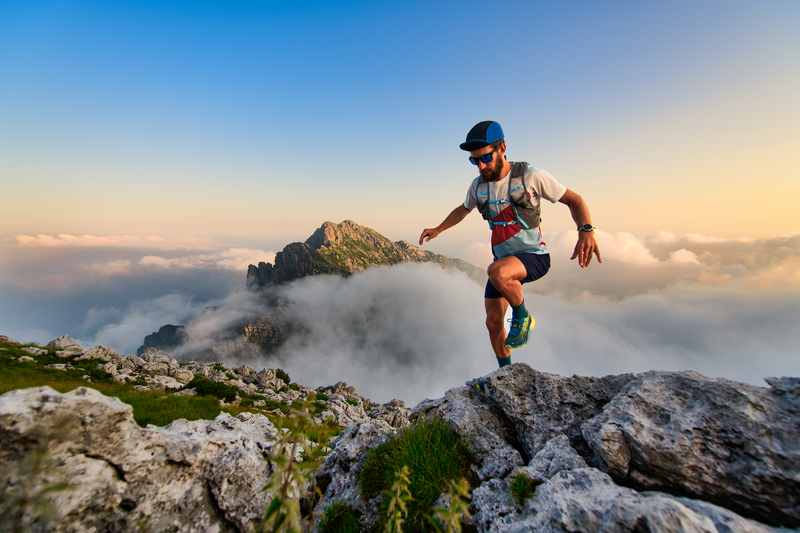
94% of researchers rate our articles as excellent or good
Learn more about the work of our research integrity team to safeguard the quality of each article we publish.
Find out more
REVIEW article
Front. Endocrinol. , 26 April 2021
Sec. Cancer Endocrinology
Volume 12 - 2021 | https://doi.org/10.3389/fendo.2021.657382
Corticotroph tumors (CTs) are pituitary neoplasms arising from the Tpit lineage, which may or not express adrenocorticotrophic hormone (ACTH). Functioning CTs cause Cushing’s disease (CD), which has high morbidity and mortality due to hypercortisolemia. “Non-functioning” or silent CTs (SCT) and the Crooke’s cell subtypes do not cause CD and may be asymptomatic until manifested by compressive symptoms and are more frequently found as macroadenoma. Both tend toward more aggressive behavior, recurrence, and a higher rate of malignant transformation to pituitary carcinoma. Tumorigenesis involves genetic, epigenetic, and post-transcriptional disruption of cell-cycle regulators, which increase cell proliferation, POMC overexpression, ACTH transcription, and/or hypersecretion. Furthermore, functioning CTs develop resistance to glucocorticoid-mediated negative feedback on ACTH secretion, through increased expression of testicular orphan nuclear receptor 4 (TR4), heat-shock protein 90 (HSP90), and loss-of-function mutation of CDK5 and ABL enzyme substrate 1 (CABLES1) gene. Overt autonomous hypercortisolemia is difficult to control, and multiple diagnostic studies and therapeutic modalities are commonly required. Cell-cycle regulation depends mainly on p27, cyclin E, cyclin-dependent kinases (CDKs), and the retinoblastoma protein (Rb)/E2F1 transcription factor complex. Gain-of-function mutations of ubiquitin-specific protease (USP) 8, USP48, and BRAF genes may subsequently cause overexpression of epithelial growth factor receptor (EGFR), and enhance POMC transcription, cell proliferation, and tumor growth. Epigenetic changes through micro RNAs and decreased DNA deacetylation by histone deacetylase type 2 (HDAC2), may also affect tumor growth. All the former mechanisms may become interesting therapeutic targets for CTs, aside from temozolomide, currently used for aggressive tumors. Potential therapeutic agents are EGFR inhibitors such as gefitinib and lapatinib, the purine analog R-roscovitine by dissociation of CDK2/Cyclin E complex, the HSP90 inhibitor silibinin (novobiocin), to reduce resistance to glucocorticoid-mediated negative feedback, and BRAF inhibitors vemurafenib and dabrafenib in BRAF V600E positive tumors. This review summarizes the molecular mechanisms related to CTs tumorigenesis, their diagnostic approach, and provides an update of the potential novel therapies, from the lab bench to the clinical translation.
Cushing’s disease (CD) is caused by oversecretion of adrenocorticotropic hormone (ACTH) by a pituitary corticotroph adenoma and is the most frequent cause of endogenous Cushing’s syndrome (CS) (1). This rare disease is usually diagnosed between the fourth and fifth decade of life, is more frequent in women, and accounts for 4 to 8% of all pituitary tumors (2). Most corticotroph tumors are sporadic and less than 5% of these have been related to familial diseases (3). Endocrine hereditary syndromes with CD include multiple endocrine neoplasia (MEN) type 1 (4), familial isolated pituitary adenomas (FIPAs) (5), Carney complex (6), and DICER1 syndrome (3, 4). A gain-of-function mutation in the ubiquitin-specific protease 8 (USP8) gene has been associated with sporadic CTs in about 20 to 60% of patients (7–10).
On the other hand, silent corticotroph adenomas (SCTs) do not cause hypercortisolism or CS but their recurrence rate reaches 36%, and up to 18% will recur multiple times, being this subtype more aggressive than other pituitary tumors (11). Moreover, pituitary carcinomas frequently arise from the corticotroph lineage (12).
CTs emerge from complex mechanisms that involve cell-cycle dysregulation, genomic abnormalities, and others that currently are not completely understood. There is also controversy on the definitions of remission and recurrence, especially in SCTs.
This manuscript summarizes the pathological mechanisms related to corticotroph tumorigenesis which are implicated in ACTH secretion or clinical silence, recurrence, and aggressive behavior, and provides a review on the potential novel therapies which may target both silent and functioning CTs.
A corticotroph tumor is currently defined as a Tpit-positive neoplasm either if ACTH-positive or not. Most corticotroph tumors have ACTH immunoreactivity and cause ACTH-dependent CS if functioning, known as Cushing’s disease (CD), or to be clinically non-functioning (silent) corticotroph tumor (SCT) (11, 13).
