- 1National Center for the Medical Consequences of Spinal Cord Injury, James J. Peters VA Medical Center, Bronx, NY, United States
- 2Department of Medicine, Icahn School of Medicine at Mount Sinai, New York, NY, United States
- 3Research Service, Birmingham VA Medical Center, Birmingham, AL, United States
- 4Department of Cell, Developmental and Integrative Biology, University of Alabama-Birmingham, Birmingham, AL, United States
- 5Department of Rehabilitation and Human Performance, Icahn School of Medicine at Mount Sinai, New York, NY, United States
- 6Research Service and Brain Rehabilitation Research Center, Malcolm Randall VA Medical Center, North Florida/South Georgia Veterans Health System, Gainesville, FL, United States
- 7Division of Endocrinology, Diabetes, and Metabolism, University of Florida College of Medicine, Gainesville, FL, United States
Spinal cord injury (SCI) results in dysregulation of carbohydrate and lipid metabolism; the underlying cellular and physiological mechanisms remain unclear. Fibroblast growth factor 21 (FGF21) is a circulating protein primarily secreted by the liver that lowers blood glucose levels, corrects abnormal lipid profiles, and mitigates non-alcoholic fatty liver disease. FGF21 acts via activating FGF receptor 1 and ß-klotho in adipose tissue and stimulating release of adiponectin from adipose tissue which in turn signals in the liver and skeletal muscle. We examined FGF21/adiponectin signaling after spinal cord transection in mice fed a high fat diet (HFD) or a standard mouse chow. Tissues were collected at 84 days after spinal cord transection or a sham SCI surgery. SCI reduced serum FGF21 levels and hepatic FGF21 expression, as well as β-klotho and FGF receptor-1 (FGFR1) mRNA expression in adipose tissue. SCI also reduced serum levels and adipose tissue mRNA expression of adiponectin and leptin, two major adipokines. In addition, SCI suppressed hepatic type 2 adiponectin receptor (AdipoR2) mRNA expression and PPARα activation in the liver. Post-SCI mice fed a HFD had further suppression of serum FGF21 levels and hepatic FGF21 expression. Elevated serum free fatty acid (FFA) levels after HFD feeding were observed in post-SCI mice but not in sham-mice, suggesting defective FFA uptake after SCI. Moreover, after SCI several genes that are implicated in insulin’s action had reduced expression in tissues of interest. These findings suggest that downregulated FGF21/adiponectin signaling and impaired responsiveness of adipose tissues to FGF21 may, at least in part, contribute to the overall picture of metabolic dysfunction after SCI.
Introduction
Spinal cord injury (SCI) causes partial or total interruption of neural signal transmission between the brain and the periphery, thereby limiting physical activity. Chronic SCI, due to an extremely sedentary lifestyle, invariably results in adverse body composition changes characterized by decreased lean mass and increased adiposity. Such changes are associated with metabolic dysfunction (1–5) leading to an increased risk for cardiovascular disease, liver disorders, and type 2 diabetes (T2DM) compared to the general population (6–9). However, the underlying mechanisms for the predisposition of persons with SCI to metabolic perturbations and the associated cardiac and hepatic disorders remain unclear. Several lines of evidence suggest that alterations in the liver or visceral adipose tissue including neutrophil infiltration, macrophage activation, increased expression of pro-inflammatory cytokines and hepatic lipid accumulation (10–13) which often is associated with development of non-alcoholic fatty liver disease (NAFLD) and non-alcoholic steatohepatitis (NASH) (14, 15). Furthermore, persons with SCI have decreased anabolic hormone levels (16) which are associated with abnormal lipid and metabolic profiles. A clear understanding of hepatic pathology that develops after SCI may provide new insight into the development of impairments of fat and carbohydrate metabolism. However, limited studies have addressed the details of pathophysiological events which occur in the liver after SCI. The mechanism underlying dyslipidemia and whether this change precedes, or is the cause of, systemic metabolic dysfunction after SCI is not known. In addition, the potential mediators, if any, that regulate the interaction between hepatic function and other tissues to achieve systemic metabolic homeostasis after SCI have yet to be investigated.
Fibroblast growth factor-21 (FGF21) is a metabolically active peptide primarily secreted by the liver in response to various nutritional, physiological and pathological stimuli (17). FGF21 is an integral component of the hormone network that modulates the action of insulin, leptin, glucagon-like peptide (GLP1), adiponectin, growth hormone, and insulin-like growth factor (18). FGF21 has been shown to regulate carbohydrate and lipid metabolism in the liver, adipose tissue, skeletal muscle, pancreas, and central nervous system (18–20). In animal models, FGF21 corrects multiple abnormalities of metabolism, including those present in persons with insulin resistance and T2DM (21–23). FGF21 reduces levels of serum triglycerides (TG), total cholesterol, and low-density lipoprotein (LDL)-cholesterol but raises the level of high-density lipoprotein (HDL)-cholesterol, all of which changes represent favorable alterations to the lipid profile (19, 21). Transgenic mice overexpressing FGF21 exhibit resistance to the development of high fat diet (HFD)-induced obesity (24). In addition, FGF21 exerts hepato-protective effects by promoting hepatic lipid and free fatty acid (FFA) metabolism, reducing hepatic adiposity, and ameliorating NAFLD and NASH (19, 22, 25–27). Moreover, FGF21 can act on cardiac muscle to protect against heart disease (28) and ameliorates obesity-related inflammation in a rat model reducing inflammatory cytokine production (29). The molecular mechanisms by which FGF21 produces its physiological effects are, however, still being elucidated. FGF21 transcription is induced by peroxisome proliferator-activated receptor (PPAR)-γ and PPAR-α, which are the targets of agents prescribed to treat T2DM (thiazolidinediones) and hyperlipidemia (fibrates), respectively (23, 30).
FGF21 requires the obligate coreceptor β-klotho (KLB) for cellular signaling both in vivo and in vitro (31). The binding of FGF21 to KLB enables its interaction with the FGF receptor 1 (FGFR1), thus activating intracellular signaling pathways (32). FGF21 signaling is tissue-specific and is chiefly determined by tissue distribution of KLB. Adipose tissue has high levels of KLB expression and is a primary site of FGF21 action (32, 33). In obese individuals, serum FGF21 levels positively correlate with the subcutaneous adipose tissue (SAT) area (34). FGF21 knockout mice show less SAT and are more insulin-resistant when fed a HFD (24, 34). Mechanistically, FGF21 improves systemic insulin sensitivity by promoting the healthy expansion of SAT (34), thereby increasing serum levels of adiponectin, a major adipokine produced by adipose tissue, which mediates the beneficial effects of FGF21 on the liver and muscle (35, 36). Adiponectin signaling is mediated by activation of two types of receptor: type 1 (AdipoR1) is predominantly expressed in skeletal muscle and activates AMP-kinase; the type 2 adiponectin receptor (AdipoR2) is predominantly expressed in the liver and activates the PPARα signaling pathway (37). Of note, the magnitude of FGF21-induced weight loss is reduced in leptin-deficient mice (33), implying a possible requirement of a functional leptin axis for FGF21 action on body weight regulation (38). While the beneficial effects of FGF21 on metabolic homeostasis have been extensively studied in able-bodied individuals, FGF21 expression and function after SCI has not been investigated.
Persons with SCI have been reported to have reduced energy expenditure resulting from a marked reduction in lean tissue mass and in the ability to ambulate and/or exercise (39, 40). On the other hand, recent reports indicated increased total food intake in persons with SCI when compared to individuals who were immobilized for reasons other than SCI (41, 42). In addition, analysis of the pattern of food intake of overweight or obese persons with SCI suggests that there is an increased proportion of total calories consumed from fat (43). These post-SCI dietary changes almost certainly accelerate the accumulation of fat in various body compartments. However, studies that investigated nutritional status in chronic SCI are limited, and knowledge with respect to how macronutrients impact metabolic gene expression and dysregulation of metabolic homeostasis after SCI is lacking. Furthermore, the relationship between serum FGF21 levels and SCI is unknown.
Using a spinal cord transection model, a high fat diet was recently reported to induce glucose intolerance without increasing fat mass or liver weights (44). To understand the possible role of FGF21 in altered fat, carbohydrate and energy metabolism following SCI, blood and tissue samples collected from SCI mice fed either a high fat diet (HFD) or control diet (ConD) were used to determine circulating FGF21 levels and expression of FGF21 mRNA. The cause of the potential perturbations in FGF21 levels and, if altered, the probable impact on lipid and carbohydrate metabolism was investigated by analyses of relevant signaling and molecular changes in fat, liver and muscle.
Methods
Animal Studies
Procedures with experimental animals were approved by the Institutional Animal Care and Use Committee at the James J. Peters VA Medical Center and were conducted in accordance with all applicable Federal standards for humane animal care and use of laboratory animals. A detailed description of the experimental protocol has been previously published (44). Briefly, three-month-old male C57BL/6 mice were purchased from Charles River. Animals were randomly assigned to 4 groups: two sham-laminectomy (Sham) groups in which the spinal cord was exposed but not transected, and two thoracic spinal cord transection (level T9) groups. At day 84 after surgery, the animals were euthanized. The liver, adipose tissue including inguinal fat, (iFAT) and omental fat (oFAT); and gastrocnemius muscles were carefully removed and immediately frozen in liquid nitrogen. Blood was collected by ventricular puncture, and serum was frozen for subsequent analysis.
High-Fat Diet (HFD)
A lard-based HFD was purchased from Research Diets [D12492; 60% fat, 20% protein, 20% carbohydrate, 5.21 kcal/g energy density]. A source and micro-nutrient control chow with a macronutrient content similar to standard rodent chow [D12450J; 10% fat, 20% protein, 70% carbohydrate, 3.82 kcal/g energy density] was fed to the control-diet (ConD) group. Animals were fed standard rodent chow pre-surgery with mice having ad libitum access to HFD and control chows beginning immediately post-surgery. Chow was replaced every 3d.
Reverse Transcriptase Quantitative Real-Time PCR (RT-qPCR)
Total RNA was extracted using RNasey mini kits (QIAGEN, Germantown, MD). Relative expression of mRNA was determined by qRt‐PCR, as described previously (45) using a model ViiA7 thermocycler (Applied Biosystems, Foster City, CA). Specific primers and TagMan Universal Master Mixer were purchased from Applied Biosystems. TagMan Reaction Mixes contained 3 μl cDNA (10 ng/μl). For each sample, the determinations were performed in triplicate. Relative mRNA levels were expressed as fold‐change using the 2−ΔΔCt method. Data were normalized relative to 18s RNA.
Preparation of Tissue Lysates and Western Blotting (WB)
The tissues were homogenized in 500 μl lysate buffer [150 mM sodium chloride, 3.2 mM Na2PO4, 0.8 mM K2PO4 (pH 7.4), 1% NP-40, 0.5% sodium deoxycholate, 0.5% sodium dodecylsulfate] using a tissue grinder bead homogenizer (MP Biomedicals Inc., Solon, OH) followed by centrifugation at 14,000 rpm for 5 min. Western blot analysis was performed as described previously (45). Antibodies against phospho-ACC and total ACC were purchased from Cell Signaling Technology (Beverly, MA). Anti-phospho-PPARα, total PPARα and β-tubulin antibodies were obtained from AbCam (Cambridge, MA). Densitometry was determined using Image Lab software (Bio-Rad, Hercules, CA). β-tubulin was used as a loading control. All assays were performed in triplicate. Mean Ct values for the replicates were used in all subsequent calculations.
Preparation of Nuclear Extracts and PPARα DNA-Binding Activity Assay
Nuclear extracts were prepared from mouse liver using a commercial kit (Pierce; Rockford, IL). The binding capacity of PPARα to DNA was assayed using a non-radioactive assay kit from AbCam (Cambridge, MA), which uses a sensitive method for detecting specific transcription factor DNA binding activity in the nuclear extracts. The assays were performed according to the manufacturer’s instructions in duplicate with mean values for the two determinations used in all subsequent calculations.
Serum Preparation and Enzyme-Linked Immunosorbent Assay (ELISA)
The blood samples were collected on ice and allowed to clot before being centrifugation for 20 min at 1000 g with the serum separated from the formed blood cell elements. The FGF21, adiponectin and leptin assays were performed using a commercial ELISA kit (R&D System; Minneapolis, MN); The high molecular weight (HMW) adiponectin assay was performed by a commercial kit (Creative Diagnostics; Shirley, NY). Alanine aminotransferase (ALT) and free fatty acid (FFA) assay kits were purchased from MyBioSource Inc. (Santa Ana, CA) and AbCam respectively. All assays were performed according to manufacturer’s instructions. Assays were performed in duplicate with mean values for the two determinations used in all subsequent calculations.
