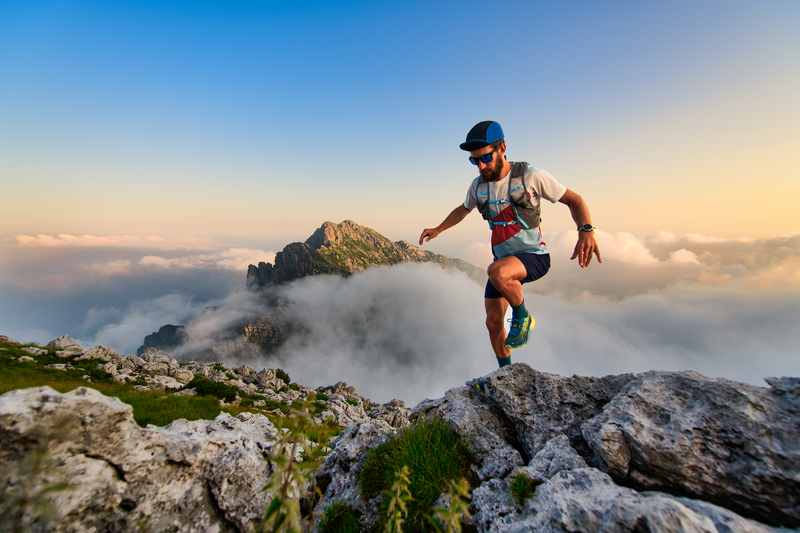
95% of researchers rate our articles as excellent or good
Learn more about the work of our research integrity team to safeguard the quality of each article we publish.
Find out more
REVIEW article
Front. Endocrinol. , 18 January 2021
Sec. Diabetes: Molecular Mechanisms
Volume 11 - 2020 | https://doi.org/10.3389/fendo.2020.598012
This article is part of the Research Topic Erasing Metabolic Memory in Vascular Complications of Diabetes Mellitus: Epigenetic Mechanisms and Interventions View all 4 articles
Diabetes mellitus (DM) has been one of the largest health concerns of the 21st century due to the serious complications associated with the disease. Therefore, it is essential to investigate the pathogenesis of DM and develop novel strategies to reduce the burden of diabetic complications. Sirtuin 1 (SIRT1), a nicotinamide adenosine dinucleotide (NAD+)-dependent deacetylase, has been reported to not only deacetylate histones to modulate chromatin function but also deacetylate numerous transcription factors to regulate the expression of target genes, both positively and negatively. SIRT1 also plays a crucial role in regulating histone and DNA methylation through the recruitment of other nuclear enzymes to the chromatin. Furthermore, SIRT1 has been verified as a direct target of many microRNAs (miRNAs). Recently, numerous studies have explored the key roles of SIRT1 and other related epigenetic mechanisms in diabetic complications. Thus, this review aims to present a summary of the rapidly growing field of epigenetic regulatory mechanisms, as well as the epigenetic influence of SIRT1 on the development and progression of diabetic complications, including cardiomyopathy, nephropathy, and retinopathy.
Diabetes mellitus (DM) refers to abnormalities in the metabolic processing of carbohydrates, fats and proteins that is characterized by persistent hyperglycemia as a result of insufficient insulin secretion, impaired insulin action or both (1). DM has gradually become one of the largest health concerns of the twenty-first century, and affects approximately 451 million adults worldwide, with projections that the disease will reach 693 million adults by 2045 (2). The majority of DM-related rates of morbidity and mortality are due to serious complications resulting from chronic hyperglycemia, including cardiovascular disorders, nephropathy, retinopathy with potential loss of vision, peripheral neuropathy and autonomic neuropathy (3). These long-term complications make DM a considerable burden to public health since they require lifelong care and treatment. Therefore, it is essential to investigate the disease pathogenesis and develop novel therapeutic strategies to reduce the burden of DM and its associated complications.
DM is a hereditary disease with a strong genetic predisposition (4). Interestingly, in addition to genetics, environmental factors including less exposure to sunlight and lack of physical activity also increase the risk of DM (5). However, numerous people exposed to these risks do not go on to develop DM. Indeed, strong evidence has shown that epigenetics plays a significant role in the complex interaction between genes and the environment (6). Epigenetics is described as stable and heritable alterations in gene expression or cellular phenotypes without changes in nucleotide sequences, and includes DNA methylation, histone modifications and non-coding RNA (ncRNA)-mediated pathways (7). Recently, a growing number of studies have focused on the role of epigenetics in DM and its related complications.
Sirtuin 1 (SIRT1), a nicotinamide adenosine dinucleotide (NAD+)-dependent deacetylase, has been reported to deacetylate histones to modulate chromatin function, such as histone 3 lysine 9 (H3K9) and H4K16 (8). SIRT1 can also deacetylate numerous transcription factors to regulate the expression of target genes either positively or negatively, including tumor protein 53 (p53) (9), forkhead box O (FOXO) (10), nuclear factor kappa B (NF-κB) (11), and peroxisome proliferator-activated receptor gamma coactivator-1 alpha (PGC-1α) (12). Moreover, SIRT1 plays a crucial role in regulating histone and DNA methylation through the recruitment of other nuclear enzymes to the chromatin (8, 13). All above mentioned findings suggest that SIRT1 is a crucial regulator of epigenetics. Furthermore, SIRT1 acts as a cellular energy sensor and plays a pivotal role in regulating energy metabolism, insulin sensitivity, and cardiovascular functions in mammals (14–16). Recently, numerous studies have concentrated on the key roles of SIRT1 and related epigenetic mechanisms in diabetic complications. Therefore, the primary purpose of this review is to summarize the recent advances regarding the effects and related epigenetic mechanisms of SIRT1 in diabetic complications, including diabetic cardiomyopathy (DCM), diabetic nephropathy (DN), and diabetic retinopathy (DR). Finally, we will list the SIRT1 agonists that have been reported to exert protective functions during the development of DM-associated complications.
DCM is one of the major diabetic complications characterized by structural abnormalities and ventricular systolic and/or diastolic dysfunction in the absence of hypertension, coronary artery disease or significant congenital cardiac diseases (17). Accumulating evidence has revealed an association between “hyperglycemic memory” and epigenetic mechanisms (18), which may exert significant roles in the development of DCM. Hyperglycemic memory, also known as metabolic memory, is a phenomenon where the negative effects of hyperglycemia persist even after glycemic control has been achieved. Yu et al. explored the effect of H3K9 trimethylation (H3K9me3), a key epigenetic chromatin marker, on high glucose (HG)-induced inflammation and metabolic memory in rat cardiomyocyte cell line H9c2. They found that HG conditions increased the levels of the inflammatory cytokine interleukin-6 (IL-6) and decreased the levels of H3K9me3 at the IL-6 promoter, which could not be reversed following the removal of HG stimulation. These results indicated that decreased levels of H3K9me3 at the IL-6 promoter following HG exposure was a main mechanism that affected hyperglycemic memory in cardiomyocytes (19).
Moreover, histone deacetylase (HDAC)-mediated epigenetic processes also have important functions in the modulation of DCM. Chen et al. investigated the role of HDAC in DCM and found HDAC inhibition could attenuate cardiac hypertrophy, interstitial fibrosis and apoptosis accompanied by increased acetylation of glucose transporter 1 (GLUT1) and phosphorylation of p38 in diabetic mice (20). p38 phosphorylation has been verified to be involved in cardioprotection induced by glucagon-like peptide-1 in myocardial ischemia and reperfusion injury (21). However, whether GLUT1 acetylation mediates its physiological functions needs to be further explored. Peroxisome proliferator-activated receptors (PPARs) have emerged as critical regulators of cardiac glucose and lipid homeostasis. HDAC inhibition in a rat model of DM increased the expression of cardiac PPAR-α and PPAR-δ but decreased cardiac PPAR-γ expression compared with untreated DM rats, suggesting that HDAC inhibition could regulate fatty acid metabolism to improve DCM (22). Previous studies have only focused on the total inhibition of HDAC activity using a non-specific HDAC inhibitor. Recently, Xu et al. explored the effect of specific HDAC inhibitor on DCM and found that HDAC3 inhibition by RGFP966 could protect against DM-induced cardiac remodeling and dysfunction in diabetic mice. Furthermore, RGFP966 could repress extracellular signal-regulated kinases 1/2 (ERK1/2), a well-known initiator of cardiac hypertrophy. This effect was mediated by increased dual specificity phosphatase 5 (DUSP5) expression through the acetylation of histone H3 on the primer region of the DUSP5 gene, a nuclear phosphatase of ERK1/2 (23). Contrary to the above, silent information regulator 2 (Sir2), an NAD+-dependent HDAC, has been reported to have a beneficial effect on DCM. A study by Dong et al. demonstrated that fidarestat, an aldose reductase inhibitor, could ameliorate cardiomyocyte dysfunction in diabetic obese mouse cardiomyocytes through regulation of Sir2 expression (24).