SCTs were defined in 1970 as ACTH-positive staining pituitary tumors which do not cause evident hypercortisolism or CD (14). The expected prevalence is 4.8 to 6.8% among adult non-functioning pituitary adenomas (11) and has been reported only once in the pediatric population (15). Diagnosis is made in a retrospective fashion with histopathological staining since clinical factors and presurgical laboratory tests cannot discern them from other silent adenomas (16). One retrospective study suggested female sex, cavernous sinus invasion, intra-tumoral hemorrhage on MRI, and decreased ACTH response to hypoglycemia, may be associated with SCTs (17). Silent and functioning corticotroph tumors can be further divided into sparsely granulated, densely granulated, and Crooke cell adenomas (13, 18). The current classification recommends against the term “atypical adenoma” and instead proposes routine use of tumor proliferation markers, clinical parameters such as tumor invasion, and evaluation of tumor types that may be more clinically aggressive. Interestingly, two adenoma subtypes which commonly show aggressive behavior fall into the corticotroph lineage: the SCTs and the Crooke’s cell adenomas (19, 20).
A clinicopathological classification have been proposed to predict disease-free and recurrence/progression-free status of pituitary tumors in order to guide clinicians to choose the best therapy (21).
Progression of the cell cycle division in pituitary cells is regulated by the pituitary tumor-transforming gene (PTTG) which encodes a securin protein, which in turn regulates activity in the G1/S phase, influences chromosomal stability, and tumorigenic activity (Figure 1) (22, 23). Such tumorigenic activity is downregulated by proteins of the INK4 (p16, p15, p18, p19) and Cip/Kip (p21, p27, and p57) families (24, 25) to control cyclins’ and cyclin-dependent kinases’ (CDKs) activity (26). CDK4 and CDK6 are active during the G1 (gap 1) phase after association with cyclins D1, D2, and D3. Progression to S (synthesis) phase occurs after cyclin E1 and E2 activation. At this point, downregulation is driven by the INK4 family proteins. Then proteins from the Cip/Kip family, particularly p27, induce cyclin A1 and A2 on CDK1 and CDK2 activity to regulate the end of S phase and move through G2, and then induce CDK1/cyclin B1 and B2 to progress into the M (mitosis) phase (Figure 1).
Figure 1 Abdnomal regulation of cell cycle division, ACTH synthesis, and potential therapeutic approach (highlighted in red) in silent vs. clinically active corticotroph tumors (see text for details).
Experimental treatment with the CDKs (1, 2, 4, 6, and 7) inhibitor flavopiridol in a double CDK4/p27 knockout mouse, resulted in tumor shrinkage by cell-cycle arrest at G1 and G2 phases, confirming the importance of CDKs on pituitary tumor growth (27). Consistent with these findings, the second-generation CDK1 and CDK2 inhibitor R-roscovitine (seliciclib), inhibiting the association of CDK2/cyclin E complexes (28), caused a reduction of POMC promoter expression in ~40% in a double transgenic POMC : PTTG zebrafish model, and in murine AtT20 corticotroph adenoma models (29–31) Other studies also found murine p27 knock-out was associated with enlarging corticotroph tumors within 12 months (32–34), and molecular research of recurrent human corticotroph adenomas or carcinomas showed lower or absent p27 staining (35). Therefore, CDKs, cyclin E, and p27 are key molecules that modify CTs’ behavior. Also, increased expression of cyclin E downregulates the tumor suppressor Brahma-related gen 1 (Brg1), which has synergic action with glucocorticoid receptor (GR), orphan nuclear receptor growth factor 1B, and histone deacetylase 1 (HDAC1) to decrease the POMC promoter expression. Disruption of this complex by cyclin E may increase POMC and ACTH synthesis and, as consequence, CD (36).
Dysfunction of the Rb protein, a tumor suppressor regulator, was first described in retinoblastoma tumor cells and then, in heterozygous or homozygous Rb knockout mouse models were identified the higher risk of pituitary tumors development (37–39). Rb protein is involved in cell-cycle regulation of many tumors, including those of the pituitary (22, 23, 37, 38, 40, 41). However, the role of Rb protein in human CTs seems to be different than in mice models because patients with familial retinoblastoma do not always harbor pituitary tumors, and CTs have been rarely reported (39, 42). Human Rb dysfunction is more commonly associated with aggressive macroadenomas or corticotroph carcinomas (43, 44). It is also a negative cell-cycle regulator that controls G1/S progression by inhibition of the corticotroph-specific E2F1 transcription factor (Figure 1) (45–47).
The proteins of the E2F family (E2F1 to E2F8) are associated with tumorigenesis of multiple cell lines. Regarding CS, the Rb/E2F1 complex formation has been described both in pituitary corticotroph tumors (CD) (48), and ectopic ACTH-secreting carcinomas (29). Araki and colleagues also reported E2F1 binding directly to POMC promoter, increasing its transcription and ACTH synthesis (29, 49). An E2F inhibitor (HLM006474) showed dose-dependent suppression over POMC mRNA expression on CT (45). Therefore, the Rb/E2F1 complex regulates the progression from G1 to S phase of the cell cycle, and free E2F1 acts directly over the POMC promoter (Figure 1) (29, 45). Further research is needed to define whether E2F1 per se has a relationship with corticotroph tumor behavior.
Tpit (formerly known as TBX19) together with Pitx1 interacts on their specific response element sequences at the POMC promoter increasing expression and synthesis of POMC which, after post-transcriptional processing and multiple sites of enzyme sliding, is converted into six main proteins including ACTH (50). When Tpit is expressed in normal corticotroph cells, cyclin E is no longer detected (24). Murine and zebrafish corticotroph tumor models found an abnormal cyclin E upregulation within the neoplastic tissue, causing abnormal reentry to cell-cycle division and centrosome instability, promoting tumorigenesis (30, 36, 51). Interestingly, R-roscovitine, which also targets the Tpit binding region (TCACACC) of the POMC promoter, interferes with cyclin E and E2F1 and causes suppression of ACTH synthesis and secretion in a dose-dependent manner (31). This suppression of the POMC promoter and ACTH release by R-roscovitine was also confirmed in ectopic ACTH-secreting tumors (29, 52). A phase II clinical trial to evaluate R-roscovitine in patients with confirmed recurrent CD is currently closed with pending results (ClinicalTrials.gov NCT02160730).
