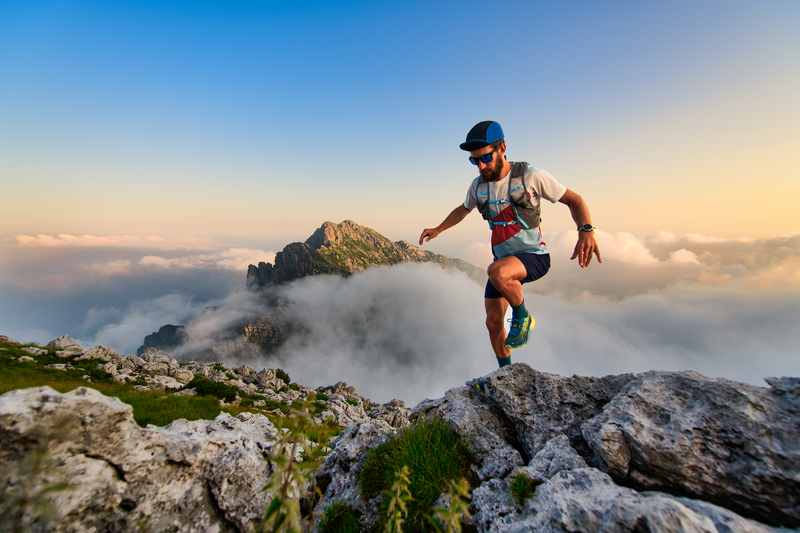
95% of researchers rate our articles as excellent or good
Learn more about the work of our research integrity team to safeguard the quality of each article we publish.
Find out more
REVIEW article
Front. Endocrinol. , 09 February 2021
Sec. Diabetes: Molecular Mechanisms
Volume 11 - 2020 | https://doi.org/10.3389/fendo.2020.627076
This article is part of the Research Topic The Role of Sphingolipid Metabolism in the Development of Type 2 Diabetes and Obesity View all 7 articles
Sphingolipids are a class of essential lipids, functioning as both cell membrane constituents and signaling messengers. In the sphingolipid metabolic network, ceramides serve as the central hub that is hydrolyzed to sphingosine, followed by phosphorylation to sphingosine 1-phosphate (S1P) by sphingosine kinase (SphK). SphK is regarded as a “switch” of the sphingolipid rheostat, as it catalyzes the conversion of ceramide/sphingosine to S1P, which often exhibit opposing biological roles in the cell. Besides, SphK is an important signaling enzyme that has been implicated in the regulation of a wide variety of biological functions. In recent years, an increasing body of evidence has suggested a critical role of SphK in type 2 diabetes mellitus (T2D), although a certain level of controversy remains. Herein, we review recent findings related to SphK in the field of T2D research with a focus on peripheral insulin resistance and pancreatic β-cell failure. It is expected that a comprehensive understanding of the role of SphK and the associated sphingolipids in T2D will help to identify druggable targets for future anti-diabetes therapy.
Type 2 diabetes mellitus (T2D) is a progressive metabolic disease caused by impaired responses to insulin in the target tissues, such as the liver, skeletal muscle and adipose tissue, and inadequate insulin production from pancreatic islets. T2D predisposes to a significantly higher risk of severe health problems in the cardiovascular system, kidney, eyes, and nervous system, leading to reduced life quality and even death. According to the International Diabetes Federation (IDF) statistics in 2019, 1 in 11 adults aged between 20 and 79 are living with T2D, incurring a heavy socioeconomic burden (1). As the largest non-contagious pandemic of the 21st century, T2D is spreading rapidly towards less developed communities and the younger population (1). Despite intensive research for decades, the molecular mechanism underlying the pathogenesis and progression of T2D remains to be identified.
Dyslipidemia has long been recognized as a chief causative factor tying T2D with lifestyle (2). However, with the advancement of understanding in lipids, it is abundantly clear that circulating lipids only represent a small portion of pathogenic factors for T2D. Intraorganic/intracellular lipids contribute to T2D at all stages, from the early prediabetes to the establishment of complications (3, 4). Indeed, T2D is often associated with intracellular lipid dysregulation, as commonly seen in obesity, non-alcoholic fatty liver disease, and lipodystrophy (5, 6). Sphingolipids are a class of essential intracellular lipids that function as both cell membrane constituents and signaling molecules. Sphingosine kinase (SphK), including the two isoforms SphK1 and SphK2, is a key enzyme of the sphingolipid metabolic pathway (7). In recent years, an increasing body of evidence has suggested an important role of SphK in T2D, although a certain level of controversy remains. This review intends to provide a comprehensive review on SphK in the research field of T2D with a focus on peripheral insulin resistance and pancreatic β-cell failure.
Sphingolipids present one of eight major lipid categories. LIPID MAPS, the largest lipid-only database, lists 1,664 curated sphingolipid species (8). Structurally, these sphingolipid metabolites possess a common sphingoid backbone, mainly in 18-carbon length (9, 10). Metabolically, sphingolipid products are interconnected as a network with ceramide as the central hub (Figure 1). In the sphingolipid biosynthetic pathway, the first committed step is the formation of sphingoid base from amino acid and fatty acyl-CoA via condensation and reduction (9, 11). In the following steps, ceramide is produced on the basis of the sphingoid backbone via acylation and desaturation (12, 13). Ceramide can be reversibly converted into complex sphingolipids, such as sphingomyelin, glycosphingolipids and acylceramide (14–16). In the sphingolipid catabolic pathway, ceramide can be hydrolyzed into sphingosine that is subsequently phosphorylated into sphingosine 1-phosphate (S1P) by SphK (17–19). S1P can be irreversibly lysed into non-lipid products to exit the sphingolipid metabolic network (20). SphK is often regarded as a key enzyme in this network primarily for two reasons: a) SphK constitutes the checkpoint that determines the cellular content of “bioactive” sphingolipid products, including ceramide, sphingosine and S1P (18, 21); b) SphK acts as a “rheostat” of sphingolipids, because ceramide and sphingosine often exhibit cellular functions opposing S1P (22). For example, ceramide and sphingosine induce apoptosis, whereas S1P protects cells from death in various types of cells (22).
Figure 1 Sphingolipid metabolic network. SPT, serine palmitoyltransferase; KDSR, 3-keto-dihydrosphingosine reductase; CerS, ceramide synthase; DEGS, dihydroceramide desaturase; CDase, ceramidase; SphK, sphingosine kinase; SPGL1, sphingosine 1-phosphate lyase.
There are two mammalian SphK isoforms, designated as SphK1 and SphK2, which are encoded by two different genes located on distinct chromosomes. SphK1 and SphK2 are evolutionarily highly conservative (18, 19). Both of them possess five conserved regions, C1-C5 (18, 19). The C1-C3 and C5 domains in SphK share a high level of sequence homology with phosphofructokinase (PFK)-like superfamily members, such as NAD kinase and diacylglycerol kinase (23). However, the crystalized structure of SphK1 reveals that its lipid-binding pocket is distinctive from NAD kinase and diacylglycerol kinase, indicative of the substrate specificity (24). Whilst the structure of SphK2 has not been characterized yet, sequence alignment analyses reveal that compared with SphK1, SphK2 possesses an additional N-terminal extension and a large insertion at its C-terminal lipid-binding domain (24, 25). SphK2, but not SphK1, possesses a nuclear localization sequence and a nuclear export sequence, which are located in its N-terminal extension and C-terminal insertion, respectively (26). Thus, SphK2 can shuttle between the nucleus and cytoplasm, whereas SphK1 is solely a cytosolic protein. SphK2 is also found to function in the endoplasmic reticulum (ER) and mitochondria (27–29), which might be related to its putative transmembrane domain and BH3 domain (30, 31).
Both SphK1 and SphK2 are ubiquitously expressed in tissues and blood. SphK1 contributes more to the level of S1P in circulation, while SphK2 is the predominant SphK isoform in the liver, kidney, pancreatic islets and brain (32–34). The ablation of either Sphk1 or Sphk2 does not cause any infertility or lethality in mice, whereas knockout of both enzymes results in embryonic death, indicating they are redundant in some essential physiological functions (35). At the cellular level, the primary biological actions of SphK1 are to promote cell proliferation, migration and survival and to inhibit apoptosis, and thus upregulation of SphK1 is often associated with cancer progression, metastasis and a poor prognosis (13, 36, 37). In addition, SphK1 modulates immune response and inflammation (38, 39). In general, the biological function of SphK2 has not been well characterized. In some cases, SphK2 and SphK1 play similar roles because of their overlapping enzymatic activity in the conversion of ceramide and sphingosine into S1P; whereas in others, SphK2 exhibits different or even opposite effects due to distinct tissue distribution and subcellular localization. In particular, the role of SphK2 is highly controversial in terms of pro-apoptotic (27, 31, 40) versus anti-apoptotic (41–43). The mechanism underlying this discrepancy warrants further elucidation.