Emerging evidence has shown that microRNAs (miRNAs) are implicated in the maintenance of tissue homeostasis in the heart. A study on the alteration of miRNAs expression in a mouse model of DM revealed that 316 out of 1,008 total miRNAs were disordered in the diabetic heart (25). Of note, microRNA-212 (miR-212) and miR-221 were massively overexpressed in the diabetic heart, which could play pivotal roles in cardiac hypertrophy and autophagy, and glycemic control was unable to restore the levels of these two miRNAs to normal (25–27). Similarly, the expression of miR-199a has been reported to be upregulated in association with myocardial hypertrophy, whereas miR-30a and miR-1 (potent inhibitors of mitochondrial fission, hypertrophy and apoptosis), as well as miR-29b (anti-fibrosis) were downregulated in diabetic mouse heart (25). Moreover, the expression of miR-451, miR-144, and miR-133a was also shown to be altered in diabetic murine hearts, and involved in the development of DCM (28–30). Exploring the epigenetic mechanisms of miR-133a found that it could regulate DNA methylation in diabetic mouse cardiomyocytes (30).
Long ncRNAs (lncRNAs), another type of ncRNA, have also been considered as a novel therapeutic strategy for the treatment of DCM. Silencing lncRNA Kcnq1ot1 decreased caspase-1 expression and repressed transforming growth factor-beta 1 (TGF-β1) signaling to improve pyroptosis and fibrosis in a mouse model of DCM (31). Moreover, downregulation of lncRNA metastasis-associated lung adenocarcinoma transcript 1 (MALAT1) and myocardial infarction associated transcript (MIAT) improved DCM by reducing apoptosis and ameliorating cardiac function (32, 33). In contrast, downregulation of lncRNA H19 by small interfering RNA (siRNA) decreased miR-675 expression and in turn upregulated the expression of voltage-dependent anion channel 1, which consequently promoted cellular apoptosis in neonatal rat cardiomyocytes exposed to HG conditions (34). The altered expression levels of the ncRNAs discussed above might explain why DM-related myocardial injury still progressively worsens even in the presence of glycemic control.
In addition to histone post-translational modifications and ncRNA-mediated pathways, the effects of DNA methylation on the development of DCM have also been reported. Mönkemann et al. investigated the epigenetic alteration of p21WAF1/CIP1 and cyclin D1 gene expression involved in cell cycle control in cardiomyocytes of streptozocin (STZ)-induced diabetic rats. They found that the p53-inducible p21WAF1/CIP1 gene was completely demethylated and activated, while the cyclin D1 gene was completely methylated and inactivated. These different methylation patterns might be the result of p53-dependent cell cycle arrest associated with DNA damage. Activated p21WAF1/CIP1 suppresses DNA replication by binding proliferating cell nuclear antigen, which is necessary to both replicative DNA synthesis and DNA repair (35). As discussed above, epigenetic changes are closely related to the development of DCM, and a complete understanding of the roles of epigenetic processes in DCM would provide novel insights into this complex disease.
Several studies have shown the possibility that SIRT1, as a class-III HDAC, exerts a protective effect on DCM by deacetylating histones. NF-κB, a pleiotropic transcription factor, can be translocated to nucleus to induce transcription of several proinflammatory genes under oxidative stress. In diabetic rats, SIRT1 activation by resveratrol could reduce the acetylation of the p65 subunit of NF-κB (NF-κB-p65) at K310. NF-κB-dependent transcription is associated with acetylation status of histone. Indeed, increased acetylation of H3K9 was observed in the hearts of diabetic rats, which was reduced after resveratrol administration (11). Based on the above findings, we propose that increased SIRT1 expression may be responsible for the deacetylation of NF-κB-p65 and H3K9, as well as the reduced NF-κB-p65-mediated actions in the diabetic heart. In addition, p66Shc expression was upregulated in the diabetic heart, which was related to oxidative stress, myocardial inflammation, and cardiac dysfunction. Importantly, three-week intensive glycemic control was unable to revert the above-mentioned phenomenon. This study focused on the epigenetic regulation of the prooxidant adaptor p66Shc in the hearts of diabetic mice and found that dysregulation of DNA methyltransferase 3b (Dnmt3b) and deacetylase SIRT1 resulted in CpG demethylation and histone H3 acetylation at the p66Shc promoter, which could lead to sustained transcription of the p66Shc (36).
Other than histone deacetylation, it has been widely reported that SIRT1 is able to deacetylate a wide range of non-histone substrates to result in activation or repression of their catalytic activity in DCM. PGC-1α, as a master regulator of mitochondrial biogenesis, can be acetylated by HG treatment. However, resveratrol decreased the acetylation of PGC-1α in HG-treated H9c2 cells. Additional studies investigating the underlying mechanisms found that the resveratrol-induced PGC-1α deacetylation depended on SIRT1. Meanwhile, resveratrol improved mitochondrial function to alleviate DCM by increasing SIRT1 expression and PGC-1α deacetylation (12). Another study by Ding et al. revealed that PGC-1α can directly bind to the dynamin-related protein 1 (Drp1) promoter to modulate protein expression. Melatonin reduces Drp1-mediated mitochondrial fission to exert its cardioprotective role in DCM through the SIRT1/PGC-1α signaling pathway (37).
Activation of poly (ADP-ribose) polymerase 1 (PARP1) is dependent on NAD+ and plays an important role in DNA repair and chromatin remodeling. In addition, PARP1 is also involved in transcriptional regulation, telomere cohesion, and mitotic spindle formation during cell division, intracellular transport and energy metabolism (38). In a mouse model of type 2 diabetes (T2D), the mice exhibited increased oxidative stress, inflammation, cardiac hypertrophy, and fibrosis that was associated with enhanced PARP1 activity and decreased SIRT1 expression, while PARP1 inhibition could increase the levels of SIRT1 and PGC-1α to improve the above-mentioned adverse effects (39). 1,25-Dihydroxyvitamin D3 (1,25(OH)2D3) has been reported to be a potential PARP1 inhibitor. Treatment with 1,25(OH)2D3 can inhibit PARP1 expression to increase the expression of SIRT1 and repress the phosphorylation of mammalian target of rapamycin (mTOR), which improves cardiac dysfunction, hypertrophy and fibrosis in rats of type 1 diabetes (T1D) (40).
Autophagic dysfunction is a common occurrence in DM. Wang et al. investigated the mechanisms underlying the protective effect of resveratrol against heart failure and explored the role of SIRT1 in the modulation of autophagic flux in diabetic mice. They found that SIRT1 activation by resveratrol could deacetylate FOXO1 and enhance FOXO1 DNA binding at the Ras-related protein Rab-7 (Rab7, a crucial factor in the maturation of autophagosomes and their fusion with lysosomes) promoter region to ameliorate dysfunctional autophagic flux in the hearts of diabetic mice, suggesting that the effect of the SIRT1/FOXO1/Rab7 axis on autophagic flux may be a therapeutic strategy for the treatment of DCM (10).