Epidermal growth factor receptor (EGFR) is a tyrosine kinase receptor that is commonly expressed in normal corticotroph cells. EGFR is one of the most important inductors of POMC transcription, and ACTH synthesis (53), and EGFR overexpression was found in CT of transgenic murine models, causing CD (45). EGFR signalizes by phosphorylating the extracellular response kinase 1/2 (pErk1/2) and this pathway induces POMC transcription through a higher expression of free E2F1. The mechanisms by which POMC transcription is induced by EGFR are not completely understood (8, 9).
USP8 is an enzyme that mediates the deubiquitination of intracellular vesicles of normal corticotroph cells and therefore avoids lysosomal degradation. Gain-of-function mutations in the USP8 gene lead to an impaired association with 14-3-3 protein, resulting in increased deubiquitination of endocytosed vesicles, including EGFR (8, 54, 55). Such deubiquitination causes EGFR recycling and overexpression. The action of both EGF and transforming growth factor-alpha (TGF-alfa) on more abundant EGFR results in higher POMC expression and ACTH synthesis (Figure 1) (8, 9). Interestingly, such USP8 mutation is specific in CTs and it was not identified in other types of pituitary tumors (10), was reported more frequently in women (8, 9, 56), and was identified in patients with higher cortisol levels and more aggressive CTs (57). In contrast, a smaller sample (n=13) of silent, less aggressive, CTs showed no USP8 mutations (56).
USP8 mutations in tumors causing CD have a prevalence of 30 to 50%. Nevertheless, CD USP8-mutated tumors did not show a higher rate of tumor aggressive behavior, suggesting that complementary mechanisms need to be elucidated (8, 58, 59). (8, 9).
Chen et al. reported recurrent mutations in the USP48, which predominantly encodes p.M4151 or p.M415V in 23% (21/91), and BRAF, encoding p.V600E, in 16% (15/91) patients with CD (60). USP48 and BRAF mutants enhance POMC transcription, suggesting an additional mechanism for ACTH excess (60). Such results may also have therapeutic implications in the future, since BRAF inhibitors vemurafenib and dabrafenib are currently FDA approved for the treatment of late-stage melanoma (see below), and might be tested in patients with corticotroph cells harboring BRAF V600E in recurrent CD (60).
Somatic mutations are present in a small proportion of patients with CD; therefore, epigenetic changes have been considered as a potential mechanism of tumorigenesis (61).
Histone deacetylase type 2 (HDAC2) regulates gene expression by removing acetyl groups from lysine residues located at the N-terminal region of core histones. Inhibition of HDAC2 reduces the survival of normal corticotroph cells and may impair ACTH secretion (62). A lower expression of this enzyme has also been associated with the development of glucocorticoid resistance (36).
DNA acetylation and methylation are directly related to changes in p53 and Rb protein expression, which have an important role in tumor development and progression (61).
The miRs are small and non-coding RNAs that play an important role in a variety of cellular processes, including cell development, differentiation, and apoptosis. Binding miRs to specific messenger-RNAs (mRNA) may block their translation to proteins. Some miRs have been described in pituitary tumorigenesis (63–65) including CTs (66–68). More aggressive behavior of CTs is associated with overexpression of miR-25, miR-93, and miR106b (miR-106b~25 cluster) (67). A higher expression of the miR-106b~25 cluster has also been described in aggressive Crooke cell adenomas (67). Crooke cell adenomas are characterized by cytoplasmic hyaline inclusions instead of normal densely or sparsely granulation (20, 69). The triggering mechanisms causing the Crooke hyaline changes are currently unknown. Galectin 3 (LGALS3), which is regulated by miR-493, is also overexpressed in CTs and correlates to increased tumor aggressiveness (70). In contrast, a decreased expression of miR-141 has been found to predict a higher probability of CD remission (66). Unfortunately, the pathological expression of miRs in CTs has been reported in a small number of patients and further research is needed.
Compared to ACTH-secreting CTs, SCT may have a different embryologic origin arising from POMC-expressing cells in the pars intermedia of the pituitary gland (71). Lack of ACTH oversecretion from SCT has been related to two mechanism: The presence of a higher amount of lysosomes in the tumor’s cytoplasm, which causes premature destruction of ACTH before release (72); and disruption of POMC-product processing due to dysfunction or reduced expression of prohormone convertase 1/3 (PC1/3) resulting in an inability to synthesize mature ACTH molecules (Figure 1) (72, 73). PC1/3 cleaves POMC in dibasic sites comprising lysine (K) and arginine (R) resulting in pro-adrenocorticotropin (pro-ACTH) and then to ACTH (50). Interestingly, SCTs transforming to functional ACTH-secreting CTs show a higher PC1/3 expression (73). Increased prevalence of USP8 mutations has not been reported in SCT, suggesting different genetic or epigenetic backgrounds (56). However, some similarities in related etiological pathways may be found soon since SCTs have been reported to become functioning CTs and vice versa (73).
Corticotroph glucocorticoid resistance and negative feedback disruption are common features of CD. Possible mechanisms have been related to USP8 mutations, testicular orphan nuclear receptor 4 (TR4), and heat shock protein 90 (HSP90) (Figure 2). TR4 overexpression is highly prevalent in CTs. This receptor increases POMC transcription, ACTH secretion, and tumor cell growth through the mitogen-activated protein kinase/extracellular signal-regulated kinase (MAPK/ERK) pathway (74, 75). TR4 directly inhibits GR’s interaction with the POMC promoter region and causes resistance to negative feedback in CD (Figure 2) (74, 75). Therapy targeting TR4 in CD may be evaluated in the future.
Figure 2 Suggested mechanisms for disruption of negative feedback in corticotroph tumors (see text for details).