Glycogen Content Assay
Tissue glycogen levels were measured using a colorimetric kit (AbCam). Briefly, mouse liver and skeletal muscle tissues were homogenized with 200 μl distilled water on ice. The homogenates were boiled for 10 min followed by centrifugation at 18,000 rpm for 10 min. Supernatant was collected and subjected to assay according to the manufacturer’s instruction. Samples were assayed in duplicate and mean values for the duplicates were used in subsequent calculations.
Statistics
Data are expressed as mean values ± SEM. The significance of differences between sham and SCI groups, with or without HFD, was determined using Two-Way ANOVA with a Bonferroni test post-hoc. Statistical calculations were performed using Prism software (GraphPad, San Diego, CA). p < 0.05 was considered statistically significant.
Results
SCI Reduced Serum FGF21 levels and Inhibited FGF21 Receptor Expression in Adipose Tissue
To determine whether FGF21 plays a role in SCI-induced dysregulation of metabolism, serum FGF21 levels were examined. There was a significant reduction in serum FGF21 levels after 84 days of SCI in mice fed a ConD (SCI-ConD) compared to sham-mice fed the same diet (sham-ConD) (Figure 1A). Because circulating FGF21 levels correlate well with hepatic gene expression (46), FGF21 mRNA expression in mouse liver was determined. As shown in Figure 1B, SCI led to a significant downregulation of hepatic FGF21 mRNA expression. In addition, we observed that sham mice fed with HFD (sham-HFD) also had reduced FGF21 serum levels and hepatic FGF21 mRNA expression; serum FGF21 and hepatic FGF21 mRNA were further decreased in SCI-HFD mice compared to SCI-ConD mice (Figure 1B). Adipose tissue expresses high levels of FGFR1 and KLB and is a primary site of FGF21 action in regulating fat and carbohydrate metabolism (32). Therefore, the effects of SCI with or without HFD on FGFR1 and KLB gene expression were examined in adipose tissues. Because increased intra-abdominal fat worsens insulin resistance, as opposed to extra-abdominal fat depots (47), both inguinal fat (iFAT) and omental fat (oFAT) were analyzed. There were significant reductions in both FGFR1 and KLB mRNA expression in iFAT (Figures 1C, D) and oFAT of SCI-ConD mice compared to sham-ConD mice. While HFD-feeding did not change FGFR1 and KLB mRNA levels in iFAT of sham mice, a significant increase in mRNA expression was seen in oFAT of sham-HFD mice (Figures 1C, D). In SCI-HFD mice, KLB mRNA levels were reduced compared to SCI-ConD mice in iFAT (Figure 1C) but not oFAT (Figure 1D).
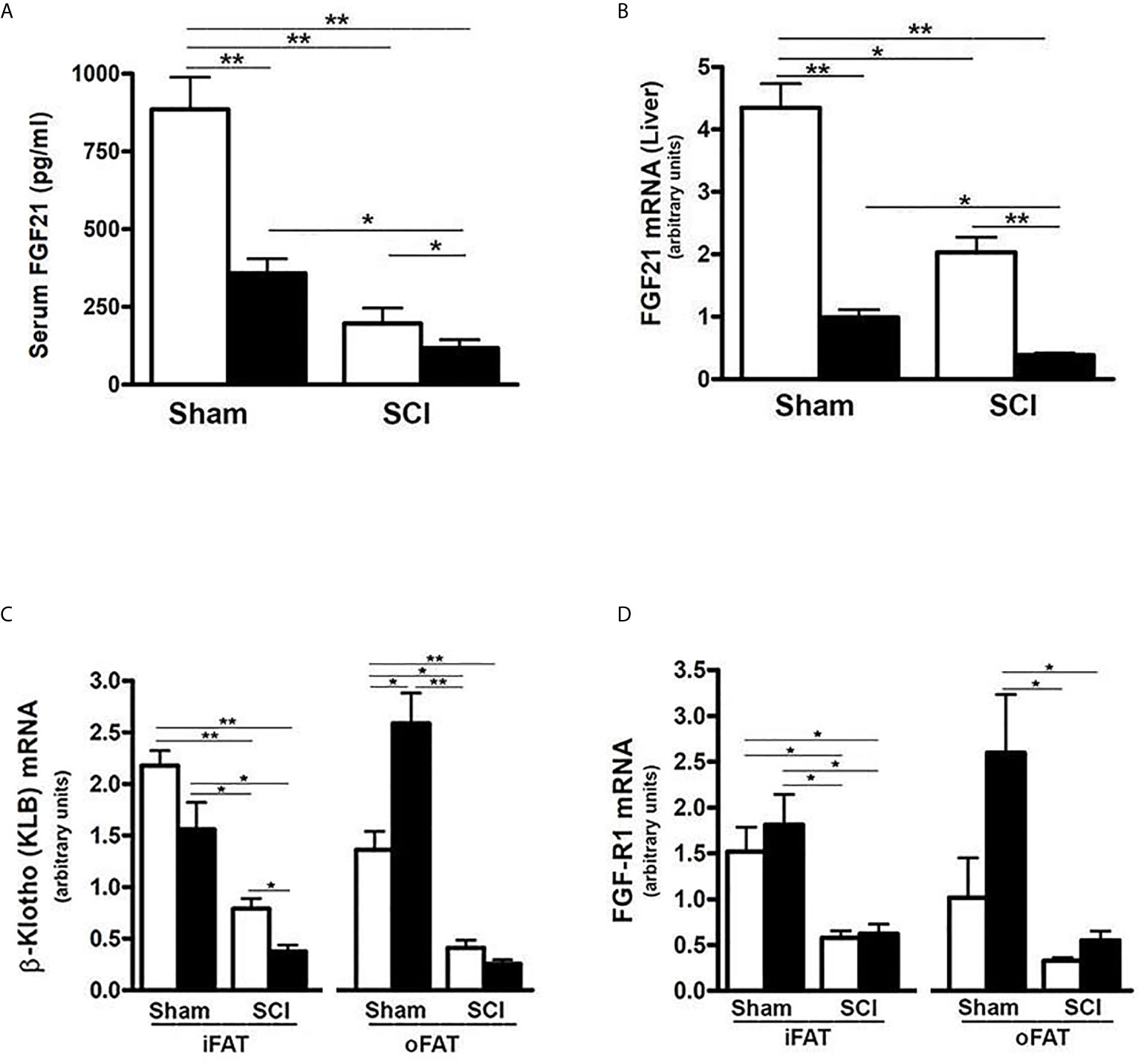
Figure 1 SCI and high-fat diet (HFD) downregulated FGF21 signaling - Mice were fed with either ConD (White Bar) or HFD (Black Bar) after surgery. (A), mouse FGF21 serum levels measured by ELISA. (B), hepatic FGF21 mRNA expression by Rt-PCR. (C), KLB mRNA expression in mouse adipose tissues by Rt-PCR, and (D), FGFR1 mRNA expression in mouse adipose tissues by Rt-PCR. Data shown are mean values ± SEM (n = 5). *p < 0.05; **p < 0.01.
SCI Reduced Expression and Serum Levels of Adiponectin and Leptin
It has been demonstrated that FGF21 increases insulin sensitivity through direct effects on fat to stimulate expression and secretion of adiponectin, a critical mediator of FGF21 actions in the liver and skeletal muscle which are two major sites of insulin-induced glucose uptake, and also by specific expansion of subcutaneous fat (34–36). Thus, we determined whether reduced serum FGF21 impacted the production of adiponectin and leptin as well as adipose tissue differentiation markers. Consistent with reduced levels of FGFR1 and KLB noted in both iFAT and oFAT of SCI-ConD mice, there was a significant decrease in mRNA expression of adiponectin in iFAT and oFAT of SCI-ConD mice as compared to sham-ConD mice (Figure 2A). Serum levels of both total adiponectin and HMW adiponectin, the most potent form of the protein (48), were also reduced by SCI (Figures 2B, C). Levels of serum total adiponectin in sham-HFD and SCI-HFD mice were further decreased as compared to sham-ConD and SCI-ConD groups, respectively (Figure 2B). HMW adiponectin was decreased in sham-HFD mice when compared to sham-ConD mice but was not different when comparing SCI-HFD and SCI-ConD mice (Figure 2C).
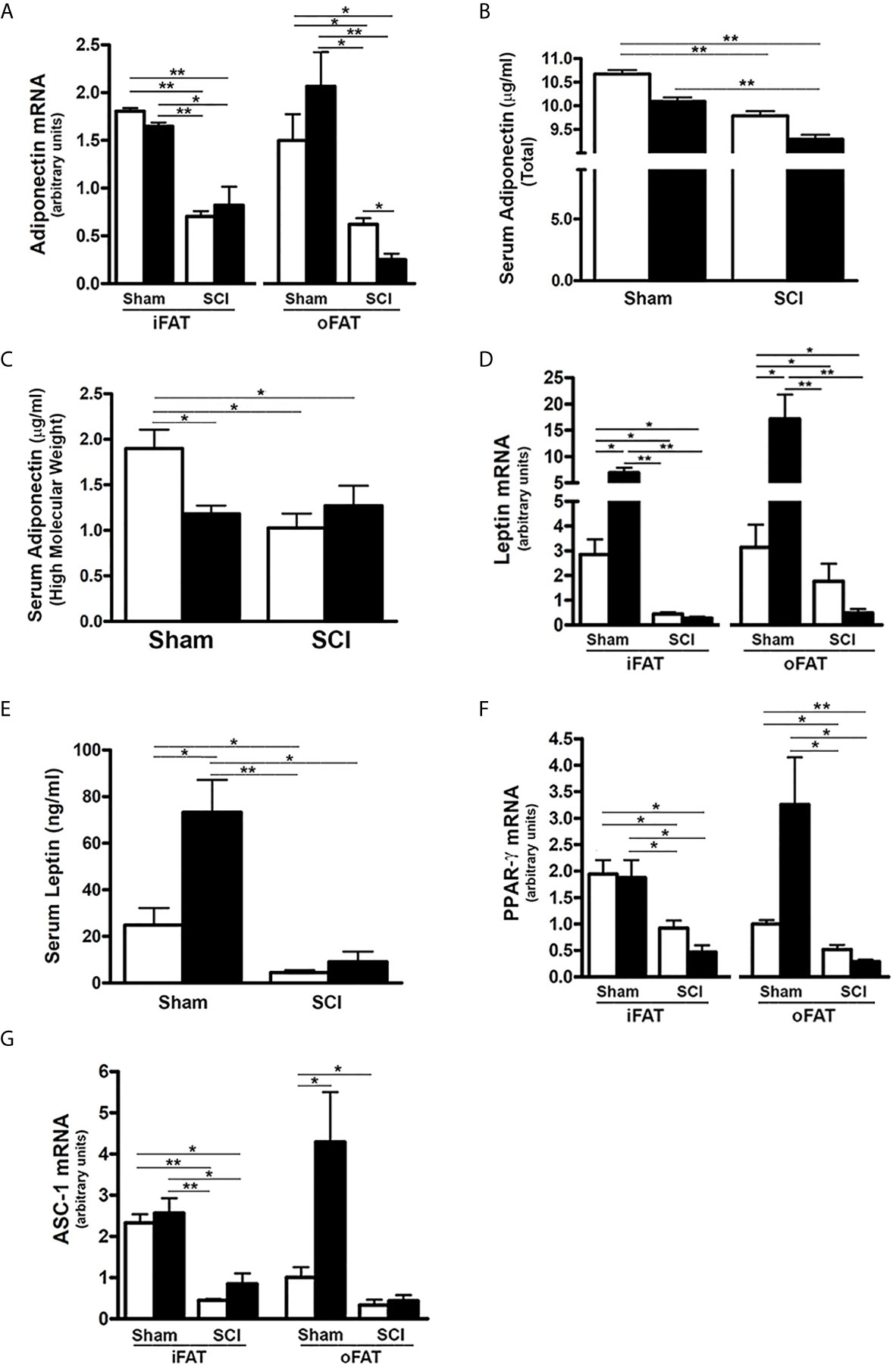
Figure 2 Effects of SCI and HFD on Gene Expression of Adipokines and Differential Markers in Mouse Adipose Tissue. Mice were fed with either ConD (White Bar) or HFD (Black Bar) after surgery. Total RNA was isolated from mouse adipose tissues and subjected to Rt-PCR analysis for (A), adiponectin mRNA expression; (B), serum total adiponectin levels measured by ELISA; (C), serum HMW adiponectin levels measured by ELISA; (D), leptin mRNA expression; (E), serum leptin secretion measured by ELISA; (F), PPARγ mRNA expression; and (G), ASC-1 mRNA expression. Data shown are mean values ± SEM (n = 5). *p < 0.05; **p < 0.01.