SphK1 and SphK2 exert their biological functions mainly via the following three approaches:
a) S1P Receptor-Dependent Mode: SphK often functions via its enzymatic product S1P. Once generated by SphK, S1P can be exported out of the cell where it binds to a group of five distinct, specific G protein-coupled receptors (S1P1-5) (44, 45). S1P can also travel to its target tissues via its carriers, such as albumin and ApoM-containing high-density lipoproteins (46, 47). Circulating S1P is mainly sourced from the SphK1 in erythrocytes (48). However, a recent study has found that SphK2 also determines the circulating level of S1P in the liver, where it dictates S1P clearance via a route of dephosphorylation, re-phosphorylation and lysis (49). Circulating S1P is undoubtedly implicated in whole-body metabolic regulation, but its physiological effects remain elusive. Plasma S1P is found increased in humans and rodents with obesity and insulin resistance (50), whereas another study identifies an opposite trend that serum S1P is decreased along with the development of human insulin resistance and T2D (51). However, the ApoM-bound S1P is recently found metabolically protective against insulin resistance (52). More extensive studies are required to clarify the role of circulating S1P. Cells from different tissues can express one to five different kinds of S1P receptors (53). Upon activation by S1P, S1P receptors trigger cell signaling coordinately or differentially. For instance, both S1P1 and S1P4 are required for T cell migration (54), while both S1P1 and S1P2 convey S1P-mediated protection from excitotoxicity in hippocampal neurons (55). In contrast, S1P1 and S1P2 exhibit opposite effects on pain sensitivity and endothelial dysfunction (56, 57). In addition, S1P receptor signaling is often crosstalk with other receptor-dependent pathways, such as growth factors and cytokines (58–62), leading to a complexity of the actions of S1P.
b) Intracellular Partner Mode: SphK and S1P can directly bind to intracellular partners, independent of S1P receptors. For instance, at the plasma membrane, SphK1 and S1P interact with the RING domain of tumor necrosis factor receptor-associated factor 2 (TRAF2), priming the ubiquitination of receptor-interacting serine/threonine-protein kinase 1 (RIP1) and subsequent activation of nuclear factor-κB (NFκB) (63, 64). In the nucleus, SphK2 and its product S1P physically interact with histone deacetylases HDAC1 and HDAC2, implicating in the epigenetic regulation (26). At the inner membrane of mitochondria, SphK2 and S1P bind to prohibitin 2, which is essential for respiratory complex IV assembly and mitochondrial fitness (29). More intraorganellar partners of SphK are to be identified, e.g., in the ER where SphK1 regulates unfolded protein responses (27, 65), endosomes where SphK1 modulates endocytotic recycling (66, 67) and lysosomes where SphK2 mediates autophagy (68).
c) Substrate Depletion Mode: Reducing levels of ceramide and sphingosine is often regarded as a mean of S1P-independent function of SphK. For examples, SphK1 counteracts ceramide-induced apoptosis, constituting the basis of cancer drug resistance (69). Overexpression of SphK1 prevents ceramide accumulation in myocytes ameliorates muscle insulin resistance in diet-induced obese mice (70). SphK has also been found to regulate certain cellular functions via sphingosine, independent of ceramide or S1P: sphingosine specifically impairs SphK1-mediated endocytotic membrane trafficking (66); sphingosine induces cell cycle arrest in SphK1/SphK2 double knockout murine embryonic stem cells (71); sphingosine accumulation suppresses insulin signaling in SphK2 deficient hepatocytes (7); and sphingosine impairs insulin-mediated glucose uptake in L6 myotubes exposed to palmitate (72).
The liver is the central organ in maintaining glucose homeostasis. Hepatic insulin resistance is considered a key pathogenic onset of prediabetes (73). Aberrant lipid metabolism in the liver impairs hepatocyte’s response to insulin, leading to increased glucose production and decreased glucose disposal (73). Conventionally, lipid dysregulation that results in hepatic insulin resistance mainly refers to ectopic fat deposition, as seen in steatotic livers. In accord, 70-80% of T2D subjects have non-alcoholic fatty liver diseases (NAFLD), and the incidence rate of NAFLD in obese, diabetic individuals is more than 90% (5, 74). In the past decade, the role of signaling lipids, including SphK-related sphingolipids like ceramides, sphingosine, and S1P, is emerging in the pathogenesis of hepatic insulin resistance (3, 7, 75).
The role of SphK1 in hepatic fitness is discrepant, mainly because it simultaneously possesses both prosurvival and proinflammatory properties. As a prosurvival factor, SphK1 protects hepatocytes from a variety of stress stimuli. SphK1 protects NAFLD livers from ischemia/reperfusion injury by reducing reactive oxygen species and alleviating oxidative stress (76). SphK1 also mitigates palmitate-induced ER stress-associated apoptosis in hepatocytes (65). In contrast, as a proinflammatory and profibrogenic factor, SphK1 promotes NAFLD progression to non-alcoholic steatohepatitis (NASH). The hepatic SphK1 mRNA level is profoundly increased in subjects with advanced fibrotic livers (77). Ablation of Sphk1 ameliorates CCl4 or bile duct ligation (BDL)-induced liver fibrosis in mice, at least in part, by suppressing the activation and migration of hepatic stellate cells and Kupffer cells (78). SphK1 promotes protein kinase C-δ activation, triggers NFκB signaling and promotes proinflammatory cytokine production, contributing to D-GaIN and lipopolysaccharide-induced acute liver failure (79). The role of SphK1 in steatosis is context-dependent. Overexpression of SphK1 by adeno-associated virus (AAV) in the liver reduces the level of hepatic triglyceride in mice on a low fat, but not high fat, diet (80). In contrast, knockout of SphK1 suppresses hepatosteatosis in mice on a high-fat diet (HFD) (81).
SphK1 was hypothesized to reinforce hepatic insulin sensitivity as it converts ceramides to S1P. On the one hand, ceramides, in particular, C16 ceramide, impairs hepatic insulin signaling (82, 83). In normal livers, the predominant form of ceramides is C24 ceramide that is believed to be beneficial to insulin sensitivity (84). However, feeding mice with a diet rich in saturated fat can selectively increase the C16 ceramide level in the liver, which represents an explanation of the hepatic insulin resistance in diet-induced obese mice (82). On the other hand, S1P activates PI3K/Akt pathway via its receptors (85, 86). In support of this notion, overexpression of SphK1 significantly enhances hepatic insulin signaling and improves glucose tolerance in KK/Ay diabetic mice (87). Acid sphingomyelinase-induced Akt phosphorylation is abrogated in livers of Sphk1-/- C57BL/6 mice (88). However, other studies hold a conclusion against this notion. Depletion of Sphk1 does not alter insulin sensitivity in HFD-fed C57BL/6 mice (65). Liver-specific overexpression of SphK1 has no impact on insulin sensitivity and glucose tolerance in high-fat, high sugar diet-fed C57BL/6 mice (80). The phospho-Akt levels are indistinguishable in the livers of wild-type and Sphk1-/- mice upon insulin challenge (89). The insulin response is unchanged in SphK1-knockdown hepatocytes as compared with control cells (7). Furthermore, S1P impairs hepatic insulin signaling via binding to S1P receptor subtype 2 in HFD-fed New Zealand Obese mice (90). Therefore, to characterize the hepatocyte-autonomous role of SphK1 in hepatic insulin signaling, the use a tissue-specific knockout mouse model is recommended. Recently, the liver-specific Sphk1-/- C57BL/6 mice have been generated (91). This mouse strain displays no prominent physiological and pathological abnormalities under a basal condition (91). However, it is still intriguing to know if the liver-specific knockout of Sphk1 affects the metabolic vulnerability of mice upon dietary or other pathologic insults.
In general, research on SphK2 is not as comprehensive as SphK1 due to its complex subcellular localization. However, the metabolic role of SphK2 in the liver has been recently addressed. SphK2 appears a metabolically protective factor in the liver. SphK2 protects mice from both alcoholic and non-alcoholic fatty liver disease (92, 93). Ablation of Sphk2 results in an elevated level of hepatic lipid accumulation and proinflammatory cytokine production, predisposing to liver injury in C57BL/6 mice exposed to 60-day chronic alcohol feeding (92). Sphk2-/- also promotes the establishment of hepatosteatosis in mice after 2-week HFD feeding (93).