HSP90 is a chaperone protein that induces conformational changes of different proteins, including the GR. HSP90 overexpression causes an increased binding to the GRs preventing dissociation from the chaperone system and their translocation to the nucleus, which otherwise suppresses POMC transcription, and disrupts glucocorticoid-mediated negative feedback (76).
Glucocorticoid resistance also has been found in four CDK5 and ABL enzyme substrate 1 (CABLES1) gene germline missense variants in 4 female patients, and a loss-of-function mutation in 146 pediatric and 35 adult patients. All cases showed aggressive tumor behavior (5, 77).
A USP8 inhibitor (9-ethyloxyimino-9H-ideno[1,2-b] pyrazine-2,3dicarbonile) has been used for in vitro studies and animal models but has not been studied for therapeutical purposes. In AtT20 cells, USP8 inhibition with this molecule downregulated EGFR expression, decreased POMC transcription and ACTH synthesis and secretion, and induced cell apoptosis (8, 54, 55, 59, 78). Future research may explore USPs as potential target molecules for the treatment of both silent and secreting corticotroph adenomas.
One phase II open-label clinical trial in patients with USP8-mutated corticotroph tumors and CD was registered in 2015 but data on results has not been updated ever since (https://clinicaltrials.gov/ct2/show/NCT02484755: Targeted Therapy With Gefitinib in Patients With USP8-mutated Cushing’s Disease) (54, 79, 80). Lapatinib is another tyrosine kinase inhibitor acting over the EGFR and HER2 receptors, which has been tested in vitro to decrease POMC mRNA and ACTH levels in murine AtT-20 corticotroph cells. It also decreased PTTG gene expression, cell proliferation, and induced apoptosis (Figure 2). Results were replicated in vivo, tested in murine animal models (54, 81) but not in humans.
In the presence of a wild USP8 phenotype, whole-exome sequencing has discovered mutation on both USP48, which causes ACTH mRNA overexpression, and BRAF genes, which in turn upregulates Erk1/2 phosphorylation with subsequent Nurr77, c-jun, and c-fos activation leading to increased POMC transcription (60). BRAF inhibitors such as vemurafenib and dabrafenib have been used to treat BRAF V600 mutation-positive malignancies and have become a potential option to treat BRAF V600-mutated CD (Figure 1). One in vitro study using vemurafenib in BRAF V600-mutated corticotroph cells showed a reduction in ACTH secretion after 1-day incubation (82, 83).
Silibinin is a HSP90 C-terminal inhibitor found in the milk thistle (Silybum marianum) previously used to treat amatoxin poisoning and studied as a potential treatment of many malignancies (prostate, breast, hepatic cell, lymphoblastic leukemia), showing an acceptable safety profile (84, 85). This compound releases GRs from HSP90, therefore reestablishing glucocorticoid-mediated negative feedback on ACTH secretion (Figure 2) (80). There are no ongoing clinical trials for the evaluation of silibinin for CD.
Cyclin-dependent kinases (CDKs) are essential regulatory proteins of the cell cycle progression (86). Pituitary cyclin E/E2F1 is a potential molecular target of pituitary ACTH-dependent hypercortisolism. In corticotroph tumor AtT20 cells murine models, R-roscovitine down-regulates cyclin E/E2F1 resulting in suppressed POMC expression (30). Also, in human pituitary corticotroph tumors treated with R-roscovitine, resulted in inhibition of the kinase activity (31).
Mechanisms of CTs tumorigenesis have identified hundreds of potential genes, miRs, proteins, and peptides which are up or downregulated in comparison to the normal anterior pituitary. These changes may represent potential targets for pharmacological treatment or have an impact on the prediction of CD and SCTs recurrence. Further research is needed to better understand CT origin and pathophysiology. This may also lead to the identification of novel markers of disease severity or progression, and diagnostic tests with higher performance to predict recurrent CD or SCT. Although novel molecular markers are still not clinically validated as predictors of recurrence in CT, some of them are currently targeted for research as potential novel therapies.
JH-A designed the review. JH-A, CL-C, and DC-R prepared the draft manuscript. DC-R reviewed and approved the submitted version. All authors contributed to the article and approved the submitted version.
The authors declare that the research was conducted in the absence of any commercial or financial relationships that could be construed as a potential conflict of interest.
The authors would like to express their deepest appreciation to their former mentors for their continuous support, valuable advice, and guidance.