It is commonly believed that histone deacetylation is tightly linked to DNA methylation. For example, the methyl-CpG-binding protein MeCP2 interacts specifically with methylated DNA and mediates transcriptional repression. Additionally, it was discovered that this repression by MeCP2 required a HDAC complex (41). Peng et al. reported that SIRT1 could deacetylate Dnmt1 to change its activity in vitro and in vivo. Specifically, deacetylation of Lys1349 and Lys1415 in the catalytic domain of Dnmt1 increases its methyltransferase activity. However, deacetylation of lysine residues in repeating glycine-lysine dipeptides (the GK linker) decreases Dnmt1’s methyltransferase-independent transcriptional repression (42). Recently, a study regarding the gestational DM-induced fetal programming of a heart ischemia-sensitive phenotype later in life found that cardiac oxidative stress and DNA hypermethylation in the offspring induced by gestational DM leaded to decreased expression of SIRT1 and aberrant development of the heart ischemia-sensitive phenotype (43). Therefore, it is easy to speculate that the crosstalk between the deacetylation of SIRT1 and DNA methylation may have an important role in DCM, and the mechanisms need to be further investigated.
Furthermore, SIRT1 is a direct target of many miRNAs. Suppression of miR-34a has been shown to restore the expression of SIRT1 to reverse the adverse epigenetic characteristics at p66Shc promoter in human cardiomyocytes exposed to HG conditions (36). Zheng et al. showed that the expression of miR-195 was upregulated while SIRT1 was downregulated in STZ-induced diabetic mouse heart. However, silencing of miR-195 expression inhibited oxidative stress, alleviated myocardial hypertrophy and improved cardiac function in diabetic mice, while at the same time the cardiac levels of B-cell lymphoma 2 (Bcl-2) and SIRT1 were upregulated (44). Therefore, it is possible that the beneficial effect of reduced miR-195 expression on DCM may be due to the upregulated expression of SIRT1. However, various miRNAs will have different effects on SIRT1, and overexpression of miR-22 upregulates SIRT1 to attenuate oxidative stress injury in a mouse model of DCM (45). LncRNA HOX transcript antisense RNA (HOTAIR), as a competing endogenous RNA, upregulates SIRT1 by sponging miR‐34a to improve DCM (46). Altogether, SIRT1 as a deacetylase plays a necessary role in the regulation of the epigenetics in DCM (Figure 1), and in-depth research will provide more information regarding potential therapeutic strategies to improve DCM in the future.
Figure 1 The mechanisms of action and signaling pathways of SIRT1 in diabetic cardiomyopathy. Diabetes mellitus (DM) can inhibit SIRT1 expression by increased expression of PARP1, miR-34a, and miR-195, or by decreased expression of miR-22. SIRT1 can deacetylate NF-κB and H3K9 to inhibit the expression of NF-κB-dependent inflammatory genes, which improves DCM. Dysregulation of Dnmt3b and SIRT1 by DM leads to CpG demethylation and histone H3 acetylation at the p66Shc promoter, which can result in sustained transcription of p66Shc to cause cardiac oxidative stress and inflammation. SIRT1 can reduce Drp1-mediated mitochondrial fission to exert its cardioprotective role in DCM through increasing PGC-1α expression. 1,25(OH)2D3 treatment can inhibit PARP1 expression to increase the expression of SIRT1 and repress the phosphorylation of mTOR, thus improving DM-induced cardiac hypertrophy and fibrosis. And SIRT1 can deacetylate FOXO1 and enhance FOXO1 DNA binding at the Rab7 promoter region to ameliorate dysfunctional autophagic flux in the hearts of diabetic mice. Finally, lncRNA HOTAIR can upregulate SIRT1 expression by sponging miR-34a to improve DCM. SIRT1, Sirtuin 1; DM, diabetes mellitus; PARP1, poly (ADP-ribose) polymerase 1; miR, microRNA; NF-κB, nuclear factor kappa B; Dnmt3b, DNA methyltransferase 3b; DCM, diabetic cardiomyopathy; Drp1, dynamin-related protein 1; PGC-1α, peroxisome proliferator-activated receptor gamma coactivator-1 alpha 1,25(OH)2D3, 1,25-Dihydroxyvitamin D3; mTOR, mammalian target of rapamycin; FOXO1, forkhead box O 1; lncRNA HOTAIR, long non-coding RNA HOX transcript antisense RNA.
DN is a common microvascular complication of DM characterized by tubular interstitial fibrosis, glomerular mesangial hypertrophy and expansion, glomerular hyperfiltration with microalbuminuria and podocyte foot process effacement. The pathogenesis of DN is still not fully understood, but it has become increasingly clear that epigenetic mechanisms are involved in its development. Podocyte cells have been reported to play a crucial role in maintaining the structure and function of the glomerular filtration barrier, which is related to the pathogenesis of DN. Zhang et al. explored the effect of DNA methylation in murine diabetic podocytes and indicated that the expression of Dnmt1, nuclear factor Sp1 and NF-κB-p65 was markedly upregulated in podocytes under diabetic conditions, while inhibition of DNA methylation attenuated albuminuria, glomerular hypertrophy, mesangial matrix expansion and podocyte injury. Further mechanistic research found that increased Sp1 could bind to the Dnmt1 promoter region and interact with NF-κB-p65 in the nucleus of HG-treated podocytes to participate in the regulation of Dnmt1. Thus, the Sp1/NF-κB-p65-Dnmt1 pathway may be a potential therapeutic target to protect against podocyte injury in DN (47). Recently, Hishikawa et al. found that lysine acetyltransferase 5 (KAT5)-mediated DNA damage repair associated with the DNA methylation status is essential for the maintenance of kidney podocytes. Podocyte-specific Kat5-knockout mice presented with severe albuminuria concomitant with increased DNA double-strand breaks and augmented DNA methylation in the nephrin promoter region (48). Aberrant DNA methylation, such as hypermethylation of kinesin family member 20B (Kif20b), claudin-18 (Cldn18), and solute carrier organic anion transporter family member 1a1 (Slco1a1), as well as hypomethylation of angiotensinogen (Agt), ATP-binding cassette sub-family C member 4 (Abcc4), cytochrome P450 4A10 (Cyp4a10), and Glut5 were also observed in the proximal tubules of diabetic mice, which could lead to continuous mRNA expression of select genes to alter the phenotype of the proximal tubules in DN (49).
Histone modifications should also be kept in mind when considering to therapeutically target epigenetics to improve DN. DM is an inflammatory disease that releases inflammatory cytokines to promote the aggregation of leukocytes by innate cells in the diabetic kidney, which facilitates progressive fibrosis in advanced stages of DN. One study concentrated on the effect of glucose on the expression and histone modifications of a proinflammatory gene, thioredoxin-interacting protein (TXNIP) and found that glucose could induce the expression of TXNIP to enhance the development of DN through histone acetylation (50). Importantly, DM is also an endothelial disease. Endothelial activation refers to the endothelial expression of cell surface adhesion molecules that promote leukocyte recruitment. Alghamdi et al. have shown that the phosphorylation of histone H3 on serine residue 10 plays an important role in mediating endothelial activation in DN (51). TGF-β can alter pivotal chromatin histone modifications at target gene promoters to modulate gene expression in mesangial cells. TGF-β has been shown to inhibit H3K27me3 to promote pathological gene-mediated glomerular mesangial dysfunction and DN through the dysregulation of associated histone modifying enzymes and miRNAs (52). The 12/15-lipoxygenase (12/15-LO) is implicated in TGF-β-associated signaling, histone modifications and lysine methyltransferase Set7 regulation in the progression of DN. Knockout of the 12/15-LO (Alox15) gene inhibited TGF-β-induced expression of Set7 (Setd7) gene and pro-fibrotic genes, in kidneys of STZ-induced diabetic mice (53).
Recently, accumulating evidence has highlighted key roles of lncRNAs in the pathophysiology of DN. For example, lncRNA nuclear-enriched abundant transcript 1 (NEAT1) increases proliferation and fibrosis in glucose-induced mouse mesangial cell model by activating the protein kinase B/mTOR signaling pathway (54). With an opposite effect, overexpression of lncRNA CYP4B1-PS1-001 suppressed proliferation and fibrosis of mesangial cells under HG conditions (55). Moreover, lncRNAs are also confirmed to be involved in the regulation of podocyte injury. LncRNA LINC01619, as a competing endogenous RNA, modulated endoplasmic reticulum (ER) stress and podocyte injury in DN via the miR-27a/FOXO1 pathway (56). Another lncRNA, MALAT1, an important oncogene in numerous cancers, was increased in the kidney of STZ-induced diabetic mice and was dysregulated in HG-treated podocytes. However, knocking it down under HG conditions reversed podocyte damage via the downregulation of serine/arginine-rich splicing factor 1 overexpression, a MALAT1-binding protein, and partial inhibition of β-catenin nuclear accumulation (57).
