- 1First Faculty of Medicine, Institute of Physiology, Charles University, Prague, Czechia
- 2First Faculty of Medicine, Institute of Pathological Physiology, Charles University, Prague, Czechia
- 3Laboratory of Cellular and Molecular Immunology, Institute of Microbiology of the Czech Academy of Sciences, Prague, Czechia
- 4Psychiatric Clinic, Eating Disorder Center, First Faculty of Medicine, Charles University and General University Hospital in Prague, Prague, Czechia
- 5Steroid Hormone and Proteofactors Department, Institute of Endocrinology, Prague, Czechia
The equilibrium and reciprocal actions among appetite-stimulating (orexigenic) and appetite-suppressing (anorexigenic) signals synthesized in the gut, brain, microbiome and adipose tissue (AT), seems to play a pivotal role in the regulation of food intake and feeding behavior, anxiety, and depression. A dysregulation of mechanisms controlling the energy balance may result in eating disorders such as anorexia nervosa (AN) and bulimia nervosa (BN). AN is a psychiatric disease defined by chronic self-induced extreme dietary restriction leading to an extremely low body weight and adiposity. BN is defined as out-of-control binge eating, which is compensated by self-induced vomiting, fasting, or excessive exercise. Certain gut microbiota-related compounds, like bacterial chaperone protein Escherichia coli caseinolytic protease B (ClpB) and food-derived antigens were recently described to trigger the production of autoantibodies cross-reacting with appetite-regulating hormones and neurotransmitters. Gut microbiome may be a potential manipulator for AT and energy homeostasis. Thus, the regulation of appetite, emotion, mood, and nutritional status is also under the control of neuroimmunoendocrine mechanisms by secretion of autoantibodies directed against neuropeptides, neuroactive metabolites, and peptides. In AN and BN, altered cholinergic, dopaminergic, adrenergic, and serotonergic relays may lead to abnormal AT, gut, and brain hormone secretion. The present review summarizes updated knowledge regarding the gut dysbiosis, gut-barrier permeability, short-chain fatty acids (SCFA), fecal microbial transplantation (FMT), blood-brain barrier permeability, and autoantibodies within the ghrelin and melanocortin systems in eating disorders. We expect that the new knowledge may be used for the development of a novel preventive and therapeutic approach for treatment of AN and BN.
Introduction
Anorexia nervosa (AN) and bulimia nervosa (BN) are serious eating disorders with a substantial impact on the long-term quality of life and broad psychological, social and economic implications. These psychiatric disorders affect as many as 2-3% of young women and adolescents (1) and exhibit substantial mortality (with a mortality rate of 5-10% after 10 years) (2). Both AN and BN are disorders with severe disturbances in eating behavior. While AN is characterized by self-induced starvation, amenorrhea, severe weight loss due to reduction of both fat mass and fat free mass mainly at the expense of adipose tissue (AT), refusal to gain and maintain a minimal normal body weight (weight criterion for the diagnosis is under 85% of normal body weight), manifestations of BN include recurrent episodes of binge eating followed by inappropriate compensatory behavior such as self-induced vomiting, laxative and diuretics misuse (3).
Despite extensive research efforts worldwide, the etiopathogenesis of AN and BN has not been elucidated to date. Fetissov et al. hypothesized that AN is an autoimmune disease caused by delayed exposure to microorganisms (such as Group A β-hemolytic Streptococcus, Escherichia coli, and Helicobacter pylori) in which autoantibodies against appetite-regulating neuropeptides, neurotransmitters, peptide hormones, and hypothalamic neurons disturb appetite and mood and lead to decreased intake of food (4). A higher prevalence of autoimmune diseases such as type 1 diabetes and Crohn’s disease was observed among patients with eating disorders (5). In this vein, the development of type 1 diabetes in adolescence seems to be a risk factor for the subsequent development of AN and BN (6). Further, patients with AN are suggested to be susceptible to autoimmune diseases and thus, a bi-directional relationship between eating disorders and autoimmunity was considered (7–9).
Recently, Watson et al. (10) identified multiple genetic loci for AN and reconceptualized AN as a metabo-psychiatric disorder. Negative genetic correlations were documented between anorexic patients and metabolic traits such as type 2 diabetes, insulin resistance, blood plasma insulin, leptin, and a significant positive genetic correlation was found with high-density lipoprotein (HDL) cholesterol. Disordered niacin metabolism leading to niacin deficiency was shown to provoke schizophrenia-like symptoms in neuropsychiatric diseases such as pellagra (11), which was seen as a secondary complication associated with a tryptophan-deficient diet in AN and BN (12, 13).
The gut, enteric nervous system, central nervous system, gut microbiome, and adipose tissue (AT) newly introduced as the AT-microbiome-gut-brain axis produce a variety of neuroactive factors with orexigenic and anorexigenic effects which are important in the regulation of food intake and body weight control (14–16) (Figure 1). The differential release of these compounds may act to initiate, maintain, or exacerbate cycles of food restriction or binge-purge behavior observed in AN and BN (17). In particular, translocation of intestinal bacterial antigens including enterobacterial caseinolytic protease B (ClpB) and food-derived antigens across the intestinal wall can trigger the production of autoantibodies cross-reacting with appetite-regulating hormones (18). This cross-reactivity is a phenomenon affecting the AT-microbiome-gut-brain axis.
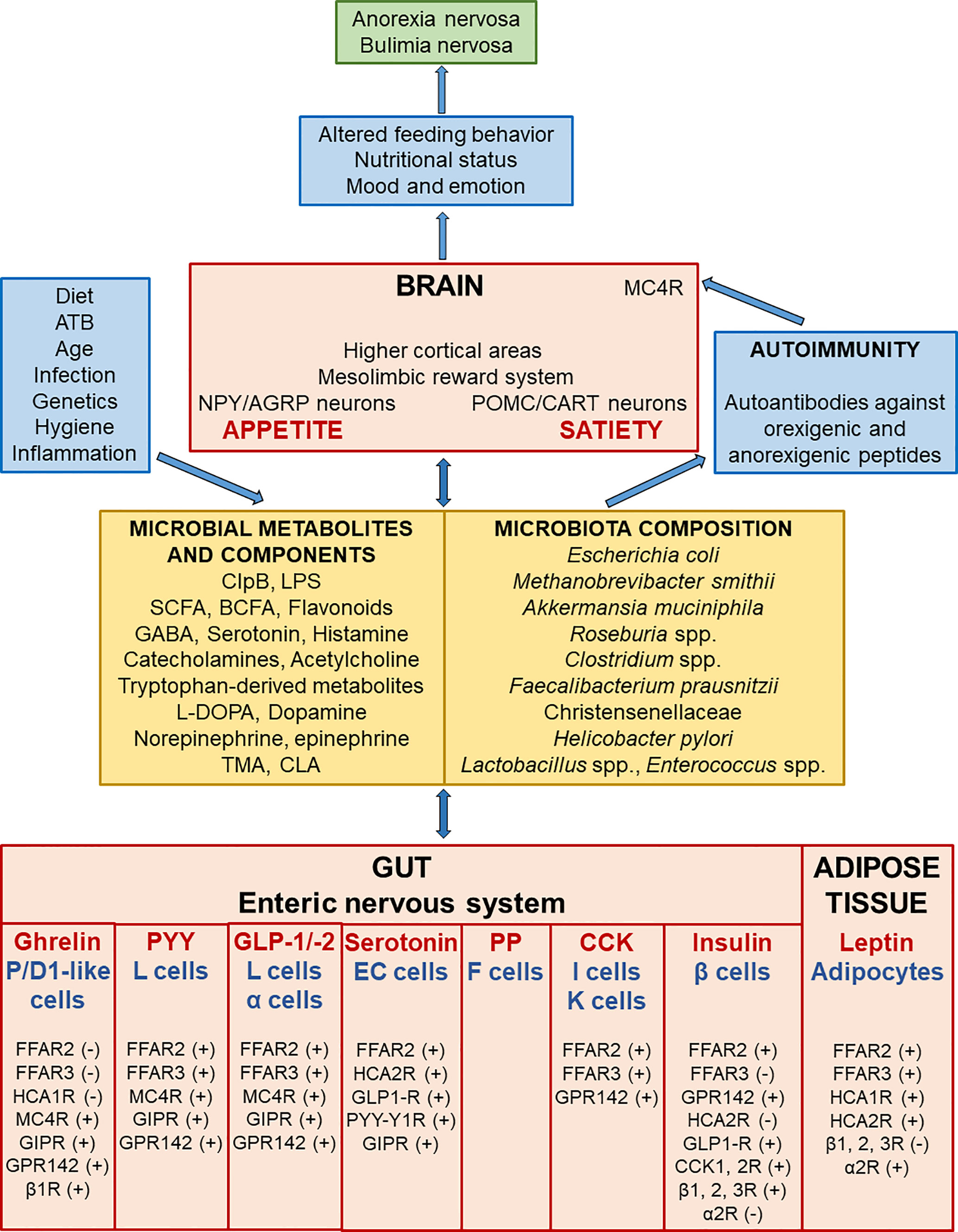
Figure 1 Scheme demonstrating the bi-directional interactions along the adipose tissue, microbiome, gut and brain leading to the development of eating disorders. Microbial composition and consequently the amount of microbial metabolites and components are affected by various factors like diet, antibiotics, infection and so on. Gut microbial metabolites and components act as signals to influence enteric nervous system and adipose tissue responses through various receptors. P/D1 like ghrelin cells in humans (or termed X/A like ghrelin cells in rodents) are localized in the oxyntic mucosa of the gastric fundus and duodenum. Enteroendocrine L cells secrete PYY and GLP-1/-2 (and/or co-release GLP-1/-2 together with PYY) in the mucosa of the distal ileum and colon. F (or PP) cells, which secrete pancreatic polypeptide (PP) under cholinergic control, are localized in the periphery of pancreatic islets of Langerhans, and also expressed in the distal gut. Enteroendocrine I and K cells, which secrete CCK and glucose-dependent insulinotropic peptide (GIP), are located in the mucosa of the upper small intestine. Short-chain fatty (FFA2-3) and hydroxy-carboxylic (HCA1-2) acid receptors are expressed on gastric P/D1 like ghrelin cells, ileal L cells, pancreatic α cells, enterochromaffin (EC) serotonin cells, duodeno-jejuno-ileal I and K cells, pancreatic β cells, and adipocytes. The signalization leads to ghrelin secretion inhibition or produce PYY, GLP-1/-2, serotonin, CCK, insulin, and leptin production. Leptin, an adipocyte-secreted hormone, is an indicator of energy stores and acts to reduce food intake and increase energy expenditure. These appetite-regulating hormones signal to NPY/AGRP and POMC/CART neurons, the mesolimbic reward system, and higher cortical areas, which all play a pivotal role in the regulation of metabolism. GABA has an inhibitory input from NPY/AGRP neurons to POMC/CART neurons in the hypothalamic arcuate nucleus. Activation of hypothalamic NPY/AGRP neurons stimulates hunger and inhibits energy expenditure and lipolysis in AT; however, stimulation of hypothalamic POMC/CART neurons together with MC4R leads to inhibition of food intake and enhancing of energy expenditure and lipolysis in AT. IgG immune complexes with orexigenic and anorexigenic peptides chronically activate MC4R leading to increased satiety in both AN and BN. Dysregulation of appetite-regulating circuits may affect altered feeding behavior leading to the onset, development, and maintenance of AN and BN. α2R, alpha-2 adrenoceptors; AGRP, agouti-related protein; AT, adipose tissue; β 1, 2, 3R, beta-1, 2, 3 adrenoceptors; BCFA, branched-chain fatty acids (isobutyrate, 2-methyl-butyrate, and isovalerate); ATB, antibiotics; CART, cocaine- and amphetamine-regulated transcript; CCK, cholecystokinin, CCK1, 2 R; cholecystokinin 1, 2 receptors; CLA, conjugated linoleic acid; ClpB, enterobacterial caseinolytic protease B; EC, enterochromaffin serotonin cells; FFAR, free fatty acid receptor; GABA, gamma-aminobutyric acid; GIP, glucose-dependent insulinotropic peptide; GIPR, glucose-dependent insulinotropic peptide receptor; GLP-1/-2, glucagon-like peptide-1 and 2; GLP1-R, glucagon-like peptide-1 receptor; GPR142, G protein receptor 142 for tryptophan, HCAR, hydroxy-carboxylic acid receptor; L-DOPA, L-3,4-dihydroxyphenylalanine; LPS, lipopolysaccharide; MC4R, melanocortin 4 receptor; NPY, neuropeptide tyrosine; POMC, pro-opiomelanocortin; PP, pancreatic polypeptide; PYY, peptide tyrosine tyrosine; PYY-Y1R, peptide tyrosine tyrosine-1 receptor, SCFA, short-chain fatty acids (butyrate, acetate, and propionate); TMA, trimethylamine; (+) = the stimulatory effect of ligands on hormone or serotonin secretion; (-) = the inhibitory effect of ligands on hormone secretion.