SphK2-mediated regulation of hepatic insulin signaling is likely related to its subcellular localization. Pharmacological inhibition or siRNA-mediated knockdown of SphK2 mitigates insulin-repressed gluconeogenic gene expression, leading to increased hepatic glucose production in C57BL/6 mice treated with interleukine-6 (94). This effect is reported to attribute to the dephosphorylation and deacetylation of STAT3 (94). Overexpression of SphK2 in the liver reinforces mitochondrial fatty acid β-oxidation and effectively ameliorates hepatic steatosis, glucose intolerance and insulin resistance in C57BL/6 mice on an HFD (95). We have recently generated liver-specific Sphk2-/- (SphK2-LKO) mice by crossbreeding floxed Sphk2 mice with Alb-Cre strain, providing a reliable model to further characterize the role of SphK2 in the liver (7). SphK2-LKO mice exhibit impaired glucose homeostasis and insulin responsiveness on both normal diet and HFD conditions. SphK2-LKO upregulates gluconeogenic genes and downregulates glucose disposal genes, leading to an increase in hepatic glucose production. Inhibition of SphK2 by the selective inhibitors or shRNA-mediated SphK2 knockdown in hepatocytes significantly suppresses insulin-induced activation of the phosphoinositide 3-kinase (PI3K)/Akt signaling pathway (7). Interestingly, treatment of SphK2-deficient hepatocytes with S1P fails to revert insulin signaling. By contrast, myriocin, an inhibitor that blocks the biosynthesis of all sphingolipids, including S1P, can restore insulin-induced Akt phosphorylation, indicating that S1P is unrelated to SphK2-mediated regulation of hepatic insulin signaling (7). Furthermore, inhibition of ceramide synthesis by fumonisin b1 has no impact on hepatic insulin sensitivity, whereas blocking ceramide degradation via pharmacological inhibition or knockdown of acid ceramidase restores insulin sensitivity in SphK2-deficient hepatocytes (7). This finding supports the notion that hepatic ceramide levels are often unrelated to hepatic insulin sensitivity in humans and rodents [as reviewed in (75)]. Notably, we found that hepatic levels of sphingosine are critically associated with insulin sensitivity both in vitro and in vivo (7). Moreover, treatment of hepatocytes with sphingosine significantly inhibits the insulin-induced PI3K activity and Akt phosphorylation (7). However, how sphingosine inhibits PI3K remains unknown. It has been demonstrated that sphingosine can both physically and functionally interact with the protein 14-3-3ζ (96), which, in turn, promotes plasma membrane recruitment and activation of PI3K (97, 98) To what extent this pathway contributes to the regulation of hepatic insulin signaling warrants further investigations.
The major contributions of skeletal muscle in maintaining euglycemia are glucose uptake and disposal (99). In both postprandial state and the hyperinsulinemic-euglycemic clamp experiments, skeletal muscle uptakes approximately 80% of blood glucose (100, 101). The insulin signaling pathway in skeletal muscle is also centered with PI3K/Akt, specifically promoting Akt-driven glucose uptake, utilization and storage via the regulation of glucose transporter type 4 (GLUT4) (102). Lipid overload is also a chief cause of insulin resistance in myocytes. HFD feeding or lipid infusion causes diacylglycerol accumulation, which activates protein kinase C θ, negatively regulating insulin signaling in skeletal muscle (103–105).
HFD feeding of mice or treatment of C2C12 myoblasts with palmitate results in a dramatic increase in myocyte SphK1 mRNA expression (106). Notably, in these lipid-induced insulin-resistant myocytes or muscle tissues, levels of sphingolipids, including S1P, are all increased (106). Levels of ceramide, sphingosine and S1P are also profoundly increased in palmitate-treated L6 myotubes (72). By far, the majority of studies support a metabolically protective role of SphK1 in muscle insulin resistance. For instance, adenoviral overexpression of SphK1 enhances, whereas siRNA-mediated knockdown of SphK1 suppresses insulin-mediated glucose uptake in murine C2C12 myoblasts (87). In line with this, transgenic overexpression of SphK1 that promotes intramuscular ceramide conversion into S1P significantly improves muscle and whole-body insulin resistance in mice on an HFD for 6 weeks (70). In addition, S1P can trans-activate myocyte insulin signaling in the absence of insulin stimulation and thus enhance glucose uptake (107). Inhibition of sphingolipid synthesis by myriocin restores, whereas inhibition of SphKs by non-selective inhibitor SKI-II further suppresses, the impaired insulin-mediated glucose uptake in L6 myotubes exposed to palmitate (72). Interestingly, the level of sphingosine, but not ceramide or S1P, is always correlated to insulin’s actions in these experimental settings, suggesting an important role for sphingosine (72). However, Ross et al. reported that SphK1/S1P promotes muscle insulin resistance in HFD-fed C57BL/6 mice through S1P3 receptor-dependent elevation of interleukin-6 production by skeletal muscle (108). Thus, the role of SphK1/S1P in muscle insulin resistance appears contradictory in the literature, which needs further clarification, especially by using myocyte-specific Sphk1-/- mice.
There is no direct experimental evidence depicting the role of SphK2 in muscle insulin resistance. Transiently overexpression of SphK2 in skeletal muscle using in vivo electroporation has no impact on sphingomyelin and ceramide levels (70). However, the study did not determine the levels of sphingosine and S1P or insulin sensitivity (70). The same research group has later found that oral administration of FTY720 for 6 weeks abrogates lipid accumulation and improves glucose uptake in the skeletal muscle of C57BL/6 mice on an HFD (109). FTY720 elicits two possible working mechanisms: a) It can be phosphorylated into FTY-720-P, primarily by SphK2, and act on S1P receptors as an S1P mimetic (110); b) It functions as an inhibitor of SphK (both SphK1 and SphK2) and ceramide synthases (111–113). Nevertheless, how FTY720 improves muscle insulin resistance and whether SphK2 is involved in this regulation remain elusive.
White adipose tissue is the main reservoir of fat storage in our body. In each adipocyte, fat is usually stored in the form of triglyceride in a unilocular lipid droplet (6). When needed, white adipose tissue expands its fat storage capacity by increasing either the number (hyperplasia) or size (hypertrophy) of adipocytes, leading to obesity (6). These two types of adipose tissue expansion result in distinct metabolic outcomes. Insulin resistance is often associated with hypertrophic obesity but not adipocyte hyperplasia (114, 115). In recent years, the expandability of white adipose tissue is emerging as a widely accepted concept to explain to non-obese/insulin-resistant and obese/insulin-sensitive populations (6). When excess fat overwhelms the storage capacity of white adipose tissue, ectopic lipid accumulation will occur in the liver, skeletal muscle and heart, resulting in metabolic disorders (6). For example, severe insulin resistance is seen in patients with congenital generalized lipodystrophy, a condition in which white adipose tissue is near completely lost (116). White adipose tissue also functions as an endocrine organ regulating glucose homeostasis via secretion of adipokines, e.g., adiponectin, resistin and leptin that improve metabolic profile (117, 118), or the cytokines that impair adipose tissue and whole-body insulin responsiveness, linking obesity to T2D (119).
Recently, two important studies on the role of SphK1 in adipose insulin resistance have been reported by Cowart laboratory (89, 120). They found that SphK1 expression is upregulated in both palmitate-treated white adipocytes in vitro and white adipose tissue in mice on an HFD in vivo (89). After 16 weeks of HFD feeding, Sphk1-/- mice develop a comparable body weight as wild-type counterparts, but they exhibit prominent adipocyte hyperplasia and upregulation of adipogenic transcription factors (89). As a result, Sphk1-/- significantly improves whole-body metabolic abnormalities and, particularly, rescues Akt signaling in white adipose tissue of HFD-fed mice (89). This is attributed to the alleviation of a proinflammatory phenotype in white adipose tissue (89). In support of this, SphK1 is found to promote adipose tissue macrophage survival and interleukin-6 production (121, 122). To further elucidate the adipose tissue-specific role of SphK1, Cowart laboratory has generated SphK1fatKO mice on a C57BL/6 background by crossbreeding floxed SphK1 mice with Adipoq-Cre strain (120). In marked contrast to the anti-diabetic effects of global depletion of Sphk1, SphK1fatKO unexpectedly causes severely impaired glucose tolerance and significantly elevated fasting glucose in diet-induced obese mice (120). Mechanistically, SphK1fatKO suppresses adipocyte lipolysis, leading to hypertrophic obesity (120). This work clearly indicates that SphK1 is essential in adipose tissue to prevent metabolic abnormalities. It also suggests that the anti-diabetic benefits seen in global Sphk1-/- mice might derive be related to the proinflammatory effects of SphK1in other cell types (89, 120). By comparing these two studies, we are more aware of the necessity of using tissue-specific genetically modified mice in SphK studies.
Opposing to Sphk1-/- that increases adiposity, the global ablation of Sphk2 progressively decreases fat mass in standard chow diet-fed mice along with aging (123). In aged mice at 50–52 weeks old, depletion of Sphk2 profoundly elevates glucose tolerance in both male and female mice (123). In males but not female mice, Sphk2-/- improves insulin sensitivity to a much lesser extent (123). Dramatically reduced white adipose tissue mass can be partially attributable to the increased adipocyte lipolysis and energy expenditure (123). Taking the lesson from Sphk1-/- models as mentioned above, the adipose-specific actions of SphK2 warrant further investigations using adipose-specific knockout mice.
Pancreatic β-cells are the only source of insulin production. Insulin secretion is primarily stimulated by glucose, which, in turn, lowers the glucose level in the circulation (124). Pancreatic β-cell failure, including both dysfunction and cell death, is the fundamental cause of hyperglycemia in diabetes. In prediabetic states, although insulin resistance exists, the blood glucose level is just subtly increased, which is attributed to a compensated hypersecretion of insulin by β-cells (125, 126). When β-cell compensation fails due to either dysfunction or cell death, hyperglycemia takes place as a key feature of the onset of diabetes (125, 126). Aberrant lipid accumulation is a chief factor of inducing β-cell dysfunction and death, designated as β-cell lipotoxicity, which is often seen in obese and prediabetic subjects (125, 126).
