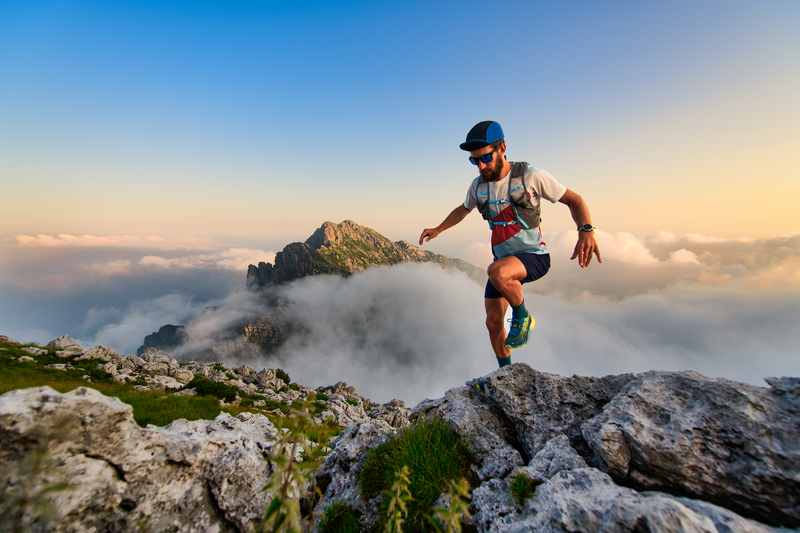
95% of researchers rate our articles as excellent or good
Learn more about the work of our research integrity team to safeguard the quality of each article we publish.
Find out more
ORIGINAL RESEARCH article
Front. Endocrinol. , 15 July 2021
Sec. Diabetes: Molecular Mechanisms
Volume 12 - 2021 | https://doi.org/10.3389/fendo.2021.700049
This article is part of the Research Topic Antidiabetic Molecular Targets: Updates on Old and Emerging Targets and their Small Molecule Modulators View all 8 articles
Dietary supplements have improved the prevention of insulin resistance and metabolic diseases, which became a research hotspot in food science and nutrition. Obesity and insulin resistance, caused by a high-fat diet, eventually result in severe metabolic diseases, can be prevented with the dietary supplement D-chiro-inositol (DCI). In this work, we isolated mice primary hepatocytes with palmitic acid stimulation and DCI was applied to compare and contrast its effects of in primary hepatocyte biology. Before and after intervention with DCI, we used RNA-Seq technology to establish a primary hepatocyte transcriptome gene profile. We found that both PA and DCI cause a wide variation in gene expression. Particularly, we found that DCI plays critical role in this model by acting on glycolysis and gluconeogenesis. Overall, we generated extensive transcripts from primary hepatocytes and uncovered new functions and gene targets for DCI.
Adjustments in diet structure play a crucial role in improving human health and disease. Excessive energy intake can easily induce obesity, which causes insulin resistance, that is becoming a serious problem that threatens human health, due to increased type 2 diabetes incidence of and other metabolic diseases (1–3). Therefore, it is necessary to adjust the diet composition and control calories to improve insulin sensitivity and prevent diseases that are caused by increased caloric intake, such as diabetes and cardiovascular disease (4, 5). At present, we have not been able to grasp the potential molecular processes that drive the physiological and metabolic differences caused by high-fat diet intake.
The metabolic regulator of the human body is the liver and dietary intake is the main link in the body’s energy supply (6, 7). The liver needs to metabolize several substances to make effective use. Therefore, changes in diet can cause changes in the metabolic mechanism of the liver. The liver energy balance and glucose homeostasis are primarily maintained by the regulation of insulin concentration. Excessive fat intake can lead to obesity and insulin resistance (8), which can affect liver blood sugar regulation, leading to hyperglycemia (9). The adjustment of diet structure and intake can reduce the abnormal accumulation of fat in the liver, improve glycogen regulation and reduce inflammation (10, 11). Therefore, analyzing the effects of dietary changes on liver regulation can improve our understanding of the liver metabolic regulation.
D-chiro inositol (DCI) is an epimer of inositol that is abundant in plants (12, 13), such as soybean (14), buckwheat (15) and pumpkin (16). At present, the method of batch preparation of DCI is through natural product extraction and the main test material is Fagopyrum esculentum Moench. It is a combination of edible and fake plant resources (17), in comparison with common cereal crops, such as wheat, rice and oats that are rich in D-chiro-inositol. In the human body, endogenous DCI is mainly derived from dietary intake (18) and inositol is converted into its stereoisomer by a putative epimerase. Buckwheat has received increasing attention due to its therapeutic effects on metabolic diseases (19), such as diabetes (20) and hypertension (21). It has been suggested that DCI improves endothelial dysfunction and has antioxidant activity in diabetic rats (22). DCI is mainly distributed in the blood and tissues sensitive to peripheral targets, such as the liver and fat. Studies have shown that elevated levels of DCI in plasma, liver, muscle and fat can improve PCOS or diabetes (23, 24). These results suggest that insulin resistance is susceptible to tissue DCI deficiency; therefore, DCI supplementation may have an effect on insulin sensitivity (25, 26). Previous laboratory studies have shown that DCI can enhance insulin sensitivity and regulate energy metabolism (27, 28).It was also found that DCI exhibits pyruvate dehydrogenase activity by inactivating the pyruvate dehydrogenase kinase (PDK) (29), which increased the possibility that DCI could transfer mitochondrial pyruvate to oxidation in liver cells; thereby, limiting the gluconeogenesis of pyruvate carboxylation (30).
With the rise and application of RNA-Seq technology, a relatively comprehensive and in-depth sequencing technology, that analyzes the transcriptome, was born (31–33). This application has given a new understanding and direction in understanding the complexity and specificity of substances. To some extent, RNA-Seq technology compared with other methods is more efficient, accurate and provides detailed analysis of the results. The transcriptome is called the “group” largely due to its own integrity that allows RNA analysis of cells at different growth periods and conditions, rendering personalized customization possible for a variety of tests (34, 35). Analyzing the transcriptome is essential for interpreting the functional components of the genome and in revealing the molecular components of cells and tissues, and for understanding development and disease. In order to fully reveal the mechanism of D-chiro-inositol’s high efficiency in stabilizing blood glucose in animal experiments, we established the pa-induced mRNA gene profile of mouse primary hepatocytes by RNA-Seq technology and explored the detailed mechanism of natural compounds that are involved in regulating the expression levels of key genes and proteins. The liver is the main organ of biotransformation and protein synthesis, where small molecules and natural active substances play a main role. The liver was studied using mice that are susceptible to the body’s regulating factors of intervention, such as D-chiro-inositol. In this study, we investigated the effect of D-chiro-inositol on the cause of insulin resistance, induced by PA, in primary hepatocyte cell model to determine the interaction between the two molecules and other biological processes.