In the present review we show that the regulation of appetite, emotion, nutritional status, and adiposity is also under the control by secretion of autoantibodies directed against neuropeptides, neurotransmitters, and neuromodulators. This may lead to the onset, development, and perpetuation of severe food restriction or binge-eating behavior and psychopathological traits in eating disorders. Better understanding of the AT-microbiome-gut-brain axis in eating disorders and elucidation of its interactions with adipocyte lipolysis and adipogenesis may provide a novel therapeutic approach for treatment of anorexia and bulimia nervosa.
The goals of the present review were to: (i) describe the role of autoantibodies cross-reacting with appetite-regulating hormones and the gut microbiome in etiopathogenesis of AN and BN, and to (ii) discuss bi-directional communication along the AT-microbiome-gut-brain axis in eating disorders.
Involvement of Autoimmunity in AN and BN Pathogenesis
Various microorganisms have been shown to exhibit protein sequence homologies with some autoantigens including appetite-regulating peptides, which can lead to the production of autoantibodies (autoAbs) cross-reacting with these peptides and to the changed appetite regulation. Molecular mimicry concept was proposed to explain autoantibodies formation directed against microbial antigens and cross-reacting with host proteins, which can explain some microorganism-triggered autoimmune diseases (19, 20).
Such homology was reported for anorexigenic/anxiogenic peptide α-melanocyte-stimulating hormone (α-MSH) and bacterial protein Escherichia coli caseinolytic protease B (ClpB) (21). ClpB, α-MSH conformational mimetic produced by the bacterial Enterobacteriaceae family induces the production of antibodies cross-reacting with human α-MSH. In patients with AN, increased levels of IgM autoantibodies against α-MSH were detected (22). Another study showed lower levels of IgG autoantibodies against α-MSH in obese patients, but increased levels in anorectic and bulimic patients (23). Furthermore, IgG from patients with AN can form immunocomplexes with α-MSH, which chronically activate the melanocortin (MC) system involved in the feeding behavior regulation (24, 25). α-MSH signals via the MC type 4 receptor (MC4R), a key molecular pathway regulating appetite (23). This interaction may thus represent a pathophysiological trigger of both AN and BN (21).
α-MSH–reactive autoAbs as well as autoAbs directed against other appetite-regulating peptides are present also in the plasma of healthy people. Fetissov et al. (25) screened the plasma of healthy women for the presence of autoantibodies directed against 14 key appetite-regulating neuropeptides or peptide hormones including α-MSH, ACTH, NPY, ghrelin, leptin, insulin, or PYY suggesting a link between IgG and IgA classes of such autoantibodies and antigenic stimulation by gut microbiota in healthy subjects (25) (Table 1). High affinity autoantibodies are responsible for the neutralization of neuropeptides preventing them from immune complexes formation, while low affinity autoantibodies do not exhibit blocking properties and can bind neuropeptides reversibly and thus play a role in peptide transport or protection from degradation by peptidases (33, 34).
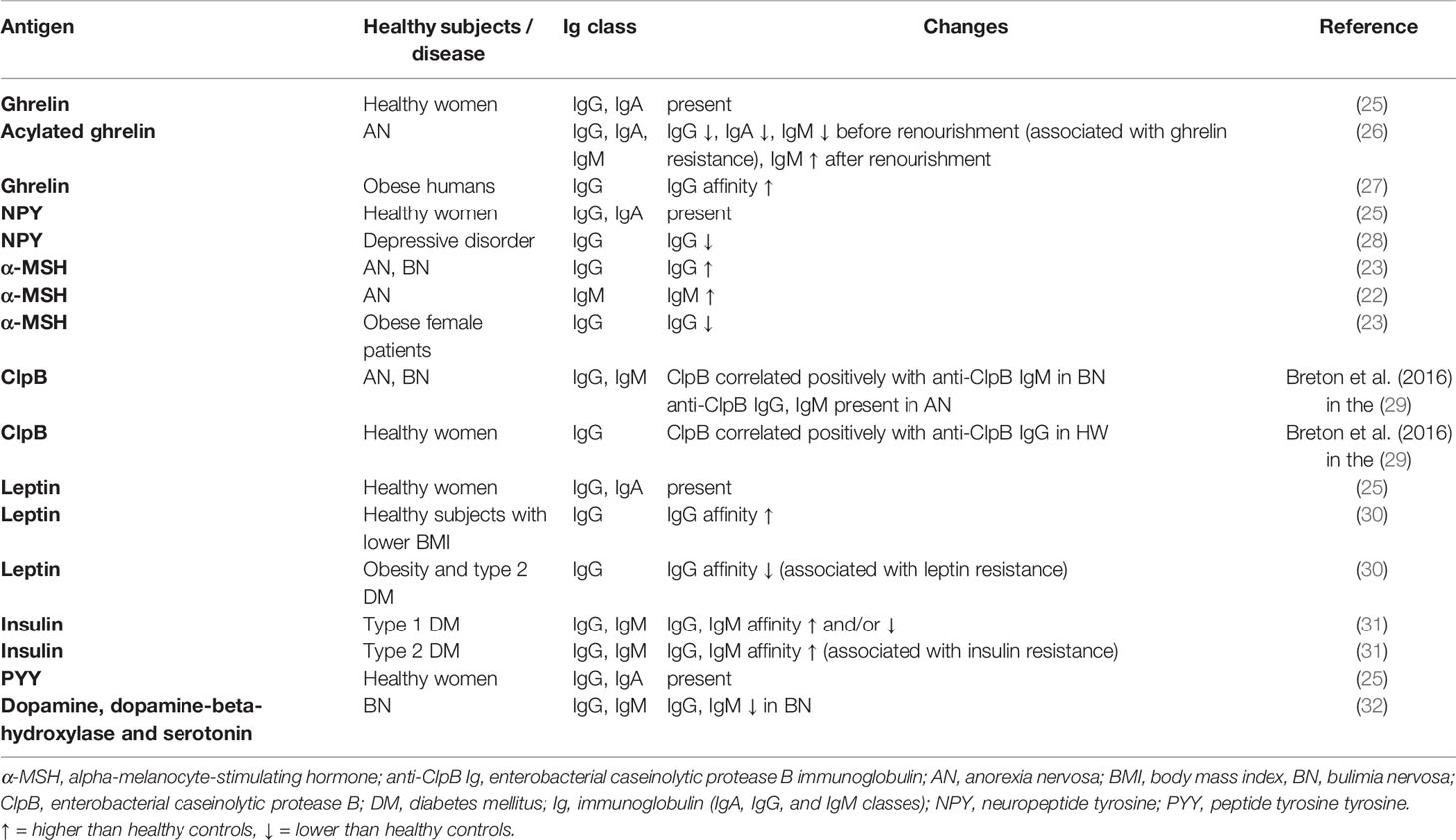
Table 1 Summary of changes in autoantibodies against appetite-regulating hormones, the ClpB-mimetic protein, and neurotransmitters in AN, BN, depression, in healthy subjects, in obesity, and diabetes.
Except higher levels of α-MSH (IgM class), higher levels of ACTH (IgG class) autoantibodies were also found in the plasma of patients with AN (22, 35). On the contrary, lower levels of acylated ghrelin (IgM class) autoantibodies (26) and lower levels of NPY (IgG class) autoantibodies in depressive disorder, a common comorbidity of eating disorders, were found (28). This is in contrast to increased levels of plasma NPY in BN and AN patients, which can act as a protective mechanism that prevents the exhaustion of energy reserves (36). Garcia et al. supported NPY protective role in depression by detection of decreased plasma levels of NPY IgG autoantibodies in patients with depression while their increased affinities were associated with lower body mass index (BMI) and reduced appetite (28).
IgG leptin-neutralizing autoantibodies were found in healthy subjects with a lower BMI; however, a decreased affinity of these antibodies was found in obese patients, which might be relevant to leptin resistance in obesity (30) (Table 1). Fetissov et al. reported that levels and affinities of autoantibodies against orexigenic and anorexigenic neuropeptides correlated with psychopathological traits in patients with eating disorders and these neutralizing autoantibodies were suggested as important attributors to mechanisms controlling motivation in AN and BN (22).
Moreover, immunoglobulin class switching of autoantibodies reacting with appetite-regulating hormones could be responsible for the differences in pathological manifestations of AN and BN.
In AN, the dysregulated immune profile includes an over-expression of anorexigenic and pro-inflammatory cytokines such as tumor necrosis factor-alpha (TNF-α), interleukin-6 (IL-6), and interleukin-1β (IL-1β). IL-1β and TNF-α influence the expression of certain crucial neuropeptides, which are known to be associated with anxiety states and AN. Importantly, it has been surmised that AN may result from an inability to produce neutralizing antibodies to TNF-α and/or IL-1β (37). Direct TNF-α, IL-1β, and IL-6 down-regulating monoclonal antibodies such as infliximab, adalimumab, etanercept, and tocilizumab as well as monoclonal antibodies against appetite-regulating hormones have not been evaluated as a treatment of AN and BN so far, although there is a strong theoretical rationale that could justify such a study (38, 39). Beneficial effects of anti-TNF-α therapy and an improvement in psychopathological traits in a case of AN with comorbid Crohn’s disease and with juvenile idiopathic arthritis were reported (40, 41).
In our previous studies, we observed in vivo increased sympathetic nervous system (SNS) activity, especially elevated norepinephrine (NE) concentrations in subcutaneous abdominal adipose tissue (AT) in AN and BN patients (36, 42, 43). NPY is synthesized in AT and co-localized with NE in perivascular sympathetic nerve fibers of the AT. NPY is also co-localized with NE and gamma-aminobutyric acid (GABA) in the brain. NPY amplifies growth hormone (GH) release, and stimulates appetite and lipogenesis (36, 44). Corcos et al. hypothesized that dopamine, dopamine-β-hydroxylase, and serotonin could be the antigenic cerebral targets reacting with “anti-brain” antibodies in BN (32). The role of up- or down-regulated neutralizing autoantibodies (IgM, IgG, and IgA classes), and changes of their affinity directed against appetite-regulating neuropeptides and neurotransmitters (dopamine, dopamine-beta-hydroxylase, and serotonin) in neuropeptidergic transmission was documented in the pathogenesis of eating disorders (26, 32) (Table 1). Moreover, a link between CNS neuroinflammation, autoimmunity, and neuropsychiatric disorders was reported (45–47).
“Leaky Gut” and the Blood-Brain Barrier Permeability in AN and BN
The gut microbiota transform dietary components, including macro- and micronutrients, fibers, and polyphenols, into a range of metabolites, including amino acid derivatives, vitamins, short-chain fatty acids (SCFA), and trimethylamines. These microbial-derived metabolites and dietary components can modulate host homeostasis, including gut and blood-brain barrier integrity (48–50).
SCFA (in particular butyrate, acetate, propionate), and other microbial metabolites can act on the intestinal epithelial barrier, the blood-brain barrier, and directly on brain neurons; they can regulate the endocrine and immune system to protect against the pathological inflammation (49). SCFA-producing gut microbiota was shown to up-regulate the expression of blood-brain barrier tight junction proteins occludin, claudin-5, and zonulin, and to reduce the permeability of the blood-brain barrier (51).
SCFA can mediate appetite reduction via increased POMC/CART neurotransmission of glutamatergic neurons and via decreased NPY/AGRP neurotransmission of GABAergic neurons in the hypothalamic arcuate nucleus (52, 53). Starvation and weight loss of AN patients may decrease the gut-barrier permeability (54) and increase the permeability of the blood-brain barrier through increased plasma free fatty acids levels and increased ketone bodies production (55, 56). Disruption of blood-brain barrier integrity in parallel with decreased expression of tight junction proteins occludin and claudin-5 have been also related to stress, post-streptococcal autoimmune disorders (PANDAS), and increased pro-inflammatory anorexigenic cytokines including TNF-α, IL-6, and IL-1β (37, 57–59). Autoantibodies against appetite-regulating peptides, and neurotransmitters can also disrupt the blood-brain barrier permeability and the gut-barrier permeability referred to as “leaky gut” underlying low-grade inflammation in AN and BN patients (18, 24). Indeed, penetration of circulating neuropeptides to the brain may be assisted by neuropeptide autoantibodies (60).
