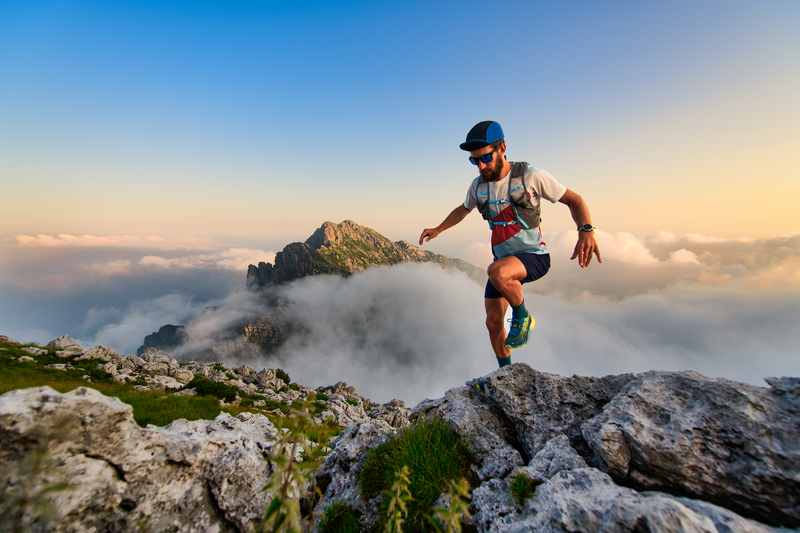
95% of researchers rate our articles as excellent or good
Learn more about the work of our research integrity team to safeguard the quality of each article we publish.
Find out more
MINI REVIEW article
Front. Endocrinol. , 14 April 2021
Sec. Gut Endocrinology
Volume 12 - 2021 | https://doi.org/10.3389/fendo.2021.668012
This article is part of the Research Topic Proglucagon-Derived Peptides View all 25 articles
Glucagon-like peptide-1 (GLP-1) is a gut hormone mainly produced in the intestinal epithelial endocrine L cells, involved in maintaining glucose homeostasis. The use of GLP-1 analogous and dipeptidyl peptidase-IV (DPP-IV) inhibitors is well-established in Type 2 Diabetes. The efficacy of these therapies is related to the activation of GLP-1 receptor (GLP-1R), which is widely expressed in several tissues. Therefore, GLP-1 is of great clinical interest not only for its actions at the level of the beta cells, but also for the extra-pancreatic effects. Activation of GLP-1R results in intracellular signaling that is regulated by availability of downstream molecules and receptor internalization. It has been shown that GLP-1R co-localizes with caveolin-1, the main component of caveolae, small invagination of the plasma membrane, which are involved in controlling receptor activity by assembling signaling complexes and regulating receptor trafficking. The aim of this review is to outline the important role of caveolin-1 in mediating biological effects of GLP-1 and its analogous.
The biological effects of the Glucagon-like peptide-1 (GLP-1) are mediated by binding to its receptor, GLP-1R, a specific seven-transmembrane receptor guanine nucleotide-binding protein (G-protein) coupled receptor (GPCR) (1). GLP1 binding to GLP-1R leads to activation of intracellular signaling pathways that take part to the regulation of glucose homeostasis. The GLP-1R localizes in caveolae (2), a subset of lipid rafts on the plasma membrane structured as flask-shaped invagination of about 50 to 100 nm, composed of Caveolins and Cavins (3, 4). Caveolae regulate several cellular processes, including protein endocytosis, intracellular trafficking, cholesterol homeostasis, and signal transduction (5–7). In particular, Caveolin 1 (Cav-1) binds several signaling and structural proteins through the caveolin-binding motifs, a conserved sequence enriched with aromatic residues [ΦXΦXXXXΦ, ΦXXXXΦXXΦ, and ΦXΦXXXXΦXXΦ (Φ = aromatic residue, X = any amino acid)], found in a lot of proteins, including the GLP-1 receptor.