Leptin, another major adipokine, has been implicated in one of the actions of FGF21 (33, 38). The effects of SCI and HFD on leptin mRNA expression and protein secretion were therefore examined. Leptin mRNA in both iFAT and oFAT (Figure 2D), and serum protein levels (Figure 2E) were reduced in SCI-ConD mice as compared to sham-ConD mice. Feeding mice with a HFD induced a significant increase in iFAT and oFAT leptin mRNA and serum leptin protein in sham mice, but did not alter serum leptin or leptin mRNA in iFAT or oFAT of SCI-HFD mice when compared to SCI-ConD mice (Figures 2D, E), suggesting to an impaired ability of adipose tissue to secrete leptin in response to a HFD.
PPARγ, a downstream target of FGF21 in adipocytes, is a master transcriptional regulator of adipocyte differentiation, and a determinant of adiponectin expression (23, 49). There was a significant reduction in PPARγ mRNA expression in iFAT and oFAT of SCI-ConD mice as compared to sham-ConD mice (Figure 2F). Dramatically reduced mRNA levels of ASC-1, a marker of white adipose tissue (50), was also observed in iFAT and oFAT of SCI-ConD mice (Figure 2G). Expression of PPARγ and ASC were not significantly changed by HFD in sham or SCI mice (Figures 2F, G). These data indicate that SCI decreased secretion of adiponectin and leptin, and inhibited adipocyte differentiation suggesting a linkage of these impairments to reduced FGF21 signaling.
SCI Reduced Hepatic Adiponectin Receptor 2 (AdipoR2) Expression and PPARα Activity
AdipoR2 is predominantly expressed in the liver and plays an important role in regulating hepatic lipid metabolism (37, 51). To evaluate whether SCI impacts hepatic adiponectin signaling, AdipoR2 expression was examined in the liver of SCI mice. There was a significant reduction in AdipoR2 mRNA expression in SCI-ConD mice compared to sham-ConD mice and these levels were further decreased in SCI-HFD mice as compared to SCI-ConD mice (Figure 3A). A HFD also decreased AdipoR2 mRNA in livers of sham-HFD mice compared to sham-ConD mice (Figure 3A).
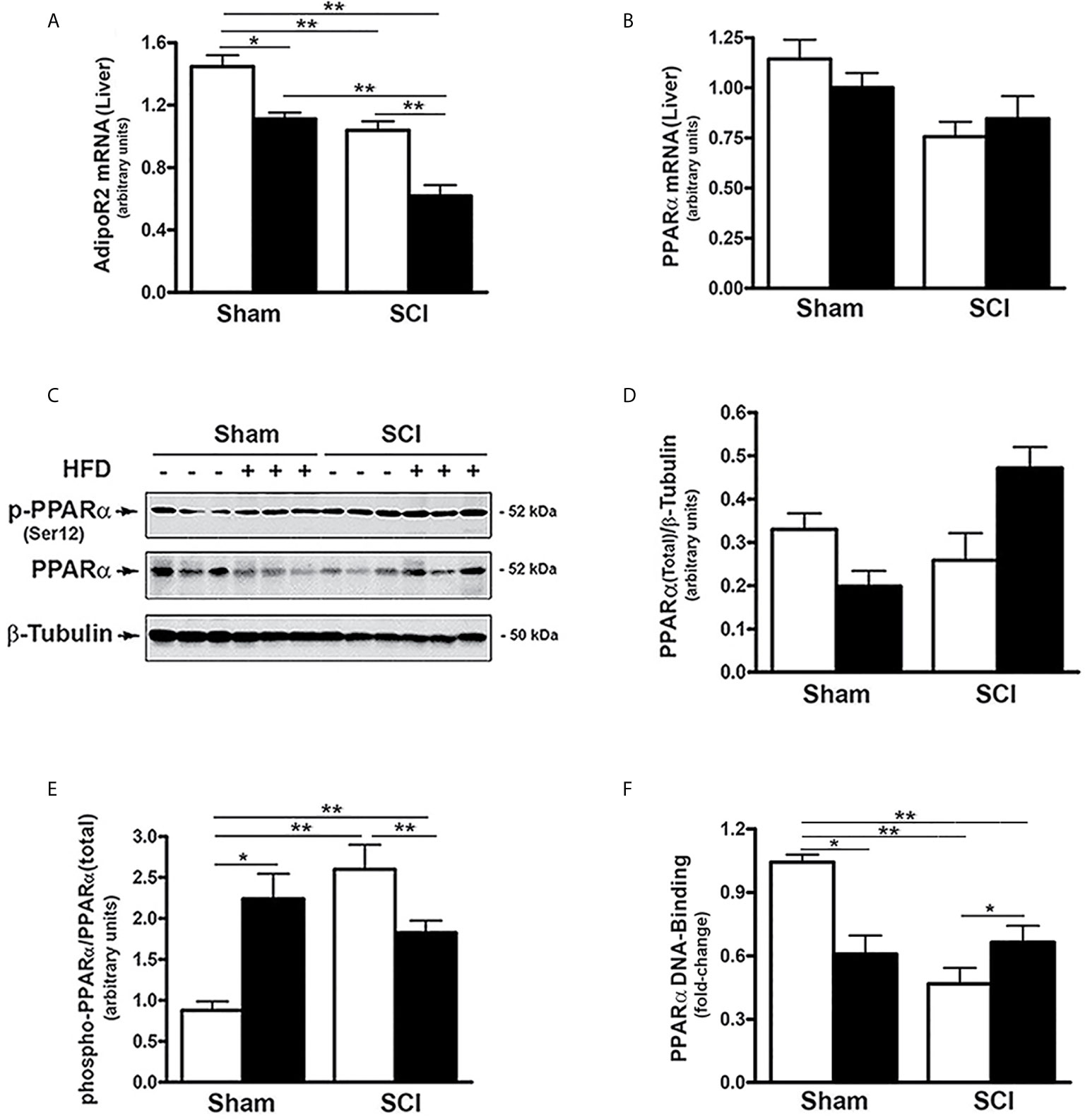
Figure 3 SCI suppressed AdipoR2 expression and PPARα activation - Mice were fed with either ConD (White Bar) or HFD (Black Bar) after surgery. Total RNA was isolated from mouse liver and subjected to Rt-PCR analysis for (A), AdipoR2 mRNA; (B), PPARα mRNA. (C), total protein lysates were extracted from mouse liver and subjected to Western blot analysis using anti-endogenous PPARα, or anti-phospho-PPARα (Ser12), or β-tubulin antibodies; (D), blots were quantified by scanning densitometry and blots in the middle panel of (C) were normalized relative to β-tubulin (lower panel); (E), blots in the upper panel of (C) were normalized relative to endogenous (total) PPARα protein (middle panel). (F), Nuclear extracts were prepared from mouse liver and subjected to PPARα DNA-binding assay. Data shown are means values ± SEM (n = 5). Data shown in C are representative Western blots; *p < 0.05; **p < 0.01.
The expression and activity of PPARα, a nuclear receptor that serves as a downstream target of AdipoR2 (37, 52), was also examined. Neither SCI nor HFD changed hepatic PPARα mRNA levels (Figure 3B). PPARα transcriptional activity is decreased by phosphorylation at Ser12, (53). To understand how SCI altered PPARα activity, the effect of SCI on PPARα phosphorylation and nuclear levels were determined. While SCI had no significant effect on total PPARα protein (Figures 3C, D). the levels of phospho-(Ser12)-PPARα was increased in SCI-ConD compared to sham-ConD groups (Figures 3C, E). Phospho-(Ser12)-PPARα was lower in SCI-HFD mice compared to SCI-ConD mice and, by contrast, was higher in sham-HFD mice than sham-ConD mice (Figures 3C, E). To determine nuclear PPARα activity in SCI vs. sham mice, a DNA-binding assay was performed using nuclear extracts of mouse liver. PPARα DNA-binding capacity was reduced in SCI-ConD mice compared to sham-ConD mice but was elevated in SCI-HFD compared to SCI-ConD mice; in contrast, sham-HFD demonstrated decreased nuclear PPARα compared to sham-ConD (Figure 3F). These data are consistent with hepatic resistance to adiponectin after SCI due to lower expression of AdipoR2, lower nuclear PPARα and perturbed response of PPARα to dietary fats (52, 54).
SCI Influenced Rate Limiting Steps in Hepatic Cholesterol and Fatty Acid Metabolism
Because FGF21 regulates hepatic lipid and fatty acid metabolism and reduced PPARα activity after SCI (17, 19, 25–27), the effects of SCI on hydroxy-methyl-glutaryl Co-A reductase (HMGCR), the rate limiting enzyme in cholesterol synthesis, and ABCA1, the cholesterol efflux regulatory protein, were tested. HMGCR mRNA levels were markedly increased in livers from SCI-ConD mice compared to sham-ConD mice, whereas reduced mRNA levels were observed in SCI-HFD mice as compared to SCI-ConD mice. HMGCR mRNA levels were also reduced by HFD in sham mice (Figure 4A). In contrast, neither SCI nor HFD had no effect on ABCA1 mRNA expression (Figure 4B) suggesting that SCI may influence hepatic cholesterol synthesis but not efflux. We next examined the effect of SCI on acetyl-coenzyme A carboxylase (ACC), the rate limiting enzyme controlling fatty acid synthesis and metabolism. Expression of ACCα mRNA levels, the predominant isoform in the liver, was not changed in the SCI-ConD group compared to the sham-ConD group (Figure 4C). ACCα mRNA was significantly reduced in the sham-HFD and SCI-HFD groups compared to sham-ConD and SCI-ConD groups, respectively (Figure 4C). Expression of ACCα protein was upregulated in SCI-ConD mice compared to sham-ConD mice without a further change in the SCI-HFD mice when compared to SCI-ConD mice; HFD did not alter total ACCα protein in sham mice (Figures 4D, E). To understand how SCI and HFD influenced ACCα activity, ACCα phosphorylation at Ser79, which inhibits ACC activity (55), was tested. There was a significant decrease in Ser79-phosphorylated ACCα protein in SCI-ConD mice compared to sham-ConD mice (Figures 4D, F). Ser79-phosphorylation was not altered by HFD in sham or SCI mice (Figures 4D, F). These results suggest that SCI suppressed FGF21 signaling associated with increased activity of HMGCR and ACCα pathways that determine hepatic lipid synthesis and metabolism.