It is believed that the access of high-affinity autoantibodies against appetite-regulating neuropeptides and peptides to the brain centers, otherwise protected by the blood-brain barrier, can trigger the development of AN and BN. The increased affinity of plasmatic IgG for acyl-ghrelin in obesity was associated with increased ghrelin function, while increased plasma IgG/α-MSH affinity in obesity was shown to decrease activation of MC4R (23, 61). Monteleone et al. reported a decrease of intestinal permeability in the small intestine by measuring lactulose/mannitol absorption in AN patients (54). Jésus et al. observed increased colonic permeability with decreased expression of the tight junction protein claudin-1 in an activity-based anorexia model in mice (62). Methotrexate-induced intestinal inflammation was shown to acutely disrupt the gut-barrier permeability and induce anorexia in rats (63). Coquerel et al. linked intestinal inflammation to the production of autoantibodies against neuropeptides and showed that changes in anti-α-MSH autoAb plasma levels may participate in the body weight control relevant to the pathophysiology of AN (64).
Intestinal fatty acid binding protein (I-FABP) was proposed as a biomarker for small intestinal epithelial damage and subsequently for potentially altered gut permeability in Crohn’s and celiac diseases (65, 66). It is a small (14-15 kD) protein, which constitutes up to 2% of the cytoplasmic protein content of mature enterocytes (67). Upon death of the enterocyte, its cytoplasmic content is liberated into the circulation. I-FABP is present in very small amounts in the plasma of healthy individuals, probably representing the normal turnover of enterocytes, but its levels rise rapidly after episodes of acute intestinal ischemia and inflammation.
We determined a significantly increased I-FABP level in patient with severe and enduring AN suffering from the small intestinal bacterial overgrowth syndrome. Patient treatment with fecal microbiota transplantation (FMT) led to an improvement of the gut barrier function reflected by a decrease in I-FABP levels within 6 months post-FMT with non-detectable values 1 year post-FMT (68).
The Ghrelin, Leptin, and Melanocortin System in AN and BN
Ghrelin is a 28-amino-acid peptide produced mainly by the neuroendocrine cells named P/D1 in humans in the oxyntic mucosa of the gastric fundus, and to a far lesser extent in the duodenum, and also in the epsilon pancreatic islet cells (69, 70). Ghrelin is cleaved from the 117-amino-acid preproghrelin protein encoded by the human ghrelin gene on chromosome 3p25-26. Two major molecular forms of ghrelin were found in the stomach and plasma, i.e. acyl ghrelin with n-octanoylated serine in position 3 attached by the GOAT (Ghrelin O-Acetyltransferase), and des-acyl ghrelin. Acyl ghrelin is involved in the regulation of growth hormone (GH) secretion, energy homeostasis, gastric emptying, cardiac performance, cardiac output and contractility, antidepressant-like and anxiolytic responses (71–73). It was reported that in contrast to acyl ghrelin, des-acyl ghrelin induces a negative energy balance by decreasing food intake and delaying gastric emptying (71, 74). The physiological role of ghrelin in food intake regulation is reflected by an increase in its plasma level before eating and its decrease after the meal (75).
Thus, des-acyl ghrelin does not bind to growth hormone secretagogue receptor type 1a (GHS-R1a). Moreover, it has anti-ghrelin effects including the loss of ghrelin’s appetite-stimulating effect via increasing expression of melanocortin 4 receptor (MC4R) in the hypothalamic arcuate nucleus (76). It was found that des-acyl ghrelin level was higher in symptomatic AN patients than in healthy controls, which may elucidate why AN patients report being less hungry compared to healthy women. On the other hand, the des-acyl ghrelin level was lower in AN patients after renourishment than in heathy women (71, 77).
Acyl ghrelin binds to GHS-R1a and its plasma levels have been documented to be decreased in AN when compared to age-matched and weight-healthy women (71, 78, 79). Therefore, treatment with acyl ghrelin and/or Relamorelin, a pentapeptide ghrelin receptor agonist, may be useful for stimulating appetite, gastric emptying, and weight regain in AN patients (80).
Plasma total ghrelin levels are increased in patients with AN; however, anorexic patients report less hunger when compared to healthy women. This discordance may be explained on the basis of ghrelin resistance in anorectic women (71, 78) or a changed acyl/des-acyl ghrelin ratio and/or ghrelin reactive autoantibodies (77, 81). Patients with AN display lower levels of autoantibodies (IgG) against acyl-ghrelin and higher levels of autoantibodies against des-acyl ghrelin present in immune complexes compared to healthy controls. Moreover, negative correlations between plasma ghrelin autoantibodies (IgG) and ghrelin peptides were found. The observed decrease in the levels of bioavailable ghrelin autoantibodies (IgG) was suggested to lead to increased ghrelin levels and ghrelin resistance in patients with AN (26) (Table 1). Moreover, a decrease in IgM and IgA classes of acyl ghrelin autoantibodies in AN was also detected (26). Subsequently, the refeeding of AN patients led to an increase in IgM acyl ghrelin autoantibodies levels, which may indicate new antigenic stimulation resulting from realimentation-induced changes in the gut-barrier permeability. Furthermore, high affinity anti-ghrelin IgG autoantibodies were proposed to enhance ghrelin’s orexigenic effect, which may contribute to increased appetite and overeating and may enhance the bioactivity of endogenous or exogenous ghrelin in obese patients (27, 61) (Table 1). In addition, this shows that ghrelin degradation is inhibited by these autoantibodies, i.e. by forming ghrelin-IgG immune complexes in obese patients (27).
The presence of immune complexes prevents des-acyl ghrelin from occurring with a decrease of the free fraction of autoantibodies binding ghrelins resulting in elevated levels of free acylated ghrelin in AN patients, and eventually in ghrelin resistance in AN (26). High-affinity insulin autoantibodies have been proposed to be involved in a mechanism underlying severe insulin resistance after insulin administration (31) and have also been also studied as a marker of type 1 diabetes (Table 1). Low-affinity autoantibodies against insulin may influence the levels of bioavailable insulin with potential effects on hypoglycemia (31). Using a homeostasis model assessment of insulin resistance (HOMA-IR), significantly lower values of HOMA-IR in malnourished and underweight patients with AN were found when compared to healthy controls (82). However, refeeding led to the onset of insulin resistance in patients with AN (83). Indeed, the onset of type 1 diabetes in adolescence seems to place female patients at risk for the subsequent development of AN and BN (5, 6, 78, 84). Importantly, a decrease in leptin-reacting immunoglobulin affinity kinetics may also be related to hyperinsulinemia, insulin resistance, and leptin resistance in patients with type 2 diabetes (30). Intravenous injection of leptin-neutralizing antibodies was reported to induce hyperinsulinemia in mice (85). Conversely, an increase in IgG affinity kinetics for leptin was found in healthy controls with lower BMI suggesting an enhancing role of IgG in leptin transduction with anorexigenic and antidiabetic properties (30) (Table 1).
Obestatin, a 23-amino-acid peptide cleaved of the pro-hormone preproghrelin, appears to function as a part of the anorexigenic gut-brain axis that decreases food intake and reduces body weight in rats (86). Obestatin has been postulated to antagonize ghrelin action on energy balance and gastrointestinal function. However, controversies exist as regards its specific effects on food intake in animals and humans (71, 87). Patients with AN displayed increased circulating levels of both obestatin and ghrelin and an increased ghrelin/obestatin ratio, whereas patients with BN did not (88).
Gastric ghrelin stimulates appetite, while gut hormones pancreatic polypeptide (PP) and peptide tyrosine tyrosine (PYY) have the opposite effect on the hypothalamic level. PP and PYY, 36 amino acid peptides, are secreted from pancreatic F cells and enteroendocrine L-cells following meals, respectively (89, 90). Importantly, G protein receptor 142 for tryptophan (GPR142) is expressed on gastro-enteroendocrine and pancreatic islet cells to stimulate ghrelin, PYY, glucagon-like peptide-1 (GLP-1), cholecystokinin (CCK), and insulin secretagogue activities, respectively (91, 92) (Figure 1). It was shown that SCFA including butyrate and lactate are ligands of FFA2, FFA3, and HCA1 receptors which are expressed on gastric ghrelin cells and ileal L cells. Their activation reduces ghrelin secretion and increases PYY secretion, respectively (69, 73, 93). Furthermore, PYY and GLP-1 stimulate serotonin (5-hydroxytryptamine, 5-HT) secretion from small intestinal and colonic enterochromaffin (EC) cells (94) (Figure 1). The SCFA receptors FFA2, FFA3, and HCA1 were found in AT where they increase the secretion of the anorexigenic hormone leptin (95, 96), and the blood-brain barrier is endowed with FFA3 (97). Interestingly, an endogenous ligand for HCA2 and FFA2 receptor is 3-hydroxy-butyrate. Thus, FFA2 and HCA2 receptors are activated by the endogenous ligand 3-hydroxy-butyrate as well as the exogenous ligand anti-dyslipidemic drug niacin having protective effects of the prebiotic fiber-derived butyrate in the gut-barrier permeability (42, 98, 99). We documented reduced ghrelin levels and increased PP, PYY, and leptin levels after administration of the niacin-like anti-dyslipidemic drug Olbetam in bulimic patients when compared to healthy-weight Czech women (42).
The subpeptide PYY3-36 is the major form of PYY in the circulation. This peptide reduces food intake in humans. In AN, unlike ghrelin, plasma levels of anorexigenic PYY are paradoxically increased (100). Elevated levels of PYY might contribute to decreased food intake and disordered eating psychopathology in AN. PYY levels remain elevated despite renourishment and weight regain (101, 102). Healthy humans showed a negative correlation between ghrelin plasma concentrations and BMI (103) and a negative correlation of PYY and body weight (104). However, two independent research groups documented that BN patients, despite of higher BMI, had increased plasma ghrelin levels before food ingestion with a decreased response of ghrelin after food ingestion (105, 106). In those patients with BN, the increase of plasma PYY levels after food ingestion was also blunted. Depressed and blunted PYY levels may result from reduced and impaired CCK secretion in BN. The anorexigenic hormone CCK is a stimulant of PYY secretion (107). PYY3-36 is known as meal terminator opposed to ghrelin considered as meal initiator in the feeding behavior. The suppression of plasma ghrelin and the increase of plasma PYY3–36 after food ingestion may indicate compensatory activation of peripheral signals promoting termination of food ingestion in healthy humans. Thus, the altered CCK, PYY, and ghrelin response to food intake may play a role in the perpetuating post-binge eating behavior in bulimic patients (108).
Ghrelin has an important role in regulation of energy homeostasis and appetite by acting centrally through GHS-R1a or via vagal afferents. Furthermore, ghrelin can activate hypothalamic GABAergic arcuate neurons that secrete the orexigenic peptides NPY and the agouti-related peptide (AGRP). It can inhibit anorexigenic neurons secreting α-MSH resulting in higher energy intake to be induced by increased GABA-mediated inhibitory inputs from NPY/AGRP neurons to hypothalamic glutamatergic arcuate neurons, which express anorexigenic pro-opiomelanocortin (a precursor of α-MSH), and cocaine- and amphetamine-regulated transcript (POMC/CART) (42, 89). Anorexigenic/anxiogenic α-MSH is a 13-amino-acid-long neuropeptide derived from POMC. Activation of POMC neurons leads to stimulation of the melanocortin satiety pathway. Cone has demonstrated that the central melanocortin system operating through α-MSH on MC4R provides the final common pathway signaling satiety (109). High plasma levels and changes of affinity kinetics of autoantibodies reacting with α-MSH and ACTH seem to be caused by the exposure to stress as a result of concomitant hypothalamic-pituitary-adrenal (HPA) axis activation (110, 111). These results support the hypothesis that changes in affinity of autoantibodies reacting with α-MSH and ACTH are involved in the pathogenesis of AN and BN and that increased levels of high-affinity anti-α-MSH or anti-ClpB (α-MSH conformational mimetic produced by Enterobacteriaceae) autoantibodies can induce bulimia, while increased levels of low-affinity anti-α-MSH autoantibodies can induce anorexia (24, 110, 112) (Table 1).