SphK1 and SphK2 are both suggested to be implicated in the regulation of β-cell’s insulin secretory function. In rat INS-1β-cell line and primary islets, SphK2 is the predominant isoform of SphK, whereas SphK1 is hardly detected (34). In contrast, SphK1 level is induced by cytokine challenge, whereas SphK2 expression is unchanged (34). Inhibition of SphK1 and SphK2 by the inhibitor SKI impairs glucose-stimulated insulin secretion (GSIS) in both MIN6 β-cell line and C57BL/6 mice (127). In line with this, blocking S1P dephosphorylation into sphingosine by knockdown of S1P phosphatase-1 enhances insulin production in MIN6 cells (127). These data indicate that the sphingolipid rheostat is a determinant of GSIS in β-cells. However, it is controversial in terms of which isoform of SphK is primarily responsible for this regulation. Hasan et al. reported that SphK1 knockdown suppresses GSIS in INS-1 cells (128). On the contrary, Cantrell et al. found that knockdown of SphK2, but not SphK1, abolishes GSIS in MIN6 cells (127). This discrepancy is yet to be resolved.
As it has been documented in many other types of cells, SphK1 and SphK2 are both implicated in the regulation of β-cell viability. Overexpression of SphK1 inhibits palmitate-induced lipotoxicity in INS-1 cells exposed to high glucose (30 mM) (129). This effect is independent of S1P receptors, as the inhibitors or antagonists of these receptors cannot compromise SphK1-mediated protection (129). According to the lipidomic analyses, overexpression of SphK1 mainly reduces the level of C16 ceramide in palmitate-treated INS-1 cells under low glucose (5 mM) condition, but C18, C24 and C26 ceramides in high glucose condition (129). It has been shown that ER-to-Golgi trafficking is essential for ceramide-induced lipotoxicity in β-cells (130). Interestingly, overexpression of SphK1 prevents palmitate-mediated impairment of ER-to-Golgi protein transport (129). Our laboratory has recently demonstrated a protective role of SphK1 in β-cell lipotoxicity in vivo (131). We found that ablation of Sphk1 promotes the development of impaired fasting glucose and impaired glucose tolerance in C57BL/6 mice on an HFD for 24 weeks. In marked contrast to hyperinsulinemia found in wild-type littermates, Sphk1-/- mice exhibit significantly reduced levels of plasma insulin. Under a similar degree of whole-body insulin resistance, Sphk1-/- potentiates lipid-induced apoptosis of pancreatic β-cells, leading to the diabetic phenotype. The antiapoptotic effects of SphK1 are reproducible in palmitate-treated isolated islets ex vivo (130). In marked contrast to the protective role of SphK1, we have recently identified SphK2 as an endogenous proapoptotic factor in β-cells, profoundly promoting lipotoxicity (40). Ablation of Sphk2 partially reserves β-cell mass and thus improves diabetic phenotype in an animal model of T2D (treated with a combination of HFD and streptozotocin) (40). In addition, knockdown of SphK2 by siRNA or inhibition of SphK2 by ABC294640 abrogates palmitate-induced apoptosis in INS-1 cells (40). Mechanistically, palmitate stimulates nuclear export of SphK2 to the cytoplasm, where SphK2 mediates mitochondria-dependent apoptosis signaling via its BH3 domain (40). Taken together, our findings that SphK1 protects but SphK2 promotes β-cell lipotoxicity suggest a new strategy by balancing between the signaling of SphK1 and SphK2 in β-cells for the prevention and treatment of diabetes.
Over the last decade, there have been extensive efforts to explore the potential roles of SphK1 and SphK2 in T2D (Figure 2). In hepatic insulin resistance, SphK2 has been illustrated as a metabolically protective factor, whereas the effects of SphK1 are controversial. In muscle insulin resistance, the role of SphK1 is still under debate, while little is known about SphK2. In white adipose tissue, SphK1 prevents obesity-associated diabetes, whereas the adipose-specific role of SphK2 remains elusive. Both SphK1 and SphK2 have been found essential for GSIS in pancreatic β-cells; however, which of the two isoforms takes in lead warrants further clarification. Remarkably, SphK1 and SphK2 exert opposite effects in protecting β-cells from lipotoxicity.
Figure 2 Roles of SphK1 and SphK2 in T2D. Most of the literature supports an anti-diabetic role (denoted by green arrows) of SphK1 in skeletal muscle, adipose tissue and pancreas as well as SphK2 in the liver. In contrast, SphK2 is primarily a pro-diabetic factor (denoted by the red arrow) for its proapoptotic effects in pancreatic β-cells. The roles of SphK1 in the liver and SphK2 in skeletal muscle and adipose tissue are controversial (denoted by grey lines). Created in BioRender.com.
It should be taken into consideration in further studies that the complicated roles for the two isoforms of SphK in T2D are related to multiple layers of complexities as follows: i) SphK1 versus SphK2. The two isoenzymes sometimes exhibit the same effects, as they share common catalytic actions in the conversion of ceramide/sphingosine into S1P, while they sometimes lead to discrepant pathophysiological outcomes due to their distinct tissue distribution, subcellular localization and molecular partners. ii) Prosurvival versus proinflammatory role of SphK1. SphK1 simultaneously possess both prosurvival and proinflammatory properties. However, its prosurvival effects are often associated with an anti-diabetic outcome, whereas proinflammatory signaling could promote the development of diabetes. This controversy can be seen in research on hepatic and muscle insulin resistance. In addition, the opposite metabolic profiles from global and adipose-specific Sphk1-/- mice draw our attention to that the proinflammatory actions of SphK1 might derive from the immune cells in the insulin target tissue, but not parenchymal cells. iii) S1P receptors. S1P receptor subtypes 1-5 have been implicated in a variety of cell signaling pathways, which can lead to different and even opposite biological functions. For any S1P-related regulation, SphK and S1P receptors should be co-investigated, particularly in vivo. iv) Sphingolipid metabolites. Increasing evidence has shown that not only ceramides and S1P, but also many other sphingolipid metabolites, such as sphingosine, sphingomyelin and ganglioside, function as critical regulators of insulin actions in different experimental settings. This notion has brought new possibilities for a better explanation of the role of SphK and sphingolipids in the pathogenesis of diabetes.
In conclusion, since identified in the late 1990s, SphK1 and SphK2 have been extensively studied in a wide variety of biomedical fields. Their potential roles in T2D have just been explored recently and remain to be further characterized. Tissue-specific knockout mice are powerful tools to dissect the cell-autonomous effects of SphK. So far, only hepatocyte-specific Sphk2-/- and adipocyte-specific Sphk1-/- have been applied in diabetes research. In addition, with the development of sphingolipidomic analyses, a more precise and comprehensive measurement of sphingolipid composition in different pathophysiological contexts should be performed. Furthermore, the knowledge related to genetic and epigenetic regulation of SphK1 and SphK2in T2D remains largely unknown. Nevertheless, a comprehensive understanding of the role of SphK and the associated sphingolipids in T2D will help to identify druggable targets for prevention and treatment of the disease in the future.
PX and YQ conceived and wrote the manuscript. WW, ZS, GA, and XL provided technical assistance. All authors contributed to the article and approved the submitted version.
This study was supported by National Natural Science Foundation of China (NSFC)–National Health and Medical Research Council, Australia (NHMRC) Joint Research Grants 81561128014 (to PX) and APP1113527 (to Mathew A Vadas), NSFC Grants 81370937 and 81870559 (to PX), NHMRC Project Grant APP1162545 (to YQ) and Fudan Distinguished Professorship (to PX).
The authors declare that the research was conducted in the absence of any commercial or financial relationships that could be construed as a potential conflict of interest.