The primary hepatocytes were isolated with HBSS (Hyclone, Logan, UT, USA) and collagenase IV (Gibco, Grand Island, NY, USA) (36, 37). The plates used were pre-coated with rat tail collagen I (BD Biosciences, China), and the cells seeded in DMEM LOW (Hyclone, Logan, UT, USA), supplemented with 10% Fetal bovine serum (FBS)(Gibco, Grand Island, NY, USA), 1% 2-hydroxyethyl, 0.5 μg/ml hydrocortisone, 0.5 μg/ml insulin (Gibco, Grand Island, NY, USA) and 100 units/mL of penicillin/streptomycin. After attachment (4 h), the medium was removed, and the hepatocytes incubated (20 h) in medium the above without FBS. We incubated the hepatocytes for 24 h with PA (100 μM, Sigma) to generate an insulin resistance model and for early intervention with DCI (10 μM) (purity > 98%, Yuanye BioTechnology Co., Shanghai, China) which was dissolved in PBS.
Total RNA was extracted from primary hepatocytes using Trizol (Trizol, Takata, Japan) and according to the manufacturer instructions. Three PA and three DCI samples were prepared. The samples were quantified by Nanodrop 2000 spectrophotometer (Thermo Fisher Scientific) and the RNA qualitatively controlled by agarose gel electrophoresis and the Agilent 2100 biological analyzer (Agilent Technologies Inc. CA, USA). All samples showed concentrations greater than 150 ng/μL and total RNA (RIN) levels were greater than 7 (showed in supplement).
The establishment of the database was carried out at Genedenovo Biotechnology Co., Ltd (Guangzhou, China). The RNA-seq library was performed using NEBNext® Ultra ™RNA Library Prep Kit for Illumina® (NEB#7530, New England Biolabs) and the 150 bp paired terminal sequencing was performed on the Illumina HiSeq 4000 platform. The sampling read quality and potential contamination were performed using FastQC v0.11.5 detection and filtration fittings. Next, the clean reads were aligned to the Mus musculus genome (GRCm38.p6) with fast and sensitive Tophat2 (v2.1.1) (37), using Mus musculus genome index and including transcript structures. The Differential expression analysis was performed by edgeR (v3.12.1). A quantitative method for gene expression was a transcribed fragment read per million bases per million markers (FPKM).
We used pheatmap package in R software for heatmap analysis. Genes with fold change ≥ 2 and false discovery rate (FDR) of < 0.05 were considered significant DEGs. After that, the DEGs were subjected to enrichment analysis of the GO function and the KEGG pathway. GO and KEGG pathways with a Q-value ≤ 0.05- corrected P-value were significantly enriched in DEGs.
To generate cDNA, the extracted RNA was subjected to RT-PCR, according to the SYBR® Premix Ex Taq II (TAKARA BIO INC. Japan) reagent instructions and using an ABI 7500 Real-Time PCR machine (Life, Qiagen, Germany). The primer sets used for the quantitative real time PCR analysis are shown in Table 1.
The inoculated cells were washed 2-3 times with PBS. Two mL of 4% Paraformaldehyde was added to each well of a 6-well plate and fixed at 4°C for 30 min. The solution was changed or directly added to PBS for shaking 2 to 3 times. Subsequently, the blocking and incubation of the antibody were carried out according to the standard of specification and the dilution concentration of the selected antibody in the pre-experiment and incubated for 1.5 to 2 h. After recovering the primary antibody, the mixture was shaken and washed 2 to 3 times, and the fluorescent secondary antibody was incubated in the dark. DAPI was used to stain the nuclei and the excess dye was discarded. After washing with PBS, the tablets were sealed and developed.
An equal amount of the sample was subjected to SDS-PAGE electrophoresis and then the protein was transferred to a 0.45 μm-well PVDF membrane (Millipore Co., Ltd.) (90 V, 90 minutes). The skimmed milk powder was sealed for 2 hours. The primary antibody required was incubated overnight at 4°C and the corresponding secondary antibody for 1.5-2 hours at room temperature on a shaker. After washing with TBST 2-3 times, an appropriate amount of ECL luminescent reagent was uniformly added to the PVDF membrane and developed in a Chemi doc + XRS system. The gray scale analysis was performed using the image Lab software to calculate the optical density ratio of the target.
All data presented in the results were analyzed by Graphpad Prism 6, ANOVA for univariate analysis, Tukey’s multiple comparison and the results were analyzed from 3 or more independent experiments using mean ± standard error (AVG ± SEM). In the statistics, p < 0.05 was significantly different and p < 0.01 was extremely significant.
RNA seq analysis was performed to determine the expression profile of mRNA in primary hepatocytes upon DCI supplementation. A total of 37.92 and 54.87 million reads were aligned to the Mus musculus reference genome (GRCm38.p6) in the PA and DCI groups, respectively. A total of 1354 differentially expressed genes (DEGs) were obtained from the 3 treatment groups (Figures 1A–D). The comparative study between different treatment groups found that in the comparison of differential genes between CK and PA group, 105 genes were up-regulated, and 102 genes were down-regulated (Figure 1E). Compared with the DCI group, the number of significantly up-regulated genes was 481 and the number of down-regulated genes was 302.The above results indicated, that compared with CK group, the PA treatment of mice primary hepatocytes could change the expression of many genes, and that DCI intervention could regulate the gene transcription.
Figure 1 Venn diagram and volcano plot of DEGs between different groups using RNA sequencing. Venn diagram shows the distribution of DEGs among three groups. CK, control group; PA, PA-treated group; CK, PA, and 10 μM D-chiro-inositol cotreated group. Red, green, and black indicate the downregulation, no significant change, and up-regulation, respectively. (A) Venn diagram; (B–D) Volcano plot of differentially expressed genes between different groups; (E) The number of differentially expressed genes.
The relevant heatmaps of DEGs in the sequencing results were analyzed to establish heatmaps of DEGs in primary hepatocytes under different treatment methods in the CK group, PA group and DCI group (Figure 2).
Figure 2 Heatmap of differentially expressed genes profiling between three different conditions via RNA-Seq.