DR is another severe microvascular complication in patients with DM, and is the leading cause of blindness. Numerous studies have focused on the roles of epigenetic modifications in DR and it has been reported that epigenetic modifications could serve as possible biomarkers for DR (58). An increase in oxidative stress has been considered a significant factor contributing to the development of DR. Ras-related C3 botulinum toxin substrate 1 (Rac1)-mediated cytosolic ROS production and the subsequent oxidative stress play key roles in mitochondrial injury and capillary cell apoptosis, which are associated with the DNA methylation status of the Rac1 promoter (59). Moreover, a decrease in manganese superoxide dismutase (MnSOD), an antioxidant enzyme, has been observed in DR. Further investigation regarding the underlying epigenetic mechanisms discovered that hyperglycemia increased acetyl-H3K9, H4K20me3, and NF-kB-p65 at the promoter and enhancer regions of retinal Sod2, and upregulated protein and gene expression of SUV420h2, one of the primary enzymes for the trimethylation of H4K20. However, silencing SUV420h2 by its siRNA in retinal endothelial cells blocked HG-induced increase in H4K20me3 at the Sod2 enhancer and decrease in Sod2 transcripts (60). Another study showed that hyperglycemia increased the binding of the histone demethylase lysine-specific demethylase-1 (LSD1) and Sp1 at Sod2, and decreased monomethyl H3K4 and dimethyl H3K4. Knocking down LSD1 with siRNA improved the HG-induced H3K4 demethylation at Sod2 to upregulate Sod2 gene expression (61). These findings indicate that epigenetic modifications play key roles in the regulation of retinal Sod2 in the development of DR.
The activity and transcription of matrix metalloproteinase 9 (MMP-9) has also been observed to be increased in DR, which could damage retinal mitochondria and enhance oxidative stress. Additional epigenetic studies have demonstrated that glucose increased the binding of Dnmt1 and hydroxymethylase ten-eleven translocation 2 (Tet2) to the MMP-9 promoter region in retinal endothelial cells. While Dnmt1 adds a methyl group to the cytosine forming methyl cytosine, Tet2 hydroxymethylates that cytosine to form 5-hydroxymethyl cytosine, in turn, activates MMP-9 transcription. These changes were reversed with the MnSOD mimesis, MnTBAP, which regulated MMP-9 transcription and improved mitochondrial damage (62, 63). The MMP-9 promoter has also been reported to undergo histone modifications in DR. Hyperglycemia increased the levels of H3K27me3 and recruitment of enhancer of zeste homolog 2 (EZH2) at the MMP-9 promoter. EZH2 suppression could reduce recruitment of Dnmt1 and Tet2 at the same promoter region of MMP-9 to reduce its transcription and mitochondrial damage (64). Additionally, mitochondrial DNA (mtDNA) is injured with elevated base mismatches and hypermethylated cytosines (65). HG conditions reduced MutL homolog 1 (Mlh1) mitochondrial localization, an enzyme responsible for repairing the mismatched bases, and hypermethylated its promoter with increased Dnmt1 binding and decreased Sp1 binding. Inhibition of Dnmt1 could reduce hypermethylation of the Mlh1 promoter, elevate its gene transcripts and decrease mtDNA mismatches, suggesting that the regulation of DNA methylation has a potential role to prevent mtDNA damage and slow or inhibit the development of DR (66).
Recent research has revealed regulatory roles of lncRNAs on inflammation in DR. The lncRNA MALAT1 was upregulated in the vitreous humors from diabetic patients and could impact the expression of inflammatory cytokines via its correlation with components of the polycomb repressive complex 2. Moreover, increased MALAT1 and related inflammatory transcripts in human retinal endothelial cells (HRECs) were detected following inhibition of Dnmts. However, HG treatment could not induce significant methylation changes in CpG sites across the MALAT1 gene (67). Therefore, the mechanism of HG-induced overexpression of MALAT1 still remains to be investigated. In addition, HG conditions facilitated cell apoptosis and attenuated the cell activity concomitant with enhanced binding activity between NF-κB and the lncRNA MIAT. Further investigation revealed that MIAT could regulate miR-29b and subsequently regulate cell apoptosis in HG-treated rat retinal Müller cells (68). Due to the key role of epigenetics in diabetic microvascular complications, such as DN and DR, it is essential to explore how changes to the epigenome influence the etiology and pathogenesis of diabetic microvascular complications for the development of novel biomarkers and drug targets.
Hasegawa et al. found that SIRT1 levels in proximal tubules were decreased prior to the development of albuminuria in STZ-induced diabetic mice. Moreover, knockout of Sirt1 specifically in the proximal tubules worsened the DM-induced glomerular changes. Importantly, nondiabetic proximal tubule-specific Sirt1 knockout mice developed albuminuria, suggesting that Sirt1 expression in the proximal tubules plays a necessary role for glomerular function. The expression of the tight junction protein CLDN1 has been shown to activate β-catenin-Snail pathway to induce podocyte effacement and cause albuminuria. However, overexpression of SIRT1 was able to blunt HG-induced upregulation of CLDN1 via the deacetylation of histones H3 and H4 with subsequent CpG methylation of Cldn1 by recruiting Dnmt1 in human-derived renal epithelial cells (69). p66Shc, a biomarker for renal oxidative injury, is upregulated in DN. SIRT1 activation can inhibit p66Shc expression to improve DN-induced oxidative injury by facilitating the binding of SIRT1 to the p66Shc promoter and deacetylation of acetyl-H3 in human proximal tubular epithelial cell line (HK-2) under HG conditions (70). A recent study revealed that the repression of SIRT1 transcription by HG conditions in renal tubular epithelial cells was dependent on the epigenetic regulation of hypermethylated in cancer 1 (HIC1), which could increase the levels of ROS and contribute to the development of DN. Mechanistically, HIC1 repressed SIRT1 transcription in response to HG stimulation through an interaction with EZH2, an H3K27 trimethyltransferase, as well as Dnmt1 (71).
Moreover, interactions between SIRT1 and miRNAs have been reported to play an important role during DN therapy. One study that focused on the role of SIRT1 in HG-induced renal tubular epithelial injury showed that overexpression of SIRT1 decreased activity of NF-κB to upregulate miR-29 expression. NF-κB was demonstrated to downregulate miR-29 expression by directly binding to its promotor. Overexpression of miR-29 directly targeted Kelch-like ECH-associated protein 1 (Keap1) mRNA to decrease Keap1 expression and subsequently increase the expression of nuclear factor erythroid 2-related factor 2 (Nrf2) and downstream antioxidases, including glutathione S-transferase (GST) and nicotinamide adenine dinucleotide phosphate (NADPH) quinone dehydrogenase 1 (NQO1), which improved HG-induced renal tubular epithelial injury (72). In addition, HG conditions can elevate the levels of miR-34a-5p to exacerbate fibrosis by targeting SIRT1 in HK-2 cells (73). Overexpression of long intergenic ncRNA (lincRNA) 1700020I14Rik has been verified to interact with miR-34a-5p through direct targeting as well as an argonaute-2 dependent manner to inhibit cell proliferation and fibrosis through the SIRT1/hypoxia-inducible factor-1α (HIF-1α) signaling pathway during the progression of DN (74). Recently, Ge et al. found that lncRNA growth arrest special 5 (GAS5) could also upregulate SIRT1 expression to inhibit cell proliferation and fibrosis in DN by acting as an miR-221 sponge (75). Furthermore, inhibition of miR-133b and miR-199b upregulated SIRT1, which in turn attenuated TGF-β1-induced endothelial to mesenchymal transition and renal fibrosis in DN (76). Another lncRNA, SOX2-overlapping transcript (SOX2OT), was significantly downregulated in HG-treated human podocyte cells (HPCs). The overexpression of SOX2OT markedly improved the HG-induced HPC injury and increased the expression of Beclin-1 and the microtubule-associated proteins 1A/1B light chain 3-II (LC3-II) to LC3-I ratio, whereas decreased the levels of p62 by sponging miR-9 to facilitate SIRT1 expression (77). miR-155-5p also has been reported to regulate autophagy, which might be upregulated in patients with DN. Under HG conditions, inhibition of miR-155-5p could stimulate SIRT1 expression to promote autophagy by reducing binding to the SIRT1 3’ untranslated region in HK-2 cells (78).