The direct interaction between GLP-1R and Cav-1 regulates the proper targeting of the GLP-1R to the plasma membrane, the receptor trafficking, and the activation of the intracellular signaling pathway. However, Cav-1 is also a multifunctional platform able to recruit several signaling molecules.
In this review we highlight the importance of Cav-1 in mediating GLP-1 action. Moreover, we speculated about the involvement of Cav-1 in regulating GLP-1 signaling at the level of the G proteins, showing that Cav-1 might modulate signal transduction by influencing not only the trafficking of GLP-1R, but also that of the signaling proteins. Finally, we hypothesize that Cav-1 may regulate GLP-1 action binding both GLP-1R and β-arrestin-1, and modulating the activity of Dipeptidyl Peptidase (DPP)-IV.
The principal protein of caveolae is Cav-1, a 22- to 24-kDa integral membrane protein with a hairpin-like conformation ubiquitously expressed in many different tissues, except striated muscle in which Caveolin 3 is highly expressed (6, 8). The hairpin loop is transmembrane, whereas the amino- and carboxy-terminal domains are oriented towards the cytoplasm. In particular, the juxtamembrane domain in the N-terminal region of the protein acts as a scaffolding protein and, besides driving caveolae formation through heterooligomeric complex with Caveolin-2 and PTRF-cavin (9), interacts with a variety of signaling molecules such as G-proteins, H-Ras, Fyn, Erk-2, Src family tyrosine kinases, and so on (10, 11). Moreover, Cav-1 directly interact with insulin and IGF1 receptor and their principal substrate IRS-1 supporting a role in metabolic regulation (12, 13).
GLP-1 is an incretin hormone derived from the proglucagon gene and secreted by the intestinal L cell in response to food ingestion to maintain glucose homeostasis (14). The secretion of GLP-1 is reduced in Type 2 Diabetes Patients (15–17). Therefore, therapies with GLP-1 receptor agonists and DPP-4 inhibitors are largely employed to restore incretin action in T2D. The improvement of pancreatic beta cell dysfunction and the protective role of GLP-1 against oxidative stress has been described both in vitro and in vivo (17–21). These biological effects of GLP-1 are selectively mediated by activation of GLP-1R, that leads to various intracellular signaling pathways mainly described in pancreatic beta-cells (22). Briefly, GLP-1 binding to its receptor triggers G-protein activation which leads to cAMP production, calcium mobilization and phosphorylation of extracellular signal-regulated kinases (ERK). In addition, GLP-1R is expressed also in peripheral tissues, including the central and peripheral nervous systems, heart, kidney, lung, gastrointestinal tract and retinal pigment epithelium (23, 24). Therefore, the great clinical interest on GLP-1 for the management of type 2 diabetes is due not only to its actions at the level of the beta cells, as well in the peripheral tissues.
The GLP-1R sequence contains a classical caveolin-1 binding motif within the second intracellular loop (247-EGVYLYTLLAFSVF-260) (Uniprot) (1, 25). The first evidence that GLP-1R directly interacts with Cav-1 has been reported by Syme et al. in 2006 (2). They showed that Cav-1 immunoprecipitated with GLP-1R, and that, on the contrary, mutation of the two tyrosine residues Y250 and Y252 to alanine in the GLP-1R amino acidic sequence abrogated the interaction of GLP-1R with Cav-1.
Direct interaction with Cav-1 is required for internalization of receptors in caveolae and also for trafficking of GLP-1R (26–28). For instance, GLP-1R containing Y250/252A mutations is trapped in intracellular compartments, and not localized on the cell surface (2). On the other contrary, GLP-1R is not internalized after agonist stimulation in cells expressing P132L-Cav-1, a mutated form of Cav-1 that results in misfolded oligomers which accumulate within the Golgi complex (29), and in cells treated with caveolae inhibitors (2).