At the same time, the DEGs of different treatment groups were classified. In the GO library, we can compare the research data with the existing mouse sequencing data in the library and classify the differential in genes expression into annotations that are based on the molecular function (MF), biological processes (Biological Process, BP) and cellular components (CC). By comparison (Figure 3A), D-chiro inositol gene regulation in primary liver cells was mainly concentrated on biological processes, including biological regulation and cellular, metabolic and single organism processes. Based on the mRNA-gene regulatory information, KEGG was used to analyze a significantly enriched mRNA to clearly study the biological effects of gene differences between different groups (Figure 3B). The study found that the major enriched signaling pathways were Lipid metabolism, energy metabolism, pyruvate metabolism, PI3K-Akt, MAPK, TNF and glucose signaling pathways. The number of differential genes enriched by cAMP, FoxO signaling pathway and Glycolysis/Gluconeogenesis was higher (p < 0.05). This is consistent with our previous studies that found that D-chiro inositol is an insulin sensitizer, further confirming the reliability of sequencing results (Figure 3C).
Figure 3 GO/KEGG Pathways of the differentially expressed genes. (A) GO terms for the DEGs; (B) KEGG terms for the DEGs; (C) The top 28 ranging KEGG pathways of the DEGs. Canonical signaling pathway (KEGG) enriched under DCI induction. control group(CK), PA treated group (PA), and 10 μM DCI and PA cotreated group (DCI).
As shown in Figure 4, the changes of intracellular proteins were investigated by immunofluorescence staining. The expression of PKCϵ is shown by red fluorescence and the nucleus that was stained by DAPI in blue. The results showed that the expression of PKCϵ on the cell membrane was significantly increased under PA stimulation. The intervention of MET and DCI inhibited the expression of PKCϵ on the cell membrane.
Figure 4 The effect of D-chiro-inositol on the expression of PKC in the PA-induced primary hepatocyte ( ± SD, n≥3).
The main proteins in the upstream and downstream of PI3K/AKT signaling pathway were selected and their protein expression levels determined. The protein sites related to IRS-2, PI3K, AKT and FOXO1 were involved in the establishment of insulin resistance model and we aimed at determining the effective regulatory role of DCI in this process. As shown in Figure 5, PA treatment of mouse primary liver cells significantly reduced p-IRS-2, AKT and FOXO1. Compared with the PA-induced group, the DCI treatment group significantly up-regulated p-IRS-2, AKT and FOXO1. At the same time, DCI significantly up-regulated the expression of PI3K protein (p < 0.05).
Figure 5 The effect of D-chiro-inositol on insulin resistance signal pathway in the PA-induced primary hepatocyte ( ± SD, n≥3). Representative western blots expression and the protein bands were quantified by densitometry of (A) p-IRS-2/IRS-2; (B) PI3K; (C) p-AKT/AKT; (D) p-FOXO1/FOXO. *p < 0.05, **p < 0.01 compared with the CK group; #p < 0.05, ##p < 0.01 compared with the PA group.
According to the RT-PCR results (Figure 6), the expression of PTP1B was significantly increased in the PA group compared with the CK group (p < 0.05). As for the PA group, the expression of PTP1B was significantly decreased in the DCI group (p < 0.05). At present, many studies are shifting the focus of XBP1S research to the regulation of glucose homeostasis. SREBP-1c acts as an important site in insulin signaling. The FABP4 can improve the sensitivity of insulin and is a possible target for T2MD. As shown in Figures 6C, D, the gene expression of SREBP-1c and FABP4 in the PA group was significantly increased compared to the CK group (p < 0.05); while, the gene expression in the DCI group was significantly decreased compared to the PA group (p < 0.05). G6Pase is a key rate-limiting enzyme that regulates hepatic gluconeogenesis. Pepck also plays a role in the regulation of gluconeogenesis, effectively utilizing non-sugar substances in the process of raising blood sugar level and achieving its active transformation and energy supply. As shown in Figures 6E, F, the gene expression of PEPCK and G6pase in the PA group was significantly increased compared to the CK group (p < 0.05); while, the expression of the gluconeogenesis pathway negative regulator gene in was significantly higher in the DCI group. (p < 0.05).
Figure 6 The effect of D-chiro-inositol on insulin resistance signal pathway gene in the PA-induced primary hepatocyte ( ± SD, n≥3). (A) PTP1B mRNA expression; (B) XBP1S mRNA expression; (C) SREBP-1c mRNA expression; (D) FABP4 mRNA expression; (E) PEPCK mRNA expression; (F) G6pase mRNA expression. *p < 0.05, **p < 0.01 compared with the CK group; #p < 0.05, ##p < 0.01 compared with the PA group.
Although DCI can play a leading role in the liver metabolic regulation and blood glucose stability, no studies have reported the effect of DCI on gene expression profiles of primary hepatocytes. Our findings indicated that DCI helps in keeping the insulin resistance in relation to signaling pathways. The DCI metabolism is associated with insulin sensitivity, and this view supports the notion that DCI acts as a second messenger of insulin response. In addition, DCI supply has been previously shown to reduces liver weight and our data is consistent with the observations by Larner, J (38).
The previous studies showed that PA-stimulated cells trigger oxidative-stress and related metabolic disorders. For example, PA stimulates HepG2 cells resulting in glucose metabolic disorders (25). In our experiments, it was found that 100 μM PA stimulated mouse liver primary cells to trigger hepatic glucose output disorder, indicating that high concentrations of PA can induce insulin resistance in primary cells. In addition, DCI has been found to prevent PA-mediated endothelial dysfunction (28). At the same time, it has been reported that DCI can activate pyruvate dehydrogenase to exert a role in improving glucose disorder and lipid metabolism in mice. In our study, DCI was shown to restore PA-induced signaling pathways involved in in primary liver cells’ insulin resistance.
