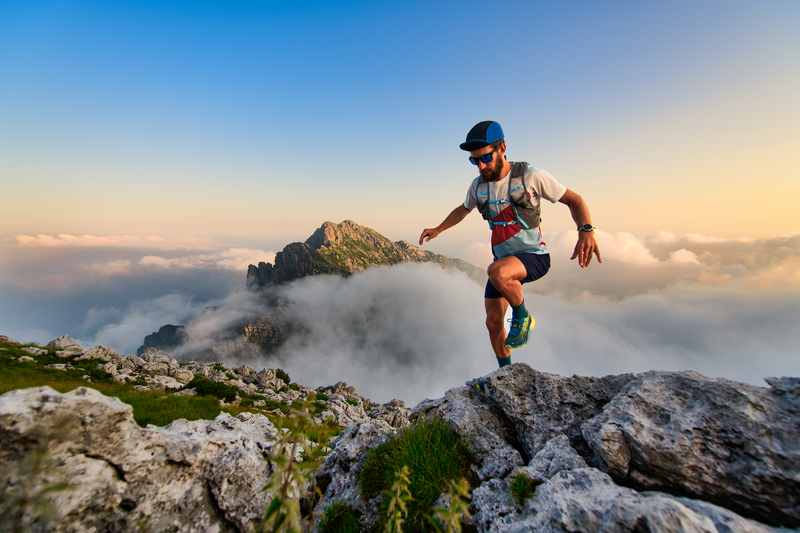
95% of researchers rate our articles as excellent or good
Learn more about the work of our research integrity team to safeguard the quality of each article we publish.
Find out more
REVIEW article
Front. Endocrinol. , 22 July 2021
Sec. Obesity
Volume 12 - 2021 | https://doi.org/10.3389/fendo.2021.696505
This article is part of the Research Topic Novel Therapeutic Strategy Against Obesity by Targeting Thermogenic Fat View all 6 articles
Brown and beige adipose tissues possess the remarkable capacity to convert energy into heat, which potentially opens novel therapeutic perspectives targeting the epidemic of metabolic syndromes such as obesity and type 2 diabetes. These thermogenic fats implement mitochondrial oxidative phosphorylation and uncouple respiration to catabolize fatty acids and glucose, which leads to an increase in energy expenditure. In particular, beige adipocytes that arise in white adipose tissue display their thermogenic capacity through various noncanonical mechanisms. This review aims to summarize the general overview of thermogenic fat, especially including the UCP1-independent adaptive thermogenesis and the emerging mechanisms of “beiging”, which may provide more evidence of targeting thermogenic fat to counteract obesity and other metabolic disorders in humans.
Because of the alarming prevalence of sedentary work and high-calorie diets, excessive energy is stored in the form of triglycerides in white adipose tissue (WAT), leading to a high incidence of obesity worldwide. Although the specific pathogenesis of obesity has not been elucidated, the main determining factor is the imbalance of long-term energy metabolism: increased energy intake and/or reduced energy expenditure (1). Suppressing appetite and gastrointestinal absorption by medical and surgical interventions are currently the main interventions to reduce energy intake, but the efficacy is limited. However, prolonged calorie restriction (CR) results in weight loss which promotes metabolic adaptation and decreases energy expenditure leading to an energy deficit (2). Physical training is another choice for energy expenditure. Nevertheless, obese patients generally have poor adherence to regular exercise, instead, the energy expenditure increases their compensatory dietary intake and sedentary time. Thus, it is urgent to find an alternative therapeutic treatment to counteract obesity (3). For the past few decades, brown and beige adipose tissues have been researched due to their distinctive characteristic to dissipate energy into heat. Functional brown adipose tissue (BAT), discovered in adult humans using 18F-flurodeoxyglucose positron emission tomography/computed tomography (18F-FDG PET/CT) (4), specifically targets thermogenic fat and provides a potential strategy to combat obesity and related complications.
In 2009, several human studies demonstrated that functional BAT is also present in adults (5, 6), which refutes the previous assumption that BAT disappears in the human body at an early age. Histologically, human brown adipocytes were found to contain multilocular smaller lipid droplets and plenty of uncoupling protein 1 (UCP1)-rich mitochondria, which are inextricably linked to its main function of thermogenesis. (Table 1) Moreover, there is a high uptake of glucose in the supraclavicular, cervical, paravertebral, and supra-adrenal regions through 18F-FDG PET/CT scan, especially after activation by cold exposure (5, 6).
BAT is formed during the embryonic stage and develops from the myf5+ lineage, consistently with skeletal muscle. BAT is mainly in the interscapular region in human infants and in the supraclavicular and neck regions in adults, with some additional localizations mentioned above (5, 7). A rich capillary bed provides sufficient oxygen and substrate during the regulation of cellular oxidation while dense sympathetic innervation works as an efferent nerve to send signals that focus on innervating and activating brown adipocytes (8, 9). Although active BAT is not abundant in adult humans, it plays a substantial role in energy metabolism depending on numerous thermogenic genes (10, 11). The improvement of PET/CT analysis also shows the metabolic capacity in human BAT is substantially higher than usually reported (12). Of note, although some samples of adult human BAT in neck share similarities with classical rodent BAT (4), more studies tend to the view that human BAT cells have a similar molecular signature with the beige fat cells of rodents (13, 14).
Despite the morphological and functional resemblance to brown adipocytes, beige adipocyte precursors are interspersed within WAT depots, especially in the inguinal region (15). These precursor cells are Myf5- but PDGFRα+ (16, 17). However, the expression of thermogenic genes in beige adipocytes is relatively low at basal, whereas it is induced by cold exposure or specific pharmacological stimulations, such as β-adrenergic stimulants or peroxisome proliferation-activated receptor γ (PPARγ) agonists, to a level equivalent to conventional brown adipocytes with good phenotypic plasticity (7–9, 18). Recently, a type of MyoD+-derived beige adipocyte has been discovered and termed as glycolytic beige adipocyte due to its characteristic of an enhanced glucose oxidation (19). Importantly, this beige fat contributes to cold adaptation in the absence of β3-adrenergic receptor (β3-AR) signaling, which is different from canonical beige fat and regarded as an innovative subtype of thermogenic fat (19–21). Under certain conditions, brown and beige adipocytes generate heat through canonical non-shivering thermogenesis (NST), which is an adaptive response to cold exposure or a high-calorie diet and differs from shivering thermogenesis induced by skeletal muscle (22). Of note, a rare subpopulation of adipocytes has been identified recently both in mice and humans through single-nucleus RNA-sequencing, which regulates BAT thermogenic capacity in a paracrine manner and may be a whole new area of research (23).
BAT quantity and quality differs among individuals. Some studies have indicated that active BAT is more frequently detected in women than men, and gradually decreases with ageing (24). The activity of BAT is inversely correlated to body mass index and glucose levels (25, 26). Thus, enhancement of BAT activation is more imperative to obese patients. Previous rodent studies showed that glucocorticoids have a powerful inhibitory effect on BAT-induced thermogenesis (27), while gonadal hormones (whether androgen or estrogen) may contribute to the differentiation of brown adipocytes (28, 29). A recent study indicated that ESR1, the gene encoding the estrogen receptor, is a requisite for both BAT mitochondrial remodeling via mitochondrial fission protein and thermogenesis via UCP1, which may reflect the mechanism of sexual dimorphism in BAT abundance and activity (30). Another earlier study showed that female rats were more cold-sensitive and had a high threshold temperature for cold-induced thermogenesis (around 22°C in females compared to 18°C in males), which may also be attributed to the higher BAT activity among women (31). As a cell fate switching, protein PR domain containing 16 (PRDM16) not only plays a decisive role in controlling the differentiation and maintaining the characteristics of brown adipocytes, but also robustly stimulates the formation of beige adipocytes in subcutaneous adipose depots (32, 33). Notably, adipocyte-specific deletion of PRDM16 leads to the absence of functional beige adipocytes, concomitant with hepatic steatosis, as another complication of obesity (34, 35). Furthermore, it has been found that cold adaptation relieves the inhibition of PRDM16 expression by down-regulating miR-133, which is highly expressed in adult skeletal muscle satellite cells and may be an effective target to recruit more active brown adipocytes (36).
The inherent ability of BAT on thermogenesis and accelerating glucose metabolism provides potential therapeutic targets to protect against obesity and its associated complications (37). BAT activation contributes not only to lower blood glucose levels (38), but also to an increase of lipolysis and the level of high density lipoprotein (HDL) cholesterol, which exerts positive effects on lipid metabolism (10, 39). In addition, as an endocrine organ, BAT secretes adipokines to keep metabolic health, including insulin-like growth factor 1 (IGF-1), neuregulin 4 (NRG4), and bone morphogenetic proteins (BMPs) (40). For instance, BAT-derived adipokine 12,13-dihydroxy-9Z-octadecenoic acid (12,13-diHOME) is increased in the intracellular and circulating levels by cold stimulation, which enhances the uptake of free fatty acids (FFAs) into brown adipocytes to facilitate thermogenesis (41). BAT-derived 12,13-diHOME also enhances cardiac function while the levels of 12,13-diHOME are decreased in human patients with heart disease (42), consistently with another cohort study which indicates a beneficial effect of BAT to cardiometabolic health (43). Multiple adipokines play a role in the crosstalk between BAT and other tissues, devoting to signal transmission, physiological regulation, and energy homeostasis (44). However, thermogenic fat also interacts with some pathological conditions. Human and murine obesity will enhance adipose tissue inflammation via direct adhesion of inflammatory macrophages, which cause the impaired beige adipogenesis (45). A signaling pathway between thermogenic fat and intestinal epithelial cells regulates intestinal disease tolerance under the condition of adrenergic activation (46). In the state of cancer cachexia, thermogenic fat is activated and whole body energy expenditure is enhanced as a maladaptive response to cachexia-induced anorexia (47, 48). Functional regulation of thermogenic fat may be involved in a promising approach to ameliorate cachexia in cancer patients. All the studies indicate that thermogenic fat plays a crucial role in a variety of pathophysiological states.