α-MSH and ClpB may exert a dual effect on the anorexigenic/orexigenic pathway. A key role in appetite regulation is played by the melanocortin 4 receptor (MC4R), which is activated by its main ligand α-MSH in both peripheral and central sites. In this vein, α-MSH and ClpB can induce the activation of MC4R expressed on intestinal enteroendocrine L cells which regulate the release of satiating hormones PYY or GLP-1/-2 leading to activation of the POMC neurons releasing α-MSH via the vagal and endocrine pathways (29, 113, 114) (Figure 1). Surprisingly, α−MSH can also induce activation of MC4R expressed on gastric ghrelin cells which stimulate orexigenic hormone ghrelin secretion (73, 92, 93) (Figure 1). In AN patients, plasma α-MSH were significantly lower all over the day. Thus, lower circadian α-MSH levels integrate the adaptive profile of appetite regulation in AN (115).
As mentioned above, the gut microbiota serving as a direct source of antigens was shown to produce molecules that share similar sequence and conformational homologies with some neuroactive peptides (25). Healthy humans display IgG and IgA autoantibodies directed against appetite-regulating hormones and neuropeptides, such as leptin, ghrelin, PYY, neuropeptide Y and others. These neuropeptides share sequence homology with various peptides produced by some commensal and pathogenic microorganisms including Lactobacilli, Bacteroides, Helicobacter pylori, Escherichia coli, and Candida species. The autoAbs may thus affect hunger and satiety pathways (25). The presence of H. pylori was also associated with decreased adiposity, high levels of stomach leptin, and insulin resistance. On the other hand, decreased ghrelin and increased obestatin were found after H. pylori eradication (116, 117). Psychological stress was shown to alter the gut microbiome, e.g. to decrease Bacteroides and to increase Clostridium abundance (118). Certain bacterial proteins of Clostridium perfringens and Enterococcus faecalis were shown to have sequence homology with orexigenic ghrelin (24, 25).
Appetite-stimulating hormone ghrelin (increased in AN) was associated with greater levels of Bacteroides and Prevotella and reduced levels of Bifidobacterium and Lactobacillus. Simultaneously, appetite-suppressing hormone leptin (decreased in AN) showed an inverse association with reduced levels of Bacteroides and Prevotella and higher levels of Bifidobacterium and Lactobacillus in rats (119). In another study incorporating an activity-based anorexia mouse model mimicking the core features of AN, bacterial taxa that correlate positively or negatively with body weight, food intake, and fat mass as well as with hypothalamic mRNA levels of orexigenic NPY and satiety inducer POMC, were identified (120).
Recently, Schalla & Stengel (121) discussed the link between ghrelin and gut microbiota. They surmised that positive effectors such as exercise, prebiotics, probiotics, and food supplements are efficient to increase Blautia cocoides, Bacteroidetes/Firmicutes ratio, Faecalibacterium, Prevotellaceae, Streptococcus, Escherichia coli, Shigella, and SCFA leading to a suppression of plasma acylated ghrelin and a decrease of GHS-R1a-induced food intake and weight regain. Conversely, negative effectors including the gut dysbiosis, food restriction, fasting, antibiotics, and pesticides are able to stimulate Coriobacteriaceae, Veillonellaceae, Clostridium sensu stricto 1, Ruminococcus, Prevotella, and Coprococcus, which may result in an increased plasma acylated ghrelin and its orexigenic and the obesogenic side effects (121).
The Gut Microbiome in AN and BN
It is now generally accepted that the immune and nervous systems maintain a state of systemic homeostasis by continuous communication. The gut microbial content plays an important role in this communication. Disruption of the pathways connecting gut and brain can lead to various psychopathologies (122, 123). In our studies we described the role of gut microbiota and the gut-barrier permeability in the pathogenesis of inflammatory, autoimmune diseases, including neurological, and psychiatric diseases (19, 124–126). Kleiman et al. showed that the intestinal microbiota plays a role in key features of AN, including weight regulation, energy metabolism, anxiety, and depression as well as a role in the development, maintenance, and recovery from BN (127).
Microbial diversity seems to be essential for health and disease prevention (14, 15). The predominant bacterial phyla in the human gut microbiome are obligate anaerobes Bacteroidetes (e.g. genera Bacteroides and Prevotella) and Firmicutes (e.g. genera Lactobacillus, Clostridium, Enterococcus, and Streptococcus), and facultative anaerobes present in lesser abundance such as Actinobacteria (e.g. Bifidobacteria), Proteobacteria (e.g. Escherichia coli), Verrucomicrobia (e.g. Akkermansia muciniphila), and Archaea (e.g. Methanobrevibacter smithii) (128). Microbiome dysbiosis is characterized by either expansion of pathobionts, loss of commensals, loss of microbial diversity, or their combinations (129). There are conflicting results regarding specific changes in microbiome composition in patients with AN (Table 2). Current research and microbiota signature associated with acute ill AN patients show a relative depletion of Firmicutes (e.g. Roseburia, Clostridium, Anaerostipes, and Faecalibacterium prausnitzii) for the benefit of Bacteroidetes (133, 134, 136–138) together with increased abundance in the archeon Methanobrevibacter smithii (130, 131, 134, 136), the mucin-degrader Akkermansia muciniphila (134, 139) and Proteobacteria (Escherichia coli) (131, 136) (Table 2).
Simultaneously, inconsistent results were reported on bacterial alpha and beta diversity in AN. The gut microbiome exerted lower alpha microbial diversity (describes intra-sample variance) in five studies in underweight AN patients (133, 135, 137, 138, 140); in three additional studies, no difference in alpha diversity was found (126, 134, 136). A significant increase in alpha microbial diversity after weight rehabilitation of patients with AN was shown in two studies (133, 134). Recently, we measured parameters of microbial alpha diversity and detected only an increased Chao 1 index in patients with AN before their renourishment considering their interindividual variation. In this study, weight gain in patients with AN led to a modification of the Chao 1 index which reached healthy control values (126).
Furthermore, differences in beta microbial diversity (describes inter-sample variation) were found in three studies showing higher heterogeneity in AN patients (126, 133, 134). This beta microbial diversity was modified during weight regain in AN patients (126, 133). Bacterial composition of the control and of patients with AN was similar in two studies (136, 138).
Various studies of the gut microbiota in patients with AN revealed an increase in Methanogens (e.g. Methanobrevibacter smithii), while Lactobacillus species were linked to obese patients (130, 131). M. smithii is known to recycle and convert hydrogen and carbon dioxide to methane, increase the transformation of nutrients to calories by free hydrogen reduction in the colon, increase the fermentation of prebiotic fiber and resistant starch generating SCFA (butyrate, acetate, and propionate), thus increasing energy harvest. Methanogens in AN may be thus associated with an adaptive response to very low caloric diet (130) (Table 2). However, M. smithii may contribute to delay gastric emptying and constipation in AN (78). Archaeal family Methanobacteriaceae co-occur with the bacterial family Christensenellaceae and are more abundant in lean individuals with lower BMI (141). Christensenella spp. can efficiently support the metabolism of M. smithii by H2 production (142).
FMT of Christensenella minuta to microbiome-lacking mice, i.e. germ-free mice, led to weight gain and adiposity reduction suggesting a role of the gut microbiome in the altered metabolism of AN (141). Furthermore, FMT from lean donors increased insulin sensitivity in patients with the metabolic syndrome and obesity-associated insulin resistance (143). Conversely, FMT from obese mice to germ-free mice led to greater adiposity and increased weight gain indicating that manipulation of gut microbiome might be a possible approach in the treatment of obesity (144, 145).
Lactobacillus intake may be associated with weight gain, anxiolytic or antidepressant effects and may reduce intestinal permeability. In a recent study, Lactobacillus rhamnosus decreased anxiety and depression and reduced stress-induced ACTH and corticosterone levels in mice. This study demonstrated that these effects are dependent on the vagus nerve and that parasympathetic innervation is necessary for Lactobacillus rhamnosus participation in the gut microbiota-brain interaction (146). Consumption of Bifidobacterium species by rats was found to change serotonin metabolism in the brain (147).
Bacterial species produce a number of neuroactive compounds including serotonin (Bacillus spp., Lactobacillus plantarum, Clostridium ramosum, and Escherichia coli), dopamine, the major disruptor of the mesolimbic-neocortical reward circuit in the brain (Lactobacillus plantarum, Clostridium spp., Escherichia coli, Bacillus spp., Serratia spp.), norepinephrine (Clostridium spp., Escherichia coli, and Bacillus spp.), and acetylcholine (Lactobacillus plantarum), and they synthesize the inhibitory neurotransmitter GABA from glutamate, reducing anxiety and stress (Bacteroides, Escherichia coli, Lactobacillus reuteri, Bifidobacterium, Lactobacillus rhamnosus, Lactobacillus brevis, and Lactobacillus plantarum) (146, 148–158). These microbially-derived neurotransmitters may induce intestine epithelial cells to release molecules that in turn modulate neural signaling within the enteric nervous system and consequently signal brain function and host behavior. Mood and depressive-like behavior regulators include serotonin which is an important neurotransmitter implicated in psychiatric disorders including AN and BN. Hata et al. observed significantly lower brainstem serotonin levels in anorectic mice, which may be associated with reduced tryptophan intake resulting from restricted food intake (159). Recently, Prochazkova et al. (126) detected lower levels of serotonin, dopamine and GABA in fecal samples of patients with AN when compared with healthy women.
The central nervous system (CNS) modulation by microbiota occurs primarily through neuroimmune and neuroendocrine mechanisms. Except neurotransmitters and hormones, this communication is mediated by gut microbial metabolites, including SCFA, bile acids, and tryptophan metabolites. SCFA are generated by microbial fermentation of non-digestible colon polysaccharides.
Overall, there are inconsistent results for fecal concentrations of SCFA and branched-chain fatty acids (BCFA; isobutyrate, 2-methyl-butyrate, and isovalerate) in AN patients (132, 134, 136, 160). In our study, we detected reduced butyrate and acetate in AN, which were not changed after weight recovery (126). Reduced levels of acetate and propionate were found in another study (132), while Borgo et al. found decreased butyrate and propionate concentrations in patients with AN (136).
FMT is a therapeutic procedure to modify the recipient’s gut microbiota. FMT is commonly used for the treatment of recurrent pseudomembranous colitis caused by toxin-producing Clostridium difficile (161). Moreover, FMT was also used to alleviate chronic intestinal pseudo-obstructive syndrome (CIPO) mimicking mechanical intestinal obstruction (162, 163) or the small intestinal bacterial overgrowth syndrome (SIBO). SIBO is a gastrointestinal disorder diagnosed as an excessive and/or abnormal bacterial colonization in the small intestine (more than 105 colony-forming units of bacteria per mL of jejunal aspirate) associated with various metabolic disorders and serious malnutrition found also in patients with AN (68, 163) who suffer from delayed gastric emptying and constipation (164, 165).
Conclusions
Various stressors, especially infectious, but also components of diet, mental stress, and others can modify the gut and the blood-brain barrier function leading to the production of antibodies directed against microbial compounds and cross-reacting with human neuropeptides and neurotransmitters. The interplay between the gut microbiome, immune, hormonal, behavioral, and emotional regulation provides a complex mechanism underlying AN pathophysiology as well as other neuropsychiatric diseases. Immunization against ClpB could be validated as a potential preventive and therapeutic option for AN and BN. The current long-term pharmacological therapy of AN and BN patients is rather inefficient, is associated with adverse side effects, and given that these disorders tend to relapse. New approaches to prevention and therapy could be suggested. The gut microbiota modulation realized by lifestyle changes and by application of prebiotics, probiotics (psychobiotics), FMT could represent an useful tool for prevention and treatment of eating and other neuropsychiatric disorders.
Author Contributions
KS, PP, RR, JD, HP, OK, MB, and HT-H: conceptualization. All authors: writing-original draft preparation, editing, and revising. HT-H: supervision. All authors contributed to the article and approved the submitted version.
Funding
This study was supported by the grant No. 17-28905A provided by the AZV Grant Agency of the Ministry of Health, Czech Republic.
Conflict of Interest
The authors declare that the research was conducted in the absence of any commercial or financial relationships that could be construed as a potential conflict of interest.
The reviewer LP declared a shared affiliation with several of the authors to the handling editor at time of review.