In this study, 1354 DEGs were obtained. As a natural sugar alcohol compound, DCI can protect against glucose-lipid metabolism disorder, induced by PA in mouse liver primary cells. By using high-throughput RNA seq technology, the gene expression changes in primary hepatocytes, under the intervention of DCI, were explored. Based on the results, 1354 unique DEGs were detected in the DCI supplementation, many of which are involved in biological regulation and metabolic processes (e.g. cell regulatory processes and biological regulation) and are primarily located in the cell membrane and cytoplasm. In addition, KEGG analysis revealed several metabolically related pathways (PI3K/AKT, MAPK, glucose metabolism pathway), FoxO and immune response-related signaling pathways. The results of the enrichment analysis also provided assurance of the accuracy and a certain degree of support for the study results. The results of pathway enrichment showed that the up-regulated DEGs were mainly enriched in the PI3K/AKT pathway. In 1985, Phosphoinositide 3-kinase (PI3K) was discovered. After several decades of research, the PI3K/AKT pathway is of great research value due to its extensive involvement (39, 40). The PI3K/AKT signaling pathway acts as an important part in key cell processes, such as growth, lipoprotein metabolism, protein synthesis, cell proliferation and apoptosis (41). Interestingly, the activation of the PI3K/AKT pathway inhibits hepatic gluconeogenesis (42) and according to the sequencing results, the differential gene enrichment in PI3K/AKT related signaling pathways, including Pik3cg, Pik3r3, Socs1 and other related genes, were significantly expressed after DCI treatment. Based on this, we put forward relevant research directions in the function mining of DCI. The possible regulatory molecular mechanism of DCI is associated with PI3K/AKT related pathway, which regulates the expression of other related nodes on the whole signaling pathway and to improve hepatic insulin resistance. Related differential genes are also abundantly enriched in the glycogen synthesis/gluconeogenesis pathway and many studies have demonstrated that the gluconeogenesis pathway plays a decisive role in hepatic insulin resistance (43).
The kidney and liver are the main sites of endogenous glucose production, but only the liver can respond to insulin by regulating glucose levels (44). In the fasting state, the energy supply of the human body is mainly achieved by the dynamic regulation of glycogen synthesis and decomposition by the liver (41). The glycogen synthesis and decomposition are transported to various tissues of the human body; while, inhibiting the synthesis of new fatty acids. After eating, the human body controls the level of hepatic glucose production (HGP) through the PI3K/AKT pathway and stores glycogen for other tissues with sufficient energy. The G6pc and Pklr genes are enriched in the gluconeogenesis pathway. The levels of G6pc and Pklr genes were significantly down-regulated after DCI intervention, indicating that DCI can reduce gluconeogenesis and increase glycogen synthesis, thus improving glucose uptake and promoting peripheral insulin resistance. In addition, studies have found that the effect of DCI on hepatic glucose output and its significant consumption in metabolic diseases (45, 46), can help in explaining the antidiabetic and insulin sensitivities of DCI (47, 48). Therefore, we hypothesize that the inhibition of gluconeogenesis may be a biological process, caused by the effects of DCI on hepatic insulin resistance. In the path rich after DCI intervention, Pik3cg, Pik3r3 and Socs1 have higher node scores. The PI3K/AKT signaling pathway regulates a variety of cellular and biological processes and multiple functional activities. Therefore, the regulation of this pathway is associated with the improvement of various diseases, such as obesity, diabetes and cancer. Under normal conditions, the PI3K/AKT pathway is activated when regulating body functions. The PI3K/AKT pathway is inhibited when excessive energy intake occurs. The relief of obesity and insulin resistance is through the activation of the PI3K/AKT pathway. When the PI3K regulation mechanism is disturbed, an abnormal expression occurs, that lead to the occurrence of many human diseases, such as obesity and cancer (49). Studies have found that the PI3K inhibition is effective in preventing obesity, which greatly reverses human diseases (50). In summary, the three DEGs, that we have enriched and screened, play a key regulatory role in the process of alleviating the disease, and play a vital role in understanding the biological activity of DCI. We are more convinced that DCI is in the liver. As there is a certain regulation in insulin resistance.
Studies have found that insulin inhibits the expression of PEPCK and G6PC. PI3K/AKT pathway increase the hepatic gluconeogenesis (51–53) and insulin reduces the expression of gluconeogenesis-related genes (54). Based on these results, AKT is not only an essential regulator of insulin, but may also stimulate the extrahepatic insulin to regulate hepatic glucose metabolism by other means. Three pathways may mediate the effects of cellular involuntary effects on hepatic glucose metabolism (55). First, the expression of G6PC and hepatic glucose (HGP) in the liver is reduced by the action of insulin in the K (ATP) channel. Subsequently, insulin controls this process through the PI3K-PIP3 signaling pathway in the AgR-related peptide (AgRP) neurons (56). Secondly, insulin inhibits lipolysis through the AKT pathway, reduces circulating FFA levels in adipose tissue, and inhibits HGP in the liver even in the absence of hepatic insulin signaling. Third, insulin function prevents the α-cells from controlling glucagon secretion in the brain via the AKT pathway, which reduces liver HGP levels (57). All three pathways require insulin to regulate HGP via PI3K. Our study also found similar pathways and sites of action through the biosignal analysis.
Our research has demonstrated the effect of D-chiro-inositol on the PI3K/AKT pathway-related genes and it has been reported that the PI3K/AKT pathway also mediates lipid synthesis. The steroid regulatory element binding protein (SREBP-1c) is a transcription factor that regulates cholesterol and phospholipid metabolism and plays an important role in insulin signaling. In the liver, the overexpression of the SREBP-1c gene is prone to lipogenesis but does not affect cholesterol synthesis. Studies have shown that AKT can affect the expression of SREBP-1c gene in the liver through the regulation of the mTORC1-S6K1 pathway (58). The role of FoxO1 in hepatic lipid metabolism is less studied and some studies have shown that FoxO1 is directly involved in the insulin-promoting expression of genes in the liver (59–61). However, our study found that FoxO1 can regulate HGP and provide additional results on the relationship between PI3K/AKT and FoxO1.
Many studies have shown that PTP1B has an important effect on the regulation of sugar homeostasis. For this reason, we have positioned this site as an important target site for affecting obesity or for the regulation of glucose homeostasis. The related regulation of PTP1B will affect the downstream expression of PI3K to some extent. XBP1S plays an important regulatory role in endoplasmic reticulum stress. Many studies reported that XBP1S can participate in the regulation of many cellular and biological activities, including cell differentiation, protein secretion and apoptosis. XBP1S plays a protective role and belongs to the positive regulators of cytokines. At present, many studies are shifting the focus of XBP1S research to the regulation of glucose homeostasis. XBP1S is closely related to the development of T1D, T2D and insulin. Reduced sensitivity, metabolic disorders and other processes can play a corresponding role. The FABP4 gene is a lipid carrier that is involved in the regulation of the metabolic syndrome and inflammation, and which improves insulin sensitivity. It is a potential target for the treatment of type 2 diabetes. G6Pase is a key rate-limiting enzyme that regulates hepatic gluconeogenesis. The G6Pase mainly controls the release of glycogen stored in the liver and the main function is to release glucose from the liver into the blood to regulate the body’s energy. Pepck also has a role in the regulation of gluconeogenesis, by effectively utilizing non-sugar substances in the process of raising blood sugar and achieving its active transformation and energy supply.