In addition, SIRT1 can regulate oxidative stress, inflammation, apoptosis and fibrosis to improve DN by mediating the expression of several downstream targets. Excessive mitochondrial ROS production is considered an initiating event in the development of DN. Zhang et al. demonstrated that SIRT1 activation by resveratrol could inhibit mitochondrial ROS production, improve respiratory chain complex I and III activity and elevate the mitochondrial membrane potential in podocytes exposed to HG conditions, which was related to upregulation of PGC-1α expression (79). Consistent with this study, salidroside (broad spectrum bioactive effects) improved DN-induced renal structure damage probably by stimulating SIRT1/PGC-1α-mediated mitochondrial biogenesis (80). NADPH oxidase 4 (NOX4), a main enzyme contributing to increased oxidative stress, is upregulated in HG conditions. Treatment with puerarin (naturally occurring isoflavonoid) suppressed NOX4 expression to exert its anti-oxidative effect in HG-treated podocytes, which was dependent on SIRT1 expression (81).
Furthermore, inhibition of inflammation is one of the important mechanisms for SIRT1 to protect the kidney from injury. Du et al. found that Tangshen formula (traditional Chinese herbal medicine for treatment of kidney disease) activated SIRT1 to reduce the expression of the proinflammatory factors NF-κB and monocyte chemoattractant protein-1, which improved the severity of DN (82). NF-κB also has been shown to regulate autophagy, which plays a crucial role in several kidney diseases including DN. Hyperglycemia downregulates the levels of Beclin 1 and LC3-II to induce renal dysfunction, which can be reversed by SIRT1-mediated deacetylation of NF-κB-p65 (83). The SIRT1-FOXO1 autophagy signal axis also plays a key role in the regulation of autophagy in DN. Xu et al. reported that metformin (used to treat T2D) upregulated the levels of autophagy to alleviate oxidative stress in renal tissue, and reduced pathological and structural changes of glomeruli through the deacetylation and activation of FOXO1 by SIRT1 (84). Furthermore, SIRT1 plays an important anti-apoptotic role in the treatment of DN. Wang et al. reported that SIRT1 activation by resveratrol could deacetylate p53 to improve the renal tubular injury induced by hyperglycemia through the inhibition of apoptosis (9). The summarizing schematic diagram about the diverse mechanisms and pathways of SIRT1 in DN is presented in Figure 2.
Figure 2 The mechanisms of action and signaling pathways of SIRT1 in diabetic nephropathy. Diabetes mellitus (DM) can inhibit SIRT1 expression by increased expression of miR-34a-5p, miR-221, miR-9, miR-133b, miR-155-5p, and miR-199b. HIC1 can repress SIRT1 transcription in response to HG stimulation via an interaction with EZH2 and Dnmt1. Overexpression of SIRT1 can blunt CLDN1/β-catenin-Snail-mediated albuminuria in DN via the deacetylation of histones H3 and H4 with subsequent CpG methylation of Cldn1 by recruiting Dnmt1. SIRT1 activation can inhibit p66Shc expression to improve oxidative stress in DN by deacetylation of acetyl-H3. SIRT1 activation can upregulate miR-29 expression by reduction of NF-κB binding to its promotor. Overexpression of miR-29 directly targets Keap1 mRNA to decrease Keap1 expression and subsequently increase Nrf2 expression. Free Nrf2 translocates to the nucleus, where it dimerizes with members of the sMaf family and binds to ARE within regulatory regions of a wide variety of cell defense genes, including GST and NQO1. LincRNA 1700020I14Rik can interact with miR-34a-5p to inhibit cell proliferation and fibrosis in DN though the SIRT1/HIF-1α signaling pathway. LncRNA GAS5 and SOX2OT can upregulate SIRT1 expression by sponging miR-221 and miR-9, respectively. SIRT1 can inhibit TGF-β1-induced renal fibrosis and NOX4-induced oxidative stress, and stimulate PGC-1α-mediated mitochondrial biogenesis to improve DN. Finally, SIRT1 can deacetylate and activate FOXO1 to upregulate the level of autophagy, and deacetylate and inhibit p53 to inhibit apoptosis, thus improving DN. SIRT1, Sirtuin 1; DM, diabetes mellitus; miR, microRNA; HIC1, hypermethylated in cancer 1; HG, high glucose; EZH2, enhancer of zeste homolog 2; Dnmt1, DNA methyltransferase 1; CLDN1, claudin-1; DN, diabetic nephropathy; NF-κB, nuclear factor kappa B; Keap1, Kelch-like ECH-associated protein 1; Nrf2, nuclear factor erythroid 2-related factor 2; sMaf, small Maf; ARE, antioxidant response element; GST, glutathione S-transferase; NQO1, nicotinamide adenine dinucleotide phosphate (NADPH) quinone dehydrogenase 1; LincRNA, long intergenic non-coding RNA; HIF-1α, hypoxia-inducible factor-1α; LncRNA GAS5, long non-coding RNA growth arrest special 5; SOX2OT, SOX2-overlapping transcript; TGF-β1, transforming growth factor-beta 1; NOX4, NADPH oxidase 4; PGC-1α, peroxisome proliferator-activated receptor gamma coactivator-1 alpha FOXO1, forkhead box O 1; p53, tumor protein 53.
Diabetic wild-type mice present with increased cell apoptosis, degenerative capillaries, and decreased vascular density accompanied with hypermethylation at the promoter of Sirt1 in retinal microvessels, but overexpression of SIRT1 ameliorated these pathological changes in DR. Overexpression of SIRT1 also protected mitochondria from DM-induced mtDNA damage and prevented the activation of mitochondria-damaging MMP-9. Mechanistically, DM suppresses Sirt1 transcription by regulating the DNA methylation of its promoter via increasing Dnmt1 expression. Importantly, Dnmt1 expression was downregulated in diabetic Sirt1 mice through decreased H3K9 acetylation of the Dnmt1 promoter, suggesting an important role for epigenetics in the transcription of Sirt1 (85). Furthermore, upregulation of Sirt1 by resveratrol led to increased deacetylation of NF-κB-p65 that reduced binding of NF-κB-p65 at the MMP-9 promoter to prevent mitochondrial damage and the development of DR (86).
ncRNAs can serve as biomarkers for various pathological conditions and participate in the initiation and progression of DR by targeting SIRT1. Zhao et al. investigated the important effect of miR-23b-3p on metabolic memory in DR. They found that the expression of miR-23b-3p was promoted by HG treatment and remained elevated even after the return to normal levels of glucose, whereas the expression of SIRT1 was decreased in HRECs. Further investigation indicated that NF-κB-p65 could mediate HG-induced transactivation of miR-23b-3p by binding to the promoter element of pri-miR-23b-27b-24-1. However, suppression of miR-23b-3p expression could inhibit acetyl-NF-κB expression, which was abolished by SIRT1 knockdown in HG-treated HRECs. These findings suggest that the miR-23b-3p/SIRT1/NF-κB feedback loop may exert a crucial role in the establishment and maintenance of metabolic memory in DR (87). In addition, inhibition of miR-211, miR-29b-3p, miR-221, miR-34a, miR-217, miR-195, miR-377, miR-543, and miR-204 was also reported to upregulate the expression of SIRT1 and improve DR by alleviating apoptosis, inflammation, oxidative stress, angiogenesis, and ER stress (88–97).
LncRNAs can also regulate the expression of miRNAs to participate in the progression of DR. For instance, lncRNA maternally expressed gene 3 (MEG3) alleviated HG-induced inflammation and apoptosis in retina epithelial cells via the inhibition of NF-κB signaling and the increase in Bcl-2/Bcl-2-associated X protein ratio by regulating the miR-34a/SIRT1 axis (91). Recently, Ke et al. reported that lncRNA small nucleolar RNA host gene 7 (SNHG7) could inhibit miR-543 to upregulate SIRT1 expression, thereby inhibiting HG-induced angiogenesis in HRECs (95). The role of ncRNAs-mediated SIRT1 changes in DR are summarized in Table 1.