Abbreviations
ACTH, adrenocorticotropin; AGRP, agouti-related protein; α-MSH, alpha-melanocyte-stimulating hormone; AN, anorexia nervosa; anti-ClpB Ig, enterobacterial caseinolytic protease B immunoglobulin; ARC, arcuate nucleus; AT, adipose tissue; autoAbs, autoantibodies; BCFA, branched-chain fatty acids; BMI, body mass index; BN, bulimia nervosa; CART, cocaine- and amphetamine- regulated transcript; CIPO, chronic intestinal pseudo-obstruction syndrome; ClpB, enterobacterial caseinolytic protease B; CNS, central nervous system; EC, enterochromaffin serotonin cells; FFA, free fatty acids; FFAR, free fatty acid receptor; FMT, fecal microbiota transplantation; GABA, gamma-aminobutyric acid; GH, growth hormone; GHS-R1a, growth hormone secretagogue receptor type 1a; GLP-1, glucagon-like peptide-1; GLP-2, glucagon-like peptide-2; GOAT, ghrelin O-acyltransferase; HCA, hydroxy-carboxylic acid; HDL, high-density lipoprotein; HOMA-IR, homeostasis model assessment of insulin resistance; HPA, hypothalamic-pituitary-adrenal axis; 5-HT, 5-hydroxytryptamine; HW, healthy women; I-FABP, intestinal fatty acid binding protein; Ig, immunoglobulin; Ig, immunoglobulin (IgA, IgG, and IgM classes); IL-1β, interleukin-1 beta; IL-6, interleukin-6; MC4R, melanocortin 4 receptor; NE, norepinephrine; NPY, neuropeptide tyrosine; PANDAS, Pediatric Autoimmune Neuropsychiatric Disorders Associated with Streptococcal infection; POMC, pro-opiomelanocortin; PP, pancreatic polypeptide; PYY, peptide tyrosine tyrosine; SCFA, short-chain fatty acids; SIBO, small intestinal bacterial overgrowth syndrome; SNS, sympathetic nervous system; TNF-α, tumor necrosis factor-alpha.
References
1. Hsu LK. Epidemiology of the eating disorders. Psychiatr Clin North Am (1996) 19:681–700c. doi: 10.1016/S0193-953X(05)70375-0
2. Arcelus J, Mitchell AJ, Wales J, Nielsen S. Mortality rates in patients with anorexia nervosa and other eating disorders. A meta-analysis of 36 studies. Arch Gen Psychiatry (2011) 68:724–31. doi: 10.1001/archgenpsychiatry.2011.74
3. American Psychiatric Association. Diagnostic and Statistical Manual of Mental Disorders, 5th ed. (DSM-5R ). 5th ed. Washington, DC: American Psychiatric Association Publishing (2013). doi: 10.1176/appi.books.9780890425596
4. Fetissov SO, Hallman J, Oreland L, Af Klinteberg B, Grenbäck E, Hulting AL, et al. Autoantibodies against alpha -MSH, ACTH, and LHRH in anorexia and bulimia nervosa patients. Proc Natl Acad Sci U S A (2002) 99(26):17155–60. doi: 10.1073/pnas.222658699
5. Raevuori A, Haukka J, Vaarala O, Suvisaari JM, Gissler M, Grainger M, et al. The increased risk for autoimmune diseases in patients with eating disorders. PloS One (2014) 9(8):e104845. doi: 10.1371/journal.pone.0104845
6. Takii M, Uchigata Y, Kishimoto J, Morita C, Hata T, Nozaki T, et al. The relationship between the age of onset of type 1 diabetes and the subsequent development of a severe eating disorder by female patients. Pediatr Diabetes (2011) 12:396–401. doi: 10.1111/j.1399-5448.2010.00708.x
7. Hedman A, Breithaupt L, Hübel C, Thornton LM, Tillander A, Norring C, et al. Bidirectional relationship between eating disorders and autoimmune diseases. J Child Psychol Psychiatry (2019) 60:803–12. doi: 10.1111/jcpp.12958
8. Zerwas S, Larsen JT, Petersen L, Thornton LM, Quaranta M, Koch SV, et al. Eating Disorders, Autoimmune, and Autoinflammatory Disease. Pediatrics (2017) 140:e20162089. doi: 10.1542/peds.2016-2089
9. Hommer RE, Swedo SE. Anorexia and Autoimmunity: Challenging the Etiologic Constructs of Disordered Eating. Pediatrics (2017) 140:e20173060. doi: 10.1542/peds.2017-3060
10. Watson HJ, Yilmaz Z, Thornton LM, Hübel C, Coleman JRI, Gaspar HA, et al. Genome-wide association study identifies eight risk loci and implicates metabo-psychiatric origins for anorexia nervosa. Nat Genet (2019) 51:1207–14. doi: 10.1038/s41588-019-0439-2
11. Periyasamy S, John S, Padmavati R, Rajendren P, Thirunavukkarasu P, Gratten J, et al. Association of Schizophrenia Risk With Disordered Niacin Metabolism in an Indian Genome-wide Association Study. JAMA Psychiatry (2019) 76:1026–36. doi: 10.1001/jamapsychiatry.2019.1335
12. Portale S, Sculati M, Sanford FC, Cena H. Pellagra and anorexia nervosa: a case report. Eat Weight Disord (2020) 25:1493–96. doi: 10.1007/s40519-019-00781-x
13. Haleem DJ. Improving therapeutics in anorexia nervosa with tryptophan. Life Sci (2017) 178:87–93. doi: 10.1016/j.lfs.2017.04.015
14. Roubalová R, Procházková P, Papežová H, Smitka K, Bilej M, Tlaskalová-Hogenová H. Anorexia nervosa: Gut microbiota-immune-brain interactions. Clin Nutr (2020) 39:676–84. doi: 10.1016/j.clnu.2019.03.023
15. Cryan JF, O’Riordan KJ, Cowan CSM, Sandhu KV, Bastiaanssen TFS, Boehme M, et al. The Microbiota-Gut-Brain Axis. Physiol Rev (2019) 99:1877–2013. doi: 10.1152/physrev.00018.2018
16. Lundgren P, Thaiss CA. The microbiome-adipose tissue axis in systemic metabolism. Am J Physiol Gastrointest Liver Physiol (2020) 318(4):G717–24. doi: 10.1152/ajpgi.00304.2019
17. Prince AC, Brooks SJ, Stahl D, Treasure J. Systematic review and meta-analysis of the baseline concentrations and physiologic responses of gut hormones to food in eating disorders. Am J Clin Nutr (2009) 89:755–65. doi: 10.3945/ajcn.2008.27056
18. Fetissov SO. Role of the gut microbiota in host appetite control: bacterial growth to animal feeding behaviour. Nat Rev Endocrinol (2017) 13(1):11–25. doi: 10.1038/nrendo.2016.150
19. Tlaskalová-Hogenová H, Stepánková R, Hudcovic T, Tucková L, Cukrowska B, Lodinová-Zádníková R, et al. Commensal bacteria (normal microflora), mucosal immunity and chronic inflammatory and autoimmune diseases. Immunol Lett (2004) 93(2-3):97–108. doi: 10.1016/j.imlet.2004.02.005
20. Oldstone MB. Molecular mimicry, microbial infection, and autoimmune disease: evolution of the concept. Curr Top Microbiol Immunol (2005) 296:1–17. doi: 10.1007/3-540-30791-5_1
21. Fetissov SO, Hökfelt T. On the origin of eating disorders: altered signaling between gut microbiota, adaptive immunity and the brain melanocortin system regulating feeding behavior. Curr Opin Pharmacol (2019) 48:82–91. doi: 10.1016/j.coph.2019.07.004
22. Fetissov SO, Harro J, Jaanisk M, Järv A, Podar I, Allik J, et al. Autoantibodies against neuropeptides are associated with psychological traits in eating disorders. Proc Natl Acad Sci U S A (2005) 102:14865–70. doi: 10.1073/pnas.0507204102
23. Lucas N, Legrand R, Bôle-Feysot C, Breton J, Coëffier M, Akkermann K, et al. Immunoglobulin G modulation of the melanocortin 4 receptor signaling in obesity and eating disorders. Transl Psychiatry (2019) 9(1):87. doi: 10.1038/s41398-019-0422-9
24. Fetissov SO, Déchelotte P. The new link between gut-brain axis and neuropsychiatric disorders. Curr Opin Clin Nutr Metab Care (2011) 14:477–82. doi: 10.1097/MCO.0b013e32834936e7
25. Fetissov SO, Sinno MH, Coëffier M, Bole-Feysot C, Ducrotte P, Hökfelt T, et al. Autoantibodies against appetite-regulating peptide hormones and neuropeptides: Putative modulation by gut microflora. Nutrition (2008) 24:348–59. doi: 10.1016/j.nut.2007.12.006
26. Terashi M, Asakawa A, Harada T, Ushikai M, Coquerel Q, Sinno MH, et al. Ghrelin reactive autoantibodies in restrictive anorexia nervosa. Nutrition (2011) 27:407–13. doi: 10.1016/j.nut.2011.01.002
27. Takagi K, Legrand R, Asakawa A, Amitani H, François M, Tennoune N, et al. Anti-ghrelin immunoglobulins modulate ghrelin stability and its orexigenic effect in obese mice and humans. Nat Commun (2013) 4:2685. doi: 10.1038/ncomms3685
28. Garcia FD, Coquerel Q, do Rego JC, Cravezic A, Bole-Feysot C, Kiive E, et al. Anti-neuropeptide Y plasma immunoglobulins in relation to mood and appetite in depressive disorder. Psychoneuroendocrinology (2012) 37(9):1457–67. doi: 10.1016/j.psyneuen.2012.01.015
29. Breton J, Jacquemot J, Yaker L, Leclerc C, Connil N, Feuilloley M, et al. Host Starvation and Female Sex Influence Enterobacterial ClpB Production: A Possible Link to the Etiology of Eating Disorders. Microorganisms (2020) 8(4):530. doi: 10.3390/microorganisms8040530
30. Bouhajja H, Bougacha-Elleuch N, Lucas N, Legrand R, Marrakchi R, Kaveri SV, et al. Affinity kinetics of leptin-reactive immunoglobulins are associated with plasma leptin and markers of obesity and diabetes. Nutr Diabetes (2018) 8(1):32. doi: 10.1038/s41387-018-0044-y
31. Hu X, Chen F. Exogenous insulin antibody syndrome (EIAS): a clinical syndrome associated with insulin antibodies induced by exogenous insulin in diabetic patients. Endocr Connect (2018) 7(1):R47–R55. doi: 10.1530/EC-17-0309
32. Corcos M, Atger F, Lévy-Soussan P, Avrameas S, Guilbert B, Cayol V, et al. Bulimia nervosa and autoimmunity. Psychiatry Res (1999) 87:77–82. doi: 10.1016/S0165-1781(99)00048-7
33. Fetissov SO. Neuropeptide autoantibodies assay. Methods Mol Biol (2011) 789:295–302. doi: 10.1007/978-1-61779-310-3_19
34. François M, Takagi K, Legrand R, Lucas N, Beutheu S, Bôle-Feysot C, et al. Increased Ghrelin but Low Ghrelin-Reactive Immunoglobulins in a Rat Model of Methotrexate Chemotherapy-Induced Anorexia. Front Nutr (2016) 3:23. doi: 10.3389/fnut.2016.00023
35. Wheatland R. Chronic ACTH autoantibodies are a significant pathological factor in the disruption of the hypothalamic-pituitary-adrenal axis in chronic fatigue syndrome, anorexia nervosa and major depression. Med Hypotheses (2005) 65(2):287–95. doi: 10.1016/j.mehy.2005.02.031
36. Smitka K, Papezova H, Vondra K, Hill M, Hainer V, Nedvidkova J. A higher response of plasma neuropeptide Y, growth hormone, leptin levels and extracellular glycerol levels in subcutaneous abdominal adipose tissue to Acipimox during exercise in patients with bulimia nervosa: single-blind, randomized, microdialysis study. Nutr Metab (2011) 8:81. doi: 10.1186/1743-7075-8-81
37. Holden RJ, Pakula IS. Tumor necrosis factor-alpha: is there a continuum of liability between stress, anxiety states and anorexia nervosa? Med Hypotheses (1999) 52(2):155–62. doi: 10.1054/mehy.1997.0641
38. Patsalos O, Dalton B, Leppanen J, Ibrahim MAA, Himmerich H. Impact of TNF-α Inhibitors on Body Weight and BMI: A Systematic Review and Meta-Analysis. Front Pharmacol (2020) 11:481. doi: 10.3389/fphar.2020.00481
39. Patsalos O, Dalton B, Himmerich H. Effects of IL-6 Signaling Pathway Inhibition on Weight and BMI: A Systematic Review and Meta-Analysis. Int J Mol Sci (2020) 21(17):6290. doi: 10.3390/ijms21176290
40. Solmi M, Santonastaso P, Caccaro R, Favaro A. A case of anorexia nervosa with comorbid Crohn’s disease: beneficial effects of anti-TNF-α therapy? Int J Eat Disord (2013) 46(6):639–41. doi: 10.1002/eat.22153
41. Barber J, Sheeran T, Mulherin D. Anti-tumour necrosis factor treatment in a patient with anorexia nervosa and juvenile idiopathic arthritis. Ann Rheum Dis (2003) 62(5):490–1. doi: 10.1136/ard.62.5.490
42. Smitka K, Nedvidkova J, Vondra K, Hill M, Papezova H, Hainer V. Acipimox Administration With Exercise Induces a Co-feedback Action of the GH, PP, and PYY on Ghrelin Associated With a Reduction of Peripheral Lipolysis in Bulimic and Healthy-Weight Czech Women: A Randomized Study. Front Endocrinol (Lausanne) (2019) 10:108. doi: 10.3389/fendo.2019.00108
43. Nedvidkova J, Dostalova I, Bartak V, Papezova H, Pacak K. Increased subcutaneous abdominal tissue norepinephrine levels in patients with anorexia nervosa: an in vivo microdialysis study. Physiol Res (2004) 53:409–13.