The subcellular localization of a receptor is an important mechanism that regulates signaling specificity. Consequently, in case of receptors containing GLP-1 Y250/252A mutations, which prevent the localization of GLP-1R on the plasmamembrane and the binding of GLP-1, the intracellular signaling is lost (2). On the contrary, defective internalization may lead to sustained activation of GLP-1R–mediated signaling (30). Therefore, the interaction between GLP-1R and Cav-1 is necessary not only for receptor trafficking to the cell membrane, but also for activation of the intracellular signaling pathway.
The fate of a receptor after activation is another important mechanism to control its signaling capacity: GLP-1R undergoes agonist-mediated endocytosis, which may lead either to recycle the receptor back to the plasma membrane or to degradative pathway (31–33). Considering that Cav-1 is required for internalization of GLP-1R after agonist stimulation (34), it is conceivable that Cav-1 may affect also the fate of GLP-1R determining its recycling or degradation.
GLP-1R internalization is also important for the spatiotemporal control of signaling. GLP-1R agonists exerted different effects on regulatory mechanisms that control the duration of receptor activation, such as desensitization and internalization (32). In particular, GLP-1 and exendin-4 are 10-fold more potent to cause GLP-1R internalization than liraglutide, but GLP-1 causes the receptor to recycle two to three times faster than when stimulated with exendin-4 or liraglutide (32). The rate at which GLP-1 and its analogs induce GLP-1R internalization may be affected by Cav-1. Indeed, Cav-1 selectively recruits and organizes proteins and lipids in membranes, therefore the different effects of GLP-1 agonists on GLP-1R activation may be due to the various compartmentalization of signaling molecules in caveolae. For instance, caveolae regulate many GPCR signaling pathways through a selective compartmentalization of G proteins, and their downstream targets in membrane microdomains (35, 36). In pancreatic beta cells GLP-1 can activate both Gαs and Gαq subunits. The Gαs pathway activates adenylyl cyclase leading to increase formation of cAMP; whereas the Gαq pathway leads to increase cytoplasmic concentration of Ca2+ (37). It is well documented that Gαs and adenylyl cyclase are localized in caveolae (38, 39), and that Gαs is quickly internalized after activation (40). Gαs internalization attenuates Gαs/adenylyl cyclase signaling and depends on Cav-1 (41, 42). Depletion of Cav-1 prevented agonist-induced internalization of Gαs in C6 glioma cells, with consequent increment of the Gαs/adenylyl cyclase signaling (42). On the other hand, depletion of Cav-1 inhibits Gαq-mediated signaling in C6 cells (43). It has been reported that Gαq is associated with Cav-1 at both plasma membrane and cytosolic level (44), and that Cav-1 binds preferentially to Gαq in its activated state, thus prolonging its activation (45). Interestingly, the activation of the Gαq pathway is required for agonist-induced GLP-1R internalization (34). Taken together these evidence suggest that Cav-1 may regulate GLP-1 action by controlling the duration of G-protein signals.
Activation of Gαs and Gαq pathways results in the translocation and exocytosis of insulin-containing secretory granules in pancreatic beta cells by increasing cytoplasmic concentration of Ca2+ through 2 mechanisms: closure of ATP-sensitive potassium channel (KATP) which leads to calcium influx via voltage-gated Ca2+ channels; and release of Ca2+ from intracellular Ca2+ stores (37). It has been reported that the KATP channel activity depends on the spatial organization of signaling pathways, requiring co-localization with adenylyl cyclase, and that the integrity of caveolae is important for adenylyl cyclase-mediated channel modulation (46). We previously demonstrated that the Kir6.2 subunit of the KATP channels is associated to Cav-1 in the pancreatic beta cell line βTC-6, and that depletion of Cav-1 reduced glucose induced insulin secretion (47). These evidence support the hypothesis that Cav-1 is also essential in GLP-1–induced insulin secretion by maintaining the correct regulation of KATP channels.