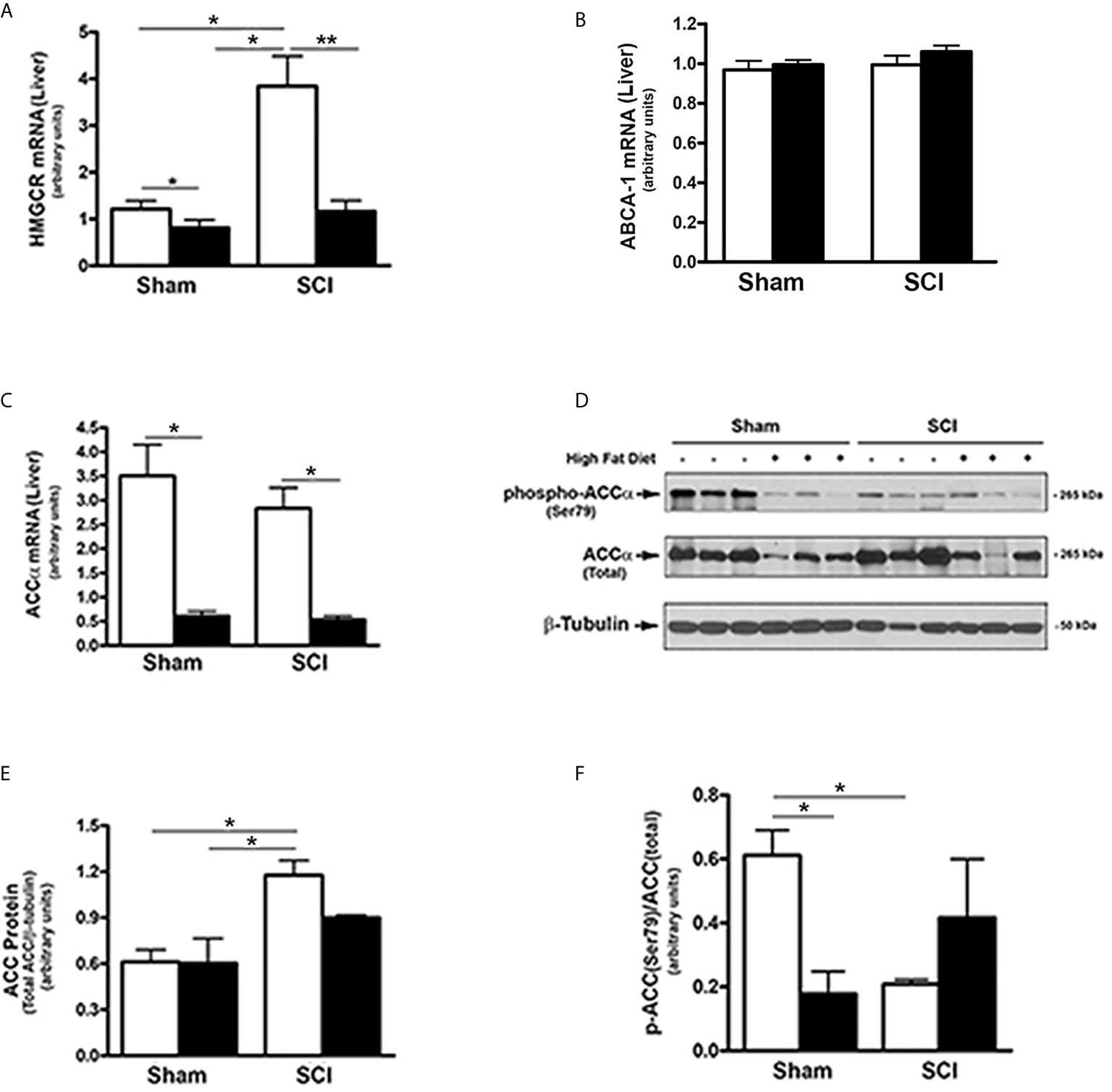
Figure 4 Effects of SCI and HFD on Metabolic Gene Expression in Mouse Liver. Mice were fed with either ConD (White Bar) or HFD (Black Bar) after surgery. Total RNA was isolated from mouse liver and subjected to Rt-PCR analysis for (A), HMGCR mRNA; (B), ABCA1 mRNA; (C), ACCα mRNA; (D), total protein lysates were extracted from mouse liver and subjected to Western blot analysis using anti-ACCα (endogenous) or anti-phospho-ACCα (T172) antibodies; (E), blots were quantified by scanning densitometry and blots in the middle panel of (D) were normalized relative to β-tubulin (lower panel); (F), blots in (D) (upper panel) were normalized relative to endogenous (total) ACCα protein (middle panel). Data shown are means values ± SEM (n = 5). Data shown in (C) are representative Western blots. *p < 0.05, **p < 0.01.
HFD Raised Serum FFA and Hepatic FABP4 Expression After SCI
To further determine the influence of SCI on lipid and fatty acid metabolism, serum FFA levels, which correlate with insulin resistance (56) were examined. When compared to sham-ConD mice, serum FFA levels were not significantly different in SCI-ConD mice although a trend toward lower values in SCI-ConD mice was noted (Figure 5A). However, as compared to SCI-ConD mice, there was a significant elevation in serum FFA level in SCI-HFD mice, whereas HFD did not alter serum FFA in sham mice (Figure 5A), suggesting defective FFA uptake by adipose or other tissues after SCI. In addition, the hepatic expression of FABP4 mRNA was examined, the level of which has been linked to insulin resistance, T2DM, hypertension and cardiac dysfunction (57). Although SCI-ConD mice had no change in FABP4 mRNA expression as compared to sham-ConD mice, SCI-HFD mice showed increased expression in hepatic FABP4 mRNA when compared to SCI-ConD mice; HFD did not change FABP4 mRNA levels in livers of sham mice (Figure 5B). This finding further implies that HFD may worsen SCI-induced metabolic dysfunction.
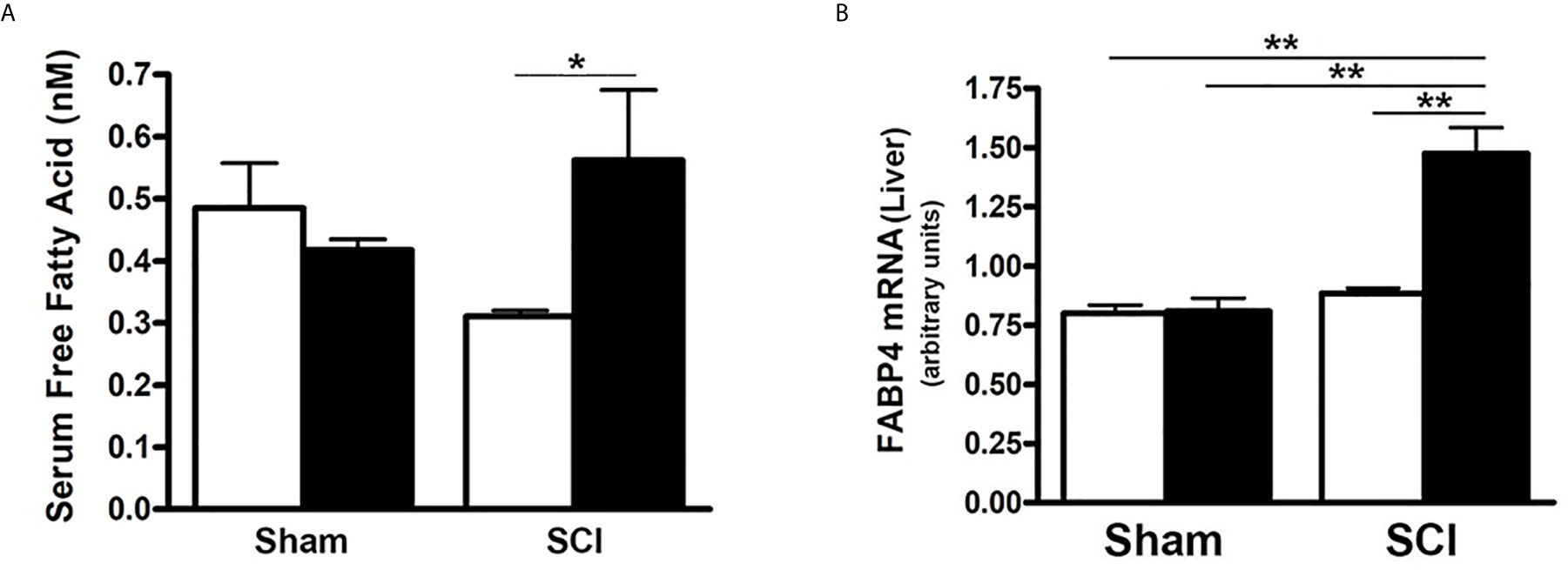
Figure 5 Differential Effect of HFD on Serum FFA Level in Post-SCI and Sham Mice. Mice were fed with either ConD (White Bar) or HFD (Black Bar) after surgery. (A), mouse serum was collected and subjected to ELISA for FFA serum level assay; (B), total RNA was isolated and subjected to Rt-PCR for hepatic FABP4 mRNA expression. Data shown are means values ± SEM (n = 5). *p < 0.05; **p < 0.01.
SCI Induced Increased Inflammatory Gene Expression in Fat and Liver
Inflammatory changes in liver and fat have been linked to impaired metabolism of carbohydrates (58). To understand if SCI and/or HFD influenced inflammatory status in liver or fat, selected mRNA levels of inflammatory cytokines were determined. Expression of TNFα mRNA in iFAT and oFAT was increased in SCI-ConD mice as compared to sham-ConD mice; a further increase in TNFα mRNA levels were observed in SCI-HFD mice when compared to SCI-ConD mice (Figure 6A). In sham mice, HFD increased TNFα mRNA expression in oFAT but not iFAT (Figure 6A). The expression levels of IL-1b and IL-6, two major cytokines induced by inflammation, were also tested. As shown in Figure 6, SCI had no effect on IL-1β or IL-6 mRNA in iFAT, but significantly upregulated IL-1β levels in oFAT (Figure 6B). HFD-feeding increased IL-1β and IL-6 mRNA levels in iFAT but not in oFAT (Figures 6B, C). While SCI had no effect on TNFα or TNF-receptor mRNA expression in mouse liver (Supplemental Data, Figure 2), significant upregulation of expression of IL-1β (Figure 6D), IL-6 (Figure 6E) and CD11b mRNA, a macrophage marker, (Figure 6F) were observed in the liver from SCI-ConD mice as compared to sham-ConD mice. The expression of IL-1β and IL-6 mRNA was not changed by HFD in sham or SCI mice. In contrast, while CD11b mRNA was not changed in sham-HFD mice, it was significantly elevated in SCI-HFD mice as compared to SCI-ConD mice (Figure 6F).
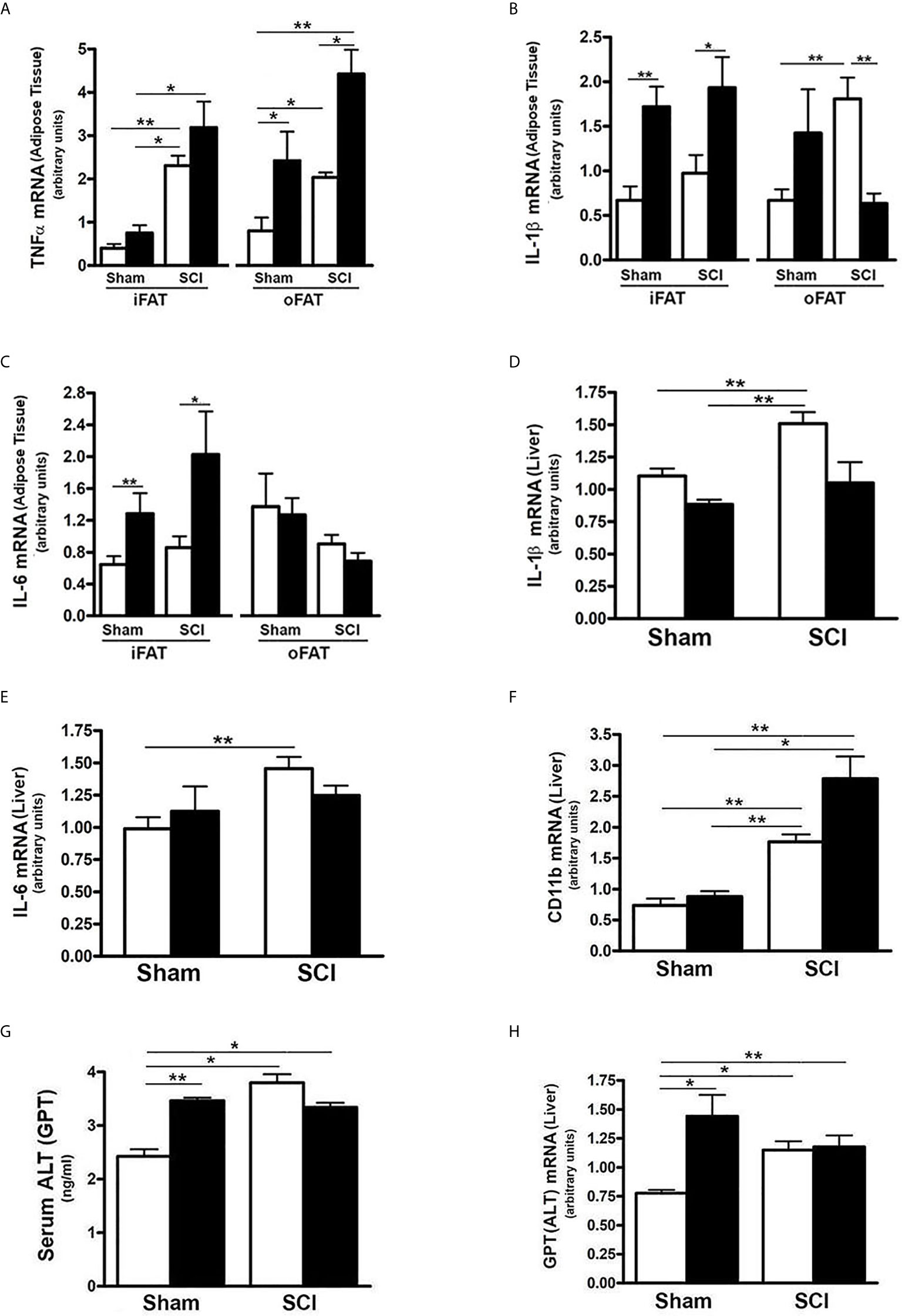
Figure 6 SCI Caused Hepatic Pro-Inflammatory State and Liver Toxicity. Mice were fed with either ConD (White Bar) or HFD (Black Bar) after surgery. (A), total RNA was isolated from mouse adipose tissues and subjected to Rt-PCR for TNFα mRNA expression; (B), IL-1β mRNA in adipose tissue; (C), IL-6 mRNA expression in adipose tissue; (D), total RNA was isolated from mouse liver and subjected to Rt-PCR for IL-1β mRNA; (E), hepatic IL-6 mRNA; (F), hepatic CD11b mRNA expression; (G), Mouse serum was collected and subjected to ELISA for ALT serum level; (H), total RNA was isolated from mouse liver and subjected to Rt-PCR for hepatic ALT mRNA expression. Data shown are means values ± SEM (n = 5). *p < 0.05; **p < 0.01.