In patients with metabolic diseases caused by obesity, the reduction of FFA absorption and glucose utilization by the body fat tissue leads to ectopic accumulation of lipids in other tissues. Ectopic accumulation of fat is the main cause of insulin resistance in the body. Abnormal elevation of FFAs increases DAG levels, resulting in massive expression of acetyl-CoA in the liver, which in turn activates pyruvate carboxylase, promotes gluconeogenesis, inhibits PI3K/AKT pathway signaling and aggravates insulin resistance (62). Chronic metabolic inflammation and endoplasmic reticulum stress can also impair hepatic insulin-AKT signaling pathway and subsequent insulin resistance (63, 64). This provides a powerful basis for to study the mechanism of hepatic insulin resistance. DCI can effectively improve the role of glucose in energy supply by inhibiting oxidative stress, and improve the effect of insulin. In a related clinical study conducted on women of childbearing age, it was found that women at this stage are very likely to cause Polycystic ovary syndrome (PCOS), and the main cause of PCOS is insulin resistance. Oral DCI can improve the patient’s symptom (65). Researchers such as Yoshida K pointed out that the main reason for inducing PCOS is the decreased sensitivity of insulin in regulating the body’s blood glucose homeostasis and various tissues and organs, which hinders the signal transmission process (66). Oral DCI can enhance insulin sensitivity and improve PCOS (67). When PCOS is relieved, the ovarian function will be restored to its original function. The ovaries can well control the body’s male hormones under normal physiological functions (68, 69). Secretion, which solves the urgent need of people with PCOS, and also points out the direction for the next in-depth study of DCI.
Based on the DEGs obtained by transcriptome sequencing and analysis, a regulatory network was constructed and the corresponding biological functions of the relevant insulin-related signaling pathways were analyzed. The results showed that DCI played a pivotal role in the hepatocellular insulin resistance of PA through the IRS-2-PI3K/AKT pathway and that PTP1B, XBP1S, SREBP-1c, FABP4, G6Pase, Pepck and other genes were potential sites in dietary supplements.
The original contributions presented in the study are publicly available. This data can be found here: https://www.ncbi.nlm.nih.gov/bioproject/739485, accession PRJNA739485.
C-pF contributed to the conception of the study. FC, M-cC, J-lM, J-yL, and Y-fC performed the experiment. FC, C-pF, S-jY, and J-lC contributed significantly to analysis and manuscript preparation. FC performed the data analyses and wrote the manuscript. S-jY, and J-lC helped perform the analysis with constructive discussions. All authors contributed to the article and approved the submitted version.
The research were supported by the Science and Technology Innovation Project of Shanxi Agricultural University (2020BQ82).
The authors declare that the research was conducted in the absence of any commercial or financial relationships that could be construed as a potential conflict of interest.
PKC, protein kinase C; InsR, insulin receptor; PI3K, phosphoinositide-3-kinase; AKT, protein kinase B; FOXO1, fork-head frame protein O1; G6Pase, glucose-6-phosphatase; PTP1B, Protein Tyrosine Phosphatase 1b; XBP1, X-box binding protein1; FABP4, fatty acid-binding protein 4; SREBP-1c, sterol regulatory element binding protein-1c; PEPCK, phosphoenolpyruvate carboxykinase; DCI, D-chiro-inositol; IPG-P, inositol phosphoglycan phosphatase; PDK, pyruvate dehydrogenase kinase; PDH, pyruvate dehydrogenase complex; IRS-2, insulin receptor substrate proteins 2; PA, palmitic acid; DAG, diacylglycerol.
1. Biddinger SB, Kahn CR. From MICE to MEN: Insights Into the Insulin Resistance Syndromes. Annu Rev Physiol (2006) 68:123–58. doi: 10.1146/annurev.physiol.68.040104.124723
2. Farrell GC, Larter CZ. Nonalcoholic Fatty Liver Disease: From Steatosis to Cirrhosis. Hepatology (2006) 43:S99–S112. doi: 10.1002/hep.20973
3. Tilg H, Moschen AR. Insulin Resistance, Inflammation, and non-Alcoholic Fatty Liver Disease. Trends Endocrinol Metabol: TEM (2008) 19:371–9. doi: 10.1016/j.tem.2008.08.005
4. Bishop NA, Guarente L. Two Neurons Mediate Diet-Restriction-Induced Longevity in C. Elegans. Nature (2007) 447:545–9. doi: 10.1038/nature05904
5. Colman RJ, Anderson RM, Johnson SC, Kastman EK, Kosmatka KJ, Beasley TM, et al. Caloric Restriction Delays Disease Onset and Mortality in Rhesus Monkeys. Science (2009) 325:201–4. doi: 10.1126/science.1173635
6. Michael MD, Kulkarni RN, Postic C, Previs SF, Shulman GI, Magnuson MA, et al. Loss of Insulin Signaling in Hepatocytes Leads to Severe Insulin Resistance and Progressive Hepatic Dysfunction. Mol Cell (2000) 6:87–97. doi: 10.1016/S1097-2765(05)00015-8
7. DeFronzo RA, Gunnarsson R, Bjorkman O, Olsson M, Wahren J. Effects of Insulin on Peripheral and Splanchnic Glucose Metabolism in Noninsulin-Dependent (Type II) Diabetes Mellitus. J Clin Invest (1985) 76:149–55. doi: 10.1172/JCI111938