44. Bi S, Kim YJ, Zheng F. Dorsomedial hypothalamic NPY and energy balance control. Neuropeptides (2012) 46(6):309–14. doi: 10.1016/j.npep.2012.09.002
45. Lancaster E, Dalmau J. Neuronal autoantigens–pathogenesis, associated disorders and antibody testing. Nat Rev Neurol (2012) 8(7):380–90. doi: 10.1038/nrneurol.2012.99
46. Najjar S, Pearlman DM, Alper K, Najjar A, Devinsky O. Neuroinflammation and psychiatric illness. J Neuroinflamm (2013) 10:43. doi: 10.1186/1742-2094-10-43
47. Margutti P, Delunardo F, Ortona E. Autoantibodies associated with psychiatric disorders. Curr Neurovasc Res (2006) 2:149–57. doi: 10.2174/156720206776875894
48. Caspani G, Swann J. Small talk: microbial metabolites involved in the signaling from microbiota to brain. Curr Opin Pharmacol (2019) 48:99–106. doi: 10.1016/j.coph.2019.08.001
49. Parker A, Fonseca S, Carding SR. Gut microbes and metabolites as modulators of blood-brain barrier integrity and brain health. Gut Microbes (2020) 11(2):135–57. doi: 10.1080/19490976.2019.1638722
50. Fasano A. All disease begins in the (leaky) gut: role of zonulin-mediated gut permeability in the pathogenesis of some chronic inflammatory diseases. F1000Res (2020) 9:F1000 Faculty Rev–69. doi: 10.12688/f1000research.20510.1
51. Braniste V, Al-Asmakh M, Kowal C, Anuar F, Abbaspour A, Tóth M, et al. The gut microbiota influences blood-brain barrier permeability in mice. Sci Transl Med (2014) 6(263):263ra158. doi: 10.1126/scitranslmed.3009759
52. Frost G, Sleeth ML, Sahuri-Arisoylu M, Lizarbe B, Cerdan S, Brody L, et al. The short-chain fatty acid acetate reduces appetite via a central homeostatic mechanism. Nat Commun (2014) 5:3611. doi: 10.1038/ncomms4611
53. Chambers ES, Morrison DJ, Frost G. Control of appetite and energy intake by SCFA: what are the potential underlying mechanisms? Proc Nutr Soc (2015) 74(3):328–36. doi: 10.1017/S0029665114001657
54. Monteleone P, Carratu R, Carteni M, Generoso M, Lamberti M, De Magistris L, et al. Intestinal permeability is decreased in anorexia nervosa. Mol Psychiatry (2004) 9:76–80. doi: 10.1038/sj.mp.4001374
55. Naisberg Y, Modai I, Weizman A. Metabolic bioenergy homeostatic disruption: a cause of anorexia nervosa. Med Hypotheses (2001) 56:454–61. doi: 10.1054/mehy.2000.1199
56. Morris AA. Cerebral ketone body metabolism. J Inherit Metab Dis (2005) 28(2):109–21. doi: 10.1007/s10545-005-5518-0
57. Puxley F, Midtsund M, Iosif A, Lask B. PANDAS anorexia nervosa–endangered, extinct or nonexistent? Int J Eat Disord (2008) 41:15–21. doi: 10.1002/eat.20462
58. Vincenzi B, O’Toole J, Lask B. PANDAS and anorexia nervosa–a spotters’ guide: suggestions for medical assessment. Eur Eat Disord Rev (2010) 8(2):116–23. doi: 10.1002/erv.977
59. Kılıç F, Işık Ü, Demirdaş A, Doğuç DK, Bozkurt M. Serum zonulin and claudin-5 levels in patients with bipolar disorder. J Affect Disord (2020) 266:37–42. doi: 10.1016/j.jad.2020.01.117
60. Fu A, Hui EK, Lu JZ, Boado RJ, Pardridge WM. Neuroprotection in stroke in the mouse with intravenous erythropoietin-Trojan horse fusion protein. Brain Res (2011) 1369:203–7. doi: 10.1016/j.brainres.2010.10.097
61. Fetissov SO, Lucas N, Legrand R. Ghrelin-Reactive Immunoglobulins in Conditions of Altered Appetite and Energy Balance. Front Endocrinol (Lausanne) (2017) 8:10. doi: 10.3389/fendo.2017.00010
62. Jésus P, Ouelaa W, François M, Riachy L, Guérin C, Aziz M, et al. Alteration of intestinal barrier function during activity-based anorexia in mice. Clin Nutr (2014) 33(6):1046–53. doi: 10.1016/j.clnu.2013.11.006
63. Sinno MH, Coquerel Q, Boukhettala N, Coëffier M, Gallas S, Terashi M, et al. Chemotherapy-induced anorexia is accompanied by activation of brain pathways signaling dehydration. Physiol Behav (2010) 101(5):639–48. doi: 10.1016/j.physbeh.2010.09.016
64. Coquerel Q, Sinno MH, Boukhettala N, Coëffier M, Terashi M, Bole-Feysot C, et al. Intestinal inflammation influences α-MSH reactive autoantibodies: relevance to food intake and body weight. Psychoneuroendocrinology (2012) 37(1):94–106. doi: 10.1016/j.psyneuen.2011.05.008
65. Wiercinska-Drapalo A, Jaroszewicz J, Siwak E, Pogorzelska J, Prokopowicz D. Intestinal fatty acid binding protein (I-FABP) as a possible biomarker of ileitis in patients with ulcerative colitis. Regul Pept (2008) 147(1-3):25–8. doi: 10.1016/j.regpep.2007.12.002
66. Henderson AL, Brand MW, Darling RJ, Maas KJ, Detzel CJ, Hostetter J, et al. Attenuation of Colitis by Serum-Derived Bovine Immunoglobulin/Protein Isolate in a Defined Microbiota Mouse Model. Dig Dis Sci (2015) 60(11):3293–303. doi: 10.1007/s10620-015-3726-5
67. Bass N. The cellular fatty acid binding proteins: aspects of structure, regulation, and function. Int Rev Cytol (1988) 111:143–84. doi: 10.1016/S0074-7696(08)61733-7
68. Prochazkova P, Roubalova R, Dvorak J, Tlaskalova-Hogenova H, Cermakova M, Tomasova P, et al. Microbiota, Microbial Metabolites, and Barrier Function in A Patient with Anorexia Nervosa after Fecal Microbiota Transplantation. Microorganisms (2019) 7(9):338. doi: 10.3390/microorganisms7090338
69. Monteiro MP, Batterham RL. The Importance of the Gastrointestinal Tract in Controlling Food Intake and Regulating Energy Balance. Gastroenterology (2017) 152:1707–17.e2. doi: 10.1053/j.gastro.2017.01.053
70. Sakata N, Yoshimatsu G, Kodama S. Development and Characteristics of Pancreatic Epsilon Cells. Int J Mol Sci (2019) 20(8):1867. doi: 10.3390/ijms20081867
71. Smitka K, Papezova H, Vondra K, Hill M, Hainer V, Nedvidkova J. The role of “mixed” orexigenic and anorexigenic signals and autoantibodies reacting with appetite-regulating neuropeptides and peptides of the adipose tissue-gut-brain axis: relevance to food intake and nutritional status in patients with anorexia nervosa and bulimia nervosa. Int J Endocrinol (2013) 2013:483145. doi: 10.1155/2013/483145
72. Mani BK, Zigman JM. Ghrelin as a Survival Hormone. Trends Endocrinol Metab (2017) 8(12):843–54. doi: 10.1016/j.tem.2017.10.001
73. Müller TD, Nogueiras R, Andermann ML, Andrews ZB, Anker SD, Argente J, et al. Ghrelin. Mol Metab (2015) 4:437–60. doi: 10.1016/j.molmet.2015.03.005
74. Asakawa A, Inui A, Fujimiya M, Sakamaki R, Shinfuku N, Ueta Y, et al. Stomach regulates energy balance via acylated ghrelin and desacyl ghrelin. Gut (2005) 54(1):18–24. doi: 10.1136/gut.2004.038737
75. Cummings DE, Purnell JQ, Frayo RS, Schmidova K, Wisse BE, Weigle DS. A preprandial rise in plasma ghrelin levels suggests a role in meal initiation in humans. Diabetes (2001) 50(8):1714–9. doi: 10.2337/diabetes.50.8.1714
76. Hassouna R, Labarthe A, Tolle V. Hypothalamic regulation of body growth and appetite by ghrelin-derived peptides during balanced nutrition or undernutrition. Mol Cell Endocrinol (2016) 438:42–51. doi: 10.1016/j.mce.2016.09.027
77. Koyama KI, Yasuhara D, Nakahara T, Harada T, Uehara M, Ushikai M, et al. Changes in acyl ghrelin, des-acyl ghrelin, and ratio of acyl ghrelin to total ghrelin with short-term refeeding in female inpatients with restricting-type anorexia nervosa. Horm Metab Res (2010) 42(8):595–8. doi: 10.1055/s-0030-1252017
78. Igudesman D, Sweeney M, Carroll IM, Mayer-Davis EJ, Bulik CM. Gut-Brain Interactions: Implications for a Role of the Gut Microbiota in the Treatment and Prognosis of Anorexia Nervosa and Comparison to Type I Diabetes. Gastroenterol Clin North Am (2019) 48:343–56. doi: 10.1016/j.gtc.2019.04.003
79. Hotta M, Ohwada R, Katakami H, Shibasaki T, Hizuka N, Takano K. Plasma levels of intact and degraded ghrelin and their responses to glucose infusion in anorexia nervosa. J Clin Endocrinol Metab (2004) 89(11):5707–12. doi: 10.1210/jc.2004-0353
80. Fazeli PK, Lawson EA, Faje AT, Eddy KT, Lee H, Fiedorek FT, et al. Treatment With a Ghrelin Agonist in Outpatient Women With Anorexia Nervosa: A Randomized Clinical Trial. J Clin Psychiatry (2018) 79(1):17m11585. doi: 10.4088/JCP.17m11585
81. Gorwood P, Blanchet-Collet C, Chartrel N, Duclos J, Dechelotte P, Hanachi M, et al. New Insights in Anorexia Nervosa. Front Neurosci (2016) 10:256. doi: 10.3389/fnins.2016.00256
82. Dostalova I, Smitka K, Papezova H, Kvasnickova H, Nedvidkova J. Increased insulin sensitivity in patients with anorexia nervosa: the role of adipocytokines. Physiol Res (2007) 56:587–94.
