR-101 isomiR Is a Functional Silencing Small RNA. BMC Genomics (2013) 14:104. doi: 10.1186/1471-2164-14-104
251. Yamane D, Selitsky SR, Shimakami T, Li Y, Zhou M, Honda M, et al. Differential Hepatitis C Virus RNA Target Site Selection and Host Factor Activities of Naturally Occurring miR-122 3′ Variants. Nucleic Acids Res (2017) 45(8):4743–55. doi: 10.1093/nar/gkw1332
252. Kawahara Y, Zinshteyn B, Sethupathy P, Iizasa H, Hatzigeorgiou AG, Nishikura K. Redirection of Silencing Targets by Adenosine-to-Inosine Editing of miRNAs. Science (2007) 315(5815):1137–40. doi: 10.1126/science.1138050
253. Kawahara Y, Megraw M, Kreider E, Iizasa H, Valente L, Hatzigeorgiou AG, et al. Frequency and Fate of microRNA Editing in Human Brain. Nucleic Acids Res (2008) 36(16):5270–80. doi: 10.1093/nar/gkn479
254. Shoshan E, Mobley AK, Braeuer RR, Kamiya T, Huang L, Vasquez ME, et al. Reduced Adenosine-to-Inosine miR-455-5p Editing Promotes Melanoma Growth and Metastasis. Nat Cell Biol (2015) 17(3):311–21. doi: 10.1038/ncb3110
255. Xu X, Wang Y, Mojumdar K, Zhou Z, Jeong KJ, Mangala LS, et al. A-To-I-Edited miRNA-379-5p Inhibits Cancer Cell Proliferation Through CD97-Induced Apoptosis. J Clin Invest (2019) 129(12):5343–56. doi: 10.1172/JCI123396
256. Wyman SK, Knouf EC, Parkin RK, Fritz BR, Lin DW, Dennis LM, et al. Post-Transcriptional Generation of miRNA Variants by Multiple Nucleotidyl Transferases Contributes to miRNA Transcriptome Complexity. Genome Res (2011) 21(9):1450–61. doi: 10.1101/gr.118059.110
257. Boele J, Persson H, Shin JW, Ishizu Y, Newie IS, Søkilde R, et al. PAPD5-Mediated 3’ Adenylation and Subsequent Degradation of miR-21 Is Disrupted in Proliferative Disease. Proc Natl Acad Sci USA (2014) 111(31):11467–72. doi: 10.1073/pnas.1317751111
258. Katoh T, Sakaguchi Y, Miyauchi K, Suzuki T, Kashiwabara S, Baba T. Selective Stabilization of Mammalian microRNAs by 3’ Adenylation Mediated by the Cytoplasmic Poly(A) Polymerase GLD-2. Genes Dev (2009) 23(4):433–8. doi: 10.1101/gad.1761509
259. Jones MR, Quinton LJ, Blahna MT, Neilson JR, Fu S, Ivanov AR, et al. Zcchc11-Dependent Uridylation of microRNA Directs Cytokine Expression. Nat Cell Biol (2009) 11(9):1157–63. doi: 10.1038/ncb1931
260. Yang A, Bofill-De Ros X, Shao TJ, Jiang M, Li K, Villanueva P, et al. 3’ Uridylation Confers miRNAs With Non-Canonical Target Repertoires. Mol Cell (2019) 75(3):511–22.e4.