8. Pilkis SJ, Granner DK. Molecular Physiology of the Regulation of Hepatic Gluconeogenesis and Glycolysis. Annu Rev Physiol (1992) 54:885–909. doi: 10.1146/annurev.ph.54.030192.004321
9. Yu H, Jia W, Guo Z. Reducing Liver Fat by Low Carbohydrate Caloric Restriction Targets Hepatic Glucose Production in Non-Diabetic Obese Adults With Non-Alcoholic Fatty Liver Disease. J Clin Med (2014) 3:1050–63. doi: 10.3390/jcm3031050
10. Larson-Meyer DE, Newcomer BR, Heilbronn LK, Volaufova J, Smith AR, Alfonso AJ, et al. Effect of 6-Month Calorie Restriction and Exercise on Serum and Liver Lipids and Markers of Liver Function. Obesity (2008) 16:1355–62. doi: 10.1038/oby.2008.201
11. Cao SX, Dhahbi JM, Mote PL, Spindler SR. Genomic Profiling of Short- and Long-Term Caloric Restriction Effects in the Liver of Aging Mice. Proc Natl Acad Sci (2001) 98:10630–5. doi: 10.1073/pnas.191313598
12. Thomas MP, Mills SJ, Potter BV. The “Other” Inositols and Their Phosphates: Synthesis, Biology, and Medicine (With Recent Advances in Myo-Inositol Chemistry). Angew Chem Int Ed Engl (2016) 55:1614–50. doi: 10.1002/chin.201611260
13. Yao Y, Cheng XZ, Ren GX. Contents of D-chiro-inositol, Vitexin, and Isovitexin in Various Varieties of Mung Bean and its Products. Agric Sci China (2011) 10:1710–5. doi: 10.1016/S1671-2927(11)60169-7
14. Kim JI, Kim JC, Joo HJ, Jung SH, Kim JJ. Determination of Total Chiro-Inositol Content in Selected Natural Materials and Evaluation of the Antihyperglycemic Effect of Pinitol Isolated From Soybean and Carob. Food Sci Biotechnol (2005) 14:441–5. doi: 10.1016/j.foodpol.2005.06.005
15. Steadman KJ, Burgoon MS, Schuster RL, Lewis BA, Edwardson SE, Obendorf RL. Fagopyritols, D-chiro-inositol, and Other Soluble Carbohydrates in Buckwheat Seed Milling FractionsJ. J Agric Food Chem (2000) 48:2843–7. doi: 10.1021/jf990709t
16. Miranda-Perez ME, Ortega-Camarillo C, EscobarVillanueva MDC, Blancas-Flores G, Alarcon-Aguilar FJ. Cucurbita Ficifolia Bouché Increases Insulin Secretion in RINm5F Cells Through an Influx of Ca2+ From the Endoplasmic Reticulum. J Ethnopharmacol (2016) 188:159–66. doi: 10.1016/j.jep.2016.04.061
17. Steadman KJ, Burgoon MS, Schuster RL, Lewis BA, Edwardson SE, Obendorf RL. Fagopyritols, D-Chiro-Inositol, and Other Soluble Carbohydrates in Buckwheat Seed Milling Fraction. J Agric Food Chem (2000) 48:2843–7. doi: 10.1021/jf990709t
18. Lin X, Ma L, Gopalan C, Ostlund RE. d-chiro-Inositol is Absorbed But Not Synthesised in Rodents. Br J Nutr (2009) 102:1426–34. doi: 10.1017/S0007114509990456
19. Zhang ZL, Zhou ML, Tang Y, Li FL, Tang YX, Shao JR, et al. Bioactive Compounds in Functional Buckwheat Food. Food Res Int (2012) 49:389–95. doi: 10.1016/j.foodres.2012.07.035
20. Qiu J, Liu Y, Yue Y, Qin Y, Li Z. Dietary Tartary Buckwheat Intake Attenuates Insulin Resistance and Improves Lipid Profiles in Patients With Type 2 Diabetes: A Randomized Controlled Trial. Nutr Res (2016) 36:1392–401. doi: 10.1016/j.nutres.2016.11.007
21. Cheng D, Zhang X, Meng M, Han L, Li Z, Hou L, et al. The Protective Effect of a Buckwheat-Enriched Diet on Renal Injury in High Salt-Induced Hypertension in Rats. Food Funct (2016) 7:3548–54. doi: 10.1039/C6FO00296J
22. Nascimento NRF, Lessa LMA, Kerntopf MR, Sousa CM, Alves RS, Queiroz MGR, et al. Inositols Prevent and Reverse Endothelial Dysfunction in Diabetic Rat and Rabbit Vasculature Metabolically and by Scavenging Superoxide. Proc Natl Acad Sci U S A (2006) 103:218–23. doi: 10.1073/pnas.0509779103
23. Baillargeon JP, Diamanti-Kandarakis E, Ostlund RE Jr, Apridonidze T, Nestler JE. Altered D-chiro-inositol Urinary Clearance in Women With Polycystic Ovary Syndrome. Diabetes Care (2006) 29:300–5. doi: 10.2337/diacare.29.02.06.dc05-1070
24. Sun TH, Heimark DB, Nguygen T, Nadler JL, Larner J. Both Myo-Inositol to Chiro-Inositol Epimerase Activities and Chiro-Inositol to Myo-Inositol Ratios are Decreased in Tissues of GK Type 2 Diabetic Rats Compared to Wistar Controls. Biochem Biophys Res Commun (2002) 293:1092–8. doi: 10.1016/S0006-291X(02)00313-3
25. Cheng f, Han L, Xiao Y, Pan C, Li Y, Ge x, et al. D-Chiro-Inositol Ameliorates High Fat Diet-Induced Hepatic Steatosis and Insulin Resistance Via Pkcϵ-Pi3k/Akt Pathway. J Agric Food Chem (2019) 67:5957–67. doi: 10.1021/acs.jafc.9b01253
26. Ou Y, Lin L, Yang X, Pan Q. Cheng, X. Antidiabetic Potential of Phycocyanin: Effects on KKAy Mice. Pharm Biol (2013) 51:539–44. doi: 10.3109/13880209.2012.747545
27. Zhang B, Guo X, Li Y, Peng Q, Gao J, Liu B, et al. D-Chiro Inositol Ameliorates Endothelial Dysfunction Via Inhibition of Oxidative Stress and Mitochondrial Fission. Mol Nutr Food Res (2017) 61(8):1600710. doi: 10.1002/mnfr.201600710
28. McLean P, Kunjara S, Greenbaum AL, Gumaa K, Lopez-Prados J, Martin-Lomas M, et al. Reciprocal Control of Pyruvate Dehydrogenase Kinase and Phosphatase by Inositol Phosphoglycans. J Biol Chem (2008) 283(48):33428–36. doi: 10.1074/jbc.M801781200
29. Fillmore N, Mori J, Lopaschuk GD. Mitochondrial Fatty Acid Oxidation Alterations in Heart Failure, Ischemic Heart Disease, and Diabetic Cardiomyopathy. Br J Pharmacol (2014) 171(8):2080– 90. doi: 10.1111/bph.12475