These above-mentioned findings highlight the importance for us to continue to study the roles of SIRT1 in diabetic microvascular complications. The related epigenetic mechanisms of SIRT1 may further provide new insights into potential therapeutic strategies for the treatment of diabetic microvascular complications.
Given that SIRT1 is a key mediator to prevent the progression of diabetic complications, it is necessary to develop specific therapeutic strategies to restore SIRT1 activity. The most obvious approach would be to simply stimulate SIRT1 activity using SIRT1 agonists. Resveratrol is a well-known polyphenolic SIRT1 agonist that has been reported to ameliorate diabetic complications through the regulation of autophagy, oxidative stress and mitochondrial function in DCM, DN and DR animal models (9–12, 79, 86). Milne et al. have shown small molecule activators of SIRT1, including SRT1460, SRT1720, and SRT2183, are structurally unrelated to resveratrol but can be 1,000-fold more potent as activators compared to resveratrol. These compounds can bind to the SIRT1 enzyme-peptide substrate complex at an allosteric site in the amino-terminal region to the catalytic domain and lower the Michaelis constant for acetylated substrates. Additional investigation found that these compounds ablated insulin resistance in mice with diet-induced obesity and genetically obese mice (Lepob/ob), and improved insulin sensitivity and glucose homeostasis in key metabolic tissues including liver, muscle, and fat of male fatty Zucker rats (98). However, recent studies have indicated that resveratrol, SRT1460, SRT1720, and SRT2183 may not be direct activators of SIRT1 (99, 100).
Another SIRT1 agonist, N-acetyl-5-methoxytryptamine, commonly known as melatonin, has been revealed to exert its protective properties in DCM through the prevention of Drp1-mediated mitochondrial fission by SIRT1/PGC1-α pathway (37). Another study showed that melatonin could also inhibit pro-inflammatory cytokine production in the progression of DR (97). Recently, Xue et al. showed that salidroside, an active component of the Traditional Chinese Medicinal plant Rhodiola rosea L., also activated SIRT1/PGC1-α signaling pathway to stimulate mitochondrial biogenesis, thus improving DN (80). Other herbal medicines or compounds, such as puerarin, Tangshen formula, astragaloside IV, and astragalus polysaccharide, have also been reported to ameliorate diabetic complications via the activation of SIRT1 (81–83, 96). Metformin, a derivative of biguanides, is a first line drug for the therapy of T2D and it has been reported to alleviate DN by inducing the SIRT1/FOXO1 autophagic signaling axis (84). Moreover, a study focused on the protective properties of acetaldehyde dehydrogenase 2 (ALDH2), a rate-limiting enzyme for alcohol metabolism, and found that ALDH2 could improve damage induced by STZ in rats with aged diabetic retinas (101). Recently, Qu et al. indicated that 1,25(OH)2D3, as a potential PARP1 inhibitor, played a crucial protective role in DCM through PARP1/SIRT1/mTOR-related mechanisms (40).
In summary, SIRT1 agonists are promising candidates to use in a novel therapeutic approach for the treatment of diabetic complications. Although the effects of numerous SIRT1 agonists on diabetic complications have been reported, the specificity of the SIRT1 agonists remains a concern. Further research is required to investigate specific SIRT1 agonists and push them closer to clinical application. SIRT1 agonists and their related roles in diabetic complications are summarized in Table 2.
Advances in the field of epigenetics have deepened and aided our understanding of gene regulation in health and disease. The evidence discussed above suggests that epigenetic mechanisms play crucial roles in the pathophysiological process of diabetic complications. Further study of epigenetic regulatory events will help us predict the onset or progression of diabetic complications more accurately. Therefore, therapeutic options based on epigenetic regulation may provide a unique treatment strategy whereby physiological gene expression patterns can be recovered and the progression of diabetic complications can be prevented. SIRT1 as a deacetylase has been found to exert protective roles in diabetic complications. To date, research regarding the important role of SIRT1 in reducing diabetic complications is underway but the exact effects in clinical applications have not been fully established due to the intricacy of the regulatory mechanisms and the multiple pathways involved. Therefore, the related epigenetic regulation of SIRT1 for the clinical treatment of diabetic complications is still a long way off. Additional research is required to identify the causal relationship between epigenetic mechanisms and disease status, and the precise mechanisms of SIRT1 involvement in epigenetic regulation for the treatment of diabetic complications require further investigation.
JW(a), JW(b), MX, and YG performed the systematic search, did data extraction, interpreted the data and drafted the review. SW, YT, and JZ contributed to the discussion. JG supervised and revised the manuscript. All authors contributed to the article and approved the submitted version.
This study was supported by the Qilu Young Scholar’s Program of Shandong University (21330089963007), the National Natural Science Foundation of China (81700329, 81770375), and the Jilin Science and Technology Department (20200801061GH).
The authors declare that the research was conducted in the absence of any commercial or financial relationships that could be construed as a potential conflict of interest.
The handling editor declared a shared affiliation with several of the authors JW(a), JW(b), MX, YG, YT, and JG at time of review.
2. Cho NH, Shaw JE, Karuranga S, Huang Y, da Rocha Fernandes JD, Ohlrogge AW, et al. IDF Diabetes Atlas: Global estimates of diabetes prevalence for 2017 and projections for 2045. Diabetes Res Clin Pract (2018) 138:271–81. doi: 10.1016/j.diabres.2018.02.023
3. Shi Y, Vanhoutte PM. Macro- and microvascular endothelial dysfunction in diabetes. J Diabetes (2017) 9:434–49. doi: 10.1111/1753-0407.12521
4. Hemminki K, Li X, Sundquist K, Sundquist J. Familial risks for type 2 diabetes in Sweden. Diabetes Care (2010) 33:293–7. doi: 10.2337/dc09-0947
5. Boljat A, Gunjaca I, Konstantinovic I, Vidan N, Boraska Perica V, Pehlic M, et al. Environmental Risk Factors for Type 1 Diabetes Mellitus Development. Exp Clin Endocrinol Diabetes (2017) 125:563–70. doi: 10.1055/s-0043-109000
6. Olioso D, Dauriz M, Bacchi E, Negri C, Santi L, Bonora E, et al. Effects of Aerobic and Resistance Training on Circulating Micro-RNA Expression Profile in Subjects With Type 2 Diabetes. J Clin Endocrinol Metab (2019) 104:1119–30. doi: 10.1210/jc.2018-01820