GLP-1 action is also mediated by β-arrestin-1 (48), a scaffolding protein that mediates receptor desensitization, receptor internalization, and links GPCRs to downstream pathways (49). Indeed, β-arrestin-1 knockdown attenuated GLP-1 signaling and impaired both glucose- and GLP-1–induced insulin secretion in INS-1 pancreatic beta cells (48). Interestingly, β-arrestin-1 directly interacts with both GLP-1R and Cav-1 (48, 50), therefore Cav-1, GLP-1R and β-arrestin-1 may form a microdomain implicated in regulating GLP-1 action. Depletion of β-arrestin-1 did not affect GLP-1R agonist-induced GLP-1 R internalization (48), suggesting that β-arrestin-1 acts downstream to Cav-1.
GLP-1 exerts also proliferative and antiapoptotic, effects activating ERK and AKT signaling pathways (17, 51). Interestingly, Cav-1 depletion protects pancreatic β cells against palmitate-induced dysfunction and apoptosis enhancing activities of Akt and ERK1/2. Considering that Cav-1 is required for internalization of GLP-1R, and that inhibition of GLP-1R internalization prolongs ERK activity (30), these findings suggest that Cav-1 depletion may affect ERK activity by regulating cellular trafficking of GLP-1R. On the other hand, β-arrestin-1 depletion decreased ERK phosphorylation (48), confirming that β-arrestin-1 acts downstream to Cav-1.
It is well known that GLP-1 has a short plasma half-life (1–7 min) due to quickly degradation by Dipeptidyl Peptidase (DPP)-IV/CD26, which is an integral membrane protein widely expressed on cell surfaces and, after cleavage, present in the circulation as “soluble” DPP-IV” (52). Interestingly, Cav-1 directly interacts with DPP-IV by binding to its serine catalytic site (53, 54). Furthermore, gene knockdown of Cav-1 suppressed the anti-inflammatory effects of the DPP-4 inhibitor teneligliptin in human monocyte/macrophage U937, showing that teneligliptin needs to bind Cav-1 to exert its effects (54). These evidence suggest that Cav-1 may affect lifespan of GLP-1 by regulating the activity of DPP-IV and of its inhibitors.
Although it is well recognized that Cav-1 is involved in all the steps that regulate GLP-1 function, these aspects are not fully elucidated. Considering the knowledge in the literature, we can conclude that: 1) the interaction between GLP-1R and Cav-1 is necessary not only for receptor trafficking to the cell membrane, but also for activation of the intracellular signaling pathway; 2) Cav-1 may affect the fate of GLP-1R; 3) Cav-1 may regulate GLP-1 action by controlling the duration of G-proteins signals; 4) Cav-1 may be a physical link between GLP-1R and β-arrestin-1; 5) Cav-1 may affect lifespan of GLP-1 (Figure 1).
All authors listed have made a substantial, direct, and intellectual contribution to the work and approved it for publication.
The authors declare that the research was conducted in the absence of any commercial or financial relationships that could be construed as a potential conflict of interest.