1. Biller BM, Grossman AB, Stewart PM, Melmed S, Bertagna X, Bertherat J, et al. Treatment of adrenocorticotropin-dependent Cushing’s syndrome: a consensus statement. J Clin Endocrinol Metab (2008) 93(7):2454–62. doi: 10.1210/jc.2007-2734
2. Pivonello R, De Leo M, Cozzolino A, Colao A. The Treatment of Cushing’s Disease. Endocr Rev (2015) 36(4):385–486. doi: 10.1210/er.2013-1048
3. Xiong Q, Ge W. Gene mutations in Cushing’s disease. Biomed Rep (2016) 5(3):277–82. doi: 10.3892/br.2016.729
4. Schernthaner-Reiter MH, Trivellin G, Stratakis CA. MEN1, MEN4, and Carney Complex: Pathology and Molecular Genetics. Neuroendocrinology (2016) 103(1):18–31. doi: 10.1159/000371819
5. Hernández-Ramírez LC, Gabrovska P, Dénes J, Stals K, Trivellin G, Tilley D, et al. Landscape of Familial Isolated and Young-Onset Pituitary Adenomas: Prospective Diagnosis in AIP Mutation Carriers. J Clin Endocrinol Metab (2015) 100(9):E1242–54. doi: 10.1210/jc.2015-1869
6. Lowe KM, Young WF, Jr., Lyssikatos C, Stratakis CA, Carney JA. Cushing Syndrome in Carney Complex: Clinical, Pathologic, and Molecular Genetic Findings in the 17 Affected Mayo Clinic Patients. Am J Surg Pathol (2017) 41(2):171–81. doi: 10.1097/PAS.0000000000000748
7. Ballmann C, Thiel A, Korah HE, Reis AC, Saeger W, Stepanow S, et al. USP8 Mutations in Pituitary Cushing Adenomas-Targeted Analysis by Next-Generation Sequencing. J Endocr Soc (2018) 2(3):266–78. doi: 10.1210/js.2017-00364
8. Ma ZY, Song ZJ, Chen JH, Wang YF, Li SQ, Zhou LF, et al. Recurrent gain-of-function USP8 mutations in Cushing’s disease. Cell Res (2015) 25(3):306–17. doi: 10.1038/cr.2015.20
9. Reincke M, Sbiera S, Hayakawa A, Theodoropoulou M, Osswald A, Beuschlein F, et al. Mutations in the deubiquitinase gene USP8 cause Cushing’s disease. Nat Genet (2015) 47(1):31–8. doi: 10.1038/ng.3166
10. Song ZJ, Reitman ZJ, Ma ZY, Chen JH, Zhang QL, Shou XF, et al. The genome-wide mutational landscape of pituitary adenomas. Cell Res (2016) 26(11):1255–9. doi: 10.1038/cr.2016.114
11. Langlois F, Lim DST, Yedinak CG, Cetas I, McCartney S, Cetas J, et al. Predictors of silent corticotroph adenoma recurrence; a large retrospective single center study and systematic literature review. Pituitary (2018) 21(1):32–40. doi: 10.1007/s11102-017-0844-4
12. McCormack A, Dekkers OM, Petersenn S, Popovic V, Trouillas J, Raverot G, et al. Treatment of aggressive pituitary tumours and carcinomas: results of a European Society of Endocrinology (ESE) survey 2016. Eur J Rndocrinol (2018) 178(3):265–76. doi: 10.1530/EJE-17-0933
13. Trouillas J, Jaffrain-Rea ML, Vasiljevic A, Raverot G, Roncaroli F, Villa C. How to Classify the Pituitary Neuroendocrine Tumors (PitNET)s in 2020. Cancers (2020) 12(2):514–31. doi: 10.3390/cancers12020514
14. Cooper O. Silent corticotroph adenomas. Pituitary (2015) 18(2):225–31. doi: 10.1007/s11102-014-0624-3
15. Prendergast N, Aldana PR, Rotondo RL, Torres-Santiago L, Beier AD. Pediatric silent corticotroph pituitary adenoma and role for proton therapy: case report. J Neurosurg Pediatr (2018) 23(2):214–8. doi: 10.3171/2018.9.PEDS18107
16. Ben-Shlomo A, Cooper O. Silent corticotroph adenomas. Pituitary (2018) 21(2):183–93. doi: 10.1007/s11102-018-0864-8
17. Kim D, Ku CR, Park SH, Moon JH, Kim EH, Kim SH, et al. Clinical Parameters to Distinguish Silent Corticotroph Adenomas from Other Nonfunctioning Pituitary Adenomas. World Neurosurg (2018) 115:e464–e71. doi: 10.1016/j.wneu.2018.04.070
18. Jahangiri A, Wagner JR, Pekmezci M, Hiniker A, Chang EF, Kunwar S, et al. A Comprehensive Long-term Retrospective Analysis of Silent Corticotrophic Adenomas vs Hormone-Negative Adenomas. Neurosurgery (2013) 73(1):8–18. doi: 10.1227/01.neu.0000429858.96652.1e
19. Liu J, He Y, Zhang X, Yan X, Huang Y. Clinicopathological analysis of 250 cases of pituitary adenoma under the new WHO classification. Oncol Lett (2020) 19(3):1890–8. doi: 10.3892/ol.2020.11263
20. Mete O, Lopes MB. Overview of the 2017 WHO Classification of Pituitary Tumors. Endocr Pathol (2017) 28(3):228–43. doi: 10.1007/s12022-017-9498-z
21. Trouillas J, Roy P, Sturm N, Dantony E, Cortet-Rudelli C, Viennet G, et al. A new prognostic clinicopathological classification of pituitary adenomas: a multicentric case-control study of 410 patients with 8 years post-operative follow-up. Acta Neuropathol (2013) 126(1):123–35. doi: 10.1007/s00401-013-1084-y
22. Pei L, Melmed S. Isolation and characterization of a pituitary tumor-transforming gene (PTTG). Mol Endocrinol (Baltimore Md) (1997) 11(4):433–41. doi: 10.1210/mend.11.4.9911
23. Tong Y, Tan Y, Zhou C, Melmed S. Pituitary tumor transforming gene interacts with Sp1 to modulate G1/S cell phase transition. Oncogene (2007) 26(38):5596–605. doi: 10.1038/sj.onc.1210339