30. Djebali S, Davis CA, Merkel A, Dobin A, Lassmann T, Mortazavi A, et al. Landscape of Transcription in Human Cells. Nature (2012) 489:101–8. doi: 10.1038/nature11233
31. Trapnell C, Hendrickson DG, Sauvageau M, Goff L, Rinn JL, Pachter L. Differential Analysis of Gene Regulation at Transcript Resolution With RNA-seq. Nat Biotechnol (2013) 31:46–53. doi: 10.1038/nbt.2450
32. Schunter C, Garza JC, Macpherson E, Pascual M. SNP Development From RNA-seq Data in a Nonmodel Fish: How Many Individuals are Needed for Accurate Allele Frequency Prediction? Mol Ecol Resour (2014) 14:157–65. doi: 10.1111/1755-0998.12155
33. Yadetie F, Zhang X, Hanna EM, Aranguren-Abadía L, Eide M, Blaser N, et al. Rna-Seq Analysis of Transcriptome Responses in Atlantic Cod (Gadus Morhua) Precision-Cut Liver Slices Exposed to Benzo[a]Pyrene and 17α- Ethynylestradiol. Aquat Toxicol (2018) 201:174–86. doi: 10.1016/j.aquatox.2018.06.003
34. Shaheen S, Fawaz F, Shah S, Büsselberg D. Differential Expression and Pathway Analysis in Drug-Resistant Triple-Negative Breast Cancer Cell Lines Using Rna-Seq Analysis. Int J Mol Sci (2018) 19:1810. doi: 10.3390/ijms19061810
35. Seglen PO. Preparation of Isolated Rat Liver Cells. Methods Cell Biol (1976) 13:29–83. doi: 10.1016/S0091-679X(08)61797-5
36. Vernia S, Cavanagh-Kyros J, Garcia-Haro L, Sabio G, Barrett T, Jung D, et al. The PPARalpha-FGF21 Hormone Axis Contributes to Metabolic Regulation by the Hepatic JNK Signaling Pathway. Cell Metab (2014) 20:512–25. doi: 10.1016/j.cmet.2014.06.010
37. Kim D, Pertea G, Trapnell C, Pimentel H, Kelley R, Salzberg SL. TopHat2: Accurate Alignment of Transcriptomes in the Presence of Insertions, Deletions and Gene Fusions. Genome Biol (2013) 14(4):R36. doi: 10.1186/gb-2013-14-4-r36
38. Larner JDL, Brautigan MO. Thorner D-Chiro-Inositol Glycans in Insulin Signaling and Insulin Resistance. Mol Med (2010) 16:543–52. doi: 10.2119/molmed.2010.00107
39. Whitman M, Kaplan DR, Schaffhausen B, Cantley L, Roberts TM. Association of Phosphatidyl Inositol Kinase Activity With Polyoma Middle-T Competent for Transformation. Nature (1985) 315(6016):239–42. doi: 10.1038/315239a0
40. Guo H, German P, Bai S, Barnes S, Guo W, Qi X, et al. The PI3K/AKT Pathway and Renal Cell Carcinoma. J Genet Genomics (2015) 42(7):343–53. doi: 10.1016/j.jgg.2015.03.003
41. Abeyrathna P, Su Y. The Critical Role of Akt in Cardiovascular Function. Vasc Pharmacol (2015) 74:38–48. doi: 10.1016/j.vph.2015.05.008
42. Wang C, Chi Y, Li J, Miao Y, Li S, Su W. FAM3A Activates PI3K p110alpha/Akt Signaling to Ameliorate Hepatic Gluconeogenesis and Lipogenesis. Hepatology (2014) 59(5):1779–90. doi: 10.1002/hep.26945
43. Liu TY, Shi CX, Gao R, Sun HJ, Xiong XQ, Ding L. Irisin Inhibits Hepatic Gluconeogenesis and Increases Glycogen Synthesis Via the PI3K/Akt Pathway in Type 2 Diabetic Mice and Hepatocytes. Clin Sci (Lond) (2015) 129(10):839–50. doi: 10.1042/CS20150009
44. Whiteman EL, Cho H, Birnbaum MJ. Role of Akt Protein Kinase B in Metabolism. Trends Endocrinol Metab (2002) 13(10):444–51. doi: 10.1016/S1043-2760(02)00662-8
45. Chang HHH, Choong B, Phillips AR, Loomes. The diabetic rat kidney mediates inosituria KM. And Selective Urinary Partitioning of D-Chiro-Inositol. Exp Biol Med (2015) 240(1):8–14. doi: 10.1177/1535370214543064
46. Baillargeon JP, Diamanti-Kandarakis E, Ostlund RE Jr., Apridonidze T, Iuorno MJ, Nestler JE. Altered D-Chiro-Inositol Urinary Clearance in Women With Polycystic Ovary Syndrome. Diabetes Care (2006) 29:300–5. doi: 10.2337/diacare.29.02.06.dc05-1070
47. Kawa JM, Taylor CG, Przybylski R. Buckwheat Concentrate Reduces Serum Glucose in Streptozotocin-Diabetic Rats. J Agric Food Chem (2003) 51:7287–91. doi: 10.1021/jf0302153
48. Nascimento NRF, Lessa LMA, Kerntopf MR, Sousa CM, Alves RS, Queiroz MGR. Inositols Prevent and Reverse Endothelial Dysfunction in Diabetic Rat and Rabbit Vasculature Metabolically and by Scavenging Superoxide. Proc Natl Acad Sci USA (2006) 103(1):218–23. doi: 10.1073/pnas.0509779103
49. Dornan GL, Burke JE. Molecular Mechanisms of Human Disease Mediated by Oncogenic and Primary Immunodeficiency Mutations in Class Ia Phosphoinositide 3-Kinases. Front Immunol (2018) 9:575. doi: 10.3389/fimmu.2018.00575
50. Ortega-Molina A, Lopez-Guadamillas E, Mattison JA, Mitchell SJ, Munoz-Martin M, Iglesias G. Pharmacological Inhibition of PI3K Reduces Adiposity and Metabolic Syndrome in Obese Mice and Rhesus Monkeys. Cell Metab (2015) 21(4):558–70. doi: 10.1016/j.cmet.2015.02.017
51. Schmoll D, Walker KS, Alessi DR, Grempler R, Burchell A, Guo S. Regulation of glucose-6-phosphatase Gene Expression by Protein Kinase Balpha and the Forkhead Transcription Factor FKHR. Evidence for Insulin Response Unit-Dependent and -Independent Effects of Insulin on Promoter Activity. J Biol Chem (2000) 275(46):36324–33. doi: 10.1074/jbc.M003616200
52. Lu M, Wan M, Leavens KF, Chu Q, Monks BR, Fernandez S, et al. Insulin Regulates Liver Metabolism In Vivo in the Absence of Hepatic Akt and Foxo1. Nat Med (2012) 18:388–95. doi: 10.1038/nm.2686
53. Dong XC, Copps KD, Guo S, Li Y, Kollipara R, DePinho RA, et al. Inactivation of Hepatic Foxo1 by Insulin Signaling is Required for Adaptive Nutrient Homeostasis and Endocrine Growth Regulation. Cell Metab (2008) 8:65–76. doi: 10.1016/j.cmet.2008.06.006