For both experimental animals and humans, cold exposure is a powerful trigger for the activation of brown and beige fat. It is interesting to note that cold-exposure-induced epigenetic programming of the sperm grants hyperactive BAT to the offspring (49). In this process, the hypothalamus and brain stem respond to the hypothermic environment and release norepinephrine (NE). NE then binds to β3-ARs on the membrane of brown adipocytes (50, 51)(Figure 1). Subsequently, activated adenosine cyclase-cAMP-protein kinase A (AC-cAMP-PKA) signaling pathway induces a cascade reaction, which results in the phosphorylation of certain proteins on the surface of intracellular lipid droplets, such as hormone-sensitive lipase (HSL) and perilipin 1 (PLIN1), followed by the hydrolysis of triglycerides and the release of FFAs. FFAs then activate UCP1, which is located on the mitochondrial inner membrane and primarily expresses in BAT. As an anion/proton symporter, UCP1 transports fatty acids into mitochondria for subsequent β-oxidation. Purine nucleotide ATP has an inhibitory effect on UCP1 while FFAs compete this effect (52, 53). However, the accomplishment of UCP1 activation is dependent on sufficient FFAs, whose level should be at least 100 times higher than ATP, suggesting that the activity of UCP1 is constantly inhibited under normal physiological conditions (53, 54). FFAs, especially long-chain fatty acids, are firmly bound to stimulate UCP1 and thereby mitochondrial respiration, which generates the proton-motive force (△P) to transport protons from mitochondrial intermembrane space into the matrix and provide energy to complete the ADP + Pi-ATP reaction with ATP synthase. Meanwhile, the overall increase in ATP/ADP ratio and △P will conversely in turn restrain mitochondrial respiration, as a negative feedback response to the uncoupling effect (51, 55).
Figure 1 Canonical non-shivering thermogenesis. In response to cold exposure and overeating, norepinephrine (NE) binds to β3-adrenoceptor (β3-AR), initiating adenosine cyclase-cAMP-protein kinase A (AC-cAMP-PKA) pathway. Subsequently the hormone-sensitive lipase (HSL) on the surface of lipid droplets is phosphorylated and the triglycerides translate into free-fatty-acid (FFA) after hydrolysis, which activates uncoupling protein 1 (UCP1) that inhibited by ATP, as a substrate for the following β-oxidation in the mitochondrial matrix. Additionally, interorgan communication provides more available substrates and fuels from exogenous sources, such as glucose, oxygen, FFA, acylcarnitine, metabolites, which come from the circulation, WAT, liver, and gut microbiome. Figure created using BioRender (https://biorender.com/).
Evidence shows that BAT works as a “metabolic sink” in human bodies. When activated by cold exposure, BAT uptakes FFAs and glucose as fuels. In addition to the above mentioned FFAs from lipid droplets, sympathetic nerve end-released NE induces the release of lipoprotein lipase (LPL) into circulation, which degrades chylomicrons and very low density lipoprotein (VLDL), followed by the generation of FFAs. In addition, glucose is another important substrate which is uptaken by BAT. Cold stress also activates the enzyme 12-lipoxygenase in BAT and then secretes an oxylipin 12-HEPE, which can shuttle glucose into brown adipocytes (56). There is a high demand of oxygen content for combusting FFAs and glucose, which is supplied from adjacent blood that absorbs the released heat. As a result, chronic cold acclimation arouses the overall metabolic capacity of BAT, which is the so-called process of BAT recruitment.
In the process of canonical non-shivering thermogenesis, thermogenic adipocytes are not the only fuel source; it also involves an interorgan communication (22). Cold exposure also promotes lipolysis in WAT, and the FFAs released into the circulation are directly utilized as an energy replenishment for heat production or are shuttled into the liver (57). In the liver, the activation of hepatocyte nuclear factor 4α (HNF4α) by FFAs promotes the expression of mitochondrial enzyme CPT1, which is involved in acylcarnitine synthesis. The synthetic acylcarnitine is another circulating substrate for BAT combustion (58). The loss-of-function experiment of the inability of hepatic acylcarnitine synthesis impairs adaptive thermogenesis as acylcarnitine application in aged mice is sufficient to prevent ageing-related hypothermia. Moreover, alternative bile acid synthesis pathway promotes the production of bile acids and shapes the intestinal microbiome, whose circulating metabolites promote the adaptive thermogenesis and energy expenditure (59). These recent findings imply that peripheral energy sources play a potential role in the canonical non-shivering thermogenesis, which involves a crosstalk between organs.
Undoubtedly, non-shivering thermogenesis(NST) plays a central role in the whole-body energy homeostasis. It is noticed that deficiency of UCP1, which is primarily thought to be necessary, or inability of adipose fatty acid oxidation in mice did not cause an obvious trend of obesity at thermoneutrality, suggesting that there are alternative thermogenic mechanisms (60).
It has been demonstrated that cold sensitivity in UCP1 knockout mice is completely restored in response to PRDM16 activation (61). The reason is that fat-selective PRDM16 overexpression in UCP1 knockout mice leads to a recruitment of beige adipocytes and produces more systemic energy output compared with the UCP1 knockout littermates. In addition, whether UCP1 is knocked out or not, the glucose tolerance and insulin sensitivity are significantly improved in PRDM16 transgenic mice under the high-fat diet state compared to the control mice. Hence, UCP1-deficient mice also possess a positive metabolic phenotype indicating that beige fat biogenesis driven by adipose-specific overexpression of PRDM16 protects from cold-induced hypothermia, diet-induced obesity, and glucose intolerance (33, 61).
Recently, UCP1-independent mechanisms involved in NST have been shown to include sarco/endoplasmic reticulum Ca2+-ATPase2b (SERCA2b)-mediated Ca2+ cycling and creatine-driven substrate cycling, however, they both mainly exist in beige fat due to a high demand of ATP (61–63). In response to cold stimuli, NE activates α1-adrenergic receptor (α1-AR) and β3-AR, which triggers intracellular Ca2+ flux. SERCA2b regulates Ca2+ uptake into the endoplasmic reticulum while ryanodine receptor 2 (RYR2) and inositol trisphosphate receptor (IP3R) promote its release, a process of futile cycling involved in ATP hydrolysis and thermogenesis. Meanwhile, SERCA2b-RYR2 pathway increases mitochondrial Ca2+ by calcium uniporter, which activates pyruvate dehydrogenase (PDH) and ATP synthase. Furthermore, beige adipocytes expend glucose as the primary fuel source through enhanced glycolysis for ATP generation in the context of UCP1 ablation and work as a “glucose-sink” to positively regulate glucose tolerance independent of body-weight loss. Thus, the loss of UCP1 in beige fat is able to be compensated, and this compensation is found not only in mice and humans, but even in pigs, a species that naturally lacks functional UCP1 (64).
Consistently, creatine-driven substrate cycling, involving its phosphorylation by mitochondria-localized creatine kinase (Mi-CK) and de-phosphorylation by tissue-nonspecifc alkaline phosphatase (TNAP), also plays a pivotal role in energy homeostasis in the lack of UCP1 (62, 65). Genes of creatine metabolism are induced after cold exposure through stimulation of creatine kinase activity, which promotes mitochondria respiration. Drug intervention to reduce creatine levels in beige fat attenuates lipid metabolism and systemic energy output, while core body temperature of UCP1−/− mice is difficult to maintain. These phenomena prove that creatine metabolism contributes to beige adipose-specific energy expenditure and thermogenesis. It should be noted that once long-term β3-adrenergic stimulation initiates “beiging”, creatine-driven substrate cycling would co-exist with canonical thermogenesis to orchestrate the effects of basal metabolic rates (66). What’s more, owing to the similar molecular characteristics between human brown adipocytes and murine beige adipocytes mentioned above, it has been identified that creatine regulates the respiration of isolated human brown adipocytes, which could open up possibilities to manipulate thermogenesis in patients with obesity and other metabolic diseases. In conclusion, these non-canonical thermogenic mechanisms may provide further therapeutic possibilities, especially for elderly and obese patients who may lack UCP1 in adipose tissue.