24. Bilodeau S, Roussel-Gervais A, Drouin J. Distinct developmental roles of cell cycle inhibitors p57Kip2 and p27Kip1 distinguish pituitary progenitor cell cycle exit from cell cycle reentry of differentiated cells. Mol Cell Biol (2009) 29(7):1895–908. doi: 10.1128/MCB.01885-08
25. Malumbres M, Barbacid M. Cell cycle, CDKs and cancer: a changing paradigm. Nat Rev Cancer (2009) 9(3):153–66. doi: 10.1038/nrc2602
26. Malumbres M, Barbacid M. Mammalian cyclin-dependent kinases. Trends Biochem Sci (2005) 30(11):630–41. doi: 10.1016/j.tibs.2005.09.005
27. Sotillo R, Renner O, Dubus P, Ruiz-Cabello J, Martín-Caballero J, Barbacid M, et al. Cooperation between Cdk4 and p27kip1 in tumor development: a preclinical model to evaluate cell cycle inhibitors with therapeutic activity. Cancer Res (2005) 65(9):3846–52. doi: 10.1158/0008-5472.CAN-04-4195
28. Meijer L, Borgne A, Mulner O, Chong JP, Blow JJ, Inagaki N, et al. Biochemical and cellular effects of roscovitine, a potent and selective inhibitor of the cyclin-dependent kinases cdc2, cdk2 and cdk5. Eur J Biochem (1997) 243(1-2):527–36. doi: 10.1111/j.1432-1033.1997.t01-2-00527.x
29. Araki T, Liu NA, Tone Y, Cuevas-Ramos D, Heltsley R, Tone M, et al. E2F1-mediated human POMC expression in ectopic Cushing’s syndrome. Endocr Relat Cancer (2016) 23(11):857–70. doi: 10.1530/ERC-16-0206
30. Liu NA, Jiang H, Ben-Shlomo A, Wawrowsky K, Fan XM, Lin S, et al. Targeting zebrafish and murine pituitary corticotroph tumors with a cyclin-dependent kinase (CDK) inhibitor. Proc Natl Acad Sci USA (2011) 108(20):8414–9. doi: 10.1073/pnas.1018091108
31. Liu NA, Araki T, Cuevas-Ramos D, Hong J, Ben-Shlomo A, Tone Y, et al. Cyclin E-Mediated Human Proopiomelanocortin Regulation as a Therapeutic Target for Cushing Disease. J Clin Endocrinol Metab (2015) 100(7):2557–64. doi: 10.1210/jc.2015-1606
32. Fero ML, Rivkin M, Tasch M, Porter P, Carow CE, Firpo E, et al. A syndrome of multiorgan hyperplasia with features of gigantism, tumorigenesis, and female sterility in p27(Kip1)-deficient mice. Cell (1996) 85(5):733–44. doi: 10.1016/S0092-8674(00)81239-8
33. Kiyokawa H, Kineman RD, Manova-Todorova KO, Soares VC, Hoffman ES, Ono M, et al. Enhanced growth of mice lacking the cyclin-dependent kinase inhibitor function of p27(Kip1). Cell (1996) 85(5):721–32. doi: 10.1016/S0092-8674(00)81238-6
34. Nakayama K, Ishida N, Shirane M, Inomata A, Inoue T, Shishido N, et al. Mice lacking p27(Kip1) display increased body size, multiple organ hyperplasia, retinal dysplasia, and pituitary tumors. Cell (1996) 85(5):707–20. doi: 10.1016/S0092-8674(00)81237-4
35. Lidhar K, Korbonits M, Jordan S, Khalimova Z, Kaltsas G, Lu X, et al. Low expression of the cell cycle inhibitor p27Kip1 in normal corticotroph cells, corticotroph tumors, and malignant pituitary tumors. J Clin Endocrinol Metab (1999) 84(10):3823–30. doi: 10.1210/jcem.84.10.6066
36. Bilodeau S, Vallette-Kasic S, Gauthier Y, Figarella-Branger D, Brue T, Berthelet F, et al. Role of Brg1 and HDAC2 in GR trans-repression of the pituitary POMC gene and misexpression in Cushing disease. Genes Dev (2006) 20(20):2871–86. doi: 10.1101/gad.1444606
37. Clarke AR, Maandag ER, van Roon M, van der Lugt NM, van der Valk M, Hooper ML, et al. Requirement for a functional Rb-1 gene in murine development. Nature (1992) 359(6393):328–30. doi: 10.1038/359328a0
38. Lee EY, Chang CY, Hu N, Wang YC, Lai CC, Herrup K, et al. Mice deficient for Rb are nonviable and show defects in neurogenesis and haematopoiesis. Nature (1992) 359(6393):288–94. doi: 10.1038/359288a0
39. Matsunaga E. On estimating penetrance of the retinoblastoma gene. Hum Genet (1980) 56(1):127–8. doi: 10.1007/BF00281583
40. Jacks T, Fazeli A, Schmitt EM, Bronson RT, Goodell MA, Weinberg RA. Effects of an Rb mutation in the mouse. Nature (1992) 359(6393):295–300. doi: 10.1038/359295a0
41. Romero F, Multon MC, Ramos-Morales F, Domínguez A, Bernal JA, Pintor-Toro JA, et al. Human securin, hPTTG, is associated with Ku heterodimer, the regulatory subunit of the DNA-dependent protein kinase. Nucleic Acids Res (2001) 29(6):1300–7. doi: 10.1093/nar/29.6.1300
42. Draper GJ, Sanders BM, Kingston JE. Second primary neoplasms in patients with retinoblastoma. Br J Cancer (1986) 53(5):661–71. doi: 10.1038/bjc.1986.110
43. Cryns VL, Alexander JM, Klibanski A, Arnold A. The retinoblastoma gene in human pituitary tumors. J Clin Endocrinol Metab (1993) 77(3):644–6. doi: 10.1210/jcem.77.3.7690360
44. Pei L, Melmed S, Scheithauer B, Kovacs K, Benedict WF, Prager D. Frequent loss of heterozygosity at the retinoblastoma susceptibility gene (RB) locus in aggressive pituitary tumors: evidence for a chromosome 13 tumor suppressor gene other than RB. Cancer Res (1995) 55(8):1613–6.