1. Brubaker PL, Drucker DJ. Structure-function of the glucagon receptor family of G protein-coupled receptors: the glucagon, GIP, GLP-1, and GLP-2 receptors. Recept Channels (2002) 8(3-4):179–88. doi: 10.3109/10606820213687
2. Syme CA, Zhang L, Bisello A. Caveolin-1 regulates cellular trafficking and function of the glucagon-like Peptide 1 receptor. Mol Endocrinol (2006) 20(12):3400–11. doi: 10.1210/me.2006-0178
3. Li XA, Everson WV, Smart EJ. Caveolae, lipid rafts, and vascular disease. Trends Cardiovasc Med (2005) 15(3):92–6. doi: 10.1016/j.tcm.2005.04.001
4. Kovtun O, Tillu VA, Ariotti N, Parton RG, Collins BM. Cavin family proteins and the assembly of caveolae. J Cell Sci (2015) 128(7):1269–78. doi: 10.1242/jcs.167866
5. Simons K, Toomre D. Lipid rafts and signal transduction. Nat Rev Mol Cell Biol (2000) 1(1):31–9. doi: 10.1038/35036052
6. Williams TM, Lisanti MP. The caveolin proteins. Genome Biol (2004) 5(3):214. doi: 10.1186/gb-2004-5-3-214
7. Parton RG, del Pozo MA. Caveolae as plasma membrane sensors, protectors and organizers. Nat Rev Mol Cell Biol (2013) 14(2):98–112. doi: 10.1038/nrm3512
8. Anderson RG. The caveolae membrane system. Annu Rev Biochem (1998) 67:199–225. doi: 10.1146/annurev.biochem.67.1.199
9. Hill MM, Bastiani M, Luetterforst R, Kirkham M, Kirkham A, Nixon SJ, et al. PTRF-Cavin, a conserved cytoplasmic protein required for caveola formation and function. Cell (2008) 132(1):113–24. doi: 10.1016/j.cell.2007.11.042
10. Couet J, Li S, Okamoto T, Ikezu T, Lisanti MP. Identification of peptide and protein ligands for the caveolin-scaffolding domain. Implications for the interaction of caveolin with caveolae-associated proteins. J Biol Chem (1997) 272(10):6525–33. doi: 10.1074/jbc.272.10.6525
11. Carman CV, Lisanti MP, Benovic JL. Regulation of G protein-coupled receptor kinases by caveolin. J Biol Chem (1999) 274(13):8858–64. doi: 10.1074/jbc.274.13.8858
12. Nystrom FH, Chen H, Cong LN, Li Y, Quon MJ. Caveolin-1 interacts with the insulin receptor and can differentially modulate insulin signaling in transfected Cos-7 cells and rat adipose cells. Mol Endocrinol (1999) 13(12):2013–24. doi: 10.1210/mend.13.12.0392
13. Panetta D, Biedi C, Repetto S, Cordera R, Maggi D. IGF-I regulates caveolin 1 and IRS1 interaction in caveolae. Biochem Biophys Res Commun (2004) 316(1):240–3. doi: 10.1016/j.bbrc.2004.02.037
14. Baggio LL, Drucker DJ. Biology of incretins: GLP-1 and GIP. Gastroenterology (2007) 132(6):2131–57. doi: 10.1053/j.gastro.2007.03.054
15. Nauck MA, Vardarli I, Deacon CF, Holst JJ, Meier JJ. Secretion of glucagon-like peptide-1 (GLP-1) in type 2 diabetes: what is up, what is down? Diabetologia (2011) 54(1):10–8. doi: 10.1007/s00125-010-1896-4
16. Ahren B. Incretin dysfunction in type 2 diabetes: clinical impact and future perspectives. Diabetes Metab (2013) 39(3):195–201. doi: 10.1016/j.diabet.2013.03.001
17. Chon S, Gautier JF. An Update on the Effect of Incretin-Based Therapies on beta-Cell Function and Mass. Diabetes Metab J (2016) 40(2):99–114. doi: 10.4093/dmj.2016.40.2.99
18. Bunck MC, Diamant M, Corner A, Eliasson B, Malloy JL, Shaginian RM, et al. One-year treatment with exenatide improves beta-cell function, compared with insulin glargine, in metformin-treated type 2 diabetic patients: a randomized, controlled trial. Diabetes Care (2009) 32(5):762–8. doi: 10.2337/dc08-1797