83. Prioletta A, Muscogiuri G, Sorice GP, Lassandro AP, Mezza T, Policola C, et al. In anorexia nervosa, even a small increase in abdominal fat is responsible for the appearance of insulin resistance. Clin Endocrinol (2011) 75:202–6. doi: 10.1111/j.1365-2265.2011.04046.x
84. Yahya AS, Khawaja S, Chukwuma J, Chukwuma C. Early Diagnosis and Management of Bulimia Nervosa in Type 1 Diabetes. Prim Care Companion CNS Disord (2020) 22(6):20nr02707. doi: 10.4088/PCC.20nr02707
85. Martínez-Ansó E, Pérez M, Martínez JA. Induction of hypothermia, hypoglycemia and hyperinsulinemia after acute leptin immunoneutralization in overnight fasted mice. Int J Mol Med (1998) 2(6):681–3. doi: 10.3892/ijmm.2.6.681
86. Zhang JV, Ren PG, Avsian-Kretchmer O, Luo CW, Rauch R, Klein C, et al. Obestatin, a peptide encoded by the ghrelin gene, opposes ghrelin’s effects on food intake. Science (2005) 310(5750):996–9. doi: 10.1126/science.1117255
87. Garg A. The ongoing saga of obestatin: is it a hormone? J Clin Endocrinol Metab (2007) 92(9):3396–8. doi: 10.1210/jc.2007-0999
88. Monteleone P, Serritella C, Martiadis V, Scognamiglio P, Maj M. Plasma obestatin, ghrelin, and ghrelin/obestatin ratio are increased in underweight patients with anorexia nervosa but not in symptomatic patients with bulimia nervosa. J Clin Endocrinol Metab (2008) 93(11):4418–21. doi: 10.1210/jc.2008-1138
89. Matafome P, Eickhoff H, Letra L, Seiça R. Neuroendocrinology of Adipose Tissue and Gut-Brain Axis. Adv Neurobiol (2017) 19:49–70. doi: 10.1007/978-3-319-63260-5_3
90. Manning S, Batterham RL. The role of gut hormone peptide YY in energy and glucose homeostasis: twelve years on. Annu Rev Physiol (2014) 76:585–608. doi: 10.1146/annurev-physiol-021113-170404
91. Rudenko O, Shang J, Munk A, Ekberg JP, Petersen N, Engelstoft MS, et al. The aromatic amino acid sensor GPR142 controls metabolism through balanced regulation of pancreatic and gut hormones. Mol Metab (2019) 19:49–64. doi: 10.1016/j.molmet.2018.10.012
92. Engelstoft MS, Schwartz TW. Opposite Regulation of Ghrelin and Glucagon-like Peptide-1 by Metabolite G-Protein-Coupled Receptors. Trends Endocrinol Metab (2016) 27(9):665–75. doi: 10.1016/j.tem.2016.07.001
93. Engelstoft MS, Park WM, Sakata I, Kristensen LV, Husted AS, Osborne-Lawrence S, et al. Seven transmembrane G protein coupled receptor repertoire of gastric ghrelin cells. Mol Metab (2013) 2:376–92. doi: 10.1016/j.molmet.2013.08.006
94. Lund ML, Egerod KL, Engelstoft MS, Dmytriyeva O, Theodorsson E, Patel BA, et al. Enterochromaffin 5-HT cells - A major target for GLP-1 and gut microbial metabolites. Mol Metab (2018) 11:70–83. doi: 10.1016/j.molmet.2018.03.004
95. Zaibi MS, Stocker CJ, O’Dowd J, Davies A, Bellahcene M, Cawthorne MA, et al. Roles of GPR41 and GPR43 in leptin secretory responses of murine adipocytes to short chain fatty acids. FEBS Lett (2010) 584(11):2381–6. doi: 10.1016/j.febslet.2010.04.027
96. Al-Lahham SH, Roelofsen H, Priebe M, Weening D, Dijkstra M, Hoek A, et al. Regulation of adipokine production in human adipose tissue by propionic acid. Eur J Clin Invest (2010) 40(5):401–7. doi: 10.1111/j.1365-2362.2010.02278.x
97. Hoyles L, Snelling T, Umlai UK, Nicholson JK, Carding SR, Glen RC, et al. Microbiome-host systems interactions: protective effects of propionate upon the blood-brain barrier. Microbiome (2018) 6(1):55. doi: 10.1186/s40168-018-0439-y
98. Friedrichs P, Saremi B, Winand S, Rehage J, Dänicke S, Sauerwein H, et al. Energy and metabolic sensing G protein-coupled receptors during lactation-induced changes in energy balance. Domest Anim Endocrinol (2014) 48:33–41. doi: 10.1016/j.domaniend.2014.01.005
99. Macia L, Tan J, Vieira AT, Leach K, Stanley D, Luong S, et al. Metabolite-sensing receptors GPR43 and GPR109A facilitate dietary fibre-induced gut homeostasis through regulation of the inflammasome. Nat Commun (2015) 6:6734. doi: 10.1038/ncomms7734
100. Schorr M, Miller KK. The endocrine manifestations of anorexia nervosa: mechanisms and management. Nat Rev Endocrinol (2017) 13(3):174–86. doi: 10.1038/nrendo.2016.175
101. Lawson EA, Eddy KT, Donoho D, Misra M, Miller KK, Meenaghan E, et al. Appetite-regulating hormones cortisol and peptide YY are associated with disordered eating psychopathology, independent of body mass index. Eur J Endocrinol (2011) 164(2):253–61. doi: 10.1530/EJE-10-0523
102. Nakahara T, Kojima S, Tanaka M, Yasuhara D, Harada T, Sagiyama K, et al. Incomplete restoration of the secretion of ghrelin and PYY compared to insulin after food ingestion following weight gain in anorexia nervosa. J Psychiatr Res (2007) 41(10):814–20. doi: 10.1016/j.jpsychires.2006.07.021
103. Otto B, Cuntz U, Fruehauf E, Wawarta R, Folwaczny C, Riepl RL, et al. Weight gain decreases elevated plasma ghrelin concentrations of patients with anorexia nervosa. Eur J Endocrinol (2001) 145(5):669–73. doi: 10.1530/EJE-1450669
104. Tong J, D’Alessio D. Eating disorders and gastrointestinal peptides. Curr Opin Endocrinol Diabetes Obes (2011) 18(1):42–9. doi: 10.1097/MED.0b013e328341e12b
105. Kojima S, Nakahara T, Nagai N, Muranaga T, Tanaka M, Yasuhara D, et al. Altered ghrelin and peptide YY responses to meals in bulimia nervosa. Clin Endocrinol (Oxf) (2005) 62(1):74–8. doi: 10.1111/j.1365-2265.2004.02176.x
106. Monteleone P, Martiadis V, Rigamonti AE, Fabrazzo M, Giordani C, Muller EE, et al. Investigation of peptide YY and ghrelin responses to a test meal in bulimia nervosa. Biol Psychiatry (2005) 57(8):926–31. doi: 10.1016/j.biopsych.2005.01.004
107. Feltrin KL, Little TJ, Meyer JH, Horowitz M, Rades T, Wishart J, et al. Comparative effects of intraduodenal infusions of lauric and oleic acids on antropyloroduodenal motility, plasma cholecystokinin and peptide YY, appetite, and energy intake in healthy men. Am J Clin Nutr (2008) 87(5):1181–7. doi: 10.1093/ajcn/87.5.1181
108. Hannon-Engel SL, Filin EE, Wolfe BE. CCK response in bulimia nervosa and following remission. Physiol Behav (2013) 122:56–61. doi: 10.1016/j.physbeh.2013.08.014
109. Cone RD. Anatomy and regulation of the central melanocortin system. Nat Neurosci (2005) 8:571–8. doi: 10.1038/nn1455
110. Sinno MH, Do Rego JC, Coëffier M, Bole-Feysot C, Ducrotté P, Gilbert D, et al. Regulation of feeding and anxiety by alpha-MSH reactive autoantibodies. Psychoneuroendocrinology (2009) 34(1):140–9. doi: 10.1016/j.psyneuen.2008.08.021
111. Schaefer JM, Fetissov SO, Legrand R, Claeyssens S, Hoekstra PJ, Verhulst FC, et al. Corticotropin (ACTH)-reactive immunoglobulins in adolescents in relation to antisocial behavior and stress-induced cortisol response. The TRAILS study. Psychoneuroendocrinology (2013) 38(12):3039–47. doi: 10.1016/j.psyneuen.2013.08.015
112. Galmiche M, Lucas N, Déchelotte P, Deroissart C, Solliec ML, Rondeaux J, et al. Plasma Peptide Concentrations and Peptide-Reactive Immunoglobulins in Patients with Eating Disorders at Inclusion in the French EDILS Cohort (Eating Disorders Inventory and Longitudinal Survey). Nutrients (2020) 12(2):522. doi: 10.3390/nu12020522
113. Dominique M, Legrand R, Galmiche M, Azhar S, Deroissart C, Guérin C, et al. Changes in Microbiota and Bacterial Protein Caseinolytic Peptidase B During Food Restriction in Mice: Relevance for the Onset and Perpetuation of Anorexia Nervosa. Nutrients (2019) 11(10):2514. doi: 10.3390/nu11102514
114. Panaro BL, Tough IR, Engelstoft MS, Matthews RT, Digby GJ, Møller CL, et al. The melanocortin-4 receptor is expressed in enteroendocrine L cells and regulates the release of peptide YY and glucagon-like peptide 1in vivo. Cell Metab (2014) 20(6):1018–29. doi: 10.1016/j.cmet.2014.10.004
115. Galusca B, Prévost G, Germain N, Dubuc I, Ling Y, Anouar Y, et al. Neuropeptide Y and α-MSH circadian levels in two populations with low body weight: anorexia nervosa and constitutional thinness. PloS One (2015) 10(3):e0122040. doi: 10.1371/journal.pone.0122040
116. Budzyński J, Kłopocka M. Brain-gut axis in the pathogenesis of Helicobacter pylori infection. World J Gastroenterol (2014) 20:5212–25. doi: 10.3748/wjg.v20.i18.5212
117. Yanagi H, Tsuda A, Matsushima M, Takahashi S, Ozawa G, Koga Y, et al. Changes in the gut microbiota composition and the plasma ghrelin level in patients with Helicobacter pylori-infected patients with eradication therapy. BMJ Open Gastroenterol (2017) 4(1):e000182. doi: 10.1136/bmjgast-2017-000182
118. Bailey MT, Dowd SE, Galley JD, Hufnagle AR, Allen RG, Lyte M. Exposure to a social stressor alters the structure of the intestinal microbiota: implications for stressor-induced immunomodulation. Brain Behav Immun (2011) 25(3):397–407. doi: 10.1016/j.bbi.2010.10.023
119. Queipo-Ortuño MI, Seoane LM, Murri M, Pardo M, Gomez-Zumaquero JM, Cardona F, et al. Gut microbiota composition in male rat models under different nutritional status and physical activity and its association with serum leptin and ghrelin levels. PloS One (2013) 8(5):e65465. doi: 10.1371/journal.pone.0065465
120. Breton J, Tirelle P, Hasanat S, Pernot A, L’Huillier C, do Rego JC, et al. Gut microbiota alteration in a mouse model of Anorexia Nervosa. Clin Nutr (2021) 40(1):181–9. doi: 10.1016/j.clnu.2020.05.002
121. Schalla MA, Stengel A. Effects of microbiome changes on endocrine ghrelin signaling - A systematic review. Peptides (2020) 133:170388. doi: 10.1016/j.peptides.2020.170388
122. Dinan TG, Cryan JF. Microbes, Immunity and Behaviour: Psychoneuroimmunology Meets the Microbiome. Neuropsychopharmacology (2016) 42(1):178–92. doi: 10.1038/npp.2016.103
123. Collins SM, Surette M, Bercik P. The interplay between the intestinal microbiota and the brain. Nat Rev Microbiol (2012) 10(11):735–42. doi: 10.1038/nrmicro2876
124. Tlaskalová-Hogenová H, Stěpánková R, Kozáková H, Hudcovic T, Vannucci L, Tučková L, et al. The role of gut microbiota (commensal bacteria) and the mucosal barrier in the pathogenesis of inflammatory and autoimmune diseases and cancer: contribution of germ-free and gnotobiotic animal models of human diseases. Cell Mol Immunol (2011) 8(2):110–20. doi: 10.1038/cmi.2010.67
125. Kostovcikova K, Coufal S, Galanova N, Fajstova A, Hudcovic T, Kostovcik M, et al. Diet Rich in Animal Protein Promotes Pro-inflammatory Macrophage Response and Exacerbates Colitis in Mice. Front Immunol (2019) 10:919. doi: 10.3389/fimmu.2019.00919
126. Prochazkova P, Roubalova R, Dvorak J, Kreisinger J, Hill M, Tlaskalova-Hogenova H, et al. The intestinal microbiota and metabolites in patients with anorexia nervosa. Gut Microbes (2021) 13(1):1–25. doi: 10.1080/19490976.2021.1902771