45. Araki T, Liu X, Kameda H, Tone Y, Fukuoka H, Tone M, et al. EGFR Induces E2F1-Mediated Corticotroph Tumorigenesis. J Endocr Soc (2017) 1(2):127–43. doi: 10.1210/js.2016-1053
46. DeCaprio JA, Ludlow JW, Lynch D, Furukawa Y, Griffin J, Piwnica-Worms H, et al. The product of the retinoblastoma susceptibility gene has properties of a cell cycle regulatory element. Cell (1989) 58(6):1085–95. doi: 10.1016/0092-8674(89)90507-2
47. Sage J, Mulligan GJ, Attardi LD, Miller A, Chen S, Williams B, et al. Targeted disruption of the three Rb-related genes leads to loss of G(1) control and immortalization. Genes Dev (2000) 14(23):3037–50. doi: 10.1101/gad.843200
48. Ohtani K, DeGregori J, Nevins JR. Regulation of the cyclin E gene by transcription factor E2F1. Proc Natl Acad Sci United States America (1995) 92(26):12146–50. doi: 10.1073/pnas.92.26.12146
49. Araki T, Liu NA. Cell Cycle Regulators and Lineage-Specific Therapeutic Targets for Cushing Disease. Front Endocrinol (2018) 9:444. doi: 10.3389/fendo.2018.00444
50. Harno E, Gali Ramamoorthy T, Coll AP, White A. POMC: The Physiological Power of Hormone Processing. Physiol Rev (2018) 98(4):2381–430. doi: 10.1152/physrev.00024.2017
51. Jordan S, Lidhar K, Korbonits M, Lowe DG, Grossman AB. Cyclin D and cyclin E expression in normal and adenomatous pituitary. Eur J Endocrinol (2000) 143(1):R1–6. doi: 10.1530/eje.0.143r001
52. Pettengill OS, Sorenson GD, Wurster-Hill DH, Curphey TJ, Noll WW, Cate CC, et al. Isolation and growth characteristics of continuous cell lines from small-cell carcinoma of the lung. Cancer (1980) 45(5):906–18. doi: 10.1002/1097-0142(19800301)45:5<906::AID-CNCR2820450513>3.0.CO;2-H
53. Theodoropoulou M, Arzberger T, Gruebler Y, Jaffrain-Rea ML, Schlegel J, Schaaf L, et al. Expression of epidermal growth factor receptor in neoplastic pituitary cells: evidence for a role in corticotropinoma cells. J Rndocrinol (2004) 183(2):385–94. doi: 10.1677/joe.1.05616
54. Ben-Shlomo A, Cooper O. Role of tyrosine kinase inhibitors in the treatment of pituitary tumours: from bench to bedside. Curr Opin Endocrinol Diabetes Obes (2017) 24(4):301–5. doi: 10.1097/MED.0000000000000344
55. Langlois F, McCartney S, Fleseriu M. Recent Progress in the Medical Therapy of Pituitary Tumors. Endocrinol Metab (Seoul Korea) (2017) 32(2):162–70. doi: 10.3803/EnM.2017.32.2.162
56. Perez-Rivas LG, Theodoropoulou M, Ferraù F, Nusser C, Kawaguchi K, Stratakis CA, et al. The Gene of the Ubiquitin-Specific Protease 8 Is Frequently Mutated in Adenomas Causing Cushing’s Disease. J Clin Endocrinol Metab (2015) 100(7):E997–1004. doi: 10.1055/s-0035-1547607
57. Albani A, Pérez-Rivas LG, Dimopoulou C, Zopp S, Colón-Bolea P, Roeber S, et al. The USP8 mutational status may predict long-term remission in patients with Cushing’s disease. Clin Endocrinol (2018) 89(4):454–8. doi: 10.1111/cen.13802
58. Faucz FR, Tirosh A, Tatsi C, Berthon A, Hernández-Ramírez LC, Settas N, et al. Somatic USP8 Gene Mutations Are a Common Cause of Pediatric Cushing Disease. J Clin Endocrinol Metab (2017) 102(8):2836–43. doi: 10.1210/jc.2017-00161
59. Hayashi K, Inoshita N, Kawaguchi K, Ibrahim Ardisasmita A, Suzuki H, Fukuhara N, et al. The USP8 mutational status may predict drug susceptibility in corticotroph adenomas of Cushing’s disease. Eur J Rndocrinol (2016) 174(2):213–26. doi: 10.1530/EJE-15-0689
60. Chen J, Jian X, Deng S, Ma Z, Shou X, Shen Y, et al. Identification of recurrent USP48 and BRAF mutations in Cushing’s disease. Nat Commun (2018) 9(1):3171. doi: 10.1038/s41467-018-05275-5
61. Zhou Y, Zhang X, Klibanski A. Genetic and epigenetic mutations of tumor suppressive genes in sporadic pituitary adenoma. Mol Cell Endocrinol (2014) 386(1-2):16–33. doi: 10.1016/j.mce.2013.09.006
62. Lu J, Chatain GP, Bugarini A, Wang X, Maric D, Walbridge S, et al. Histone Deacetylase Inhibitor SAHA Is a Promising Treatment of Cushing Disease. J Clin Endocrinol Metab (2017) 102(8):2825–35. doi: 10.1210/jc.2017-00464
63. Wang Y, Yin X, Zhao L, Li S, Duan J, Kuang R, et al. MicroRNA-200b inhibits pituitary tumor cell proliferation and invasion by targeting PKCα. Exp Ther Med (2017) 14(2):1706–14. doi: 10.3892/etm.2017.4681
64. Wierinckx A, Roche M, Legras-Lachuer C, Trouillas J, Raverot G, Lachuer J. MicroRNAs in pituitary tumors. Mol Cell Rndocrinol (2017) 456:51–61. doi: 10.1016/j.mce.2017.01.021
65. Zhang T, Yang Z, Gao H. Advancements in the study of miRNA regulation during the cell cycle in human pituitary adenomas. J Neuro-Oncol (2017) 134(2):253–8. doi: 10.1007/s11060-017-2518-5
66. Amaral FC, Torres N, Saggioro F, Neder L, Machado HR, Silva WA, Jr., et al. MicroRNAs differentially expressed in ACTH-secreting pituitary tumors. J Clin Endocrinol Metab (2009) 94(1):320–3. doi: 10.1210/jc.2008-1451
67. Garbicz F, Mehlich D, Rak B, Sajjad E, Maksymowicz M, Paskal W, et al. Increased expression of the microRNA 106b~25 cluster and its host gene MCM7 in corticotroph pituitary adenomas is associated with tumor invasion and Crooke’s cell morphology. Pituitary (2017) 20(4):450–63. doi: 10.1007/s11102-017-0805-y
68. Gentilin E, Tagliati F, Filieri C, Molè D, Minoia M, Rosaria Ambrosio M, et al. miR-26a plays an important role in cell cycle regulation in ACTH-secreting pituitary adenomas by modulating protein kinase Cδ. Endocrinology (2013) 154(5):1690–700. doi: 10.1210/en.2012-2070
69. Oldfield EH, Vance ML, Louis RG, Pledger CL, Jane JA, Jr., Lopes MB. Crooke’s Changes In Cushing’s Syndrome Depends on Degree of Hypercortisolism and Individual Susceptibility. J Clin Endocrinol Metab (2015) 100(8):3165–71. doi: 10.1210/JC.2015-2493
70. Riss D, Jin L, Qian X, Bayliss J, Scheithauer BW, Young WF, Jr., et al. Differential expression of galectin-3 in pituitary tumors. Cancer Res (2003) 63(9):2251–5.