261. Kim H, Kim J, Yu S, Lee YY, Park J, Choi RJ, et al. A Mechanism for microRNA Arm Switching Regulated by Uridylation. Mol Cell (2020) 78(6):1224–36.e5. doi: 10.1016/j.molcel.2020.04.030
262. Yang L, Huang P, Li F, Zhao L, Zhang Y, Li S, et al. C-Jun Amino-Terminal Kinase-1 Mediates Glucose-Responsive Upregulation of the RNA Editing Enzyme ADAR2 in Pancreatic Beta-Cells. PLoS One (2012) 7(11):e48611. doi: 10.1371/journal.pone.0048611
263. Yang L, Zhao L, Gan Z, He Z, Xu J, Gao X, et al. Deficiency in RNA Editing Enzyme ADAR2 Impairs Regulated Exocytosis. FASEB J (2010) 24(10):3720–32. doi: 10.1096/fj.09-152363
264. Gan Z, Zhao L, Yang L, Huang P, Zhao F, Li W, et al. RNA Editing by ADAR2 Is Metabolically Regulated in Pancreatic Islets and Beta-Cells. J Biol Chem (2006) 281(44):33386–94. doi: 10.1074/jbc.M604484200
265. Salzman DW, Nakamura K, Nallur S, Dookwah MT, Metheetrairut C, Slack FJ, et al. miR-34 Activity Is Modulated Through 5’-End Phosphorylation in Response to DNA Damage. Nat Commun (2016) 7:10954. doi: 10.1038/ncomms10954
266. Lin X, Guan H, Huang Z, Liu J, Li H, Wei G, et al. Downregulation of Bcl-2 Expression by miR-34a Mediates Palmitate-Induced Min6 Cells Apoptosis. J Diabetes Res (2014) 2014:258695. doi: 10.1155/2014/258695
267. Uhlemeyer C, Müller N, Grieß K, Wessel C, Schlegel C, Kuboth J, et al. ATM and P53 Differentially Regulate Pancreatic Beta Cell Survival in Ins1E Cells. PLoS One (2020) 15(8):e0237669.
268. Schirle NT, MacRae IJ. The Crystal Structure of Human Argonaute2. Science (2012) 336(6084):1037–40. doi: 10.1126/science.1221551
269. Diederichs S, Haber DA. Dual Role for Argonautes in microRNA Processing and Posttranscriptional Regulation of microRNA Expression. Cell (2007) 131(6):1097–108. doi: 10.1016/j.cell.2007.10.032
270. Sahin U, Lapaquette P, Andrieux A, Faure G, Dejean A. Sumoylation of Human Argonaute 2 at Lysine-402 Regulates Its Stability. PLoS One (2014) 9(7):e102957. doi: 10.1371/journal.pone.0102957
271. Bronevetsky Y, Villarino AV, Eisley CJ, Barbeau R, Barczak AJ, Heinz GA, et al. T Cell Activation Induces Proteasomal Degradation of Argonaute and Rapid Remodeling of the microRNA Repertoire. J Exp Med (2013) 210(2):417–32. doi: 10.1084/jem.20111717
272. Qi HH, Ongusaha PP, Myllyharju J, Cheng D, Pakkanen O, Shi Y, et al. Prolyl 4-Hydroxylation Regulates Argonaute 2 Stability. Nature (2008) 455(7211):421–4. doi: 10.1038/nature07186
273. Leung A, Todorova T, Ando Y, Chang P. Poly(ADP-Ribose) Regulates Post-Transcriptional Gene Regulation in the Cytoplasm. RNA Biol (2012) 9(5):542–8. doi: 10.4161/rna.19899
274. Bottini S, Hamouda-Tekaya N, Mategot R, Zaragosi LE, Audebert S, Pisano S, et al. Post-Transcriptional Gene Silencing Mediated by microRNAs Is Controlled by Nucleoplasmic Sfpq. Nat Commun (2017) 8(1):1189. doi: 10.1038/s41467-017-01126-x
275. Kedde M, Strasser MJ, Boldajipour B, Oude Vrielink JA, Slanchev K, le Sage C, et al. RNA-Binding Protein Dnd1 Inhibits microRNA Access to Target mRNA. Cell (2007) 131(7):1273–86. doi: 10.1016/j.cell.2007.11.034
276. Kedde M, van Kouwenhove M, Zwart W, Oude Vrielink JA, Elkon R. A Pumilio-Induced RNA Structure Switch in P27-3’ UTR Controls miR-221 and miR-222 Accessibility. Nat Cell Biol (2010) 12(10):1014–20. doi: 10.1038/ncb2105