To understand the potential impact of elevated CD11b on hepatocytes, serum and hepatic mRNA levels of ALT, a hepatic enzyme that has been used as a sensitive marker of liver injury, were determined. Elevated levels of both serum ALT (Figure 6G) and expression of hepatic ALT (Figure 6H) were observed in SCI-ConD mice as compared to sham-ConD mice. Serum ALT was increased in sham-HFD mice compared to sham-ConD mice but was not different between SCI-HFD and SCI-ConD mice (Figure 6G). Expression of hepatic ALT mRNA was increased in sham-HFD mice compared to sham-ConD mice, but not changed in SCI-HFD mice when compared to SCI-ConD mice (Figure 6H). Taken together, these findings suggest that SCI may trigger inflammatory responses marked by increased production of cytokines from adipose tissue and enhanced numbers of macrophages migrating to the liver, which could result in hepatocellular injury and liver dysfunction, as well as contribute to the general metabolic perturbations observed after SCI.
SCI Resulted in Tissue Specific Reduction of Insulin Signaling-Related Gene Expression
Because there are links among FGF21, adiponectin signaling, hepatic inflammation and insulin action (22, 25, 27, 36, 59), we determined whether the dysregulated FGF21 and adiponectin signaling observed after SCI were associated with altered mRNA expression of selected components of the insulin signaling pathway. As compared to sham-ConD, SCI-ConD mice demonstrated reduced expression of IRS-1 mRNA in iFAT and oFAT (Figure 7A), as well as in liver (Figure 7C). When comparing SCI-ConD and sham-ConD mice, decreased Glut4 mRNA expression was seen in iFAT and oFAT (Figure 7B). It is appreciated that muscle is the primary site of glucose uptake and disposal. In gastrocnemius muscle, expression of insulin receptor mRNA level was reduced in SCI-ConD mice as compared to sham-ConD mice (Figure 7E). However, there was no significant difference in Glut4 or IRS-1 mRNA in gastrocnemius muscle from SCI-ConD as compared to sham-ConD mice, and these parameters were not altered by HFD (Supplemental Data, Figure 1). To further evaluate how impaired FGF21 and adiponectin pathways impact insulin signaling, glycogen content was measured in mouse liver and skeletal muscle. There was a significant reduction in glycogen content in gastrocnemious muscle in SCI-ConD compared to sham-ConD mice (Figure 7F); muscle glycogen levels were not altered by HFD in either sham or SCI mice. In contrast, SCI with or without HFD had no effect on glycogen levels in liver (Figure 7D).
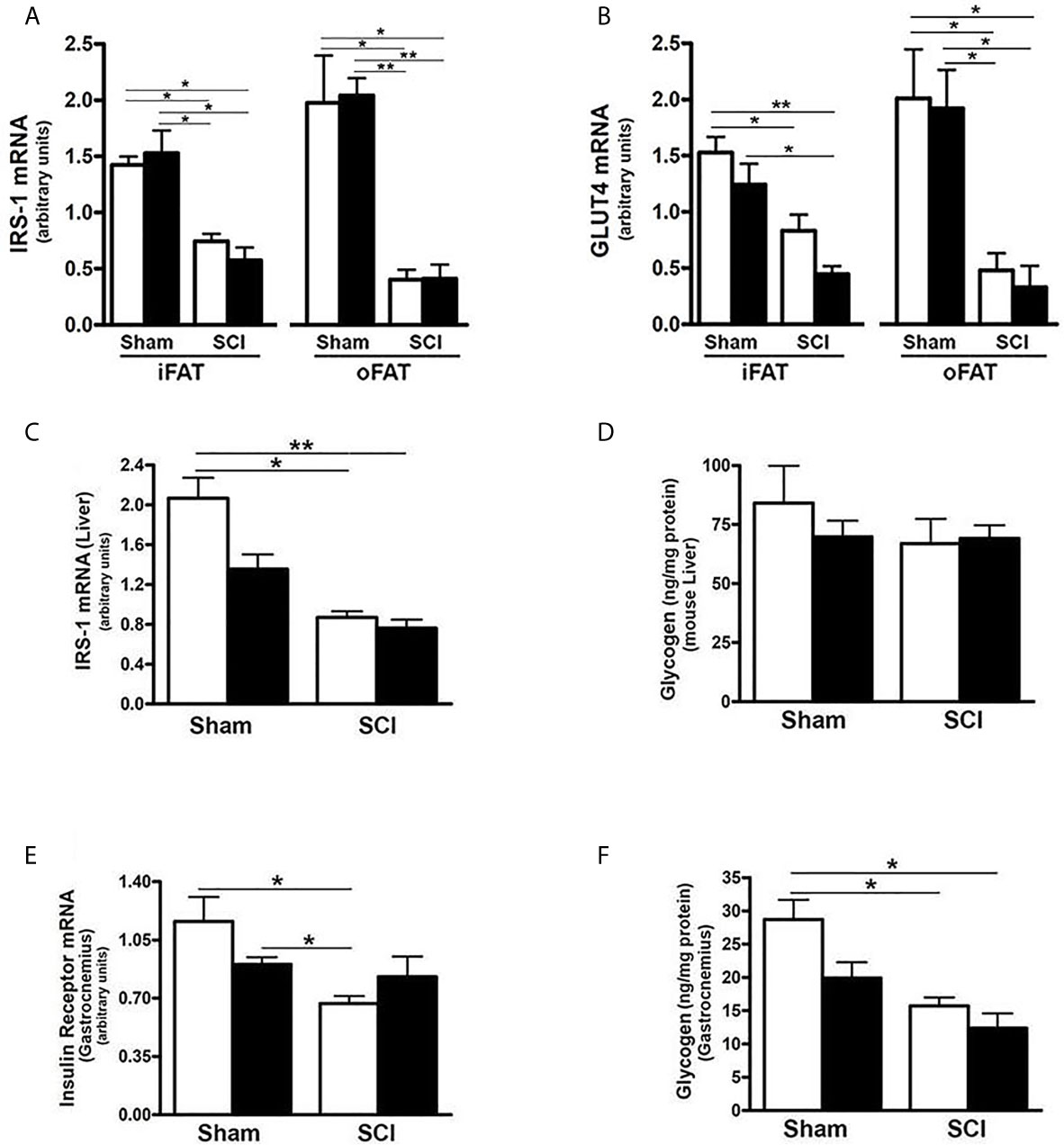
Figure 7 SCI Dysregulated Tissue Specific Expression of Insulin Signaling-Related Genes. Mice were fed with either ConD (White Bar) or HFD (Black Bar) after surgery. Total RNA was isolated from adipose tissues and subjected to Rt-PCR for (A), IRS-1 mRNA expression, and (B), Glut4 mRNA expression; (C), total RNA was isolated from mouse liver and subjected to Rt-PCR for hepatic IRS-1 mRNA expression; (D), liver glycogen content measured by colorimetric assay; (E), total RNA was isolated from mouse gastrocnemius and subjected to Rt-PCR for insulin receptor mRNA expression; (F), colorimetric assay for glycogen content in mouse gastrocnemius. Data shown are mean values ± SEM (n = 5) *p < 0.05; **p < 0.01.
Discussion
The central question addressed in this study was whether SCI per se has an impact to change serum FGF21 levels or activity of FGF21-dependent signaling, including that through adiponectin, in a mouse model of complete spinal cord transection. To better understand the meaning of changes in levels of these regulatory factors, the analysis employed a broad approach that examined signaling in liver, iFAT, oFAT and skeletal muscle which, collectively, are responsible for most effects of insulin on blood glucose levels, with adipose tissue and liver also playing key roles in synthesis, storage and release of fats. The principal conclusions of our work herein are that SCI reduced serum FGF21 and adiponectin levels, including total and the more bioactive HMW moiety, and the expression of hepatic FGF21 and adipocyte expression of adiponectin. The data suggest that SCI reduced signaling in adipocytes in response to FGF21 via KLB and FGFR1, and diminished adiponectin action on the liver through reduced hepatic AdipoR2 expression and decreased PPARα activation. In addition, SCI increased levels in liver of inflammatory cytokines, including IL-1β, IL-6 and CD11b, as well as in both iFAT and oFAT of TNFα mRNA, the changes were exacerbated by HFD suggesting that these adipose tissue deposits contribute to the state of low-grade chronic inflammation reported after SCI (58), which was associated with elevated liver expression of ALT mRNA and serum levels of ALT, indicative of hepatocellular injury. Moreover, SCI reduced insulin receptor in gastrocnemius muscle, and reduced mRNA levels for IRS-1 and Glut-4 in iFAT and oFAT, and IRS-1 in liver suggesting impaired insulin signaling pathway after SCI. Evidence of perturbations in regulation of synthesis of steroids, fatty acids and fatty acid metabolism after SCI included greatly elevated HMGCR mRNA expression and ACCα activity in liver after SCI. During administration of a HFD, serum FFA rose in SCI mice on HFD, but not control mice, suggesting impaired ability to store FFA as triglycerides in adipocytes or to use FFA as fuel in muscle and other tissues. FABP4 mRNA was elevated in liver of SCI-HFD mice which is notable because serum levels of the corresponding protein are linked to T2DM and other disorders (52). Taken together, the data indicate defects in metabolism of fats and carbohydrates in multiple tissues after SCI and implicate profound dysregulation of the FGF21 – adiponectin metabolic regulatory circuit as one key driver of these many perturbations.
Hepatic FGF21 expression is regulated by many factors that include nutrients, physical activity and medications (17, 59). Reasons that hepatic FGF21 expression is reduced by SCI are unclear. Because of the key role played by PPARα in activation of FGF21 transcription in liver, the reduced nuclear levels and transcriptional activity of PPARα provide insight with respect to one possible mechanism by which FGF21 mRNA levels are reduced in liver after SCI (60), but other possibilities exist. While it remains uncertain how exercise increases FGF21, the linkage between physical activity and FGF21 suggests that just as exercise raises levels of this hepatokine (61, 62), a sedentary lifestyle may lower them. The possibility should also be considered that cues from hepatic or adipocyte inflammation, which was present in both adipose tissues (iFAT and oFAT) and liver, as suggested by the elevated expression of mRNA levels for inflammatory cytokines, particularly for TNFα in the adipose tissue, and IL-1β, IL-6 and CD11b mRNA in the liver, could lead to reduced hepatic FGF21 expression. These data suggest that an increased focus on derangements in liver function as a key factor in impaired fat and carbohydrate metabolism after SCI should be considered.
The reduction in adipocyte expression of adiponectin mRNA and serum adiponectin level observed in spinal cord transected mice in the current study may be linked in part to the lower level of serum FGF21 observed after SCI; the data also suggest resistance to FGF21 actions based on lower expression of FGFR1 and KLB in iFAT and oFAT cells. While controversial, some evidence for FGF21 resistance has been presented (63). Other explanations cannot be excluded from the data available and include reduced capacity for PPARγ to activate adiponectin transcription as a result of local pro-inflammatory signals (49). Whatever the mechanisms responsible for reduced serum adiponectin after SCI might be, they were coupled with several molecular changes in liver that might be interpreted as indirect evidence of resistance to adiponectin action. These include lower levels of AdipoR2 mRNA and PPARα activity. While not extensively studied, the concept of adiponectin resistance has been proposed previously and some experimental support for its existence has been presented (64).