7. Ganesan A. Epigenetics: the first 25 centuries. Philos Trans R Soc Lond B Biol Sci (2018) 373:1–4. doi: 10.1098/rstb.2017.0067
8. Vaquero A, Scher M, Lee D, Erdjument-Bromage H, Tempst P, Reinberg D. Human SirT1 interacts with histone H1 and promotes formation of facultative heterochromatin. Mol Cell (2004) 16:93–105. doi: 10.1016/j.molcel.2004.08.031
9. Wang XL, Wu LY, Zhao L, Sun LN, Liu HY, Liu G, et al. SIRT1 activator ameliorates the renal tubular injury induced by hyperglycemia in vivo and in vitro via inhibiting apoptosis. BioMed Pharmacother (2016) 83:41–50. doi: 10.1016/j.biopha.2016.06.009
10. Wang B, Yang Q, Sun YY, Xing YF, Wang YB, Lu XT, et al. Resveratrol-enhanced autophagic flux ameliorates myocardial oxidative stress injury in diabetic mice. J Cell Mol Med (2014) 18:1599–611. doi: 10.1111/jcmm.12312
11. Bagul PK, Deepthi N, Sultana R, Banerjee SK. Resveratrol ameliorates cardiac oxidative stress in diabetes through deacetylation of NFkB-p65 and histone 3. J Nutr Biochem (2015) 26:1298–307. doi: 10.1016/j.jnutbio.2015.06.006
12. Fang WJ, Wang CJ, He Y, Zhou YL, Peng XD, Liu SK. Resveratrol alleviates diabetic cardiomyopathy in rats by improving mitochondrial function through PGC-1alpha deacetylation. Acta Pharmacol Sin (2018) 39:59–73. doi: 10.1038/aps.2017.50
13. O’Hagan HM, Mohammad HP, Baylin SB. Double strand breaks can initiate gene silencing and SIRT1-dependent onset of DNA methylation in an exogenous promoter CpG island. PLoS Genet (2008) 4:e1000155. doi: 10.1371/journal.pgen.1000155
14. Li Y, Wong K, Giles A, Jiang J, Lee JW, Adams AC, et al. Hepatic SIRT1 attenuates hepatic steatosis and controls energy balance in mice by inducing fibroblast growth factor 21. Gastroenterology (2014) 146:539–49.e7. doi: 10.1053/j.gastro.2013.10.059
15. Liu P, Feng T, Zuo X, Wang X, Luo J, Li N, et al. A novel SIRT1 activator E6155 improves insulin sensitivity in type 2 diabetic KKAy mice. Biochem Biophys Res Commun (2018) 498:633–9. doi: 10.1016/j.bbrc.2018.03.034
16. D’Onofrio N, Servillo L, Balestrieri ML. SIRT1 and SIRT6 Signaling Pathways in Cardiovascular Disease Protection. Antioxid Redox Signal (2018) 28:711–32. doi: 10.1089/ars.2017.7178
17. Seferovic PM, Paulus WJ. Clinical diabetic cardiomyopathy: a two-faced disease with restrictive and dilated phenotypes. Eur Heart J (2015) 36:1718–27 1727a–c. doi: 10.1093/eurheartj/ehv134
18. Cooper ME. Metabolic memory: implications for diabetic vascular complications. Pediatr Diabetes (2009) 10:343–6. doi: 10.1111/j.1399-5448.2008.00491.x
19. Yu XY, Geng YJ, Liang JL, Zhang S, Lei HP, Zhong SL, et al. High levels of glucose induce “metabolic memory” in cardiomyocyte via epigenetic histone H3 lysine 9 methylation. Mol Biol Rep (2012) 39:8891–8. doi: 10.1007/s11033-012-1756-z
20. Chen Y, Du J, Zhao YT, Zhang L, Lv G, Zhuang S, et al. Histone deacetylase (HDAC) inhibition improves myocardial function and prevents cardiac remodeling in diabetic mice. Cardiovasc Diabetol (2015) 14:99. doi: 10.1186/s12933-015-0262-8
21. Zhao T, Parikh P, Bhashyam S, Bolukoglu H, Poornima I, Shen YT, et al. Direct effects of glucagon-like peptide-1 on myocardial contractility and glucose uptake in normal and postischemic isolated rat hearts. J Pharmacol Exp Ther (2006) 317:1106–13. doi: 10.1124/jpet.106.100982
22. Lee TI, Kao YH, Tsai WC, Chung CC, Chen YC, Chen YJ. HDAC Inhibition Modulates Cardiac PPARs and Fatty Acid Metabolism in Diabetic Cardiomyopathy. PPAR Res (2016) 2016:5938740. doi: 10.1155/2016/5938740
23. Xu Z, Tong Q, Zhang Z, Wang S, Zheng Y, Liu Q, et al. Inhibition of HDAC3 prevents diabetic cardiomyopathy in OVE26 mice via epigenetic regulation of DUSP5-ERK1/2 pathway. Clin Sci (Lond) (2017) 131:1841–57. doi: 10.1042/CS20170064
24. Dong F, Ren J. Fidarestat improves cardiomyocyte contractile function in db/db diabetic obese mice through a histone deacetylase Sir2-dependent mechanism. J Hypertens (2007) 25:2138–47. doi: 10.1097/HJH.0b013e32828626d1
25. Costantino S, Paneni F, Luscher TF, Cosentino F. MicroRNA profiling unveils hyperglycaemic memory in the diabetic heart. Eur Heart J (2016) 37:572–6. doi: 10.1093/eurheartj/ehv599
26. Ucar A, Gupta SK, Fiedler J, Erikci E, Kardasinski M, Batkai S, et al. The miRNA-212/132 family regulates both cardiac hypertrophy and cardiomyocyte autophagy. Nat Commun (2012) 3:1078. doi: 10.1038/ncomms2090
27. Su M, Wang J, Wang C, Wang X, Dong W, Qiu W, et al. MicroRNA-221 inhibits autophagy and promotes heart failure by modulating the p27/CDK2/mTOR axis. Cell Death Differ (2015) 22:986–99. doi: 10.1038/cdd.2014.187
28. Liang C, Gao L, Liu Y, Liu Y, Yao R, Li Y, et al. MiR-451 antagonist protects against cardiac fibrosis in streptozotocin-induced diabetic mouse heart. Life Sci (2019) 224:12–22. doi: 10.1016/j.lfs.2019.02.059
29. Yu M, Liu Y, Zhang B, Shi Y, Cui L, Zhao X. Inhibiting microRNA-144 abates oxidative stress and reduces apoptosis in hearts of streptozotocin-induced diabetic mice. Cardiovasc Pathol (2015) 24:375–81. doi: 10.1016/j.carpath.2015.06.003
30. Chavali V, Tyagi SC, Mishra PK. MicroRNA-133a regulates DNA methylation in diabetic cardiomyocytes. Biochem Biophys Res Commun (2012) 425:668–72. doi: 10.1016/j.bbrc.2012.07.105
31. Yang F, Qin Y, Lv J, Wang Y, Che H, Chen X, et al. Silencing long non-coding RNA Kcnq1ot1 alleviates pyroptosis and fibrosis in diabetic cardiomyopathy. Cell Death Dis (2018) 9:1000. doi: 10.1038/s41419-018-1029-4
32. Zhang M, Gu H, Xu W, Zhou X. Down-regulation of lncRNA MALAT1 reduces cardiomyocyte apoptosis and improves left ventricular function in diabetic rats. Int J Cardiol (2016) 203:214–6. doi: 10.1016/j.ijcard.2015.10.136
33. Zhou X, Zhang W, Jin M, Chen J, Xu W, Kong X. lncRNA MIAT functions as a competing endogenous RNA to upregulate DAPK2 by sponging miR-22-3p in diabetic cardiomyopathy. Cell Death Dis (2017) 8:e2929. doi: 10.1038/cddis.2017.321
34. Li X, Wang H, Yao B, Xu W, Chen J, Zhou X. lncRNA H19/miR-675 axis regulates cardiomyocyte apoptosis by targeting VDAC1 in diabetic cardiomyopathy. Sci Rep (2016) 6:36340. doi: 10.1038/srep36340
35. Monkemann H, De Vriese AS, Blom HJ, Kluijtmans LA, Heil SG, Schild HH, et al. Early molecular events in the development of the diabetic cardiomyopathy. Amino Acids (2002) 23:331–6. doi: 10.1007/s00726-001-0146-y
36. Costantino S, Paneni F, Mitchell K, Mohammed SA, Hussain S, Gkolfos C, et al. Hyperglycaemia-induced epigenetic changes drive persistent cardiac dysfunction via the adaptor p66(Shc). Int J Cardiol (2018) 268:179–86. doi: 10.1016/j.ijcard.2018.04.082
37. Ding M, Feng N, Tang D, Feng J, Li Z, Jia M, et al. Melatonin prevents Drp1-mediated mitochondrial fission in diabetic hearts through SIRT1-PGC1alpha pathway. J Pineal Res (2018) 65:e12491. doi: 10.1111/jpi.12491
38. Schreiber V, Dantzer F, Ame JC, de Murcia G. Poly(ADP-ribose): novel functions for an old molecule. Nat Rev Mol Cell Biol (2006) 7:517–28. doi: 10.1038/nrm1963