277. Min KW, Jo MH, Shin S, Davila S, Zealy RW, Kang SI, et al. AUF1 Facilitates microRNA-Mediated Gene Silencing. Nucleic Acids Res (2017) 45(10):6064–73. doi: 10.1093/nar/gkx149
278. Kim HH, Kuwano Y, Srikantan S, Lee EK, Martindale JL, Gorospe M. HuR Recruits Let-7/RISC to Repress C-Myc Expression. Genes Dev (2009) 23(15):1743–8. doi: 10.1101/gad.1812509
279. Mukherjee N, Corcoran DL, Nusbaum JD, Reid DW, Georgiev S, Hafner M, et al. Integrative Regulatory Mapping Indicates That the RNA-Binding Protein HuR Couples pre-mRNA Processing and mRNA Stability. Mol Cell (2011) 43(3):327–39. doi: 10.1016/j.molcel.2011.06.007
280. Lee EK, Kim W, Tominaga K, Martindale JL, Yang X, Subaran SS, et al. RNA-Binding Protein HuD Controls Insulin Translation. Mol Cell (2012) 45(6):826–35. doi: 10.1016/j.molcel.2012.01.016
281. Hong Y, Tak H, Kim C, Kang H, Ji E, Ahn S, et al. RNA Binding Protein HuD Contributes to β-Cell Dysfunction by Impairing Mitochondria Dynamics. Cell Death Differ (2020) 27(5):1633–43. doi: 10.1038/s41418-019-0447-x
282. Kim C, Kim W, Lee H, Ji E, Choe YJ, Martindale JL, et al. The RNA-Binding Protein HuD Regulates Autophagosome Formation in Pancreatic β Cells by Promoting Autophagy-Related Gene 5 Expression. J Biol Chem (2014) 289(1):112–21. doi: 10.1074/jbc.M113.474700
283. Thomson DW, Dinger ME. Endogenous microRNA Sponges: Evidence and Controversy. Nat Rev Genet (2016) 17(5):272–83. doi: 10.1038/nrg.2016.20
284. Patop IL, Wüst S, Kadener S. Past, Present, and Future of circRNAs. EMBO J (2019) 38(16):e100836. doi: 10.15252/embj.2018100836
285. Wu L, Xiong L, Li J, Peng Z, Zhang L, Shi P, et al. Circ-Tulp4 Promotes β-Cell Adaptation to Lipotoxicity by Regulating Soat1 Expression. J Mol Endocrinol (2020) 65(4):149–61. doi: 10.1530/JME-20-0079
286. Kong X, Liu CX, Wang GD, Yang H, Yao XM, Hua Q, et al. LncRNA LEGLTBC Functions as a ceRNA to Antagonize the Effects of miR-34a on the Downregulation of SIRT1 in Glucolipotoxicity-Induced INS-1 Beta Cell Oxidative Stress and Apoptosis. Oxid Med Cell Longev 2019 (2019) p:4010764. doi: 10.1155/2019/4010764
287. Cheng Y, Hu Q, Zhou J. Silencing of lncRNA PVT1 Ameliorates Streptozotocin-Induced Pancreatic β Cell Injury and Enhances Insulin Secretory Capacity by Regulating miR-181a-5p. Can J Physiol Pharmacol (2021) 99(3):303–12. doi: 10.1139/cjpp-2020-0268
288. Brozzi F, Regazzi R. Circular RNAs as Novel Regulators of β-Cell Functions Under Physiological and Pathological Conditions. Int J Mol Sci (2021) 22(4). doi: 10.3390/ijms22041503
289. Guay C, Jacovetti C, Bayazit MB, Brozzi F, Rodriguez-Trejo A, Wu K, et al. Roles of Noncoding RNAs in Islet Biology. Compr Physiol (2020) 10(3):893–932. doi: 10.1002/cphy.c190032
290. Khudayberdiev SA, Zampa F, Rajman M, Schratt G. A Comprehensive Characterization of the Nuclear microRNA Repertoire of Post-Mitotic Neurons. Front Mol Neurosci (2013) 6:43. doi: 10.3389/fnmol.2013.00043
291. Younger ST, Corey DR. Transcriptional Gene Silencing in Mammalian Cells by miRNA Mimics That Target Gene Promoters. Nucleic Acids Res (2011) 39(13):5682–91. doi: 10.1093/nar/gkr155
292. Kim DH, Saetrom P, Snøve O, Rossi JJ. MicroRNA-Directed Transcriptional Gene Silencing in Mammalian Cells. Proc Natl Acad Sci USA (2008) 105(42):16230–5. doi: 10.1073/pnas.0808830105