19. Campbell RK. Clarifying the role of incretin-based therapies in the treatment of type 2 diabetes mellitus. Clin Ther (2011) 33(5):511–27. doi: 10.1016/j.clinthera.2011.04.015
20. Puddu A, Sanguineti R, Mach F, Dallegri F, Viviani GL, Montecucco F. Update on the protective molecular pathways improving pancreatic beta-cell dysfunction. Mediators Inflamm (2013) 2013:750540. doi: 10.1155/2013/750540
21. van Raalte DH, Verchere CB. Improving glycaemic control in type 2 diabetes: Stimulate insulin secretion or provide beta-cell rest? Diabetes Obes Metab (2017) 19(9):1205–13. doi: 10.1111/dom.12935
22. Tomas A, Jones B, Leech C. New Insights into Beta-Cell GLP-1 Receptor and cAMP Signaling. J Mol Biol (2020) 432(5):1347–66. doi: 10.1016/j.jmb.2019.08.009
23. Drucker DJ, Nauck MA. The incretin system: glucagon-like peptide-1 receptor agonists and dipeptidyl peptidase-4 inhibitors in type 2 diabetes. Lancet (2006) 368(9548):1696–705. doi: 10.1016/S0140-6736(06)69705-5
24. Puddu A, Sanguineti R, Montecucco F, Viviani GL. Retinal pigment epithelial cells express a functional receptor for glucagon-like peptide-1 (GLP-1). Mediators Inflamm (2013) 2013:975032. doi: 10.1155/2013/975032
25. uniprot. (2021). www.uniprot.org/uniprot/P43220.
26. Del Galdo F, Lisanti MP, Jimenez SA. Caveolin-1, transforming growth factor-beta receptor internalization, and the pathogenesis of systemic sclerosis. Curr Opin Rheumatol (2008) 20(6):713–9. doi: 10.1097/bor.0b013e3283103d27
27. Salani B, Passalacqua M, Maffioli S, Briatore L, Hamoudane M, Contini P, et al. IGF-IR internalizes with Caveolin-1 and PTRF/Cavin in HaCat cells. PloS One (2010) 5(11):e14157. doi: 10.1371/journal.pone.0014157
28. Hamoudane M, Maffioli S, Cordera R, Maggi D, Salani B. Caveolin-1 and polymerase I and transcript release factor: new players in insulin-like growth factor-I receptor signaling. J Endocrinol Invest (2013) 36(3):204–8. doi: 10.3275/8848
29. Lee H, Park DS, Razani B, Russell RG, Pestell RG, Lisanti MP. Caveolin-1 mutations (P132L and null) and the pathogenesis of breast cancer: caveolin-1 (P132L) behaves in a dominant-negative manner and caveolin-1 (-/-) null mice show mammary epithelial cell hyperplasia. Am J Pathol (2002) 161(4):1357–69. doi: 10.1016/S0002-9440(10)64412-4
30. Fletcher MM, Halls ML, Zhao P, Clydesdale L, Christopoulos A, Sexton PM, et al. Glucagon-like peptide-1 receptor internalisation controls spatiotemporal signalling mediated by biased agonists. Biochem Pharmacol (2018) 156:406–19. doi: 10.1016/j.bcp.2018.09.003
31. Kuna RS, Girada SB, Asalla S, Vallentyne J, Maddika S, Patterson JT, et al. Glucagon-like peptide-1 receptor-mediated endosomal cAMP generation promotes glucose-stimulated insulin secretion in pancreatic beta-cells. Am J Physiol Endocrinol Metab (2013) 305(2):E161–70. doi: 10.1152/ajpendo.00551.2012
32. Roed SN, Wismann P, Underwood CR, Kulahin N, Iversen H, Cappelen KA, et al. Real-time trafficking and signaling of the glucagon-like peptide-1 receptor. Mol Cell Endocrinol (2014) 382(2):938–49. doi: 10.1016/j.mce.2013.11.010
33. Jones B, Buenaventura T, Kanda N, Chabosseau P, Owen BM, Scott R, et al. Targeting GLP-1 receptor trafficking to improve agonist efficacy. Nat Commun (2018) 9(1):1602. doi: 10.1038/s41467-018-03941-2