BAT activation negatively correlates with body weight (18). An early study reported that the metabolic response to NE infusion and the adaptive thermogenesis are attenuated in obese patients with family history (67). It suggests that obese patients find it more difficult to lose weight through BAT thermogenesis. In recent years, it has been documented that thermogenic fat can be induced in WAT in both mice and humans. It opens up an intriguing treatment intervention, especially for obese patients with a large surplus of WAT (68). In addition to physiological stimuli such as chronic cold exposure, pharmacological treatment including PPARγ agonist and β-adrenergic stimulation contribute to “beiging”. Synthetic PPARγ ligands thiazolidinedione drugs (TZDs) has been shown to induce “beiging” by prolonging the half-life of PRDM16 (69). One of the natural molecules, flavonoids, induce “beiging” by activating AMP-activated protein kinase (AMPK)-PGC1α/Sirtuin 1 (SIRT1) and PPARγ signaling pathways. Furthermore, they also promote the differentiation of brown preadipocytes and inhibit their apoptosis (70, 71). In addition, there are a subset of common natural compounds which promote “beiging” in WAT (Table 2). For instance, capsaicin and dietary fish oil induce “beiging” in WAT via activation of transient receptor potential vanilloid 1 (TRPV1) (75, 76), which arouse interests about the effect to attenuate fat accumulation for someone who prefers to take chili and fish oil. As a popular beverage, green and black tea contains catechins and theaflavins that promote beige adipogenesis by activating AMPK (78, 79). Other regulatory factors which are involved in beige fat development include fibroblast growth factor 21 (FGF21), bone morphogenetic protein 7 (BMP7), irisin, and cyclooxygenase 2 (COX-2) (84, 85). Specifically, FGF21 plays a physiologic role in recruiting beige fat cells in WAT depots by increasing transcription factor PGC1α level (86). Moreover, muscle-derived irisin, which is regulated by PGC1α, stimulates beige adipogenesis and exercise induces the release of irisin into the blood to drive the process of “beiging” (87).
Along with several special molecules and regulators, physical exercise also contributes to “beiging”. In mice, exercise training promotes the mitochondrial synthesis, activity, and glucose uptake in subcutaneous white adipocytes, which are dependent on endothelial nitric oxide synthase (eNOS) (88). Furthermore, transplanting subcutaneous WAT of the exercised mice into the sedentary mice obviously improved the glucose uptake of skeletal muscle and the overall metabolic balance (89). Importantly, the circulating adipokine concentration and their expression in adipose tissue is altered in both human and mouse after exercises (89). These adipokines may play a prominent role in the dramatic metabolic effects which need to be further identified. In humans, the origin of beige fat is still controversial and whether “beiging” increases energy expenditure remains unclear. The capacity of “beiging” in humans specifically needs further investigation (68). Of note, improved flow cytometry is available to sort and quantify UCP1+ beige adipocytes after various interventions and as an effective method (90). Here, we focus on a few novel perspectives on the mechanisms of “beiging”, which have been discovered so far (Figure 2).
Figure 2 Emerging mechanisms involving in “beiging” of WAT. ADIPOR, adiponectin receptors; ALK, anaplastic lymphoma kinase; AR, adrenergic receptor; CGI-58, comparative gene identification-58; Clstn3β, calsyntenin 3β; CXCL14, C-X-C motif chemokine ligand-14; EODF, every-other-day fasting; ER, endoplasmic reticulum; IRE1α, inositol-requiring enzyme 1α; PPARγ, peroxisome proliferation-activated receptor gamma; PRDM16, protein PR domain containing 16; TH, tyrosine hydroxylase; Zfp423, C2H2 zinc-finger protein. The content in the boxes corresponds to the relevant mechanism and the asterisk indicates the progress of “beiging” does not involve β-adrenergic pathway. The signs (+/-) in red represent the activation/inhibition status while the arrows in red mean the elevating level of related substance. Figure created using BioRender (https://biorender.com/).
Roscovitine is an inhibitor of cyclin-dependent kinase (CDK) and prevents S273 phosphorylation of PPARγ, which in turn induces beige adipocyte development, increases energy expenditure, and mitigates insulin resistance (91). These adipocytes express UCP1 and are distinct from canonical beige adipocytes both in morphology and mRNA signature. For instance, Cdsn, Rhbg, and Gpx8 are enriched in roscovitine-induced adipocytes, which are paucilocular and different from multilocular beige adipocyte. Besides, they are similar to the beige adipocytes induced by rosiglitazone. This is probably because these two drugs share the same mechanism of PPARγ stimulation rather than the β-adrenergic pathway. These phenomena indicate that there are various subtypes of thermogenic adipocytes. They have been found to include glycolytic beige adipocyte and UCP1+ adipocytes upon transforming from white adipocytes or differentiation from multiple developmental origins (19, 20). Future studies will possibly focus on the mechanism of the development of different UCP1+ adipocytes, which may contribute to the adaptive thermogenesis.
EODF, as a form of intermittent fasting, has been reported to initiate “beiging” in the subcutaneous inguinal WAT by reshaping gut microbiota and contributing to metabolic homeostasis (92). Long-term EODF leads to a gradual change in the gut microbiota composition, which results in an augment of fermentation products such as acetate and lactate. They act as “beiging” agonists to dramatically upregulate the expression of UCP1 and stimulate “beiging” in WAT independent on β3-AR and FGF21 signaling pathway. Intriguingly, EODF diet does not influence cumulative food intake, which reveals the effect of weight loss is the increased energy expenditure. Conceivably, for people with poor compliance of EODF regimen, further exploration on the mechanisms of EODF-induced “beiging” is particularly conducive to capturing new therapeutic methods to combat obesity and metabolic diseases.
However, the effect of microbiota depletion on “beiging” is controversial. Some studies show microbiota depletion stimulates the development of beige fat and gains metabolic improvements whereas another study presents negative effects that microbiota depletion reduces the process of “beiging” (93, 94). Although the results are different, the fact is that microbiota-fat signaling pathway may be correlated to beige fat recruitment. Nevertheless, gut microbiota exist in the body, so that the applications of microbiota depletion in humans would be limited in clinical studies.
As a transcriptional regulator of white adipogenesis, C2H2 zinc-finger protein Zfp423 plays a critical role in maintaining the phenotype of WAT (95). A 2016 paper from the Pfeifer Lab indicates that in the progress of BAT whitening, a reversible process characterized by mitochondrial dysfunction, lipid droplet accumulation, and decreased vascularity (96), Zfp423 expression increases and effectively inhibits the expression of some thermogenic genes including Ucp1 (97). There also shows a negative correlation between Zfp423 and the activity of Ebf2 and PRDM16, which drive the activation of thermogenic program and keep the identity of brown and/or beige fat (98). In mice lacking Zfp423, β-adrenergic stimulation strengthens the effect of Zfp423 deletion to transform white adipocytes to beige adipocytes in inguinal and gonadal fat, leading to the reverse of diet-induced obesity and glucose metabolism disorders. From an evolutionary viewpoint, intervention on Zfp423 highlights the potential of bi-directional interconversion between white and beige adipocytes. It should be noted that chronic cold exposure induces beige adipocyte formation while subsequent heat adaptation converts them into the same cells as white adipocytes in morphology and gene phenotype. Moreover, these specific adipocytes can transform into beige adipocytes once again after further cold stimulus, which indicates a promising adaptive plasticity of white adipocytes (99, 100). In sum, future efforts focused on certain determination factors which would regulate the bi-directional differentiation of white-beige adipocytes should be made to find new potential targets for ameliorating obesity and sustaining metabolic balance.
An adipokine, chemokine C-X-C motif chemokine ligand-14 (CXCL14), has been identified to be released by activated brown adipocytes, which mediates a brown fat-macrophage communication and drives the adaptive thermogenesis by promoting both BAT activation and “beiging” in WAT (101). This positive feedback effect depends on CXCL14 induced activation of type 2 cytokine signaling and recruitment of macrophages, especially M2 phenotype, in WAT. However, the specific mechanisms by which M2 macrophages’ recruitment improves the adaptive thermogenesis remain controversial. One of the underlying causes was thought to be the secretion of catecholamines triggered by the enhanced activation of adipose tissue macrophages (ATMs) (102), although this has since been refuted (103).
Additionally, the most conserved endoplasmic reticulum (ER) stress sensor inositol-requiring enzyme 1α (IRE1α) governs M1-M2 macrophage polarization and drives obesity by influencing BAT activity and “beiging” in WAT (104). Some obesogenic factors, such as overnutrition, activates IRE1α in ATMs and increases ER stress, which disequilibrates the M1-M2 polarization and decreases M2 recruitment. Nevertheless, IRE1α abrogation reverses the imbalance and promotes M2 activation of ATMs, leading to “beiging” in WAT and augmentation of energy output. Overnutrition and obesity also increase the content of adipose sphingolipids, whose intermediates include ceramide and its metabolites (105). Whole-body and fat-specific inhibition of ceramide synthesis induces “beiging” and M2 macrophage recruitment preferentially in subcutaneous WAT, consistently with an improvement in thermogenic gene expression, mitochondrial function, and energy expenditure. Physiologically, two subtypes of adiponectin receptors, ADIPOR1 and ADIPOR2, possess intrinsic activity of ceramidase and can hydrolyze ceramide (106), which may cause similar metabolic effects of ceramide synthesis inhibition. Hence, it reveals that M2 macrophage polarization and recruitment orchestrating adaptive thermogenesis have potential therapeutic benefits on obesity.