293. Fasanaro P, Greco S, Lorenzi M, Pescatori M, Brioschi M, Kulshreshtha R, et al. An Integrated Approach for Experimental Target Identification of Hypoxia-Induced miR-210. J Biol Chem (2009) 284(50):35134–43. doi: 10.1074/jbc.M109.052779
294. Leucci E, Patella F, Waage J, Holmstrøm K, Lindow M, Porse B, et al. microRNA-9 Targets the Long Non-Coding RNA MALAT1 for Degradation in the Nucleus. Sci Rep (2013) 3:2535. doi: 10.1038/srep02535
295. Wang D, Sun X, Wei Y, Liang H, Yuan M, Jin F, et al. Nuclear miR-122 Directly Regulates the Biogenesis of Cell Survival oncomiR miR-21 at the Posttranscriptional Level. Nucleic Acids Res (2018) 46(4):2012–29. doi: 10.1093/nar/gkx1254
296. Turchinovich A, Tonevitsky AG, Burwinkel B. Extracellular miRNA: A Collision of Two Paradigms. Trends Biochem Sci (2016) 41(10):883–92. doi: 10.1016/j.tibs.2016.08.004
297. Ahadi A, Brennan S, Kennedy PJ, Hutvagner G, Tran N. Long Non-Coding RNAs Harboring miRNA Seed Regions Are Enriched in Prostate Cancer Exosomes. Sci Rep (2016) 6:24922. doi: 10.1038/srep24922
298. Villarroya-Beltri C, Gutiérrez-Vázquez C, Sánchez-Cabo F, Pérez-Hernández D, Vázquez J, Martin-Cofreces N, et al. Sumoylated Hnrnpa2b1 Controls the Sorting of miRNAs Into Exosomes Through Binding to Specific Motifs. Nat Commun (2013) 4:2980. doi: 10.1038/ncomms3980
299. Teng Y, Ren Y, Hu X, Mu J, Samykutty A, Zhuang X, et al. MVP-Mediated Exosomal Sorting of miR-193a Promotes Colon Cancer Progression. Nat Commun (2017) 8:14448. doi: 10.1038/ncomms14448
300. Geekiyanage H, Rayatpisheh S, Wohlschlegel JA, Brown R, Ambros V. Extracellular microRNAs in Human Circulation Are Associated With miRISC Complexes That Are Accessible to Anti-AGO2 Antibody and Can Bind Target Mimic Oligonucleotides. Proc Natl Acad Sci USA (2020) 117(39):24213–23. doi: 10.1073/pnas.2008323117
301. Turchinovich A, Weiz L, Langheinz A, Burwinkel B. Characterization of Extracellular Circulating microRNA. Nucleic Acids Res (2011) 39(16):7223–33. doi: 10.1093/nar/gkr254
302. O’Brien J, Hayder H, Zayed Y, Peng C. Overview of MicroRNA Biogenesis, Mechanisms of Actions, and Circulation. Front Endocrinol (Lausanne) (2018) 9:402. doi: 10.3389/fendo.2018.00402
303. Sedgeman LR, Beysen C, Ramirez Solano MA, Michell DL, Sheng Q, Zhao S, et al. Beta Cell Secretion of miR-375 to HDL Is Inversely Associated With Insulin Secretion. Sci Rep (2019) 9(1):3803. doi: 10.1038/s41598-019-40338-7
304. de Candia P, Spinetti G, Specchia C, Sangalli E, La Sala L, Uccellatore A, et al. A Unique Plasma microRNA Profile Defines Type 2 Diabetes Progression. PLoS One (2017) 12(12):e0188980. doi: 10.1371/journal.pone.0188980
305. Satake E, Pezzolesi MG, Md Dom ZI, Smiles AM, Niewczas MA, Krolewski AS. Circulating miRNA Profiles Associated With Hyperglycemia in Patients With Type 1 Diabetes. Diabetes (2018) 67(5):1013–23. doi: 10.2337/db17-1207
306. Lamadrid-Romero M, Solís KH, Cruz-Reséndiz MS, Pérez JE, Díaz NF, Flores-Herrera H, et al. Central Nervous System Development-Related microRNAs Levels Increase in the Serum of Gestational Diabetic Women During the First Trimester of Pregnancy. Neurosci Res (2018) 130:8–22. doi: 10.1016/j.neures.2017.08.003