2. Albrink MJ. Diet, Diabetes, and Serum Lipids. Diabetes (1964) 13:425–9. doi: 10.2337/diab.13.4.425
3. Perry RJ, Samuel VT, Petersen KF, Shulman GI. The role of hepatic lipids in hepatic insulin resistance and type 2 diabetes. Nature (2014) 510:84–91. doi: 10.1038/nature13478
4. Eid S, Sas KM, Abcouwer SF, Feldman EL, Gardner TW, Pennathur S, et al. New insights into the mechanisms of diabetic complications: role of lipids and lipid metabolism. Diabetologia (2019) 62:1539–49. doi: 10.1007/s00125-019-4959-1
5. Xia MF, Bian H, Gao X. NAFLD and Diabetes: Two Sides of the Same Coin? Rationale for Gene-Based Personalized NAFLD Treatment. Front Pharmacol (2019) 10:877. doi: 10.3389/fphar.2019.00877
6. Qi Y, Sun L, Yang H. Lipid droplet growth and adipocyte development: mechanistically distinct processes connected by phospholipids. Biochim Biophys Acta Mol Cell Biol Lipids (2017) 1862:1273–83. doi: 10.1016/j.bbalip.2017.06.016
7. Aji G, Huang Y, Ng ML, Wang W, Lan T, Li M, et al. Regulation of hepatic insulin signaling and glucose homeostasis by sphingosine kinase 2. Proc Natl Acad Sci U S A (2020) 117:24434–42. doi: 10.1073/pnas.2007856117
8. Fahy E, Sud M, Cotter D, Subramaniam S. LIPID MAPS online tools for lipid research. Nucleic Acids Res (2007) 35:W606–12. doi: 10.1093/nar/gkm324
9. Rosenberg A, Stern N. Changes in sphingosine and fatty acid components of the gangliosides in developing rat and human brain. J Lipid Res (1966) 7:122–31. doi: 10.1016/S0022-2275(20)39594-8
10. Fyrst H, Herr DR, Harris GL, Saba JD. Characterization of free endogenous C14 and C16 sphingoid bases from Drosophila melanogaster. J Lipid Res (2004) 45:54–62. doi: 10.1194/jlr.M300005-JLR200
11. Stoffel W. Studies on the biosynthesis and degradation of sphingosine bases. Chem Phys Lipids (1970) 5:139–58. doi: 10.1016/0009-3084(70)90014-9
12. Hannun YA, Obeid LM. Sphingolipids and their metabolism in physiology and disease. Nat Rev Mol Cell Biol (2018) 19:175–91. doi: 10.1038/nrm.2017.107
13. Ogretmen B. Sphingolipid metabolism in cancer signalling and therapy. Nat Rev Cancer (2018) 18:33–50. doi: 10.1038/nrc.2017.96
14. Huitema K, van den Dikkenberg J, Brouwers JF, Holthuis JC. Identification of a family of animal sphingomyelin synthases. EMBO J (2004) 23:33–44. doi: 10.1038/sj.emboj.7600034
15. Basu S, Kaufman B, Roseman S. Enzymatic synthesis of ceramide-glucose and ceramide-lactose by glycosyltransferases from embryonic chicken brain. J Biol Chem (1968) 243:5802–4. doi: 10.1016/S0021-9258(18)91935-6
16. Senkal CE, Salama MF, Snider AJ, Allopenna JJ, Rana NA, Koller A, et al. Ceramide Is Metabolized to Acylceramide and Stored in Lipid Droplets. Cell Metab (2017) 25:686–97. doi: 10.1016/j.cmet.2017.02.010
17. Gatt S. Enzymatic hydrolysis of sphingolipids. I. Hydrolysis and synthesis of ceramides by an enzyme from rat brain. J Biol Chem (1966) 241:3724–30. doi: 10.1016/S0021-9258(18)99832-7
18. Kohama T, Olivera A, Edsall L, Nagiec MM, Dickson R, Spiegel S. Molecular cloning and functional characterization of murine sphingosine kinase. J Biol Chem (1998) 273:23722–8. doi: 10.1074/jbc.273.37.23722
19. Liu H, Sugiura M, Nava VE, Edsall LC, Kono K, Poulton S, et al. Molecular cloning and functional characterization of a novel mammalian sphingosine kinase type 2 isoform. J Biol Chem (2000) 275:19513–20. doi: 10.1074/jbc.M002759200
20. Saba JD, Nara F, Bielawska A, Garrett S, Hannun YA. The BST1 gene of Saccharomyces cerevisiae is the sphingosine-1-phosphate lyase. J Biol Chem (1997) 272:26087–90. doi: 10.1074/jbc.272.42.26087
21. Taha TA, Hannun YA, Obeid LM. Sphingosine kinase: biochemical and cellular regulation and role in disease. J Biochem Mol Biol (2006) 39:113–31. doi: 10.5483/bmbrep.2006.39.2.113
22. Cuvillier O, Pirianov G, Kleuser B, Vanek PG, Coso OA, Gutkind S, et al. Suppression of ceramide-mediated programmed cell death by sphingosine-1-phosphate. Nature (1996) 381:800–3. doi: 10.1038/381800a0
23. Labesse G, Douguet D, Assairi L, Gilles AM. Diacylglyceride kinases, sphingosine kinases and NAD kinases: distant relatives of 6-phosphofructokinases. Trends Biochem Sci (2002) 27:273–5. doi: 10.1016/s0968-0004(02)02093-5
24. Wang Z, Min X, Xiao SH, Johnstone S, Romanow W, Meininger D, et al. Molecular basis of sphingosine kinase 1 substrate recognition and catalysis. Structure (2013) 21:798–809. doi: 10.1016/j.str.2013.02.025
25. Wang J, Knapp S, Pyne NJ, Pyne S, Elkins JM. Crystal Structure of Sphingosine Kinase 1 with PF-543. ACS Med Chem Lett (2014) 5:1329–33. doi: 10.1021/ml5004074
26. Hait NC, Allegood J, Maceyka M, Strub GM, Harikumar KB, Singh SK, et al. Regulation of histone acetylation in the nucleus by sphingosine-1-phosphate. Science (2009) 325:1254–7. doi: 10.1126/science.1176709
27. Maceyka M, Sankala H, Hait NC, Le Stunff H, Liu H, Toman R, et al. SphK1 and SphK2, sphingosine kinase isoenzymes with opposing functions in sphingolipid metabolism. J Biol Chem (2005) 280:37118–29. doi: 10.1074/jbc.M502207200
28. Chipuk JE, McStay GP, Bharti A, Kuwana T, Clarke CJ, Siskind LJ, et al. Sphingolipid metabolism cooperates with BAK and BAX to promote the mitochondrial pathway of apoptosis. Cell (2012) 148:988–1000. doi: 10.1016/j.cell.2012.01.038
29. Strub GM, Paillard M, Liang J, Gomez L, Allegood JC, Hait NC, et al. Sphingosine-1-phosphate produced by sphingosine kinase 2 in mitochondria interacts with prohibitin 2 to regulate complex IV assembly and respiration. FASEB J (2011) 25:600–12. doi: 10.1096/fj.10-167502
30. Don AS, Rosen H. A lipid binding domain in sphingosine kinase 2. Biochem Biophys Res Commun (2009) 380:87–92. doi: 10.1016/j.bbrc.2009.01.075
31. Liu H, Toman RE, Goparaju SK, Maceyka M, Nava VE, Sankala H, et al. Sphingosine kinase type 2 is a putative BH3-only protein that induces apoptosis. J Biol Chem (2003) 278:40330–6. doi: 10.1074/jbc.M304455200
32. Allende ML, Sasaki T, Kawai H, Olivera A, Mi Y, van Echten-Deckert G, et al. Mice deficient in sphingosine kinase 1 are rendered lymphopenic by FTY720. J Biol Chem (2004) 279:52487–92. doi: 10.1074/jbc.M406512200
33. Blondeau N, Lai Y, Tyndall S, Popolo M, Topalkara K, Pru JK, et al. Distribution of sphingosine kinase activity and mRNA in rodent brain. J Neurochem (2007) 103:509–17. doi: 10.1111/j.1471-4159.2007.04755.x
34. Mastrandrea LD, Sessanna SM, Laychock SG. Sphingosine kinase activity and sphingosine-1 phosphate production in rat pancreatic islets and INS-1 cells: response to cytokines. Diabetes (2005) 54:1429–36. doi: 10.2337/diabetes.54.5.1429
35. Mizugishi K, Yamashita T, Olivera A, Miller GF, Spiegel S, Proia RL. Essential role for sphingosine kinases in neural and vascular development. Mol Cell Biol (2005) 25:11113–21. doi: 10.1128/MCB.25.24.11113-11121.2005
36. Maceyka M, Rohrbach T, Milstien S, Spiegel S. Role of Sphingosine Kinase 1 and Sphingosine-1-Phosphate Axis in Hepatocellular Carcinoma. Handb Exp Pharmacol (2020) 259:3–17. doi: 10.1007/164_2019_217
37. Vadas M, Xia P, McCaughan G, Gamble J. The role of sphingosine kinase 1 in cancer: oncogene or non-oncogene addiction? Biochim Biophys Acta (2008) 1781:442–7. doi: 10.1016/j.bbalip.2008.06.007
38. Pyne S, Adams DR, Pyne NJ. Sphingosine 1-phosphate and sphingosine kinases in health and disease: Recent advances. Prog Lipid Res (2016) 62:93–106. doi: 10.1016/j.plipres.2016.03.001
39. Weigert A, Olesch C, Brune B. Sphingosine-1-Phosphate and Macrophage Biology-How the Sphinx Tames the Big Eater. Front Immunol (2019) 10:1706. doi: 10.3389/fimmu.2019.01706