Lower expression in iFAT and oFAT of leptin and reduced serum leptin levels were also observed. These changes are consistent with a prior report of low serum leptin after a contusion SCI in rats (65). Implications of these changes are unclear but it should be noted that leptin has been implicated in multiple biological responses including of FGF21 action (33, 38). Regulation of leptin expression remains incompletely understood, but it is clear that leptin levels correspond to adipose tissue mass and are reduced by low calorie intake (66). Thus, the low mass of adipose tissue deposits in the SCI mice (44) observed in this investigation may explain, at least in partial, the low leptin levels observed.
Serum FFA levels were significantly elevated in HFD-fed SCI mice compared to SCI-ConD mice but not in sham-HFD mice. These observations suggest impaired ability of SCI mice to clear FFA from the circulation or to metabolize FFA such that they accumulate in blood. Elevated serum FFA levels have been shown to reduce tissue insulin sensitivity through multiple mechanisms that include reduced glucose uptake by skeletal muscle and increased TNFα release by liver Kupfer cells (67, 68), Importantly, elevated FFA levels are a risk factor for insulin resistance that may be independent of total fat mass (56). Elevations in serum FFA in SCI-HFD mice were associated with increased hepatic expression of FABP4. FABP4 is thought to have numerous functions that include its ability to bind free fatty acids and retinoids within cells, to participate in uptake of FFA from the extracellular space, and to route FFA to nuclear PPAR receptors to appropriately regulate the gene expression programs they control (69, 70); the implications of elevated hepatic FABP4 following SCI fed with a HFD are uncertain, but it is noteworthy that elevated serum levels of the protein encoded by this gene showed a positive correlation with high-sensitivity C-reactive protein (a measure of systemic inflammation), lipid parameters, and the homeostatic model assessment of insulin resistance (HOMA-IR) (56, 69–71). Taken together, these findings indicate that following SCI, mice were less tolerant of a HFD, and that this type of diet elicited several significant and deleterious changes.
Effects of SCI on the serum lipid profile have been evaluated in several other studies. Elevated serum triglycerides were observed at one month after SCI in T3-transected rats associated with increased visceral adiposity (72). In a separate study, serum FFA and glucose were elevated 42 days after a 200 kdyne contusion injury in rats (73). While extensive study of serum lipid profiles has been performed in patients with SCI (1), to date these studies have not characterized serum FFA levels.
An unexpected finding was the upregulation in SCI-ConD mice of HMGCR, a rate-limiting enzyme in the synthesis of cholesterol, possibly reflecting increased tissue demand, which might be expected during reparative responses in the spinal cord or to support neuroplasticity. In SCI patients, abnormalities of lipid metabolism include reduced HDL cholesterol (1, 2) and elevated triglycerides in response to a high fat challenge when compared to data for historical controls (74). In rats that were studied 1 month after thoracic level-10 transection, serum triglycerides, total and HDL cholesterol levels tended to be lower as compared to sham-operated controls, although this difference was not significant (72). Further study is needed to understand how SCI impacts metabolism of fats or how SCI perturbs the networks of adipokines and hepatokines that regulate them.
The data presented suggest that at 84 days after spinal transection, there is accumulation of tissue macrophages in the liver, as reflected by increased expression of inflammatory cytokines, including IL-1β, IL-6 and CD11b. There is also some evidence of inflammation in fatty tissue based on elevated expression of TNFα mRNA in both iFAT and oFAT, and increased IL-1β mRNA in oFAT. These alterations would be expected to stress hepatocytes, impair insulin action on hepatocellular regulation of glucose uptake and glucose output and, possibly, injure hepatocytes as well. Evidence of hepatocellular impairment, and possibly injury, was observed in post-SCI mice based on elevated circulating levels of ALT, as well as increased hepatic ALT expression. It is noteworthy that Kupffer cells, the resident hepatic macrophages, have been linked to NAFLD, a risk factor for insulin resistance (75), thus indirectly implicating the changes in CD11b to liver disease and insulin resistance after SCI. Elevated cytokine expression in fat deposits is also thought to be a risk factor for impaired glucose homeostasis (76, 77), suggesting that such alterations in adipose tissues observed in the current study may further contribute to impaired glucose handling and insulin action in SCI mice. This postulate is indirectly supported by findings of reduced expression of several key genes in insulin signaling in iFAT, oFAT, liver and gastrocnemius muscle. However, the data do not establish causal relationship between TNFα expression in adipose tissue and altered fat or carbohydrate metabolism. Also unclear is the cellular source of cytokines in adipose tissues, but prior studies indicate that infiltration of fat deposits by tissue macrophages is the primary explanation for such changes (76). Evidence of hepatic inflammation and hepatocellular injury that increases with time to 21 days after SCI has been observed in rats following a 200 kdyne contusion SCI. In the same study, elevations in serum ALT were observed in rats with cervical (C5), thoracic (T8) and lumbar (T12) contusion injuries (11). In that study, hepatic mRNA levels for IL-1ß increased at days 3 and 7 post-SCI while TNFα increased at 14 and 21 days, suggesting a time-dependent response. Hepatic lipids, assessed by Oil red O staining, were also increased at 14 days post injury (11). A more recent study from the same group found elevated hepatic CD11b at 6-weeks after a 200 kdyne injury at T8 that was associated with increased mRNA levels for several cytokines, including TNFα and IL-1ß, and increased hepatocellular lipids and iron levels (73). These findings are in agreement with the increased expression of CD11b in liver in the current study. However, no significant alteration in hepatic expression of cytokines was observed in the present study. Reasons for these discrepant findings are unclear but may include differences in time since injury, species and/or responses to contusion versus transection SCI.
It was previously reported that, in mice, spinal cord transection results in marked decreases in weights of iFAT and oFAT and that HFD did not increase weights of these fat deposits in SCI mice (44). Similarly, a reduction in total fat mass in rats after transection at thoracic level-4 has been reported and was associated with a small increase in metabolic rate and food intake (78). There is insufficient data to determine why the observed decreases in fat depots occurred. It has been argued that because body weights of the mice from which tissues were obtained for the current study increased over time after SCI that their net caloric intake exceeded metabolic demands. However, it remains unclear why gains in body weight were not associated with a return toward normal adipose tissue mass; possible explanations include impaired ability of adipocytes to take up and store lipids or, possibly, a loss of the normal replacement of aging adipocytes from the pool of adipocyte progenitors such that adipose tissues become depleted of healthy and fully functional adipocytes. Similarly, the reduced levels of PPARγ and ASC-1 observed in the current study could simply reflect low fat mass. Adipocytes undergo turnover whereby there is constant replenishment of aging or damaged adipocytes ones formed from a committed pool of progenitors (79). Thus, failure of HFD to promote gains in adipose tissue mass may indicate damage to adipocytes and/or inadequate replenishment of adipocytes from pools of progenitors. Of note, it was reported that FGF21 knockout in mice displayed disorders of adipose tissue due to defects in PPARγ signaling and PPARγ-dependent gene expression (34, 80). This report demonstrates that it is plausible that the reduced levels of FGFR1 and PPARγ activity observed in iFAT and oFAT could result in dysfunction of adipose tissue, low adipose tissue mass and impaired adiponectin expression and release.
It is now accepted that fat deposits are highly specialized in their functions depending on their location. oFAT is particularly important when considering the pathogenesis of insulin resistance and diabetes, perhaps because adipokines and free fatty acids liberated by oFAT are sent to the liver by the portal circulation. Unexpectedly then, in the present study, gene expression changes in iFAT and oFAT from SCI mice, and responses in SCI mice of these fat deposits to HFD were similar in magnitude and direction suggesting that physiological adaptations to SCI in mice overcame phenotypic differences related to localization of these fat deposits.
It was previously reported that HFD induced glucose intolerance in spinal cord transected mice at 84 days after injury and that fasting glucose was modestly increased in SCI-ConD mice at this time point (44). When these findings are taken together with the ability of FGF21 to improve insulin action, and low FGF21 observed in SCI in mice, it was of interest to examine insulin signaling pathways in tissues from these SCI mice. Whereas no reduction in IRS-1 or Glut-4 mRNA was observed in skeletal muscle, reduced levels of IRS-1 mRNA were observed in iFAT and oFAT, as well as liver, and these changes were not influenced by administration of a HFD. IRS-1 is critical to coupling insulin receptor and downstream signaling pathways while Glut4 represents the primary mechanism for insulin-induced glucose uptake. The findings suggest that, in the spinal cord transected mouse model at least, loss of insulin responsiveness of liver and fat deposits is an important mechanism leading to insulin resistance and diabetes. However, as discussed above, the data do not exclude a possible deterioration of function of adipose tissue as an explanation for the uniform decrease in insulin signaling pathways genes in adipose tissues. Further studies are necessary to better understand the respective roles of fat, liver and muscle in development of T2DM after SCI.
A limitation to be considered when interpreting our findings or considering their translation is that the approach employed did not directly test for physiological significance of lower FGF21 levels in the SCI mouse model used. Consequently, although it is attractive to posit that the changes observed downstream of serum FGF21 may be attributable to the lower serum FGF21 levels observed after spinal cord transection, this mechanistic linkage is yet to be proven. It should also be noted that whereas persons with SCI usually gain body fat mass, mice with spinal cord transection lost fat mass (44). This difference may limit how well changes in the spinal cord transection mouse model used in the current model reflect what occurs in individuals with SCI. Another limitation of these studies is that they evaluated only male mice; males were selected because male C57B6 mice are more sensitive to high-fat-diet-induced glucose intolerance than females. We expect that the pattern of changes in serum and hepatic FGF21 levels would be the same between males and females after SCI although some gender effects on the magnitude of changes cannot be excluded.
In summary, this study, the impact of SCI on expression of and signaling by FGF21 was investigated. Major findings of the study are that at 84 days after a complete spinal cord transection, hepatic FGF21 expression and serum FGF21 protein were reduced, associated with lower serum total and HMW adiponectin and leptin. A HFD raised serum FFA in SCI but not sham mice suggesting impaired ability for tissue to take up or metabolize fats. Prior studies demonstrated glucose intolerance in the SCI-HFD mice model that was used for these studies. Decreased expression for insulin signaling-related genes were observed in adipose tissue and liver but, surprisingly, not skeletal muscle. Taken together, reduced levels of FGF21 and adiponectin provide attractive mechanisms for the links between SCI and insulin resistance and T2DM. Moreover, the findings suggest key roles for perturbed function of adipose tissue and liver in the pathogenesis of T2DM after SCI. Further research will be needed to formally prove a causal relationship between abnormal levels of these proteins and carbohydrate and fat metabolism after SCI.
Data Availability Statement
The raw data supporting the conclusions of this article will be made available by the authors, without undue reservation.
Ethics Statement
The animal study was reviewed and approved by Institutional Animal Care and Use Committee at the James J. Peters VA Medical Center.
Author Contributions
X-HL designed the study, carried out the research, analyzed, interpreted the results, and wrote the manuscript. ZG designed the study, carried out the research and edited the manuscript. LH carried out the research (animal studies). JP carried out the research. DA carried out the research. WB designed the study, analyzed, interpreted the results, edited the manuscript. JY analyzed, interpreted the results, edited the manuscript. CC designed the study, analyzed, interpreted the results, and wrote/edited the manuscript. All authors contributed to the article and approved the submitted version.
Funding
This work was supported by the Department of Veterans Affairs Office of Research and Development, Rehabilitation Research and Development (RR&D) Service. SPiRE 1I21RX001273-01 and PECASE B9280-O to JY, CDA-2 IK2RX002781-01 to ZG, National Center for the Medical Consequences of Spinal Cord Injury (B2020C), and by resources provided by the North Florida/South Georgia Veterans Health System and James J. Peters Veterans Affairs Medical Center.
Disclaimer
The work reported herein does not represent the views of the US Department of Veterans Affairs or the US Government.
Conflict of Interest
The authors declare that the research was conducted in the absence of any commercial or financial relationships that could be construed as a potential conflict of interest.
Acknowledgments
We are very grateful to Dr. Kenneth Cusi for his very insightful discussions about the project.