A recent study has indicated that there is still general thermogenic capacity without cold stimulation in mice when BAT lipolysis is inhibited by tissue-specific knockout of comparative gene identification-58 (CGI-58), which is a vital lipolysis catalyst (107). The mechanism to compensate for this defect includes a development of beige fat, which depends strongly on the sympathetic innervation through elevating the level of tyrosine hydroxylase (TH), promoting the production of dopamine and β3-ARs in WAT. Because of the recruited BAT and beige fat, most of the circulating nutrients, such as glucose, flow into these thermogenic tissues for heat production while fewer nutrients enter other tissues for pathological accumulation. Moreover, the CGI-58 knockout mice in the fed generally show a higher body temperature and energy output during cold exposure than the littermates. Thus, selective inhibition of BAT lipolysis by CGI-58 ablation contributes to beige fat development through an improved sympathetic innervation in WAT, which results in a steady-state metabolism.
Dense sympathetic arborizations in WAT have been directly observed under microscope by the volume fluorescence-imaging technique. They are embedded in WAT and closely positioned to most adipocytes, which are notably indispensable for “beiging” induced by cold acclimation (108). In mice with an ablation of sympathetic arborization, it failed to increase the expression of thermogenic genes and induce the formation of multilocular adipocytes in inguinal WAT (iWAT). Furthermore, catecholamines released from sympathetic arborization and β3-ARs play a critical role on the progress of “beiging” during cold exposure. Of note, a recent discovery from Spiegelman’s group has identified calsyntenin 3β (Clstn3β), which is a thermogenic fat-specific protein located in the endoplasmic reticulum. It enhances the secretion of S100b in response to cold, which promotes sympathetic innervation and thereby improves non-shivering thermogenesis (109).
Furthermore, another factor, anaplastic lymphoma kinase (ALK), has been identified to be a thinness gene candidate (110), which is derived from hypothalamic paraventricular nucleus. Its deletion results in an increase in NE level and enhancement in lipolysis both in BAT and WAT (involvement of sympathetic innervation). Consistently with the effect of NE-induced “beiging” (111), the thermogenic and mitochondrial gene expression is also substantially upregulated in WAT. Overall, ALK is a negative regulator of “beiging” and lipolysis via sympathetic tone. Its inhibition could be a promising strategy for maintaining energy homeostasis and therefore resisting weight gain.
Classical non-shivering thermogenesis, which is UCP1-dependent, contributes to energy dissipation by boosting mitochondria-mediated disposal of glucose and lipids, which benefits obesity and its related complications such as diabetes and hyperlipidemia. As a main fuel source for heat generation, lipids also exert regulatory effects on mitochondrial dynamics and bioenergetics, thermogenic gene expression, thermogenic adipocytes differentiation, signal transduction, and interorgan communication (22), thereby, a comprehensive understanding of the diversity of lipid functions in thermogenic fat is necessary to be further explored for the treatment of human obesity.
The most direct way to elevate thermogenic effect is to potentiate the quality and quantity of active thermogenic fat. As previously known, cold exposure has an intense activating effect on brown and beige fat. In a human study, daily cold exposure (10°C; 2h/d) for four consecutive weeks promotes BAT oxidative capacity and attenuates shivering intensity and thermogenesis in skeletal muscles, which demonstrates a reciprocal effect between BAT and skeletal muscle and an enhanced contribution of BAT activation to cold-induced heat production (112). However, chronic cold adaptation challenges the clinical feasibility to engage in obese patients because of the general preference for thermal comfort. Selective β3-AR agonists also activate thermogenic fat and promote “beiging”, yet are concomitant with poor bioavailability, not only because of less expression and lower efficacy of β3-AR agonists in human white adipocytes, but also due to some serious side effects, especially on the cardiovascular system (113). In point of fact, human brown adipocytes primarily express β2-AR, which co-localize with UCP1 and present a novel pharmacological target to stimulate human BAT (114). A similar situation is for the PPARγ agonists, as pioglitazone also causes various side effects such as exacerbations on congestive heart failure, bladder cancer, and bone fractures (115). One of the artificial FGF21 analogs, LY2405319 (LY), has been trialed in patients with obesity and type 2 diabetes, and results in significant improvements in dyslipidemia, body weight, and fasting insulin (116, 117). However, bone loss is a safety concern of FGF21 analog, which has been reported in rodents, and its potential effect on atherogenesis in humans may be worth considering (118).
Thermogenic fat recruitment could be another approach of therapeutic interventions to treat obesity. Preadipocytes “beiging” in WAT is a pivotal recruitment source of thermogenic fat. As mentioned above, some pharmaceuticals and common natural compounds promote the progress of “beiging”, and several regulators influence “beiging” in WAT involving the mechanisms of sympathetic innervation, M2 macrophages recruitment, gut microbiota remodeling, etc. All these show “beiging” in WAT is a holistic process with interorgan communication and provides an extensive research field of bi-directional differentiation of white and thermogenic adipocytes. Moreover, initial mesodermal induction of human pluripotent stem cells (hiPSCs) activate the differentiation of adipocytes with a thermogenic phenotype through a distinct protocol (18). It is valuable to provide an unlimited cell source focusing on human thermogenic adipocyte recruitment, which is still under investigation (18, 119). Bone marrow-derived cells (BMCs) could infiltrate into iWAT through circulation and differentiate to white adipocytes under high fat diet (HFD) feeding or UCP1+ thermogenic adipocytes following β3-AR activation (120). Hence, BMCs act as nonresident progenitors contributing to the adipose tissue thermogenesis. In addition to hiPSCs and BMCs, adipocyte precursors have drawn attention to develop intracellular gene-editing methods for drug screening and transplantable therapeutics (121). As is expected, BAT transplantation for 2-3 months strikingly ameliorates HFD-induced insulin resistance and hyperlipidemia, lowers body weight, and improves the whole-body energy balance following “dose effect” in mice (122). Moreover, BAT transplantation enhances the gene expression, which is related to FFA oxidation in endogenous BAT, and may potentiate the inherent adaptive thermogenesis (123). Remarkably, implantation of human beige adipocytes into recipient mice generates a similar beneficial metabolic response, enlightening that it may become a feasible intervention for diabetes and obesity treatment (124). CRISPR-engineered technology has been used on human white preadipocytes to create brown-like cells, which are endogenously activated of UCP1. Transplantation of these cells improves metabolism in diet-induced obese mice and also activates endogenous BAT (125). Nevertheless, there are fewer thermogenic genes in the transplanted BAT. Likely owing to the absence of propriate sympathetic stimulation, and the ubiquitous transplantation rejection, BAT-related transplantation needs to be focused on in further research (123). Taken together, although drugs that activate thermogenic fat have no widespread application due to their side effects and insufficient feasibility, the induced differentiation of pluripotent stem cells and adipose precursors from various cell linages into thermogenic adipocytes and the transplantation of thermogenic fat as emerging research field could hold promise as a new class of therapeutics.
Another point worth noting is that there may be multiple subtypes of thermogenic adipocytes, which originate from different precursor cells, are regulated by distinctive molecular switches, and have unique biological roles besides thermogenesis. UCP1 is not indispensable because cold sensitivity can be compensated by PRDM16 activation and recruitment of beige adipocytes with UCP1-independent thermogenic mechanisms. While PRDM16 is not always the molecular switch of thermogenic adipocytes, Gabpα also drives myogenic beige fat differentiation which burns mainly sugar (19). These show the thermogenic adipose cell heterogeneity of developmental origin and regulation of cellular metabolism with canonical or noncanonical mechanisms.
In sum, thermogenic fat draws considerable attention owing to the contribution to systemic energy homeostasis through dissipating energy into heat. More studies have shown various subtypes of thermogenic adipocytes, different mechanisms of UCP1-independent thermogenesis, and “beiging” with interorgan communication, which enrich the knowledge of thermogenic fat and raise the possibility for clinical approaches for the management of obesity and related complications.
ZW and YC wrote the manuscript. XY and YC edited the manuscript. All authors contributed to the article and approved the submitted version.
This study was supported by the National Natural Science Foundation of China (82070859 to YC, ZW) and a grant from Tongji Hospital in Huazhong University of Science and Technology (Grant No. 2201103295 to YC).
The authors declare that the research was conducted in the absence of any commercial or financial relationships that could be construed as a potential conflict of interest.