40. Song Z, Wang W, Li N, Yan S, Rong K, Lan T, et al. Sphingosine kinase 2 promotes lipotoxicity in pancreatic beta-cells and the progression of diabetes. FASEB J (2019) 33:3636–46. doi: 10.1096/fj.201801496R
41. Neubauer HA, Pham DH, Zebol JR, Moretti PA, Peterson AL, Leclercq TM, et al. An oncogenic role for sphingosine kinase 2. Oncotarget (2016) 7:64886–99. doi: 10.18632/oncotarget.11714
42. Van Brocklyn JR, Jackson CA, Pearl DK, Kotur MS, Snyder PJ, Prior TW. Sphingosine kinase-1 expression correlates with poor survival of patients with glioblastoma multiforme: roles of sphingosine kinase isoforms in growth of glioblastoma cell lines. J Neuropathol Exp Neurol (2005) 64:695–705. doi: 10.1097/01.jnen.0000175329.59092.2c
43. Panneer Selvam S, Roth BM, Nganga R, Kim J, Cooley MA, Helke K, et al. Balance between senescence and apoptosis is regulated by telomere damage-induced association between p16 and caspase-3. J Biol Chem (2018) 293:9784–800. doi: 10.1074/jbc.RA118.003506
44. Spiegel S, Milstien S. Sphingosine-1-phosphate: an enigmatic signalling lipid. Nat Rev Mol Cell Biol (2003) 4:397–407. doi: 10.1038/nrm1103
45. Kim RH, Takabe K, Milstien S, Spiegel S. Export and functions of sphingosine-1-phosphate. Biochim Biophys Acta (2009) 1791:692–6. doi: 10.1016/j.bbalip.2009.02.011
46. Christoffersen C, Obinata H, Kumaraswamy SB, Galvani S, Ahnstrom J, Sevvana M, et al. Endothelium-protective sphingosine-1-phosphate provided by HDL-associated apolipoprotein M. Proc Natl Acad Sci USA (2011) 108:9613–8. doi: 10.1073/pnas.1103187108
47. Yatomi Y. Plasma sphingosine 1-phosphate metabolism and analysis. Biochim Biophys Acta (2008) 1780:606–11. doi: 10.1016/j.bbagen.2007.10.006
48. Pappu R, Schwab SR, Cornelissen I, Pereira JP, Regard JB, Xu Y, et al. Promotion of lymphocyte egress into blood and lymph by distinct sources of sphingosine-1-phosphate. Science (2007) 316:295–8. doi: 10.1126/science.1139221
49. Kharel Y, Huang T, Salamon A, Harris TE, Santos WL, Lynch KR. Mechanism of sphingosine 1-phosphate clearance from blood. Biochem J (2020) 477:925–35. doi: 10.1042/BCJ20190730
50. Kowalski GM, Carey AL, Selathurai A, Kingwell BA, Bruce CR. Plasma sphingosine-1-phosphate is elevated in obesity. PloS One (2013) 8:e72449. doi: 10.1371/journal.pone.0072449
51. Sui J, He M, Wang Y, Zhao X, He Y, Shi B. Sphingolipid metabolism in type 2 diabetes and associated cardiovascular complications. Exp Ther Med (2019) 18:3603–14. doi: 10.3892/etm.2019.7981
52. Kurano M, Tsukamoto K, Shimizu T, Kassai H, Nakao K, Aiba A, et al. Protection Against Insulin Resistance by Apolipoprotein M/Sphingosine-1-Phosphate. Diabetes (2020) 69:867–81. doi: 10.2337/db19-0811
53. Wang C, Mao J, Redfield S, Mo Y, Lage JM, Zhou X. Systemic distribution, subcellular localization and differential expression of sphingosine-1-phosphate receptors in benign and malignant human tissues. Exp Mol Pathol (2014) 97:259–65. doi: 10.1016/j.yexmp.2014.07.013
54. Xiong Y, Piao W, Brinkman CC, Li L, Kulinski JM, Olivera A, et al. CD4 T cell sphingosine 1-phosphate receptor (S1PR)1 and S1PR4 and endothelial S1PR2 regulate afferent lymphatic migration. Sci Immunol (2019) 4:eaav1263. doi: 10.1126/sciimmunol.aav1263
55. Tran C, Heng B, Teo JD, Humphrey SJ, Qi Y, Couttas TA, et al. Sphingosine 1-phosphate but not Fingolimod protects neurons against excitotoxic cell death by inducing neurotrophic gene expression in astrocytes. J Neurochem (2020) 153:173–88. doi: 10.1111/jnc.14917
56. Li Y, Li H, Han J. Sphingosine-1-phosphate receptor 2 modulates pain sensitivity by suppressing the ROS-RUNX3 pathway in a rat model of neuropathy. J Cell Physiol (2020) 235:3864–73. doi: 10.1002/jcp.29280
57. Chen S, Yang J, Xiang H, Chen W, Zhong H, Yang G, et al. Role of sphingosine-1-phosphate receptor 1 and sphingosine-1-phosphate receptor 2 in hyperglycemia-induced endothelial cell dysfunction. Int J Mol Med (2015) 35:1103–8. doi: 10.3892/ijmm.2015.2100
58. Hobson JP, Rosenfeldt HM, Barak LS, Olivera A, Poulton S, Caron MG, et al. Role of the sphingosine-1-phosphate receptor EDG-1 in PDGF-induced cell motility. Science (2001) 291:1800–3. doi: 10.1126/science.1057559
59. Sukocheva O, Wadham C, Holmes A, Albanese N, Verrier E, Feng F, et al. Estrogen transactivates EGFR via the sphingosine 1-phosphate receptor Edg-3: the role of sphingosine kinase-1. J Cell Biol (2006) 173:301–10. doi: 10.1083/jcb.200506033
60. Dai L, Qi Y, Chen J, Kaczorowski D, Di W, Wang W, et al. Sphingosine kinase (SphK) 1 and SphK2 play equivalent roles in mediating insulin’s mitogenic action. Mol Endocrinol (2014) 28:197–207. doi: 10.1210/me.2013-1237
61. Igarashi J, Erwin PA, Dantas AP, Chen H, Michel T. VEGF induces S1P1 receptors in endothelial cells: Implications for cross-talk between sphingolipid and growth factor receptors. Proc Natl Acad Sci USA (2003) 100:10664–9. doi: 10.1073/pnas.1934494100
62. Hutami IR, Tanaka E, Izawa T. Crosstalk between Fas and S1P1 signaling via NF-kB in osteoclasts controls bone destruction in the TMJ due to rheumatoid arthritis. Jpn Dent Sci Rev (2019) 55:12–9. doi: 10.1016/j.jdsr.2018.09.004
63. Alvarez SE, Harikumar KB, Hait NC, Allegood J, Strub GM, Kim EY, et al. Sphingosine-1-phosphate is a missing cofactor for the E3 ubiquitin ligase TRAF2. Nature (2010) 465:1084–8. doi: 10.1038/nature09128
64. Xia P, Wang L, Moretti PA, Albanese N, Chai F, Pitson SM, et al. Sphingosine kinase interacts with TRAF2 and dissects tumor necrosis factor-alpha signaling. J Biol Chem (2002) 277:7996–8003. doi: 10.1074/jbc.M111423200
65. Qi Y, Wang W, Chen J, Dai L, Kaczorowski D, Gao X, et al. Sphingosine Kinase 1 Protects Hepatocytes from Lipotoxicity via Down-regulation of IRE1alpha Protein Expression. J Biol Chem (2015) 290:23282–90. doi: 10.1074/jbc.M115.677542
66. Young MM, Takahashi Y, Fox TE, Yun JK, Kester M, Wang HG. Sphingosine Kinase 1 Cooperates with Autophagy to Maintain Endocytic Membrane Trafficking. Cell Rep (2016) 17:1532–45. doi: 10.1016/j.celrep.2016.10.019
67. Shen H, Giordano F, Wu Y, Chan J, Zhu C, Milosevic I, et al. Coupling between endocytosis and sphingosine kinase 1 recruitment. Nat Cell Biol (2014) 16:652–62. doi: 10.1038/ncb2987
68. Ishimaru K, Yoshioka K, Kano K, Kurano M, Saigusa D, Aoki J, et al. Sphingosine kinase-2 prevents macrophage cholesterol accumulation and atherosclerosis by stimulating autophagic lipid degradation. Sci Rep (2019) 9:18329. doi: 10.1038/s41598-019-54877-6
69. Bektas M, Jolly PS, Muller C, Eberle J, Spiegel S, Geilen CC. Sphingosine kinase activity counteracts ceramide-mediated cell death in human melanoma cells: role of Bcl-2 expression. Oncogene (2005) 24:178–87. doi: 10.1038/sj.onc.1208019
70. Bruce CR, Risis S, Babb JR, Yang C, Kowalski GM, Selathurai A, et al. Overexpression of sphingosine kinase 1 prevents ceramide accumulation and ameliorates muscle insulin resistance in high-fat diet-fed mice. Diabetes (2012) 61:3148–55. doi: 10.2337/db12-0029
71. Pandey S, Banks KM, Kumar R, Kuo A, Wen D, Hla T, et al. Sphingosine kinases protect murine embryonic stem cells from sphingosine-induced cell cycle arrest. Stem Cells (2020) 38:613–23. doi: 10.1002/stem.3145
72. Miklosz A, Lukaszuk B, Baranowski M, Gorski J, Chabowski A. Effects of inhibition of serine palmitoyltransferase (SPT) and sphingosine kinase 1 (SphK1) on palmitate induced insulin resistance in L6 myotubes. PloS One (2013) 8:e85547. doi: 10.1371/journal.pone.0085547
73. Santoleri D, Titchenell PM. Resolving the Paradox of Hepatic Insulin Resistance. Cell Mol Gastroenterol Hepatol (2019) 7:447–56. doi: 10.1016/j.jcmgh.2018.10.016