34. Thompson A, Kanamarlapudi V. Agonist-induced internalisation of the glucagon-like peptide-1 receptor is mediated by the Galphaq pathway. Biochem Pharmacol (2015) 93(1):72–84. doi: 10.1016/j.bcp.2014.10.015
35. Allen JA, Halverson-Tamboli RA, Rasenick MM. Lipid raft microdomains and neurotransmitter signalling. Nat Rev Neurosci (2007) 8(2):128–40. doi: 10.1038/nrn2059
36. Patel HH, Murray F, Insel PA. Caveolae as organizers of pharmacologically relevant signal transduction molecules. Annu Rev Pharmacol Toxicol (2008) 48:359–91. doi: 10.1146/annurev.pharmtox.48.121506.124841
37. Holz GG. Epac: A new cAMP-binding protein in support of glucagon-like peptide-1 receptor-mediated signal transduction in the pancreatic beta-cell. Diabetes (2004) 53(1):5–13. doi: 10.2337/diabetes.53.1.5
38. Rybin VO, Xu X, Lisanti MP, Steinberg SF. Differential targeting of beta -adrenergic receptor subtypes and adenylyl cyclase to cardiomyocyte caveolae. A mechanism to functionally regulate the cAMP signaling pathway. J Biol Chem (2000) 275(52):41447–57. doi: 10.1074/jbc.M006951200
39. Head BP, Patel HH, Roth DM, Lai NC, Niesman IR, Farquhar MG, et al. G-protein-coupled receptor signaling components localize in both sarcolemmal and intracellular caveolin-3-associated microdomains in adult cardiac myocytes. J Biol Chem (2005) 280(35):31036–44. doi: 10.1074/jbc.M502540200
40. Yu JZ, Rasenick MM. Real-time visualization of a fluorescent G(alpha)(s): dissociation of the activated G protein from plasma membrane. Mol Pharmacol (2002) 61(2):352–9. doi: 10.1124/mol.61.2.352
41. Allen JA, Yu JZ, Donati RJ, Rasenick MM. Beta-adrenergic receptor stimulation promotes G alpha s internalization through lipid rafts: a study in living cells. Mol Pharmacol (2005) 67(5):1493–504. doi: 10.1124/mol.104.008342
42. Allen JA, Yu JZ, Dave RH, Bhatnagar A, Roth BL, Rasenick MM. Caveolin-1 and lipid microdomains regulate Gs trafficking and attenuate Gs/adenylyl cyclase signaling. Mol Pharmacol (2009) 76(5):1082–93. doi: 10.1124/mol.109.060160
43. Bhatnagar A, Sheffler DJ, Kroeze WK, Compton-Toth B, Roth BL. Caveolin-1 interacts with 5-HT2A serotonin receptors and profoundly modulates the signaling of selected Galphaq-coupled protein receptors. J Biol Chem (2004) 279(33):34614–23. doi: 10.1074/jbc.M404673200
44. Oh P, Schnitzer JE. Segregation of heterotrimeric G proteins in cell surface microdomains. G(q) binds caveolin to concentrate in caveolae, whereas G(i) and G(s) target lipid rafts by default. Mol Biol Cell (2001) 12(3):685–98. doi: 10.1091/mbc.12.3.685
45. Sengupta P, Philip F, Scarlata S. Caveolin-1 alters Ca(2+) signal duration through specific interaction with the G alpha q family of G proteins. J Cell Sci (2008) 121(Pt 9):1363–72. doi: 10.1242/jcs.020081
46. Sampson LJ, Hayabuchi Y, Standen NB, Dart C. Caveolae localize protein kinase A signaling to arterial ATP-sensitive potassium channels. Circ Res (2004) 95(10):1012–8. doi: 10.1161/01.RES.0000148634.47095.ab
47. Puddu A, Salani B, Cordera R, Viviani GL, Maggi D. Caveolin-1 is essential for glimepiride-induced insulin secretion in the pancreatic betaTC-6 cell line. Biochem Biophys Res Commun (2008) 375(2):235–7. doi: 10.1016/j.bbrc.2008.08.003