1. Poher AL, Altirriba J, Veyrat-Durebex C, Rohner-Jeanrenaud F, et al. Brown Adipose Tissue Activity as a Target for the Treatment of Obesity/Insulin Resistance. Front Physiol (2015) 6:4. doi: 10.3389/fphys.2015.00004
2. Heilbronn LK, de Jonge L, Frisard MI, DeLany JP, Larson-Meyer DE, Rood J, et al. Effect of 6-Month Calorie Restriction on Biomarkers of Longevity, Metabolic Adaptation, and Oxidative Stress in Overweight Individuals: A Randomized Controlled Trial. JAMA (2006) 295(13):1539–48. doi: 10.1001/jama.295.13.1539
3. Fernandez-Verdejo R, Marlatt R, Ravussin KL, Galgani E, et al. Contribution of Brown Adipose Tissue to Human Energy Metabolism. Mol Aspects Med (2019) 68:82–9. doi: 10.1016/j.mam.2019.07.003
4. Cypess AM, White AP, Vernochet C, Schulz TJ, Xue R, Sass CA, et al. Anatomical Localization, Gene Expression Profiling and Functional Characterization of Adult Human Neck Brown Fat. Nat Med (2013) 19(5):635–9. doi: 10.1038/nm.3112
5. Cypess AM, Lehman S, Williams G, Tal I, Rodman D, Goldfine AB, et al. Identification and Importance of Brown Adipose Tissue in Adult Humans. N Engl J Med (2009) 360(15):1509–17. doi: 10.1056/NEJMoa0810780
6. Saito M, Okamatsu-Ogura Y, Matsushita M, Watanabe K, Yoneshiro T, Nio-Kobayashi J, et al. High Incidence of Metabolically Active Brown Adipose Tissue in Healthy Adult Humans: Effects of Cold Exposure and Adiposity. Diabetes (2009) 58(7):1526–31. doi: 10.2337/db09-0530
7. Cohen P, Spiegelman BM. Brown and Beige Fat: Molecular Parts of a Thermogenic Machine. Diabetes (2015) 64(7):2346–51. doi: 10.2337/db15-0318
8. Kajimura S, Spiegelman BM, Seale P. Brown and Beige Fat: Physiological Roles Beyond Heat Generation. Cell Metab (2015) 22(4):546–59. doi: 10.1016/j.cmet.2015.09.007
9. Wang WS, Seale P. Control of Brown and Beige Fat Development. Nat Rev Mol Cell Biol (2016) 17(11):691–702. doi: 10.1038/nrm.2016.96
10. Nedergaard J, Cannon B. The Changed Metabolic World With Human Brown Adipose Tissue: Therapeutic Visions. Cell Metab (2010) 11(4):268–72. doi: 10.1016/j.cmet.2010.03.007
11. Concha F, Prado G, Quezada J, Ramirez A, Bravo N, Flores C, et al. Nutritional and non-Nutritional Agents That Stimulate White Adipose Tissue Browning. Rev Endocrine Metab Disord (2019) 20(2):161–71. doi: 10.1007/s11154-019-09495-y
12. Leitner BP, Huang S, Brychta RJ, Duckworth CJ, Baskin AS, McGehee S, et al. Mapping of Human Brown Adipose Tissue in Lean and Obese Young Men. Proc Natl Acad Sci USA (2017) 114(32):8649–54. doi: 10.1073/pnas.1705287114
13. Shinoda K, Luijten IH, Hasegawa Y, Hong H, Sonne SB, Kim M, et al. Genetic and Functional Characterization of Clonally Derived Adult Human Brown Adipocytes. Nat Med (2015) 21(4):389–94. doi: 10.1038/nm.3819
14. Sharp LZ, Shinoda K, Ohno H, Scheel DW, Tomoda E, Ruiz L, et al. Human BAT Possesses Molecular Signatures That Resemble Beige/Brite Cells. PloS One (2012) 7(11):e49452. doi: 10.1371/journal.pone.0049452
15. Sebo ZL, Rendina-Ruedy E, Ables GP, Lindskog DM, Rodeheffer MS, Fazeli PK, et al. Bone Marrow Adiposity: Basic and Clinical Implications. Endocrine Rev (2019) 40(5):1187–206. doi: 10.1210/er.2018-00138
16. Wu J, Bostrom P, Sparks LM, Ye L, Choi JH, Giang AH, et al. Beige Adipocytes Are a Distinct Type of Thermogenic Fat Cell in Mouse and Human. Cell (2012) 150(2):366–76. doi: 10.1016/j.cell.2012.05.016
17. Zhang X, Wang X, Yin H, Zhang L, Feng A, Zhang QX, et al. Functional Inactivation of Mast Cells Enhances Subcutaneous Adipose Tissue Browning in Mice. Cell Rep (2019) 28(3):792–803 e4. doi: 10.1016/j.celrep.2019.06.044
18. Guenantin AC, Briand N, Capel E, Dumont F, Morichon R, Provost C, et al. Functional Human Beige Adipocytes From Induced Pluripotent Stem Cells. Diabetes (2017) 66(6):1470–8. doi: 10.2337/db16-1107
19. Chen Y, Ikeda K, Yoneshiro T, Scaramozza A, Tajima K, Wang Q, et al. Thermal Stress Induces Glycolytic Beige Fat Formation via a Myogenic State. Nature (2018) 565(7738):180–5. doi: 10.1038/s41586-018-0801-z
20. Pan R, Zhu X, Maretich P, Chen Y. Combating Obesity With Thermogenic Fat: Current Challenges and Advancements. Front Endocrinol (2020) 11:185. doi: 10.3389/fendo.2020.00185
21. Sun WF, Wolfrum C. Fat Cells With a Sweet Tooth. Nature (2019) 565(7738):167–8. doi: 10.1038/d41586-018-07739-6
22. Park H, He A, Lodhi IJ. Lipid Regulators of Thermogenic Fat Activation. Trends Endocrinol Metab (2019) 30(10):710–23. doi: 10.1016/j.tem.2019.07.020
23. Sun W, Dong H, Balaz M, Slyper M, Drokhlyansky E, Colleluori G, et al. snRNA-Seq Reveals a Subpopulation of Adipocytes That Regulates Thermogenesis. Nature (2020) 587(7832):98–102. doi: 10.1038/s41586-020-2856-x
24. Ouellet V, Routhier-Labadie A, Bellemare W, Lakhal-Chaieb L, Turcotte E, Carpentier AC, et al. Outdoor Temperature, Age, Sex, Body Mass Index, and Diabetic Status Determine the Prevalence, Mass, and Glucose-Uptake Activity of 18F-FDG-Detected BAT in Humans. J Clin Endocrinol Metab (2011) 96(1):192–9. doi: 10.1210/jc.2010-0989
25. Lee P, Greenfield JR, Ho KK, Fulham MJ. A Critical Appraisal of the Prevalence and Metabolic Significance of Brown Adipose Tissue in Adult Humans. Am J Physiol Endocrinol Metab (2010) 299(4):E601–6. doi: 10.1152/ajpendo.00298.2010
26. Saely CH, Geiger K, Drexel H. Brown Versus White Adipose Tissue: A Mini-Review. Gerontology (2012) 58(1):15–23. doi: 10.1159/000321319
27. Soumano K, Desbiens S, Rabelo R, Bakopanos E, Camirand A, Silva JE, et al. Glucocorticoids Inhibit the Transcriptional Response of the Uncoupling Protein-1 Gene to Adrenergic Stimulation in a Brown Adipose Cell Line. Mol Cell Endocrinol (2000) 165(1-2):7–15. doi: 10.1016/S0303-7207(00)00276-8
28. Yanase T, Fan W, Kyoya K, Min L, Takayanagi R, Kato S, et al. Androgens and Metabolic Syndrome: Lessons From Androgen Receptor Knock Out (ARKO) Mice. J Steroid Biochem Mol Biol (2008) 109(3-5):254–7. doi: 10.1016/j.jsbmb.2008.03.017
29. Rodriguez-Cuenca S, Monjo M, Frontera M, Gianotti M, Proenza AM, Roca P, et al. Sex Steroid Receptor Expression Profile in Brown Adipose Tissue. Effects Formonal Status Cell Physiol Biochem (2007) 20(6):877–86. doi: 10.1159/000110448
30. Zhou Z, Moore TM, Drew BG, Ribas V, Wanagat J, Civelek M, et al. Estrogen Receptor Alpha Controls Metabolism in White and Brown Adipocytes by Regulating Polg1 and Mitochondrial Remodeling. Sci Trans Med (2020) 12(555):eaax8096. doi: 10.2337/db20-295-OR
31. Quevedo S, Roca P, Picó C, Palou A, et al. Sex-Associated Differences in Cold-Induced UCP1 Synthesis in Rodent Brown Adipose Tissue. Pflugers Arch (1998) 436(5):689–95. doi: 10.1007/s004240050690
32. Seale P, Kajimura S, Yang W, Chin S, Rohas LM, Uldry M, et al. Transcriptional Control of Brown Fat Determination by PRDM16. Cell Metab (2007) 6(1):38–54. doi: 10.1016/j.cmet.2007.06.001
33. Seale P, Conroe HM, Estall J, Kajimura S, Frontini A, Ishibashi J, et al. Prdm16 Determines the Thermogenic Program of Subcutaneous White Adipose Tissue in Mice. J Clin Invest (2011) 121(1):96–105. doi: 10.1172/JCI44271