74. Tolman KG, Fonseca V, Dalpiaz A, Tan MH. Spectrum of liver disease in type 2 diabetes and management of patients with diabetes and liver disease. Diabetes Care (2007) 30:734–43. doi: 10.2337/dc06-1539
75. Petersen MC, Shulman GI. Roles of Diacylglycerols and Ceramides in Hepatic Insulin Resistance. Trends Pharmacol Sci (2017) 38:649–65. doi: 10.1016/j.tips.2017.04.004
76. Li Q, Qian J, Li Y, Huang P, Liang H, Sun H, et al. Generation of sphingosine-1-phosphate by sphingosine kinase 1 protects nonalcoholic fatty liver from ischemia/reperfusion injury through alleviating reactive oxygen species production in hepatocytes. Free Radic Biol Med (2020) 159:136–49. doi: 10.1016/j.freeradbiomed.2020.07.004
77. Sato M, Ikeda H, Uranbileg B, Kurano M, Saigusa D, Aoki J, et al. Sphingosine kinase-1, S1P transporter spinster homolog 2 and S1P2 mRNA expressions are increased in liver with advanced fibrosis in human. Sci Rep (2016) 6:32119. doi: 10.1038/srep32119
78. Lan T, Li C, Yang G, Sun Y, Zhuang L, Ou Y, et al. Sphingosine kinase 1 promotes liver fibrosis by preventing miR-19b-3p-mediated inhibition of CCR2. Hepatology (2018) 68:1070–86. doi: 10.1002/hep.29885
79. Lei YC, Yang LL, Li W, Luo P. Sphingosine kinase 1 dependent protein kinase C-delta activation plays an important role in acute liver failure in mice. World J Gastroenterol (2015) 21:13438–46. doi: 10.3748/wjg.v21.i48.13438
80. Kowalski GM, Kloehn J, Burch ML, Selathurai A, Hamley S, Bayol SA, et al. Overexpression of sphingosine kinase 1 in liver reduces triglyceride content in mice fed a low but not high-fat diet. Biochim Biophys Acta (2015) 1851:210–9. doi: 10.1016/j.bbalip.2014.12.002
81. Chen J, Wang W, Qi Y, Kaczorowski D, McCaughan GW, Gamble JR, et al. Deletion of sphingosine kinase 1 ameliorates hepatic steatosis in diet-induced obese mice: Role of PPARgamma. Biochim Biophys Acta (2016) 1861:138–47. doi: 10.1016/j.bbalip.2015.11.006
82. Turpin SM, Nicholls HT, Willmes DM, Mourier A, Brodesser S, Wunderlich CM, et al. Obesity-induced CerS6-dependent C16:0 ceramide production promotes weight gain and glucose intolerance. Cell Metab (2014) 20:678–86. doi: 10.1016/j.cmet.2014.08.002
83. Raichur S, Wang ST, Chan PW, Li Y, Ching J, Chaurasia B, et al. CerS2 Haploinsufficiency Inhibits beta-Oxidation and Confers Susceptibility to Diet-Induced Steatohepatitis and Insulin Resistance. Cell Metab (2014) 20:919. doi: 10.1016/j.cmet.2014.10.007
84. Montgomery MK, Brown SH, Lim XY, Fiveash CE, Osborne B, Bentley NL, et al. Regulation of glucose homeostasis and insulin action by ceramide acyl-chain length: A beneficial role for very long-chain sphingolipid species. Biochim Biophys Acta (2016) 1861:1828–39. doi: 10.1016/j.bbalip.2016.08.016
85. Baudhuin LM, Jiang Y, Zaslavsky A, Ishii I, Chun J, Xu Y. S1P3-mediated Akt activation and cross-talk with platelet-derived growth factor receptor (PDGFR). FASEB J (2004) 18:341–3. doi: 10.1096/fj.03-0302fje
86. Baudhuin LM, Cristina KL, Lu J, Xu Y. Akt activation induced by lysophosphatidic acid and sphingosine-1-phosphate requires both mitogen-activated protein kinase kinase and p38 mitogen-activated protein kinase and is cell-line specific. Mol Pharmacol (2002) 62:660–71. doi: 10.1124/mol.62.3.660
87. Ma MM, Chen JL, Wang GG, Wang H, Lu Y, Li JF, et al. Sphingosine kinase 1 participates in insulin signalling and regulates glucose metabolism and homeostasis in KK/Ay diabetic mice. Diabetologia (2007) 50:891–900. doi: 10.1007/s00125-006-0589-5
88. Osawa Y, Seki E, Kodama Y, Suetsugu A, Miura K, Adachi M, et al. Acid sphingomyelinase regulates glucose and lipid metabolism in hepatocytes through AKT activation and AMP-activated protein kinase suppression. FASEB J (2011) 25:1133–44. doi: 10.1096/fj.10-168351
89. Wang J, Badeanlou L, Bielawski J, Ciaraldi TP, Samad F. Sphingosine kinase 1 regulates adipose proinflammatory responses and insulin resistance. Am J Physiol Endocrinol Metab (2014) 306:E756–68. doi: 10.1152/ajpendo.00549.2013
90. Fayyaz S, Henkel J, Japtok L, Kramer S, Damm G, Seehofer D, et al. Involvement of sphingosine 1-phosphate in palmitate-induced insulin resistance of hepatocytes via the S1P2 receptor subtype. Diabetologia (2014) 57:373–82. doi: 10.1007/s00125-013-3123-6
91. Yu J, Dong J, Chen K, Ding Y, Yang Z, Lan T. Generation of mice with hepatocyte-specific conditional deletion of sphingosine kinase 1. Transgenic Res (2020) 29:419–28. doi: 10.1007/s11248-020-00211-0
92. Kwong EK, Liu R, Zhao D, Li X, Zhu W, Wang X, et al. The role of sphingosine kinase 2 in alcoholic liver disease. Dig Liver Dis (2019) 51:1154–63. doi: 10.1016/j.dld.2019.03.020
93. Nagahashi M, Takabe K, Liu R, Peng K, Wang X, Wang Y, et al. Conjugated bile acid-activated S1P receptor 2 is a key regulator of sphingosine kinase 2 and hepatic gene expression. Hepatology (2015) 61:1216–26. doi: 10.1002/hep.27592
94. Yuan J, Qiao J, Mu B, Lin L, Qiao L, Yan L, et al. Inhibition of SphK2 Stimulated Hepatic Gluconeogenesis Associated with Dephosphorylation and Deacetylation of STAT3. Arch Med Res (2018) 49:335–41. doi: 10.1016/j.arcmed.2018.11.001
95. Lee SY, Hong IK, Kim BR, Shim SM, Sung Lee J, Lee HY, et al. Activation of sphingosine kinase 2 by endoplasmic reticulum stress ameliorates hepatic steatosis and insulin resistance in mice. Hepatology (2015) 62:135–46. doi: 10.1002/hep.27804
96. Woodcock JM, Ma Y, Coolen C, Pham D, Jones C, Lopez AF, et al. Sphingosine and FTY720 directly bind pro-survival 14-3-3 proteins to regulate their function. Cell Signal (2010) 22:1291–9. doi: 10.1016/j.cellsig.2010.04.004
97. Powell DW, Rane MJ, Chen Q, Singh S, McLeish KR. Identification of 14-3-3zeta as a protein kinase B/Akt substrate. J Biol Chem (2002) 277:21639–42. doi: 10.1074/jbc.M203167200
98. Landa-Galvan HV, Rios-Castro E, Romero-Garcia T, Rueda A, Olivares-Reyes JA. Metabolic syndrome diminishes insulin-induced Akt activation and causes a redistribution of Akt-interacting proteins in cardiomyocytes. PloS One (2020) 15:e0228115. doi: 10.1371/journal.pone.0228115
99. Petersen KF, Shulman GI. Pathogenesis of skeletal muscle insulin resistance in type 2 diabetes mellitus. Am J Cardiol (2002) 90:11G–8G. doi: 10.1016/s0002-9149(02)02554-7
100. DeFronzo RA, Tripathy D. Skeletal muscle insulin resistance is the primary defect in type 2 diabetes. Diabetes Care (2009) 32 Suppl 2:S157–63. doi: 10.2337/dc09-S302
101. Shulman GI, Rothman DL, Jue T, Stein P, DeFronzo RA, Shulman RG. Quantitation of muscle glycogen synthesis in normal subjects and subjects with non-insulin-dependent diabetes by 13C nuclear magnetic resonance spectroscopy. N Engl J Med (1990) 322:223–8. doi: 10.1056/NEJM199001253220403
102. Petersen MC, Shulman GI. Mechanisms of Insulin Action and Insulin Resistance. Physiol Rev (2018) 98:2133–223. doi: 10.1152/physrev.00063.2017
103. Bell KS, Schmitz-Peiffer C, Lim-Fraser M, Biden TJ, Cooney GJ, Kraegen EW. Acute reversal of lipid-induced muscle insulin resistance is associated with rapid alteration in PKC-theta localization. Am J Physiol Endocrinol Metab (2000) 279:E1196–201. doi: 10.1152/ajpendo.2000.279.5.E1196
104. Griffin ME, Marcucci MJ, Cline GW, Bell K, Barucci N, Lee D, et al. Free fatty acid-induced insulin resistance is associated with activation of protein kinase C theta and alterations in the insulin signaling cascade. Diabetes (1999) 48:1270–4. doi: 10.2337/diabetes.48.6.1270
105. Yu C, Chen Y, Cline GW, Zhang D, Zong H, Wang Y, et al. Mechanism by which fatty acids inhibit insulin activation of insulin receptor substrate-1 (IRS-1)-associated phosphatidylinositol 3-kinase activity in muscle. J Biol Chem (2002) 277:50230–6. doi: 10.1074/jbc.M200958200