71. Tateno T, Izumiyama H, Doi M, Akashi T, Ohno K, Hirata Y. Defective expression of prohormone convertase 1/3 in silent corticotroph adenoma. Endocr J (2007) 54(5):777–82. doi: 10.1507/endocrj.K07-059
72. Kovacs K, Horvath E, Bayley TA, Hassaram ST, Ezrin C. Silent corticotroph cell adenoma with lysosomal accumulation and crinophagy. A distinct clinicopathologic entity. Am J Med (1978) 64(3):492–9. doi: 10.1016/0002-9343(78)90236-X
73. Righi A, Faustini-Fustini M, Morandi L, Monti V, Asioli S, Mazzatenta D, et al. The changing faces of corticotroph cell adenomas: the role of prohormone convertase 1/3. Endocrine (2017) 56(2):286–97. doi: 10.1007/s12020-016-1028-0
74. Du L, Bergsneider M, Mirsadraei L, Young SH, Jonker JW, Downes M, et al. Evidence for orphan nuclear receptor TR4 in the etiology of Cushing disease. Proc Natl Acad Sci United States America (2013) 110(21):8555–60. doi: 10.1073/pnas.1306182110
75. Zhang D, Du L, Heaney AP. Testicular Receptor-4: Novel Regulator of Glucocorticoid Resistance. J Clin Endocrinol Metab (2016) 101(8):3123–33. doi: 10.1210/jc.2016-1379
76. Kirschke E, Goswami D, Southworth D, Griffin PR, Agard DA. Glucocorticoid receptor function regulated by coordinated action of the Hsp90 and Hsp70 chaperone cycles. Cell (2014) 157(7):1685–97. doi: 10.1016/j.cell.2014.04.038
77. Roussel-Gervais A, Couture C, Langlais D, Takayasu S, Balsalobre A, Rueda BR, et al. The Cables1 Gene in Glucocorticoid Regulation of Pituitary Corticotrope Growth and Cushing Disease. J Clin Endocrinol Metab (2016) 101(2):513–22. doi: 10.1210/jc.2015-3324
78. Jian FF, Li YF, Chen YF, Jiang H, Chen X, Zheng LL, et al. Inhibition of Ubiquitin-specific Peptidase 8 Suppresses Adrenocorticotropic Hormone Production and Tumorous Corticotroph Cell Growth in AtT20 Cells. Chin Med J (2016) 129(17):2102–8. doi: 10.4103/0366-6999.189047
79. Fukuoka H, Cooper O, Ben-Shlomo A, Mamelak A, Ren SG, Bruyette D, et al. EGFR as a therapeutic target for human, canine, and mouse ACTH-secreting pituitary adenomas. J Clin Invest (2011) 121(12):4712–21. doi: 10.1172/JCI60417
80. Langlois F, Chu J, Fleseriu M. Pituitary-Directed Therapies for Cushing’s Disease. Front Endocrinol (2018) 9:164. doi: 10.3389/fendo.2018.00164
81. Asari Y, Kageyama K, Sugiyama A, Kogawa H, Niioka K, Daimon M. Lapatinib decreases the ACTH production and proliferation of corticotroph tumor cells. Endocr J (2019) 66(6):515–22. doi: 10.1507/endocrj.EJ18-0491
82. Hyman DM, Puzanov I, Subbiah V, Faris JE, Chau I, Blay JY, et al. Vemurafenib in Multiple Nonmelanoma Cancers with BRAF V600 Mutations. New Engl J Med (2015) 373(8):726–36. doi: 10.1056/NEJMoa1502309
83. Sosman JA, Kim KB, Schuchter L, Gonzalez R, Pavlick AC, Weber JS, et al. Survival in BRAF V600-mutant advanced melanoma treated with vemurafenib. New Engl J Med (2012) 366(8):707–14. doi: 10.1056/NEJMoa1112302
84. Mengs U, Pohl RT, Mitchell T. Legalon® SIL: the antidote of choice in patients with acute hepatotoxicity from amatoxin poisoning. Curr Pharm Biotechnol (2012) 13(10):1964–70. doi: 10.2174/138920112802273353
85. Tiwari P, Mishra K. Silibinin in cancer therapy: A promising prospect. Cancer Res Front (2015) 1:303–18. doi: 10.17980/2015.303
Keywords: ACTH, corticotropin, Cushing disease, cortisol, molecular biology, corticotroph tumor, pituitary tumor
Citation: Hinojosa-Amaya JM, Lam-Chung CE and Cuevas-Ramos D (2021) Recent Understanding and Future Directions of Recurrent Corticotroph Tumors. Front. Endocrinol. 12:657382. doi: 10.3389/fendo.2021.657382
Received: 22 January 2021; Accepted: 29 March 2021;
Published: 26 April 2021.
Edited by:
Veronica Vella, University of Catania, ItalyReviewed by:
Hidenori Fukuoka, Kobe University, JapanCopyright © 2021 Hinojosa-Amaya, Lam-Chung and Cuevas-Ramos. This is an open-access article distributed under the terms of the Creative Commons Attribution License (CC BY). The use, distribution or reproduction in other forums is permitted, provided the original author(s) and the copyright owner(s) are credited and that the original publication in this journal is cited, in accordance with accepted academic practice. No use, distribution or reproduction is permitted which does not comply with these terms.
*Correspondence: Daniel Cuevas-Ramos, ZGFuaWVsLmN1ZXZhc3JAaW5jbW5zei5teA==
Disclaimer: All claims expressed in this article are solely those of the authors and do not necessarily represent those of their affiliated organizations, or those of the publisher, the editors and the reviewers. Any product that may be evaluated in this article or claim that may be made by its manufacturer is not guaranteed or endorsed by the publisher.
Research integrity at Frontiers
Learn more about the work of our research integrity team to safeguard the quality of each article we publish.