34. Seale P, Bjork B, Yang W, Kajimura S, Chin S, Kuang S, et al. PRDM16 Controls a Brown Fat/Skeletal Muscle Switch. Nature (2008) 454(7207):961–7. doi: 10.1038/nature07182
35. Cohen P, Levy JD, Zhang Y, Frontini A, Kolodin DP, Svensson KJ, et al. Ablation of PRDM16 and Beige Adipose Causes Metabolic Dysfunction and a Subcutaneous to Visceral Fat Switch. Cell (2014) 156(1-2):304–16. doi: 10.1016/j.cell.2013.12.021
36. Yin H, Pasut A, Soleimani Vahab D, Bentzinger CF, Antoun G, Thorn S, et al. MicroRNA-133 Controls Brown Adipose Determination in Skeletal Muscle Satellite Cells by Targeting Prdm16. Cell Metab (2013) 17(2):210–24. doi: 10.1016/j.cmet.2013.01.004
37. Hyppönen E, Mulugeta A, Zhou A, Santhanakrishnan VK. A Data-Driven Approach for Studying the Role of Body Mass in Multiple Diseases: A Phenome-Wide Registry-Based Case-Control Study in the UK Biobank. Lancet Digital Health (2019) 1(3):e116–26. doi: 10.1016/S2589-7500(19)30028-7
38. Matsushita M, Yoneshiro T, Aita S, Kameya T, Sugie H, Saito M, et al. Impact of Brown Adipose Tissue on Body Fatness and Glucose Metabolism in Healthy Humans. Int J Obes (Lond) (2014) 38(6):812–7. doi: 10.1038/ijo.2013.206
39. Gaudry MJ, Keuper M, Jastroch M. Molecular Evolution of Thermogenic Uncoupling Protein 1 and Implications for Medical Intervention of Human Disease. Mol Aspects Med (2019) 68:6–17. doi: 10.1016/j.mam.2019.06.006
40. White JD, Dewal RS, Stanford KI. The Beneficial Effects of Brown Adipose Tissue Transplantation. Mol Aspects Med (2019) 68:74–81. doi: 10.1016/j.mam.2019.06.004
41. Lynes MD, Leiria LO, Lundh M, Bartelt A, Shamsi D, Huang TL, et al. The Cold-Induced Lipokine 12,13-diHOME Promotes Fatty Acid Transport Into Brown Adipose Tissue. Nat Med (2017) 23(5):631–7. doi: 10.1038/nm.4297
42. Pinckard KM, Shettigar VK, Wright KR, Abay E, Baer LA, Vidal P, et al. A Novel Endocrine Role for the BAT-Released Lipokine 12,13-diHOME to Mediate Cardiac Function. Circulation (2021) 143(2):145–59. doi: 10.1161/CIRCULATIONAHA.120.049813
43. Becher T, Palanisamy S, Kramer DJ, Eljalby M, Marx SJ, Wibmer AG, et al. Brown Adipose Tissue Is Associated With Cardiometabolic Health. Nat Med (2021) 27(1):58–65. doi: 10.1038/s41591-020-1126-7
44. Klepac K, Georgiadi A, Tschop M, Herzig S. The Role of Brown and Beige Adipose Tissue in Glycaemic Control. Mol Aspects Med (2019) 68:90–100. doi: 10.1016/j.mam.2019.07.001
45. Chung KJ, Chatzigeorgiou A, Economopoulou M, Garcia-Martin R, Alexaki VI, Mitroulis I, et al. A Self-Sustained Loop of Inflammation-Driven Inhibition of Beige Adipogenesis in Obesity. Nat Immunol (2017) 18(6):654–64. doi: 10.1038/ni.3728
46. Man K, Bowman C, Braverman KN, Escalante V, Tian Y, Bisanz JE, et al. A Thermogenic Fat-Epithelium Cell Axis Regulates Intestinal Disease Tolerance. Proc Natl Acad Sci USA (2020) 117(50):32029–37. doi: 10.1073/pnas.2012003117
47. Tsoli M, Moore M, Burg D, Painter A, Taylor R, Lockie SH, et al. Activation of Thermogenesis in Brown Adipose Tissue and Dysregulated Lipid Metabolism Associated With Cancer Cachexia in Mice. Cancer Res (2012) 72(17):4372–82. doi: 10.1158/0008-5472.CAN-11-3536
48. Petruzzelli M, Schweiger M, Schreiber R, Campos-Olivas R, Tsoli M, Allen J, et al. A Switch From White to Brown Fat Increases Energy Expenditure in Cancer-Associated Cachexia. Cell Metab (2014) 20(3):433–47. doi: 10.1016/j.cmet.2014.06.011
49. Sun W, Dong H, Becker AS, Dapito DH, Modica S, Grandl G, et al. Cold-Induced Epigenetic Programming of the Sperm Enhances Brown Adipose Tissue Activity in the Offspring. Nat Med (2018) 24(9):1372–83. doi: 10.1038/s41591-018-0102-y
50. Lizcano F. The Beige Adipocyte as a Therapy for Metabolic Diseases. Int J Mol Sci (2019) 20(20):5058. doi: 10.3390/ijms20205058
51. Chouchani ET, Kazak L, Spiegelman BM. New Advances in Adaptive Thermogenesis: UCP1 and Beyond. Cell Metab (2019) 29(1):27–37. doi: 10.1016/j.cmet.2018.11.002
52. Shabalina IG, Jacobsson A, Cannon B, Nedergaard J. Native UCP1 Displays Simple Competitive Kinetics Between the Regulators Purine Nucleotides and Fatty Acids. J Biol Chem (2004) 279(37):38236–48. doi: 10.1074/jbc.M402375200
53. Fedorenko A, Lishko PV, Kirichok Y. Mechanism of Fatty-Acid-Dependent UCP1 Uncoupling in Brown Fat Mitochondria. Cell (2012) 151(2):400–13. doi: 10.1016/j.cell.2012.09.010
54. Kajimura S, Saito M. A New Era in Brown Adipose Tissue Biology: Molecular Control of Brown Fat Development and Energy Homeostasis. Annu Rev Physiol (2014) 76:225–49. doi: 10.1146/annurev-physiol-021113-170252
55. Mitchell P. Vectorial Chemistry And Molecular Mechanics Of Chemiosmotic Coupling - Power Transmission By Proticity. Biochem Soc Trans (1976) 4(3):399–430. doi: 10.1042/bst0040399
56. Leiria LO, Wang CH, Lynes MD, Yang K, Shamsi F, Sato M, et al. 12-Lipoxygenase Regulates Cold Adaptation and Glucose Metabolism by Producing the Omega-3 Lipid 12-HEPE From Brown Fat. Cell Metab (2019) 30(4):768–83.e7. doi: 10.1016/j.cmet.2019.07.001
57. Heine M, Fischer AW, Schlein C, Jung C, Straub LG, Gottschling K, et al. Lipolysis Triggers a Systemic Insulin Response Essential for Efficient Energy Replenishment of Activated Brown Adipose Tissue in Mice. Cell Metab (2018) 28(4):644–55.e4. doi: 10.1016/j.cmet.2018.06.020
58. Simcox J, Geoghegan G, Maschek JA, Bensard CL, Pasquali M, Miao R, et al. Global Analysis of Plasma Lipids Identifies Liver-Derived Acylcarnitines as a Fuel Source for Brown Fat Thermogenesis. Cell Metab (2017) 26(3):509–522 e6. doi: 10.1016/j.cmet.2017.08.006
59. Worthmann A, John C, Ruhlemann MC, Baguhl M, Heinsen FA, Schaltenberg N, et al. Cold-Induced Conversion of Cholesterol to Bile Acids in Mice Shapes the Gut Microbiome and Promotes Adaptive Thermogenesis. Nat Med (2017) 23(7):839–49. doi: 10.1038/nm.4357
60. Lee J, Choi J, Aja S, Scafidi S, Wolfgang MJ. Loss of Adipose Fatty Acid Oxidation Does Not Potentiate Obesity at Thermoneutrality. Cell Rep (2016) 14(6):1308–16. doi: 10.1016/j.celrep.2016.01.029
61. Ikeda K, Kang Q, Yoneshiro T, Camporez JP, Maki H, Homma M, et al. UCP1-Independent Signaling Involving SERCA2b-Mediated Calcium Cycling Regulates Beige Fat Thermogenesis and Systemic Glucose Homeostasis. Nat Med (2017) 23(12):1454–65. doi: 10.1038/nm.4429
62. Kazak L, Chouchani Edward T, Jedrychowski Mark P, Erickson Brian K, Shinoda K, Cohen P, et al. A Creatine-Driven Substrate Cycle Enhances Energy Expenditure and Thermogenesis in Beige Fat. Cell (2015) 163(3):643–55. doi: 10.1016/j.cell.2015.09.035
63. Chouchani ET, Kajimura S. Metabolic Adaptation and Maladaptation in Adipose Tissue. Nat Metab (2019) 1(2):189–200. doi: 10.1038/s42255-018-0021-8
64. Berg F, Gustafson U, Andersson L. The Uncoupling Protein 1 Gene (UCP1) Is Disrupted in the Pig Lineage: A Genetic Explanation for Poor Thermoregulation in Piglets. PloS Genet (2006) 2(8):e129. doi: 10.1371/journal.pgen.0020129
65. Sun Y, Rahbani JF, Jedrychowski MP, Riley CL, Vidoni S, Bogoslavski D, et al. Mitochondrial TNAP Controls Thermogenesis by Hydrolysis of Phosphocreatine. Nature (2021) 593(7860):580–5. doi: 10.1038/s41586-021-03533-z