48. Sonoda N, Imamura T, Yoshizaki T, Babendure JL, Lu JC, Olefsky JM. Beta-Arrestin-1 mediates glucagon-like peptide-1 signaling to insulin secretion in cultured pancreatic beta cells. Proc Natl Acad Sci USA (2008) 105(18):6614–9. doi: 10.1073/pnas.0710402105
49. Dalle S, Ravier MA, Bertrand G. Emerging roles for beta-arrestin-1 in the control of the pancreatic beta-cell function and mass: new therapeutic strategies and consequences for drug screening. Cell Signal (2011) 23(3):522–8. doi: 10.1016/j.cellsig.2010.09.014
50. Carneiro AP, Fonseca-Alaniz MH, Dallan LAO, Miyakawa AA, Krieger JE. beta-arrestin is critical for early shear stress-induced Akt/eNOS activation in human vascular endothelial cells. Biochem Biophys Res Commun (2017) 483(1):75–81. doi: 10.1016/j.bbrc.2017.01.003
51. Muller TD, Finan B, Bloom SR, D’Alessio D, Drucker DJ, Flatt PR, et al. Glucagon-like peptide 1 (GLP-1). Mol Metab (2019) 30:72–130. doi: 10.1016/j.molmet.2019.09.010
52. Kieffer TJ, McIntosh CH, Pederson RA. Degradation of glucose-dependent insulinotropic polypeptide and truncated glucagon-like peptide 1 in vitro and in vivo by dipeptidyl peptidase IV. Endocrinology (1995) 136(8):3585–96. doi: 10.1210/endo.136.8.7628397
53. Ohnuma K, Uchiyama M, Yamochi T, Nishibashi K, Hosono O, Takahashi N, et al. Caveolin-1 triggers T-cell activation via CD26 in association with CARMA1. J Biol Chem (2007) 282(13):10117–31. doi: 10.1074/jbc.M609157200
54. Hiromura M, Nohtomi K, Mori Y, Kataoka H, Sugano M, Ohnuma K, et al. Caveolin-1, a binding protein of CD26, is essential for the anti-inflammatory effects of dipeptidyl peptidase-4 inhibitors on human and mouse macrophages. Biochem Biophys Res Commun (2018) 495(1):223–9. doi: 10.1016/j.bbrc.2017.11.016
Keywords: glucagon-like peptide-1, caveolin-1, GLP-1 receptor, G proteins, β-arrestin-1
Citation: Puddu A and Maggi D (2021) Emerging Role of Caveolin-1 in GLP-1 Action. Front. Endocrinol. 12:668012. doi: 10.3389/fendo.2021.668012
Received: 15 February 2021; Accepted: 22 March 2021;
Published: 14 April 2021.
Edited by:
Andrei I. Tarasov, Ulster University, United KingdomReviewed by:
Venkateswarlu Kanamarlapudi, Swansea University, United KingdomCopyright © 2021 Puddu and Maggi. This is an open-access article distributed under the terms of the Creative Commons Attribution License (CC BY). The use, distribution or reproduction in other forums is permitted, provided the original author(s) and the copyright owner(s) are credited and that the original publication in this journal is cited, in accordance with accepted academic practice. No use, distribution or reproduction is permitted which does not comply with these terms.
*Correspondence: Alessandra Puddu, YWxlcDEwMEBob3RtYWlsLmNvbQ==
†These authors have contributed equally to this work
Disclaimer: All claims expressed in this article are solely those of the authors and do not necessarily represent those of their affiliated organizations, or those of the publisher, the editors and the reviewers. Any product that may be evaluated in this article or claim that may be made by its manufacturer is not guaranteed or endorsed by the publisher.
Research integrity at Frontiers
Learn more about the work of our research integrity team to safeguard the quality of each article we publish.