127. Kleiman SC, Carroll IM, Tarantino LM, Bulik CM. Gut feelings: A role for the intestinal microbiota in anorexia nervosa? Int J Eat Disord (2015) 48(5):449–51. doi: 10.1002/eat.22394
128. Breton J, Dechelotte P, Ribet D. Intestinal microbiota and anorexia nervosa. Clin Nutr Exp (2019) 28:11–21. doi: 10.1016/j.yclnex.2019.05.001
129. Levy M, Kolodziejczyk AA, Thaiss CA, Elinav E. Dysbiosis and the immune system. Nat Rev Immunol (2017) 17(4):219–32. doi: 10.1038/nri.2017.7
130. Armougom F, Henry M, Vialettes B, Raccah D, Raoult D. Monitoring bacterial community of human gut microbiota reveals an increase in Lactobacillus in obese patients and Methanogens in anorexic patients. PloS One (2009) 4(9):e7125. doi: 10.1371/journal.pone.0007125
131. Million M, Angelakis E, Maraninchi M, Henry M, Giorgi R, Valero R, et al. Correlation between body mass index and gut concentrations of Lactobacillus reuteri, Bifidobacterium animalis, Methanobrevibacter smithii and Escherichia coli. Int J Obes (Lond) (2013) 37(11):1460–6. doi: 10.1038/ijo.2013.20
132. Morita C, Tsuji H, Hata T, Gondo M, Takakura S, Kawai K, et al. Gut Dysbiosis in Patients with Anorexia Nervosa. PloS One (2015) 10(12):e0145274. doi: 10.1371/journal.pone.0145274
133. Kleiman SC, Watson HJ, Bulik-Sullivan EC, Huh EY, Tarantino LM, Bulik CM, et al. The Intestinal Microbiota in Acute Anorexia Nervosa and During Renourishment: Relationship to Depression, Anxiety, and Eating Disorder Psychopathology. Psychosom Med (2015) 77(9):969–81. doi: 10.1097/PSY.0000000000000247
134. Mack I, Cuntz U, Gramer C, Niedermaier S, Pohl C, Schwiertz A, et al. Weight gain in anorexia nervosa does not ameliorate the faecal microbiota, branched chain fatty acid profiles, and gastrointestinal complaints. Sci Rep (2016) 6:1–16. doi: 10.1038/srep26752
135. Mörkl S, Lackner S, Müller W, Gorkiewicz G, Kashofer K, Oberascher A, et al. Gut microbiota and body composition in anorexia nervosa inpatients in comparison to athletes, overweight, obese, and normal weight controls. Int J Eat Disord (2017) 50(12):1421–31. doi: 10.1002/eat.22801
136. Borgo F, Riva A, Benetti A, Casiraghi MC, Bertelli S, Garbossa S, et al. Microbiota in anorexia nervosa: The triangle between bacterial species, metabolites and psychological tests. PloS One (2017) 12(6):e0179739. doi: 10.1371/journal.pone.0179739
137. Hanachi M, Manichanh C, Schoenenberger A, Pascal V, Levenez F, Cournède N, et al. Altered host-gut microbes symbiosis in severely malnourished anorexia nervosa (AN) patients undergoing enteral nutrition: An explicative factor of functional intestinal disorders? Clin Nutr (2019) 38(5):2304–10. doi: 10.1016/j.clnu.2018.10.004
138. Monteleone AM, Troisi J, Fasano A, Dalle Grave R, Marciello F, Serena G, et al. Multi-omics data integration in anorexia nervosa patients before and after weight regain: A microbiome-metabolomics investigation. Clin Nutr (2021) 40(3):1137–46. doi: 10.1016/j.clnu.2020.07.021
139. Di Lodovico L, Mondot S, Doré J, Mack I, Hanachi M, Gorwood P. Anorexia nervosa and gut microbiota: A systematic review and quantitative synthesis of pooled microbiological data. Prog Neuropsychopharmacol Biol Psychiatry (2021) 22:110114. doi: 10.1016/j.pnpbp.2020.110114
140. Mörkl S, Lackner S, Meinitzer A, Gorkiewicz G, Kashofer K, Painold A, et al. [Pilot study: Gut microbiome and intestinal barrier in anorexia nervosa]. Fortschr Neurol Psychiatr (2019) 87:39–45. doi: 10.1055/s-0043-123826
141. Goodrich JK, Waters JL, Poole AC, Sutter JL, Koren O, Blekhman R, et al. Human genetics shape the gut microbiome. Cell (2014) 159(4):789–99. doi: 10.1016/j.cell.2014.09.053
142. Ruaud A, Esquivel-Elizondo S, de la Cuesta-Zuluaga J, Waters JL, Angenent LT, Youngblut ND, et al. Syntrophy via Interspecies H2 Transfer between Christensenella and Methanobrevibacter Underlies Their Global Cooccurrence in the Human Gut. mBio (2020) 11(1):e03235–19. doi: 10.1128/mBio.03235-19
143. Vrieze A, Van Nood E, Holleman F, Salojärvi J, Kootte RS, Bartelsman JF, et al. Transfer of intestinal microbiota from lean donors increases insulin sensitivity in individuals with metabolic syndrome. Gastroenterology (2012) 143(4):913–6.e7. doi: 10.1053/j.gastro.2012.06.031
144. Ley RE, Turnbaugh PJ, Klein S, Gordon JI. Microbial ecology: human gut microbes associated with obesity. Nature (2006) 444(7122):1022–3. doi: 10.1038/4441022a
145. Turnbaugh PJ, Ley RE, Mahowald MA, Magrini V, Mardis ER, Gordon JI. An obesity-associated gut microbiome with increased capacity for energy harvest. Nature (2006) 444(7122):1027–31. doi: 10.1038/nature05414
146. Bravo JA, Forsythe P, Chew MV, Escaravage E, Savignac HM, Dinan TG, et al. Ingestion of Lactobacillus strain regulates emotional behaviour and central GABA receptor expression in a mouse via the vagus nerve. Proc Natl Acad Sci USA (2011) 108:16050–55. doi: 10.1073/pnas.1102999108
147. Desbonnet L, Garrett L, Clarke G, Bienenstock J, Dinan TG. The probiotic Bifidobacteria infantis: An assessment of potential antidepressant properties in the rat. J Psychiatr Res (2008) 43:164. doi: 10.1016/j.jpsychires.2008.03.009
148. Valles-Colomer M, Falony G, Darzi Y, Tigchelaar EF, Wang J, Tito RY, et al. The neuroactive potential of the human gut microbiota in quality of life and depression. Nat Microbiol (2019) 4):623–32. doi: 10.1038/s41564-018-0337-x
149. Liu WH, Chuang HL, Huang YT, Wu CC, Chou GT, Wang S, et al. Alteration of behavior and monoamine levels attributable to Lactobacillus plantarum PS128 in germ-free mice. Behav Brain Res (2016) 298(Pt B):202–9. doi: 10.1016/j.bbr.2015.10.046
150. Shishov VA, Kirovskaia TA, Kudrin VS, Oleskin AV. Amine neuromediators, their precursors, and oxidation products in the culture of Escherichia coli K-12. Appl Biochem Mikrobiol (2009) 45:494–7. doi: 10.1134/S0003683809050068
151. Asano Y, Hiramoto T, Nishino R, Aiba Y, Kimura T, Yoshihara K, et al. Critical role of gut microbiota in the production of biologically active, free catecholamines in the gut lumen of mice. Am J Physiol Gastrointest Liver Physiol (2012) 303(11):G1288–95. doi: 10.1152/ajpgi.00341.2012
152. Tsavkelova EA, Botvinko IV, Kudrin VS, Oleskin AV. Detection of neurotransmitter amines in microorganisms with the use of high-performance liquid chromatography. Dokl Biochem (2000) 372(1-6):115–7.
153. Stanaszek PM, Snell JF, O’Neill JJ. Isolation, extraction, and measurement of acetylcholine from Lactobacillus plantarum. Appl Environ Microbiol (1977) 34(2):237–9. doi: 10.1128/AEM.34.2.237-239.1977
154. Strandwitz P, Kim KH, Terekhova D, Liu JK, Sharma A, Levering J, et al. GABA-modulating bacteria of the human gut microbiota. Nat Microbiol (2019) 4(3):396–403. doi: 10.1038/s41564-018-0307-3
155. Kunze WA, Mao YK, Wang B, Huizinga JD, Ma X, Forsythe P, et al. Lactobacillus reuteri enhances excitability of colonic AH neurons by inhibiting calcium-dependent potassium channel opening. J Cell Mol Med (2009) 13(8B):2261–70. doi: 10.1111/j.1582-4934.2009.00686.x
156. De Vadder F, Grasset E, Mannerås Holm L, Karsenty G, Macpherson AJ, Olofsson LE, et al. Gut microbiota regulates maturation of the adult enteric nervous system via enteric serotonin networks. Proc Natl Acad Sci U S A (2018) 115(25):6458–63. doi: 10.1073/pnas.1720017115
157. Barrett E, Ross RP, O’Toole PW, Fitzgerald GF, Stanton C. gamma-Aminobutyric acid production by culturable bacteria from the human intestine. Appl Microbiol (2012) 113(2):411–7. doi: 10.1111/j.1365-2672.2012.05344.x
158. Siragusa S, De Angelis M, Di Cagno R, Rizzello CG, Coda R, Gobbetti M. Synthesis of gamma-aminobutyric acid by lactic acid bacteria isolated from a variety of Italian cheeses. Appl Environ Microbiol (2007) 73(22):7283–90. doi: 10.1128/AEM.01064-07
159. Hata T, Miyata N, Takakura S, Yoshihara K, Asano Y, Kimura-Todani T, et al. The Gut Microbiome Derived From Anorexia Nervosa Patients Impairs Weight Gain and Behavioral Performance in Female Mice. Endocrinology (2019) 160(10):2441–52. doi: 10.1210/en.2019-00408
160. Monteleone P, Monteleone AM, Troisi J, Dalle Grave R, Corrivetti G, Calugi S, et al. Metabolomics signatures of acutely ill and short-term weight recovered women with anorexia nervosa. Mol Psychiatry (2019). doi: 10.1038/s41380-019-0573-3
161. Cammarota G, Masucci L, Ianiro G, Bibbò S, Dinoi G, Costamagna G, et al. Randomised clinical trial: faecal microbiota transplantation by colonoscopy vs. vancomycin for the treatment of recurrent Clostridium difficile infection. Aliment Pharmacol Ther (2015) 41(9):835–43. doi: 10.1111/apt.13144
162. Zenzeri L, Tambucci R, Quitadamo P, Giorgio V, De Giorgio R, Di Nardo G. Update on chronic intestinal pseudo-obstruction. Curr Opin Gastroenterol (2020) 36(3):230–7. doi: 10.1097/MOG.0000000000000630
163. Quigley EMM. The Spectrum of Small Intestinal Bacterial Overgrowth (SIBO). Curr Gastroenterol Rep (2019) 21(1):3. doi: 10.1007/s11894-019-0671-z
164. Santonicola A, Gagliardi M, Guarino MPL, Siniscalchi M, Ciacci C, Iovino P. Eating Disorders and Gastrointestinal Diseases. Nutrients (2019) 11(12):3038. doi: 10.3390/nu11123038
Keywords: anorexia nervosa and bulimia, ghrelin, alpha-MSH, caseinolytic peptidase B, gut and blood-brain barrier permeability, fecal microbial transplantation, microbiome, autoantibody
Citation: Smitka K, Prochazkova P, Roubalova R, Dvorak J, Papezova H, Hill M, Pokorny J, Kittnar O, Bilej M and Tlaskalova-Hogenova H (2021) Current Aspects of the Role of Autoantibodies Directed Against Appetite-Regulating Hormones and the Gut Microbiome in Eating Disorders. Front. Endocrinol. 12:613983. doi: 10.3389/fendo.2021.613983
Received: 04 October 2020; Accepted: 09 March 2021;
Published: 19 April 2021.
Edited by:
Ana Rosa Pérez, Consejo Nacional de Investigaciones Científicas y Técnicas (CONICET), ArgentinaReviewed by:
Sergueï O. Fetissov, Université de Rouen, FranceLudmila Prokesova, Charles University, Czechia
Copyright © 2021 Smitka, Prochazkova, Roubalova, Dvorak, Papezova, Hill, Pokorny, Kittnar, Bilej and Tlaskalova-Hogenova. This is an open-access article distributed under the terms of the Creative Commons Attribution License (CC BY). The use, distribution or reproduction in other forums is permitted, provided the original author(s) and the copyright owner(s) are credited and that the original publication in this journal is cited, in accordance with accepted academic practice. No use, distribution or reproduction is permitted which does not comply with these terms.
*Correspondence: Kvido Smitka, a3ZpZG8uc21pdGthQGYxLmN1bmkuY3o=