54. Titchenell PM, Chu Q, Monks BR, Birnbaum MJ. Hepatic Insulin Signalling is Dispensable for Suppression of Glucose Output by Insulin In Vivo. Nat Commun (2015) 6:7078. doi: 10.1038/ncomms8078
55. Titchenell PM, Quinn WJ, Lu M, Chu Q, Lu W, Li C. Direct Hepatocyte Insulin Signaling is Required for Lipogenesis But Is Dispensable for the Suppression of Glucose Production. Cell Metab (2016) 23(6):1154–66. doi: 10.1016/j.cmet.2016.04.022
56. Konner AC, Janoschek R, Plum L, Jordan SD, Rother E, Ma X. Insulin Action in AgRP-expressing Neurons is Required for Suppression of Hepatic Glucose Production. Cell Metab (2007) 5(6):438–49. doi: 10.1016/j.cmet.2007.05.004
57. Ramnanan CJ, Edgerton DS, Cherrington AD. Evidence Against a Physiologic Role for Acute Changes in CNS Insulin Action in the Rapid Regulation of Hepatic Glucose Production. Cell Metab (2012) 15(5):656–64. doi: 10.1016/j.cmet.2012.03.006
58. Li S, Ogawa W, Emi A, Hayashi K, Senga Y, Nomura K, et al. Role of S6K1 in Regulation of SREBP1c Expression in the Liver. Biochem Biophys Res Commun (2011) 412(2):197–202. doi: 10.1016/j.bbrc.2011.07.038
59. Banks AS, Kim-Muller JY, Mastracci TL, Kofler NM, Qiang L, Haeusler RA, et al. Dissociation of the Glucose and Lipid Regulatory Functions of FoxO1 by Targeted Knockin of Acetylation-Defective Alleles in Mice. Cell Metab (2011) 14(5):587–97. doi: 10.1016/j.cmet.2011.09.012
60. Haeusler RA, Hartil K, Vaitheesvaran B, Arrieta-Cruz I, Knight CM, Cook JR, et al. Integrated Control of Hepatic Lipogenesis Versus Glucose Production Requires FoxO Transcription Factors. Nat Commun (2014) 5:5190. doi: 10.1038/ncomms6190
61. Deng X, Zhang W, OS I, Williams JB, Dong Q, Park EA, et al. FoxO1 Inhibits Sterol Regulatory Element-Binding protein-1c (Srebp-1c) Gene Expression Via Transcription Factors Sp1 and SREBP-1c. J Biol Chem (2012) 287(24):20132–43. doi: 10.1074/jbc.M112.347211
62. Bugianesi E, Gastaldelli A, Vanni E, Gambino R, Cassader M, Baldi S, et al. Insulin Resistance in non-Diabetic Patients With non-Alcoholic Fatty Liver Disease: Sites and Mechanisms. Diabetologia (2005) 48(4):634–42. doi: 10.1007/s00125-005-1682-x
63. Utzschneider KM, Kahn SE. Review: The Role of Insulin Resistance in Nonalcoholic Fatty Liver Disease. J Of Clin Endocrinol Metab (2006) 91(12):4753–61. doi: 10.1210/jc.2006-0587
64. Chen W, Balland E, Cowley MA. Hypothalamic Insulin Resistance in Obesity: Effects on Glucose Homeostasis. Neuroendocrinology (2017) 104(4):364–81. doi: 10.1159/000455865
65. Iuorno MJ, Jakubowicz DJ, Baillargeon JP, Dillon P, Gunn RD, Allan G, et al. Effects of D-Chiro-Inositol in Lean Women With the Polycystic Ovary Syndrome. Endocrine Pract (2002) 8:417–23. doi: 10.4158/EP.8.6.417
66. Yoshida K, Yamaguchi T M, Morinaga M. Genetic Modification of Bacillus Subtilis for Production of D-Chiro-Inositol, an Investigational Drug Candidate for Treatment of Type 2 Diabetes and Polycystic Ovary Syndrome. Appl Environ Microbiol (2006) 72:1310–5. doi: 10.1128/AEM.72.2.1310-1315.2006
67. Cianci A, Panella M, Fichera M, Falduzzi C, Bartolo M, Caruso S. D-chiro-Inositol and Alpha Lipoic Acid Treatment of Metabolic and Menses Disorders in Women With PCOS. Gynecol Endocrinol (2015) 31(6):483–96. doi: 10.3109/09513590.2015.1014784
68. Unfer V, Dinicola S, Laganà AS, Bizzarri M. Altered Ovarian Inositol Ratios may Account for Pathological Steroidogenesis in PCOS. Int J Mol Sci (2020) 21:7157. doi: 10.3390/ijms21197157
Keywords: primary hepatocyte, D-chiro-inositol, RNA‐Seq, insulin resistance, molecule mechanism
Citation: Cheng F, Yun S-j, Cao J-l, Chang M-c, Meng J-l, Liu J-y, Cheng Y-f and Feng C-p (2021) Differential Gene Expression and Biological Analyses of Primary Hepatocytes Following D-Chiro-Inositol Supplement. Front. Endocrinol. 12:700049. doi: 10.3389/fendo.2021.700049
Received: 25 April 2021; Accepted: 02 June 2021;
Published: 15 July 2021.
Edited by:
Kamaldeep Paul, Thapar Institute of Engineering & Technology, IndiaReviewed by:
Vittorio Unfer, Sapienza University of Rome, ItalyCopyright © 2021 Cheng, Yun, Cao, Chang, Meng, Liu, Cheng and Feng. This is an open-access article distributed under the terms of the Creative Commons Attribution License (CC BY). The use, distribution or reproduction in other forums is permitted, provided the original author(s) and the copyright owner(s) are credited and that the original publication in this journal is cited, in accordance with accepted academic practice. No use, distribution or reproduction is permitted which does not comply with these terms.
*Correspondence: Cui-ping Feng, bmRmY3BAMTYzLmNvbQ==
Disclaimer: All claims expressed in this article are solely those of the authors and do not necessarily represent those of their affiliated organizations, or those of the publisher, the editors and the reviewers. Any product that may be evaluated in this article or claim that may be made by its manufacturer is not guaranteed or endorsed by the publisher.
Research integrity at Frontiers
Learn more about the work of our research integrity team to safeguard the quality of each article we publish.