Supplementary Material
The Supplementary Material for this article can be found online at: https://www.frontiersin.org/articles/10.3389/fendo.2021.668984/full#supplementary-material
Supplementary Figure 1 | Effect of SCI and HFD on Glut4 and IRS-1 mRNA Expression in Skeletal Muscle. Mice were fed with either ConD (White Bar) or HFD (Black Bar) after surgery. Total RNA was isolated from mouse gastrocnemius and subjected to PCR analysis. (A), IRS-1 mRNA expression; and (B), Glut4 mRNA expression. Data shown are mean values ± SEM (n = 5).
Supplementary Figure 2 | Effect of SCI and HFD on Hepatic TNFα and TNFα receptor mRNA Expression. Mice were fed with either ConD (White Bar) or HFD (Black Bar) after surgery. Total RNA was isolated from mouse liver and subjected to PCR. (A), TNFα mRNA expression; and (B), TNFα receptor mRNA expression. Data shown are mean values ± SEM (n = 5).
Supplementary Figure 3 | Effects of SCI and HFD on lipid and fatty acid metabolism-related gene expression in the liver - Mice were fed with either ConD (White Bar) or HFD (Black Bar) after surgery. Total RNA was isolated from mouse liver and subjected to PCR. (A), LDL receptor mRNA; (B), Liver X receptor-α (LXRα) mRNA; (C), SREBP1 mRNA; (D), UCP1 mRNA. Data shown are mean values ± SEM (n = 5).
Supplementary Table 1 | Summary of Metabolic Gene Expression after SCI with or without HFD.
References
1. Bauman WA, Nash MS. Endocrinology and Metabolism of Persons With Spinal Cord Injury. Chapter 20. In: Spinal Cord Med, 3rd edition. Springer Publishing Company (2018). p. 278–318. doi: 10.1891/9780826137753
2. Bauman WA, Spungen AM. Carbohydrate and Lipid Metabolism in Chronic SCI. J Spinal Cord Med (2001) 24(4):266–77. doi: 10.1080/10790268.2001. 11753584.
3. Gupta N, White KT, Sandford PR. Body Mass Index in SCI - a Retrospective Study. Spinal Cord (2006) 44(2):92–4. doi: 10.1038/sj.sc.3101790
4. Gorgey AS, Gater DR. Prevalence of Obesity After SCI. Top Spinal Cord Inj Rehabil (2007) 12:1–7. doi: 10.1310/sci1204-1
5. Gorgey AS, Dolbow DR, Dolbow JD, Khalil RK, Castillo C, Gater DR. Effects of SCI on Body Composition and Metabolic Profile-Part I. J Spinal Cord Med (2014) 37(6):693–702. doi: 10.1179/2045772314Y.0000000245
6. Sezer N, Akkus S, Ugurlu FG. Chronic Complications of Spinal Cord Injury. World J Orthop (2015) 6(1):24–33. doi: 10.5312/wjo.v6.i1.24
7. Gorgey AS, Gater DR. A Preliminary Report on the Effects of the Levels of SCI on the Association Between Central Adiposity and Metabolic Profile. PM R (2011) 3(5):440–6. doi: 10.1016/j.pmrj.2011.01.011
8. Weaver FM, Collins EG, Kurichi J, Miskevics S, Smith B, Rajan S, et al. Prevalence of Obesity and High Blood Pressure in Veterans With SCI and Disorders. Am J Phys Med Rehabil (2007) 86(1):22–9. doi: 10.1097/phm.0b013e31802b8937
9. Nash MS, Mendez AT. A Guideline-Driven Assessment of Need for Cardiovascular Disease Risk Intervention in Persons With Chronic Paraplegia. Arch Phys Med Rehabil (2007) 88(6):751–7. doi: 10.1016/j.apmr.2007.02.031
10. Sun X, Jones ZB, Chen X, Zhou L, So K-F, Ren Y. Multiple Organ Dysfunction and Systemic Inflammation After Spinal Cord Injury: A Complex Relationship. J Neuroinflammation (2016) 13(1):260–71. doi: 10.1186/s12974-016-0736-y
11. Sauerbeck AD, Laws JL, Bandaru VV, Popovich PG, Haughey NJ, McTigue DM. SCI Causes Chronic Liver Pathology in Rats. J Neurotrauma (2015) 32(3):159–69. doi: 10.1089/neu.2014.3497
12. Lavela SL, Weaver FM, Goldstein B, Chen K, Miskevice S, Rajan S, et al. Diabetes in Individuals With SCI or Disorders. J Spinal Cord Med (2006) 29(4):387–95. doi: 10.1080/10790268.2006.11753887
13. Sipski ML, Estores IM, Alexander CJ, Guo X, Chandralapaty SK. Lack of Justification for Routine Abdominal Ultrasonography in Patients With Chronic Spinal Cord Injury. J Rehabil Res Dev (2004) 41(1):101–8. doi: 10.1682/jrrd.2004.01.0101
14. Shin JC, Park C, Ki SH, Yang EJ, Kim EJ, Rha DW. Abdominal Ultrasonography Findings in Patients With Spinal Cord Injury in Korea. J Korean Med Sci (2006) 21(5):927–31. doi: 10.3346/jkms.2006.21.5.927
15. Nardone R, Holler Y, Storti M, Lochner P, Tezzon F, Golaszewski S, et al. Spinal Cord Involvement in Patients With Cirrhosis. World J Gastroenterol (2014) 20(6):2578–85. doi: 10.3748/wjg.v20.i10.2578
16. Durga A, Sepahpanah F, Regozzi M, Hastings J, Crane DA. Prevalence of Testosterone Deficiency After Spinal Cord Injury. PM R (2011) 3(10):929–32. doi: 10.1016/j.pmrj.2011.07.008
17. Staiger H, Keuper M, Berti L, Hrabe de Angelis M, Haring H-U. Fibroblast Growth Factor 21-Metabolic Role in Mice and Men. Endocr Rev (2017) 38(5):468–88. doi: 10.1210/er.2017-00016
18. Potthoff MJ. FGF21 and Metabolic Disease in 2016: A New Frontier in FGF21 Biology. Nat Rev Endocrinol (2017) 13(2):74–6. doi: 10.1038/nrendo.2016.206
19. Schlein C, Talukdar S, Heine M, Fischer AW, Krott LM, Nilsson SK, et al. FGF21 Lowers Plasma TG by Accelerating Lipoprotein Catabolism in White and Brown Adipose Tissues. Cell Metab (2016) 23(3):441–53. doi: 10.1016/j.cmet.2016.01.006
20. Gimeno RE, Moller DE. FGF21-Based Pharmacotherapy – Potential Utility for Metabolic Disorders. Trends Endocrinol Metab (2014) 25(6):303–11. doi: 10.1016/j.tem.2014.03.001
21. Talukdar S, Zhou Y, Li D, Rossulek M, Dong J, Somayaji V, et al. A Long-Acting FGF21 Molecular, PF-05231023, Decreases Body Weight and Improves Lipid Profile in Non-Human Primates and Type 2 Diabetic Subjects. Cell Metab (2016) 23(3):427–40. doi: 10.1016/j.cmet.2016.02.001
22. Kharitonenkov A, DiMarchi R. FGF21 Revolutions: Recent Advances Illuminating FGF21 Biology and Medicinal Properties. Trends Endocrinol Metab (2015) 26(11):608–17. doi: 10.1016/j.tem.2015.09.007
23. Reitman ML. FGF21 Mimetics Shows Therapeutic Promise. Cell Metab (2013) 18(3):307–9. doi: 10.1016/j.cmet.2013.08.014
24. Kharitonenkov A, Shiyanova TL, Koester A, Ford AM, Micanovic R, Galbreath EJ, et al. FGF21 as a Novel Metabolic Regulator. J Clin Invest (2005) 115:1627–35. doi: 10.1172/JCI23606
25. Maratos-Flier E. Fatty Liver and FGF21 Physiology. Exp Cell Res (2017) 360(1):2–5. doi: 10.1016/j.yexcr.2017.05.006
26. Fisher FM, Estall JL, Adams AC, Antonellis PJ, Bina HA, Flier JS, et al. Integrated Regulation of Hepatic Metabolism by FGF21 In Vivo. Endocrinology (2011) 152(8):2996–3004. doi: 10.1210/en.2011-0281
27. Inagaki T. Research Perspective on the Regulation and Physiological Function of FGF21 and Its Association With NAFLD. Front Endocrinol (2015) 6:147. eCollection 2015. doi: 10.3389/fendo.2015.00147. eCollection 2015
28. Planavila A, Redondo I, Hondares E, Vinciguerra M, Munts C, Iglesias R, et al. FGF21 Protects Against Cardiac Hypertrophy in Mice. Nat Commun (2013) 4:2019. doi: 10.1038/ncomms3019
29. Wang WF, Li SM, Ren GP, Zheng W, Lu YJ, Yu YH, et al. Recombinant Murine FGF21 Ameliorates Obesity-Related Inflammation in MSG-Induced Obesity Rats. Endocrinology (2015) 49(1):119–29. doi: 10.1007/s12020-014-0433-5. 2015.