66. Bertholet AM, Kazak L, Chouchani ET, Bogaczynska MG, Paranjpe I, Wainwright GL, et al. Mitochondrial Patch Clamp of Beige Adipocytes Reveals UCP1-Positive and UCP1-Negative Cells Both Exhibiting Futile Creatine Cycling. Cell Metab (2017) 25(4):811–22.e4. doi: 10.1016/j.cmet.2017.03.002
67. Jung RT, Shetty PS, James WPT, Barrand MA, Callingham BA. Reduced Thermogenesis In Obesity. Nature (1979) 279(5711):322–3. doi: 10.1038/279322a0
68. Herz CT, Kiefer FW. Adipose Tissue Browning in Mice and Humans. J Endocrinol (2019) 241(3):R97–R109. doi: 10.1530/JOE-18-0598
69. Ohno H, Shinoda K, Spiegelman BM, Kajimura S, et al. PPARgamma Agonists Induce a White-to-Brown Fat Conversion Through Stabilization of PRDM16 Protein. Cell Metab (2012) 15(3):395–404. doi: 10.1016/j.cmet.2012.01.019
70. Zhang XJ, Li X, Fang H, Guo FJ, Li F, Chen AM, et al. Flavonoids as Inducers of White Adipose Tissue Browning and Thermogenesis: Signalling Pathways and Molecular Triggers. Nutr Metab (2019) 16:47. doi: 10.1186/s12986-019-0370-7
71. Qiang L, Wang L, Kon N, Zhao W, Lee S, Zhang Y, et al. Brown Remodeling of White Adipose Tissue by SirT1-Dependent Deacetylation of Ppargamma. Cell (2012) 150(3):620–32. doi: 10.1016/j.cell.2012.06.027
72. Wang S, Wang X, Ye Z, Xu C, Zhang M, Ruan B, et al. Curcumin Promotes Browning of White Adipose Tissue in a Norepinephrine-Dependent Way. Biochem Biophys Res Commun (2015) 466(2):247–53. doi: 10.1016/j.bbrc.2015.09.018
73. Wang S, Liang X, Yang Q, Fu X, Rogers CJ, Zhu M, et al. Resveratrol Induces Brown-Like Adipocyte Formation in White Fat Through Activation of AMP-Activated Protein Kinase (AMPK) Alpha1. Int J Obes (Lond) (2015) 39(6):967–76. doi: 10.1038/ijo.2015.23
74. Zhang Z, Zhang H, Li B, Meng C, Wang J, Zhang Y, et al. Berberine Activates Thermogenesis in White and Brown Adipose Tissue. Nat Commun (2014) 5:5493. doi: 10.1038/ncomms6493
75. Baskaran P, Krishnan V, Ren J, Thyagarajan B. Capsaicin Induces Browning of White Adipose Tissue and Counters Obesity by Activating TRPV1 Channel-Dependent Mechanisms. Br J Pharmacol (2016) 173(15):2369–89. doi: 10.1111/bph.13514
76. Kim M, Goto T, Yu R, Uchida K, Tominaga M, Kano Y, et al. Fish Oil Intake Induces UCP1 Upregulation in Brown and White Adipose Tissue via the Sympathetic Nervous System. Sci Rep (2015) 5:18013. doi: 10.1038/srep18013
77. Jiang CY, Zhai MZ, Yan D, Li D, Li C, Zhang YH, et al. Dietary Menthol-Induced TRPM8 Activation Enhances WAT 1c “Browning 1D” and Ameliorates Diet-Induced Obesity. Oncotarget (2017) 8(43):75114–26. doi: 10.18632/oncotarget.20540
78. Wang S, Huang Y, Xu H, Zhu Q, Lu H, Zhang M, et al. Oxidized Tea Polyphenols Prevent Lipid Accumulation in Liver and Visceral White Adipose Tissue in Rats. Eur J Nutr (2017) 56(6):2037–48. doi: 10.1007/s00394-016-1241-x
79. Yamashita Y, Wang L, Wang L, Tanaka Y, Zhang T, Ashida H, et al. Oolong, Black and Pu-Erh Tea Suppresses Adiposity in Mice via Activation of AMP-Activated Protein Kinase. Food Funct (2014) 5(10):2420–9. doi: 10.1039/C4FO00095A
80. Hong Y, Lin Y, Si Q, Yang L, Dong W, Gu X, et al. Ginsenoside Rb2 Alleviates Obesity by Activation of Brown Fat and Induction of Browning of White Fat. Front Endocrinol (Lausanne) (2019) 10:153. doi: 10.3389/fendo.2019.00153
81. Lee K, Seo YJ, Song JH, Chei S, Lee BY. Ginsenoside Rg1 Promotes Browning by Inducing UCP1 Expression and Mitochondrial Activity in 3T3-L1 and Subcutaneous White Adipocytes. J Ginseng Res (2019) 43(4):589–99. doi: 10.1016/j.jgr.2018.07.005
82. Lone J, Yun JW. Honokiol Exerts Dual Effects on Browning and Apoptosis of Adipocytes. Pharmacol Rep (2017) 69(6):1357–65. doi: 10.1016/j.pharep.2017.06.004
83. Rebello CJ, Greenway FL, Lau FH, Lin Y, Stephens JM, Johnson WD, et al. Naringenin Promotes Thermogenic Gene Expression in Human White Adipose Tissue. Obes (Silver Spring) (2019) 27(1):103–11. doi: 10.1002/oby.22352
84. Lo KA, Sun L. Turning WAT Into BAT: A Review on Regulators Controlling the Browning of White Adipocytes. Biosci Rep (2013) 33(5):e00065. doi: 10.1042/BSR20130046
85. Vegiopoulos A, Mueller-Decker K, Strzoda D, Schmitt I, Chichelnitskiy E, Ostertag A, et al. Cyclooxygenase-2 Controls Energy Homeostasis in Mice by De Novo Recruitment of Brown Adipocytes. Science (2010) 328(5982):1158–61. doi: 10.1126/science.1186034
86. Fisher FM, Kleiner S, Douris N, Fox EC, Mepani RJ, Verdeguer F, et al. FGF21 Regulates PGC-1α and Browning of White Adipose Tissues in Adaptive Thermogenesis. Genes Dev (2012) 26(3):271–81. doi: 10.1101/gad.177857.111
87. Boström P, Wu J, Jedrychowski MP, Korde A, Ye L, Lo JC, et al. A PGC1-α-Dependent Myokine That Drives Brown-Fat-Like Development of White Fat and Thermogenesis. Nature (2012) 481(7382):463–8. doi: 10.1038/nature10777
88. Trevellin E, Scorzeto M, Olivieri M, Granzotto M, Valerio A, Tedesco L, et al. Exercise Training Induces Mitochondrial Biogenesis and Glucose Uptake in Subcutaneous Adipose Tissue Through eNOS-Dependent Mechanisms. Diabetes (2014) 63(8):2800–11. doi: 10.2337/db13-1234
89. Stanford KI, Middelbeek RJW, Goodyear LJ. Exercise Effects on White Adipose Tissue: Beiging and Metabolic Adaptations. Diabetes (2015) 64(7):2361–8. doi: 10.2337/db15-0227
90. Hagberg CE, Li Q, Kutschke M, Bhowmick D, Kiss E, Shabalina IG, et al. Flow Cytometry of Mouse and Human Adipocytes for the Analysis of Browning and Cellular Heterogeneity. Cell Rep (2018) 24(10):2746–56.e5. doi: 10.1016/j.celrep.2018.08.006
91. Wang H, Liu L, Lin JZ, Aprahamian TR, Farmer SR. Browning of White Adipose Tissue With Roscovitine Induces a Distinct Population of UCP1(+) Adipocytes. Cell Metab (2016) 24(6):835–47. doi: 10.1016/j.cmet.2016.10.005
92. Li G, Xie C, Lu S, Nichols RG, Tian Y, Li L, et al. Intermittent Fasting Promotes White Adipose Browning and Decreases Obesity by Shaping the Gut Microbiota. Cell Metab (2017) 26(4):672–85.e4. doi: 10.1016/j.cmet.2017.08.019
93. Suárez-Zamorano N, Fabbiano S, Chevalier C, Stojanović O, Colin DJ, Stevanović A, et al. Microbiota Depletion Promotes Browning of White Adipose Tissue and Reduces Obesity. Nat Med (2015) 21(12):1497–501. doi: 10.1038/nm.3994
94. Li B. Microbiota Depletion Impairs Thermogenesis of Brown Adipose Tissue and Browning of White Adipose Tissue. Cell Rep (2019) 26(10):2720–37.e5. doi: 10.1016/j.celrep.2019.02.015
95. Gupta RK, Arany Z, Seale P, Mepani RJ, Ye L, Conroe HM, et al. Transcriptional Control of Preadipocyte Determination by Zfp423. Nature (2010) 464(7288):619–23. doi: 10.1038/nature08816
96. Shimizu I, Aprahamian T, Kikuchi R, Shimizu A, Papanicolaou KN, MacLauchlan S, et al. Vascular Rarefaction Mediates Whitening of Brown Fat in Obesity. J Clin Invest (2014) 124(5):2099–112. doi: 10.1172/JCI71643
97. Shao M, Ishibashi J, Kusminski CM, Wang QA, Hepler C, Vishvanath L, et al. Zfp423 Maintains White Adipocyte Identity Through Suppression of the Beige Cell Thermogenic Gene Program. Cell Metab (2016) 23(6):1167–84. doi: 10.1016/j.cmet.2016.04.023