307. Guay C, Menoud V, Rome S, Regazzi R. Horizontal Transfer of Exosomal microRNAs Transduce Apoptotic Signals Between Pancreatic Beta-Cells. Cell Commun Signal (2015) 13:17. doi: 10.1186/s12964-015-0097-7
308. Guay C, Kruit JK, Rome S, Menoud V, Mulder NL, Jurdzinski A, et al. Lymphocyte-Derived Exosomal MicroRNAs Promote Pancreatic β Cell Death and May Contribute to Type 1 Diabetes Development. Cell Metab (2018). doi: 10.1016/j.cmet.2018.09.011
309. Li J, Zhang Y, Ye Y, Li D, Liu Y, Lee E, et al. Pancreatic β Cells Control Glucose Homeostasis via the Secretion of Exosomal miR-29 Family. J Extracell Vesicles (2021) 10(3):e12055. doi: 10.1002/jev2.12055
310. Thomou T, Mori MA, Dreyfuss JM, Konishi M, Sakaguchi M, Wolfrum C, et al. Adipose-Derived Circulating miRNAs Regulate Gene Expression in Other Tissues. Nature (2017) 542(7642):450–5. doi: 10.1038/nature21365
311. Ying W, Riopel M, Bandyopadhyay G, Dong Y, Birmingham A, Seo JB, et al. Adipose Tissue Macrophage-Derived Exosomal miRNAs Can Modulate In Vivo and In Vitro Insulin Sensitivity. Cell (2017) 171(2):372–84.e12. doi: 10.1016/j.cell.2017.08.035
312. Pomatto MAC, Gai C, Deregibus MC, Tetta C, Camussi G. Noncoding RNAs Carried by Extracellular Vesicles in Endocrine Diseases. Int J Endocrinol (2018) 2018:4302096. doi: 10.1155/2018/4302096
313. Nasteska D, Hodson DJ. The Role of Beta Cell Heterogeneity in Islet Function and Insulin Release. J Mol Endocrinol (2018) 61(1). doi: 10.1530/JME-18-0011
314. Ellenbroek JH, Töns HA, de Graaf N, Loomans CJ, Engelse MA, Vrolijk H, et al. Topologically Heterogeneous Beta Cell Adaptation in Response to High-Fat Diet in Mice. PLoS One (2013) 8(2):e56922. doi: 10.1371/journal.pone.0056922
315. Piñeros AR, Gao H, Wu W, Liu Y, Tersey SA, Mirmira RG. Single-Cell Transcriptional Profiling of Mouse Islets Following Short-Term Obesogenic Dietary Intervention. Metabolites (2020) 10(12). doi: 10.3390/metabo10120513
316. Faridani OR, Abdullayev I, Hagemann-Jensen M, Schell JP, Lanner F, Sandberg R. Single-Cell Sequencing of the Small-RNA Transcriptome. Nat Biotechnol (2016) 34(12):1264–6. doi: 10.1038/nbt.3701
Keywords: miRNA, islet, β-cell, type 2 diabetes, glucose, nutrients, diet
Citation: Sałówka A and Martinez-Sanchez A (2021) Molecular Mechanisms of Nutrient-Mediated Regulation of MicroRNAs in Pancreatic β-cells. Front. Endocrinol. 12:704824. doi: 10.3389/fendo.2021.704824
Received: 04 May 2021; Accepted: 02 August 2021;
Published: 04 November 2021.
Edited by:
Magalie A. Ravier, U1191 Institut de Génomique Fonctionnelle (IGF) (INSERM), FranceReviewed by:
Lena Eliasson, Lund University, SwedenSushant Bhatnagar, University of Alabama at Birmingham, United States
Copyright © 2021 Sałówka and Martinez-Sanchez. This is an open-access article distributed under the terms of the Creative Commons Attribution License (CC BY). The use, distribution or reproduction in other forums is permitted, provided the original author(s) and the copyright owner(s) are credited and that the original publication in this journal is cited, in accordance with accepted academic practice. No use, distribution or reproduction is permitted which does not comply with these terms.
*Correspondence: Aida Martinez-Sanchez, YS5tYXJ0aW5lei1zYW5jaGV6QGltcGVyaWFsLmFjLnVr