30. Montagner A, Polizzi A, Fouche E, Ducheix S, Lippi Y, Lasserre F, et al. Liver PPARα is Crucial for Whole-Body Fatty Acid Homeostasis and is Protective Against NAFLD. Gut (2016) 65(7):1202–14. doi: 10.1136/gutjnl-2015-310798
31. Ding X, Boney-Montoya J, Owen BM, Bookout AL, Coate KC, Mangelsdorf DJ, et al. β-Klotho is Required for FGF21 Effects on Growth and Metabolism. Cell Metab (2012) 16(3):387–93. doi: 10.1016/j.cmet.2012.08.002
32. Kurosu H, Choi M, Ogawa Y, Dickson AS, Goetz R, Eliseenkova AV, et al. Tissue-Specific Expression of β-Klotho and FGF Receptor Isoforms Determines Metabolic Activity of FGF19 and FGF21. J Biol Chem (2007) 282(37):26687–95. doi: 10.1074/jbcM704165200
33. Veniant MM, Hale C, Helmering J, Chen MM, Stanislaus S, Busby J, et al. FGF21 Promotes Metabolic Homeostasis Via White Adipose and Leptin in Mice. PloS One (2012) 7(7):e40164. doi: 10.1371/journal.pone.0040164
34. Li H, Wu G, Fang Q, Zhang M, Hui X, Sheng B, et al. Fibroblast Growth Factor 21 Increases Insulin Sensitivity Through Specific Expansion of Subcutaneous Fat. Nat Commun (2018) 9:272. doi: 10.1038/s41467-017-02677-9
35. Lin Z, Tian H, Lam KS, Lin S, Hoo RC, Konishi M, et al. Adiponectin Mediates the Metabolic Effects of FGF21 on Glucose Homeostasis and Insulin Sensitivity in Mice. Cell Metab (2013) 17(5):779–89. doi: 10.1016/j.cmet.2013.04.005
36. Hui X, Feng T, Liu Q, Gao Y, Xu A. The FGF21-Adiponectin Axis in Controlling Energy and Vascular Homeostasis. J Mol Cell Biol (2016) 8(2):110–19. doi: 10.1093/jmcb/mjw013
37. Yamauchi T, Kamon J, Ito Y, Tsuchida A, Yokomizo T, Kita S, et al. Cloning of Adiponectin Receptors That Mediate Antidiabetic Metabolic Effects. Nature (2003) 423(6941):762–9. doi: 10.1038/nature01705
38. Asrih M, Veyrat-Durebex C, Poher AL, Lyautey J, Rohner-Jeanrenaud F, Jornayvaz FR. Leptin as a Potential Regulator of FGF21. Cell Physiol Biochem (2016) 38(3):1218–25. doi: 10.1159/000443070
39. Stenholm S, Harris TB, Rantanen T, Visser M, Kritchevsky SB, Ferrucci L. Sarcopenia Obesity–Definition, Etiology and Consequences. Curr Opin Clin Nutr Metab Care (2008) 11(6):693–700. doi: 10.1097/MCO.0b013e328312c37d
40. Farkas GJ, Gorgey AS, Dolbow DR, Berg AS, Gater DR. Caloric Intake Relative to Total Daily Energy Expenditure Using a Spinal Cord Injury - Specific Correction Factor: an Analysis by Level of Injury. Am J Phys Med Rehabil (2019) 98(11):947–52. doi: 10.1097/PHM.0000000000001166
41. Farkas GJ, Pitot MA, Berg AS, Gater DR. Nutritional Status in Chronic Spinal Cord Injury: A Systematic Review and Meta-Analysis. Spinal Cord (2019) 57(1):3–17. doi: 10.1038/s41393-018-0218-4
42. Pellicane AJ, Millis SR, Zimmerman SE, Roth EJ. Calorie and Protein Intake in Acute Rehabilitation Inpatients With Traumatic Spinal Cord Injury Versus Other Diagnoses. Top Spinal Cord Inj Rehabil (2013) 19(3):229–35. doi: 10.1310/sci1903-229
43. Silveira SL, Winter LL, Clark R, Ledoux T, Robinson-Whelen S. Baseline Dietary Intake of Individuals With Spinal Cord Injury Who are Overweight or Obese. J Acad Nutr Diet (2019) 119(2):301–9. doi: 10.1016/j.jand.2018.08.153
44. Graham ZA, Liu XH, Harlow L, Pan J, Azulai D, Tawfeek HA, et al. Effects of a High-Fat Diet on Tissue Mass, Bone, and Glucose Tolerance After Chronic Complete Spinal Cord Transection in Male Mice. Neurotrauma Rep (2020) 1.1:17–23. doi: 10.1089/neur.2020.0014
45. Liu XH, Wu Y, Yao S, Levine AC, Kirschenbaum A, Collier L, et al. Androgens Upregulate Transcription of the Notch Inhibitor Numb in C2C12 Myoblasts Via Wnt/β-Catenin Signaling to T Cell Factor Elements in the Numb Promoter. J Biol Chem (2013) 28(25):17990–8. doi: 10.1074/jbc.M113.478487
46. Markan KR, Naber MC, Ameka MK, Anderegg MD, Mangeldorf DJ, Kliewer SA, et al. Circulating FGF21 is Liver Derived and Enhances Glucose Uptake During Refeeding and Overfeeding. Diabetes (2014) 63(12):4057–63. doi: 10.2337/db14-0595
47. Patel P, Abate N. Body Fat Distribution and Insulin Resistance. Nutrients (2013) 5(6):2019–27. doi: 10.3390/nu5062019
48. van Andel M, Heijboer AC, Drent ML. Adiponectin and Its Isoforms in Pathophysiology. Adv Clin Chem (2018) 85:115–47. doi: 10.1016/bs.acc.2018.02.007
49. Chawla A, Schwarz EJ, Dimaculangan DD, Lazar MA. Peroxisome Proliferator-Activated Receptor (PPAR) Gamma: Adipose-Predominant Expression and Induction Early in Adipocyte Differentiation. Endocrinology (1994) 135(2):798–800. doi: 10.1210/endo.135.2.8033830
50. Ussar S, Lee KY, Dankel SN, Boucher J, Haering MF, Kleinridders A, et al. ASC-1, PAT2, and P2RX5 are Cell Surface Markers of White, Beige, and Brown Adipocytes. Sci Transl Med (2014) 6(247):247ra103. doi: 10.1126/scitranslmed.3008490
51. Yamauchi T, Kadowaki T. Physiological and Pathophysiological Roles of Adiponectin and Receptors in the Integrated Regulation of Metabolic and Cardiovascular Diseases. Int J Obes (2008) 32 Suppl 7:S13–8. doi: 10.1038/ijo.2008.233
52. Kersten S, Stienstra R. The Role and Regulation of the Peroxisome Proliferator Activated Receptor Alpha (PPARα) in Human Liver. Biochimie (2017) 136:75–84. doi: 10.1016/j.biochi.2016.12.019
53. Tamasi V, Miller KKM, Ripp SL, Vila E, Geoghagen TE, Prough RA. Modulation of Receptor Phosphorylation Contributes to Activation of PPARα by Dehydro-Epiandrosterone and Other Peroxisome Proliferators. Mol Pharmacol (2007) 73(3):968–76. doi: 10.1124/mol.107.036780
54. Inagaki T, Dutchak P, Zhao G, Ding X, Gautron L, Parameswara V, et al. Endocrine Regulation of the Fasting Response by PPARα-Mediated Induction of FGF21. Cell Metab (2007) 5(6):415–25. doi: 10.1016/j.cmet.2007.05.003
55. Park SH, Gammon SR, Knippers JD, Paulsen SR, Rubink DS, Winder WW. Phosphorylation-Activity Relationships of AMPK and Acetyl-Coa Carboxylase in Muscle. J Appl Physiol (2002) 92(6):2475–82. doi: 10.1152/japplphysiol.00071.2002
56. Miller MR, Pereira RI, Langefeld CD, Lorenzo C, Rotter JI, Chen IY, et al. Levels of Free Fatty Acids (FFA) are Associated With Insulin Resistance But Do Not Explain the Relationship Between Adiposity and Insulin Resistance in Hispanic Americans: The IRAS Family Study. J Clin Endocrinol Metab (2012) 97(9):3285–91. doi: 10.1210/jc.2012-1318
57. Furuhashi M, Saitoh S, Shimamoto K, Miura T. FABP4: Pathophysiological Insights and Potent Clinical Biomarker of Metabolic and Cardiovascular Disease. Clin Med Insights Cardiol (2015) 8(Suppl 3):23–33. doi: 10.4137/CMCS17067 eCollection 2014
58. Schwab JM, Zhang Y, Kopp MA, Brommer B, Popovich PG. The Paradox of Chronic Neuroinflammation, Systemic Immune Suppression, Autoimmunity After Traumatic Chronic Spinal Cord Injury. Exp Neurol (2014) 258:121–9. doi: 10.1016/j.expneurol.2014.04.023
59. Kliewer SA, Mangelsdorf DJ. A Dozen Years of Discovery: Insights Into the Physiology and Pharmacology of FGF21. Cell Metab (2019) 29(2):246–53. doi: 10.1016/j.cmety.2019.01.004
60. Badman MK, Pissios P, Kennedy AR, Koukos G, Flier JS, Maratos-Flier E. Hepatic FGF21 is Regulated by PPARα and is a Key Mediator of Hepatic Lipid Metabolism in Ketotic States. Cell Metab (2007) 5(6):426–37. doi: 10.1016/j.cmet.2007.05.002
61. Weigert C, Hoene M, Plomgaard P. Hepatokines - a Novel Group of Exercise Factors. Pflugers Arch (2019) 471(3):383–96. doi: 10.1007/s00424-018-2216-y
62. Loyd C, Magrisso IJ, Haas M, Balusu S, Krishna R, Itoh N, et al. FGF21 is Required for Beneficial Effects of Exercise in HF Feeding. J Appl Physiol (2016) 121(3):687–98. doi: 10.1152/japplphysiol.00456.2016
63. Fisher FM, Chui PC, Antonellis PJ, Bina HA, Kharitonenkov A, Flier JS. Obesity is a Fibroblast Growth Factor 21 (FGF21)-Resistant State. Diabetes (2010) 59(11):2781–9. doi: 10.2337/db10-0193
64. Mullen KL, Pritchard J, Ritchie I, Snook LA, Chabowski A, Bonen A. Adiponectin Resistance Precedes the Accumulation of Skeletal Muscle Lipid and Insulin Resistance in High-Fat-Fed Rats. Am J Physiol Regul Integr Comp Physiol (2009) 296(2):R243–51. doi: 10.1152/ajpregu.90774.2008
65. Otzel DM, Conover CF, Ye F, Phillips EG, Taylor Bassett T, Wnek RD, et al. Longitudinal Examination of Bone Loss in Male Rats After Moderate-Severe Contusion Spinal Cord Injury. Calcif Tissue Int (2019) 104(1):79–91. doi: 10.1007/s00223-018-0471-8
66. Münzberg H, Heymsfield SB. New Insights Into the Regulation of Leptin Gene Expression. Cell Metab (2019) 29(5):1013–4. doi: 10.1016/j.cmet.2019.04.005
67. Boden G, She P, Mozzoli M, Cheung P, Gumireddy K, Reddy P, et al. Free Fatty Acids Produce Insulin Resistance and Activate the Proinflammatory Nuclear Factor-Kappa B Pathway in Rat Liver. Diabetes (2005) 54(12):3458–65. doi: 10.2337/diabetes.54.12.3458
68. Roden M, Price TB, Perseghin G, Peterson KF, Rothman DL, Cline GW, et al. Mechanism of Free Fatty Acid-Induced Insulin Resistance in Humans. J Clin Invest (1996) 97(12):2859–65. doi: 10.1172/JCI118742
69. Smathers R, Petersen DR. The Human Fatty Acid-Binding Protein Family: Evolutionary Divergence and Function. Hum Genomics (2011) 5(3):170–91. doi: 10.1186/1479-7364-5-3-17071
70. Hotamisligil GS, d Bernlohr DA. Metabolic Functions of FABPs – Mechanisms and Therapeutic Implications. Nat Rev Endocrinol (2015) 11(10):592–605. doi: 10.1038/nrendo.2015.122
71. Ishimura S, Furuhashi M, Watanabe Y, Hoshina K, Fuseya T, Mita T, et al. Circulating Levels of Fatty Acid-Binding Protein Family and Metabolic Phenotype in the General Population. PloS One (2013) 8(11):e81318. doi: 10.1371/journal.pone.0081318.eCollection 2013
72. Inskip J, Plunet W, Ramer L, Ramsey JB, Yung A, Kozlowski P, et al. Cardiometabolic Risk Factors in Experimental Spinal Cord Injury. J Neurotrauma (2010) 27(1):275–85. doi: 10.1089/neu.2009.1064
73. Goodus MT, Sauerbeck AD, Popovich PG, Bruno RS, McTigue DM. Dietary Green Tea Extract Prior to Spinal Cord Injury Prevents Hepatic Iron Overload But Does Not Improve Chronic Hepatic and Spinal Cord Pathology in Rats. J Neurotrauma (2018) 35(24):2872–82. doi: 10.1089/neu.2018.5771
74. Nash MS, DeGroot J, Martinez-Arizala A, Mendez AJ. Evidence for an Exaggerated Postprandial Lipemia in Chronic Paraplegia. J Spinal Cord Med (2005) 28(4):320–25. doi: 10.1080/10790268.2005.11753827
75. Tosello-Trampont A-C, Landes SG, Nguyen V, Novobrantseva TI, Hahn YS. Kuppfer Cells Trigger Nonalcoholic Steatohepatitis Development in Diet-Induced Mouse Model Through Tumor Necrosis Factor-a Production. J Biol Chem (2012) 287(48):40161–72. doi: 10.1074/jbc.M112.417014
76. Rosen ED, Spiegelman BM. What We Talk About When We Talk About Fat. Cell (2014) 156(1-2):20–44. doi: 10.1016/j.cell.2013.12.012
77. Goossens GH. The Metabolic Phenotype in Obesity: Fat Mass, Body Fat Distribution, and Adipose Tissue Function. Obes Facts (2017) 10(3):207–15. doi: 10.1159/000471488
78. Primeaux SD, Tong M, Holmes GM. Effects of Chronic Spinal Cord Injury on Body Weight and Body Composition in Rats Fed a Standard Chow Diet. Am J Physiol Regul Integr Comp Physiol (2007) 293(3):R1102–9. doi: 10.1016/bs.acc.2018.02.007
79. Cohen P, Spiegelman BM. Cell Biology of Fat Storage. Mol Biol Cell (2016) 27(16):2523–7. doi: 10.1091/mbc.E15-10-0749
Keywords: FGF21, spinal cord injury, adiponectin, high fat diet, liver, adipose tissue, metabolism, diabetes
Citation: Liu X-H, Graham ZA, Harlow L, Pan J, Azulai D, Bauman WA, Yarrow J and Cardozo CP (2021) Spinal Cord Injury Reduces Serum Levels of Fibroblast Growth Factor-21 and Impairs Its Signaling Pathways in Liver and Adipose Tissue in Mice. Front. Endocrinol. 12:668984. doi: 10.3389/fendo.2021.668984
Received: 17 February 2021; Accepted: 23 April 2021;
Published: 11 May 2021.
Edited by:
T John Wu, Uniformed Services University of the Health Sciences, United StatesReviewed by:
Melinda E. Wilson, University of Kentucky HealthCare, United StatesYan Chen, University of Chinese Academy of Sciences, China
Copyright © 2021 Liu, Graham, Harlow, Pan, Azulai, Bauman, Yarrow and Cardozo. This is an open-access article distributed under the terms of the Creative Commons Attribution License (CC BY). The use, distribution or reproduction in other forums is permitted, provided the original author(s) and the copyright owner(s) are credited and that the original publication in this journal is cited, in accordance with accepted academic practice. No use, distribution or reproduction is permitted which does not comply with these terms.
*Correspondence: Xin-Hua Liu, eGluLWh1YS5saXVAdmEuZ292