98. Wang W, Kissig M, Rajakumari S, Huang L, Lim HW, Won KJ, et al. Ebf2 is a Selective Marker of Brown and Beige Adipogenic Precursor Cells. Proc Natl Acad Sci USA (2014) 111(40):14466–71. doi: 10.1073/pnas.1412685111
99. Rosenwald M, Perdikari A, Rulicke T, Wolfrum C. Bi-Directional Interconversion of Brite and White Adipocytes. Nat Cell Biol (2013) 15(6):659–67. doi: 10.1038/ncb2740
100. Cohen P, Kajimura S. The Cellular and Functional Complexity of Thermogenic Fat. Nat Rev Mol Cell Biol (2021) 22(6):393–409. doi: 10.1038/s41580-021-00350-0
101. Cereijo R, Gavaldà-Navarro A, Cairó M, Quesada-López T, Villarroya J, Morón-Ros S, et al. CXCL14, a Brown Adipokine That Mediates Brown-Fat-To-Macrophage Communication in Thermogenic Adaptation. Cell Metab (2018) 28(5):750–63.e6. doi: 10.1016/j.cmet.2018.07.015
102. Nguyen KD, Qiu Y, Cui X, Goh YP, Mwangi J, David T, et al. Alternatively Activated Macrophages Produce Catecholamines to Sustain Adaptive Thermogenesis. Nature (2011) 480(7375):104–8. doi: 10.1038/nature10653
103. Fischer K, Ruiz HH, Jhun K, Finan B, Oberlin DJ, van der Heide V, et al. Alternatively Activated Macrophages Do Not Synthesize Catecholamines or Contribute to Adipose Tissue Adaptive Thermogenesis. Nat Med (2017) 23(5):623–30. doi: 10.1038/nm.4316
104. Shan B, Wang X, Wu Y, Xu C, Xia Z, Dai J, et al. The Metabolic ER Stress Sensor IRE1alpha Suppresses Alternative Activation of Macrophages and Impairs Energy Expenditure in Obesity. Nat Immunol (2017) 18(5):519–29. doi: 10.1038/ni.3709
105. Chaurasia B, Kaddai VA, Lancaster GI, Henstridge DC, Sriram S, Galam DL, et al. Adipocyte Ceramides Regulate Subcutaneous Adipose Browning, Inflammation, and Metabolism. Cell Metab (2016) 24(6):820–34. doi: 10.1016/j.cmet.2016.10.002
106. Vasiliauskaite-Brooks I, Sounier R, Rochaix P, Bellot G, Fortier M, Hoh F, et al. Structural Insights Into Adiponectin Receptors Suggest Ceramidase Activity. Nature (2017) 544(7648):120–3. doi: 10.1038/nature21714
107. Shin H, Ma Y, Chanturiya T, Cao Q, Wang Y, Kadegowda AKG, et al. Lipolysis in Brown Adipocytes Is Not Essential for Cold-Induced Thermogenesis in Mice. Cell Metab (2017) 26(5):764–77.e5. doi: 10.1016/j.cmet.2017.09.002
108. Jiang H, Ding X, Cao Y, Wang H, Zeng W. Dense Intra-Adipose Sympathetic Arborizations Are Essential for Cold-Induced Beiging of Mouse White Adipose Tissue. Cell Metab (2017) 26(4):686–92.e3. doi: 10.1016/j.cmet.2017.08.016
109. Zeng X, Ye M, Resch JM, Jedrychowski MP, Hu B, Lowell BB, et al. Innervation of Thermogenic Adipose Tissue via a Calsyntenin 3β–S100b Axis. Nature (2019) 569(7755):229–35. doi: 10.1038/s41586-019-1156-9
110. Orthofer M, Valsesia A, Magi R, Wang QP, Kaczanowska J, Kozieradzki I, et al. Identification of ALK in Thinness. Cell (2020) 181(6):1246–62.e22. doi: 10.1016/j.cell.2020.04.034
111. Harms M, Seale P. Brown and Beige Fat: Development, Function and Therapeutic Potential. Nat Med (2013) 19(10):1252–63. doi: 10.1038/nm.3361
112. Blondin DP, Daoud A, Taylor T, Tingelstad HC, Bezaire V, Richard D, et al. Four-Week Cold Acclimation in Adult Humans Shifts Uncoupling Thermogenesis From Skeletal Muscles to Brown Adipose Tissue. J Physiol (2017) 595(6):2099–113. doi: 10.1113/JP273395
113. Arch JR. Challenges in Beta(3)-Adrenoceptor Agonist Drug Development. Ther Adv Endocrinol Metab (2011) 2(2):59–64. doi: 10.1177/2042018811398517
114. Blondin DP, Nielsen S, Kuipers EN, Severinsen MC, Jensen VH, Miard S, et al. Human Brown Adipocyte Thermogenesis Is Driven by Beta2-AR Stimulation. Cell Metab (2020) 32(2):287–300.e7. doi: 10.1016/j.cmet.2020.07.005
115. Kung J, Henry RR. Thiazolidinedione Safety. Expert Opin Drug Saf (2012) 11(4):565–79. doi: 10.1517/14740338.2012.691963
116. Fisher FM, Maratos-Flier E. Understanding the Physiology of FGF21. Annu Rev Physiol (2016) 78:223–41. doi: 10.1146/annurev-physiol-021115-105339
117. Gaich G, Chien JY, Fu H, Glass LC, Deeg MA, Holland WL, et al. The Effects of LY2405319, an FGF21 Analog, in Obese Human Subjects With Type 2 Diabetes. Cell Metab (2013) 18(3):333–40. doi: 10.1016/j.cmet.2013.08.005
118. Gimeno RE, Moller DE. FGF21-Based Pharmacotherapy–Potential Utility for Metabolic Disorders. Trends Endocrinol Metab (2014) 25(6):303–11. doi: 10.1016/j.tem.2014.03.001
119. Brown AC. Brown Adipocytes From Induced Pluripotent Stem Cells-How Far Have We Come? Ann NY Acad Sci (2019). doi: 10.1111/nyas.14257
120. Yoneshiro T, Shin W, Machida K, Fukano K, Tsubota A, Chen Y, et al. Differentiation of Bone Marrow-Derived Cells Toward Thermogenic Adipocytes in White Adipose Tissue Induced by the β3 Adrenergic Stimulation. FASEB J (2019) 33(4):5196–207. doi: 10.1096/fj.201801757RR
121. Singh AM, Dalton S. What Can ‘Brown-Ing’ Do For You? Trends Endocrinol Metab (2018) 29(5):349–59. doi: 10.1016/j.tem.2018.03.002
122. Stanford KI, Middelbeek RJ, Townsend KL, An D, Nygaard EB, Hitchcox KM, et al. Brown Adipose Tissue Regulates Glucose Homeostasis and Insulin Sensitivity. J Clin Invest (2013) 123(1):215–23. doi: 10.1172/JCI62308
123. Liu X, Zheng Z, Zhu X, Meng M, Li L, Shen Y, et al. Brown Adipose Tissue Transplantation Improves Whole-Body Energy Metabolism. Cell Res (2013) 23(6):851–4. doi: 10.1038/cr.2013.64
124. Min SY, Kady J, Nam M, Rojas-Rodriguez R, Berkenwald A, Kim JH, et al. Human ‘Brite/Beige’ Adipocytes Develop From Capillary Networks, and Their Implantation Improves Metabolic Homeostasis in Mice. Nat Med (2016) 22(3):312–8. doi: 10.1038/nm.4031
Keywords: beiging, brown/beige fat, UCP1, thermogenesis, obesity
Citation: Wang Z, Yu X and Chen Y (2021) Recruitment of Thermogenic Fat: Trigger of Fat Burning. Front. Endocrinol. 12:696505. doi: 10.3389/fendo.2021.696505
Received: 16 April 2021; Accepted: 04 June 2021;
Published: 22 July 2021.
Edited by:
Takeshi Yoneshiro, The University of Tokyo, JapanReviewed by:
Masayuki Saito, Hokkaido University, JapanCopyright © 2021 Wang, Yu and Chen. This is an open-access article distributed under the terms of the Creative Commons Attribution License (CC BY). The use, distribution or reproduction in other forums is permitted, provided the original author(s) and the copyright owner(s) are credited and that the original publication in this journal is cited, in accordance with accepted academic practice. No use, distribution or reproduction is permitted which does not comply with these terms.
*Correspondence: Yong Chen, dGoueS5jaGVuQHZpcC4xNjMuY29t
Disclaimer: All claims expressed in this article are solely those of the authors and do not necessarily represent those of their affiliated organizations, or those of the publisher, the editors and the reviewers. Any product that may be evaluated in this article or claim that may be made by its manufacturer is not guaranteed or endorsed by the publisher.
Research integrity at Frontiers
Learn more about the work of our research integrity team to safeguard the quality of each article we publish.