106. Hu W, Bielawski J, Samad F, Merrill AH Jr., Cowart LA. Palmitate increases sphingosine-1-phosphate in C2C12 myotubes via upregulation of sphingosine kinase message and activity. J Lipid Res (2009) 50:1852–62. doi: 10.1194/jlr.M800635-JLR200
107. Rapizzi E, Taddei ML, Fiaschi T, Donati C, Bruni P, Chiarugi P. Sphingosine 1-phosphate increases glucose uptake through trans-activation of insulin receptor. Cell Mol Life Sci (2009) 66:3207–18. doi: 10.1007/s00018-009-0106-3
108. Ross JS, Hu W, Rosen B, Snider AJ, Obeid LM, Cowart LA. Sphingosine kinase 1 is regulated by peroxisome proliferator-activated receptor alpha in response to free fatty acids and is essential for skeletal muscle interleukin-6 production and signaling in diet-induced obesity. J Biol Chem (2013) 288:22193–206. doi: 10.1074/jbc.M113.477786
109. Bruce CR, Risis S, Babb JR, Yang C, Lee-Young RS, Henstridge DC, et al. The sphingosine-1-phosphate analog FTY720 reduces muscle ceramide content and improves glucose tolerance in high fat-fed male mice. Endocrinology (2013) 154:65–76. doi: 10.1210/en.2012-1847
110. Hogenauer K, Billich A, Pally C, Streiff M, Wagner T, Welzenbach K, et al. Phosphorylation by sphingosine kinase 2 is essential for in vivo potency of FTY720 analogues. ChemMedChem (2008) 3:1027–9. doi: 10.1002/cmdc.200800037
111. Pchejetski D, Bohler T, Brizuela L, Sauer L, Doumerc N, Golzio M, et al. FTY720 (fingolimod) sensitizes prostate cancer cells to radiotherapy by inhibition of sphingosine kinase-1. Cancer Res (2010) 70:8651–61. doi: 10.1158/0008-5472.CAN-10-1388
112. Berdyshev EV, Gorshkova I, Skobeleva A, Bittman R, Lu X, Dudek SM, et al. FTY720 inhibits ceramide synthases and up-regulates dihydrosphingosine 1-phosphate formation in human lung endothelial cells. J Biol Chem (2009) 284:5467–77. doi: 10.1074/jbc.M805186200
113. Li MH, Hla T, Ferrer F. FTY720 inhibits tumor growth and enhances the tumor-suppressive effect of topotecan in neuroblastoma by interfering with the sphingolipid signaling pathway. Pediatr Blood Cancer (2013) 60:1418–23. doi: 10.1002/pbc.24564
114. Muir LA, Neeley CK, Meyer KA, Baker NA, Brosius AM, Washabaugh AR, et al. Adipose tissue fibrosis, hypertrophy, and hyperplasia: Correlations with diabetes in human obesity. Obesity (Silver Spring) (2016) 24:597–605. doi: 10.1002/oby.21377
115. Arner E, Westermark PO, Spalding KL, Britton T, Ryden M, Frisen J, et al. Adipocyte turnover: relevance to human adipose tissue morphology. Diabetes (2010) 59:105–9. doi: 10.2337/db09-0942
116. Patni N, Garg A. Congenital generalized lipodystrophies–new insights into metabolic dysfunction. Nat Rev Endocrinol (2015) 11:522–34. doi: 10.1038/nrendo.2015.123
117. Stern JH, Rutkowski JM, Scherer PE. Adiponectin, Leptin, and Fatty Acids in the Maintenance of Metabolic Homeostasis through Adipose Tissue Crosstalk. Cell Metab (2016) 23:770–84. doi: 10.1016/j.cmet.2016.04.011
118. Rabe K, Lehrke M, Parhofer KG, Broedl UC. Adipokines and insulin resistance. Mol Med (2008) 14:741–51. doi: 10.2119/2008-00058.Rabe
119. Zatterale F, Longo M, Naderi J, Raciti GA, Desiderio A, Miele C, et al. Chronic Adipose Tissue Inflammation Linking Obesity to Insulin Resistance and Type 2 Diabetes. Front Physiol (2019) 10:1607. doi: 10.3389/fphys.2019.01607
120. Anderson AK, Lambert JM, Montefusco DJ, Tran BN, Roddy P, Holland WL, et al. Depletion of adipocyte sphingosine kinase 1 leads to cell hypertrophy, impaired lipolysis, and nonalcoholic fatty liver disease. J Lipid Res (2020) 61:1328–40. doi: 10.1194/jlr.RA120000875
121. Gabriel TL, Mirzaian M, Hooibrink B, Ottenhoff R, van Roomen C, Aerts J, et al. Induction of Sphk1 activity in obese adipose tissue macrophages promotes survival. PloS One (2017) 12:e0182075. doi: 10.1371/journal.pone.0182075
122. Zhang W, Mottillo EP, Zhao J, Gartung A, VanHecke GC, Lee JF, et al. Adipocyte lipolysis-stimulated interleukin-6 production requires sphingosine kinase 1 activity. J Biol Chem (2014) 289:32178–85. doi: 10.1074/jbc.M114.601096
123. Ravichandran S, Finlin BS, Kern PA, Ozcan S. Sphk2(-/-) mice are protected from obesity and insulin resistance. Biochim Biophys Acta Mol Basis Dis (2019) 1865:570–6. doi: 10.1016/j.bbadis.2018.12.012
124. Fu Z, Gilbert ER, Liu D. Regulation of insulin synthesis and secretion and pancreatic Beta-cell dysfunction in diabetes. Curr Diabetes Rev (2013) 9:25–53. doi: 10.2174/157339913804143225
125. Oh YS, Bae GD, Baek DJ, Park EY, Jun HS. Fatty Acid-Induced Lipotoxicity in Pancreatic Beta-Cells During Development of Type 2 Diabetes. Front Endocrinol (Lausanne) (2018) 9:384. doi: 10.3389/fendo.2018.00384
126. Xia P, Qi Y. Cellular inhibitor of apoptosis protein-1 and survival of beta cells undergoing endoplasmic reticulum stress. Vitam Horm (2014) 95:269–98. doi: 10.1016/B978-0-12-800174-5.00011-9
127. Cantrell Stanford J, Morris AJ, Sunkara M, Popa GJ, Larson KL, Ozcan S. Sphingosine 1-phosphate (S1P) regulates glucose-stimulated insulin secretion in pancreatic beta cells. J Biol Chem (2012) 287:13457–64. doi: 10.1074/jbc.M111.268185
128. Hasan NM, Longacre MJ, Stoker SW, Kendrick MA, Druckenbrod NR, Laychock SG, et al. Sphingosine kinase 1 knockdown reduces insulin synthesis and secretion in a rat insulinoma cell line. Arch Biochem Biophys (2012) 518:23–30. doi: 10.1016/j.abb.2011.11.016
129. Veret J, Coant N, Gorshkova IA, Giussani P, Fradet M, Riccitelli E, et al. Role of palmitate-induced sphingoid base-1-phosphate biosynthesis in INS-1 beta-cell survival. Biochim Biophys Acta (2013) 1831:251–62. doi: 10.1016/j.bbalip.2012.10.003
130. Boslem E, MacIntosh G, Preston AM, Bartley C, Busch AK, Fuller M, et al. A lipidomic screen of palmitate-treated MIN6 beta-cells links sphingolipid metabolites with endoplasmic reticulum (ER) stress and impaired protein trafficking. Biochem J (2011) 435:267–76. doi: 10.1042/BJ20101867
Keywords: insulin resistance, β-cell, sphingolipid, sphingosine 1-phosphate, ceramide
Citation: Qi Y, Wang W, Song Z, Aji G, Liu XT and Xia P (2021) Role of Sphingosine Kinase in Type 2 Diabetes Mellitus. Front. Endocrinol. 11:627076. doi: 10.3389/fendo.2020.627076
Received: 08 November 2020; Accepted: 21 December 2020;
Published: 09 February 2021.
Edited by:
Eric Hajduch, Institut National de la Santé et de la Recherche Médicale (INSERM), FranceReviewed by:
Wilfried Le Goff, Institut National de la Santé et de la Recherche Médicale (INSERM), FranceCopyright © 2021 Qi, Wang, Song, Aji, Liu and Xia. This is an open-access article distributed under the terms of the Creative Commons Attribution License (CC BY). The use, distribution or reproduction in other forums is permitted, provided the original author(s) and the copyright owner(s) are credited and that the original publication in this journal is cited, in accordance with accepted academic practice. No use, distribution or reproduction is permitted which does not comply with these terms.
*Correspondence: Yanfei Qi, ai5xaUBjZW50ZW5hcnkub3JnLmF1; Pu Xia, eGlhLnB1QHpzLWhvc3BpdGFsLnNoLmNu
Disclaimer: All claims expressed in this article are solely those of the authors and do not necessarily represent those of their affiliated organizations, or those of the publisher, the editors and the reviewers. Any product that may be evaluated in this article or claim that may be made by its manufacturer is not guaranteed or endorsed by the publisher.
Research integrity at Frontiers
Learn more about the work of our research integrity team to safeguard the quality of each article we publish.