39. Waldman M, Nudelman V, Shainberg A, Abraham NG, Kornwoski R, Aravot D, et al. PARP-1 inhibition protects the diabetic heart through activation of SIRT1-PGC-1alpha axis. Exp Cell Res (2018) 373:112–8. doi: 10.1016/j.yexcr.2018.10.003
40. Qu H, Lin K, Wang H, Wei H, Ji B, Yang Z, et al. 1,25(OH)2 D3 improves cardiac dysfunction, hypertrophy, and fibrosis through PARP1/SIRT1/mTOR-related mechanisms in type 1 diabetes. Mol Nutr Food Res (2017) 61:1–11. doi: 10.1002/mnfr.201600338
41. Nan X, Ng HH, Johnson CA, Laherty CD, Turner BM, Eisenman RN, et al. Transcriptional repression by the methyl-CpG-binding protein MeCP2 involves a histone deacetylase complex. Nature (1998) 393:386–9. doi: 10.1038/30764
42. Peng L, Yuan Z, Ling H, Fukasawa K, Robertson K, Olashaw N, et al. SIRT1 deacetylates the DNA methyltransferase 1 (DNMT1) protein and alters its activities. Mol Cell Biol (2011) 31:4720–34. doi: 10.1128/MCB.06147-11
43. Chen Z, Gong L, Zhang P, Li Y, Liu B, Zhang L, et al. Epigenetic Down-Regulation of Sirt 1 via DNA Methylation and Oxidative Stress Signaling Contributes to the Gestational Diabetes Mellitus-Induced Fetal Programming of Heart Ischemia-Sensitive Phenotype in Late Life. Int J Biol Sci (2019) 15:1240–51. doi: 10.7150/ijbs.33044
44. Zheng D, Ma J, Yu Y, Li M, Ni R, Wang G, et al. Silencing of miR-195 reduces diabetic cardiomyopathy in C57BL/6 mice. Diabetologia (2015) 58:1949–58. doi: 10.1007/s00125-015-3622-8
45. Tang Q, Len Q, Liu Z, Wang W. Overexpression of miR-22 attenuates oxidative stress injury in diabetic cardiomyopathy via Sirt 1. Cardiovasc Ther (2018) 36:1–11. doi: 10.1111/1755-5922.12318
46. Gao L, Wang X, Guo S, Xiao L, Liang C, Wang Z, et al. LncRNA HOTAIR functions as a competing endogenous RNA to upregulate SIRT1 by sponging miR-34a in diabetic cardiomyopathy. J Cell Physiol (2019) 234:4944–58. doi: 10.1002/jcp.27296
47. Zhang L, Zhang Q, Liu S, Chen Y, Li R, Lin T, et al. DNA methyltransferase 1 may be a therapy target for attenuating diabetic nephropathy and podocyte injury. Kidney Int (2017) 92:140–53. doi: 10.1016/j.kint.2017.01.010
48. Hishikawa A, Hayashi K, Abe T, Kaneko M, Yokoi H, Azegami T, et al. Decreased KAT5 Expression Impairs DNA Repair and Induces Altered DNA Methylation in Kidney Podocytes. Cell Rep (2019) 26:1318–32.e4. doi: 10.1016/j.celrep.2019.01.005
49. Marumo T, Yagi S, Kawarazaki W, Nishimoto M, Ayuzawa N, Watanabe A, et al. Diabetes Induces Aberrant DNA Methylation in the Proximal Tubules of the Kidney. J Am Soc Nephrol (2015) 26:2388–97. doi: 10.1681/ASN.2014070665
50. De Marinis Y, Cai M, Bompada P, Atac D, Kotova O, Johansson ME, et al. Epigenetic regulation of the thioredoxin-interacting protein (TXNIP) gene by hyperglycemia in kidney. Kidney Int (2016) 89:342–53. doi: 10.1016/j.kint.2015.12.018
51. Alghamdi TA, Batchu SN, Hadden MJ, Yerra VG, Liu Y, Bowskill BB, et al. Histone H3 Serine 10 Phosphorylation Facilitates Endothelial Activation in Diabetic Kidney Disease. Diabetes (2018) 67:2668–81. doi: 10.2337/db18-0124
52. Jia Y, Reddy MA, Das S, Oh HJ, Abdollahi M, Yuan H, et al. Dysregulation of histone H3 lysine 27 trimethylation in transforming growth factor-beta1-induced gene expression in mesangial cells and diabetic kidney. J Biol Chem (2019) 294:12695–707. doi: 10.1074/jbc.RA119.007575
53. Yuan H, Reddy MA, Deshpande S, Jia Y, Park JT, Lanting LL, et al. Epigenetic Histone Modifications Involved in Profibrotic Gene Regulation by 12/15-Lipoxygenase and Its Oxidized Lipid Products in Diabetic Nephropathy. Antioxid Redox Signal (2016) 24:361–75. doi: 10.1089/ars.2015.6372
54. Huang S, Xu Y, Ge X, Xu B, Peng W, Jiang X, et al. Long noncoding RNA NEAT1 accelerates the proliferation and fibrosis in diabetic nephropathy through activating Akt/mTOR signaling pathway. J Cell Physiol (2019) 234:11200–7. doi: 10.1002/jcp.27770
55. Wang M, Wang S, Yao D, Yan Q, Lu W. A novel long non-coding RNA CYP4B1-PS1-001 regulates proliferation and fibrosis in diabetic nephropathy. Mol Cell Endocrinol (2016) 426:136–45. doi: 10.1016/j.mce.2016.02.020
56. Bai X, Geng J, Li X, Wan J, Liu J, Zhou Z, et al. Long Noncoding RNA LINC01619 Regulates MicroRNA-27a/Forkhead Box Protein O1 and Endoplasmic Reticulum Stress-Mediated Podocyte Injury in Diabetic Nephropathy. Antioxid Redox Signal (2018) 29:355–76. doi: 10.1089/ars.2017.7278
57. Hu M, Wang R, Li X, Fan M, Lin J, Zhen J, et al. LncRNA MALAT1 is dysregulated in diabetic nephropathy and involved in high glucose-induced podocyte injury via its interplay with beta-catenin. J Cell Mol Med (2017) 21:2732–47. doi: 10.1111/jcmm.13189
58. Maghbooli Z, Hossein-nezhad A, Larijani B, Amini M, Keshtkar A. Global DNA methylation as a possible biomarker for diabetic retinopathy. Diabetes Metab Res Rev (2015) 31:183–9. doi: 10.1002/dmrr.2584
59. Duraisamy AJ, Mishra M, Kowluru A, Kowluru RA. Epigenetics and Regulation of Oxidative Stress in Diabetic Retinopathy. Invest Ophthalmol Vis Sci (2018) 59:4831–40. doi: 10.1167/iovs.18-24548
60. Zhong Q, Kowluru RA. Epigenetic Changes in Mitochondrial Superoxide Dismutase in the Retina and the Development of Diabetic Retinopathy. Diabetes (2011) 60:1304–13. doi: 10.2337/db10-0133
61. Zhong Q, Kowluru RA. Epigenetic modification of Sod2 in the development of diabetic retinopathy and in the metabolic memory: role of histone methylation. Invest Ophthalmol Vis Sci (2013) 54:244–50. doi: 10.1167/iovs.12-10854
62. Kowluru RA, Shan Y. Role of oxidative stress in epigenetic modification of MMP-9 promoter in the development of diabetic retinopathy. Graefes Arch Clin Exp Ophthalmol (2017) 255:955–62. doi: 10.1007/s00417-017-3594-0
63. Kowluru RA, Shan Y, Mishra M. Dynamic DNA methylation of matrix metalloproteinase-9 in the development of diabetic retinopathy. Lab Invest (2016) 96:1040–9. doi: 10.1038/labinvest.2016.78
64. Duraisamy AJ, Mishra M, Kowluru RA. Crosstalk Between Histone and DNA Methylation in Regulation of Retinal Matrix Metalloproteinase-9 in Diabetes. Invest Ophthalmol Vis Sci (2017) 58:6440–8. doi: 10.1167/iovs.17-22706
65. Mishra M, Kowluru RA. DNA Methylation-a Potential Source of Mitochondria DNA Base Mismatch in the Development of Diabetic Retinopathy. Mol Neurobiol (2019) 56:88–101. doi: 10.1007/s12035-018-1086-9
66. Mohammad G, Radhakrishnan R, Kowluru RA. Epigenetic Modifications Compromise Mitochondrial DNA Quality Control in the Development of Diabetic Retinopathy. Invest Ophthalmol Vis Sci (2019) 60:3943–51. doi: 10.1167/iovs.19-27602
67. Biswas S, Thomas AA, Chen S, Aref-Eshghi E, Feng B, Gonder J, et al. MALAT1: An Epigenetic Regulator of Inflammation in Diabetic Retinopathy. Sci Rep (2018) 8:6526. doi: 10.1038/s41598-018-24907-w
68. Zhang J, Chen M, Chen J, Lin S, Cai D, Chen C, et al. Long non-coding RNA MIAT acts as a biomarker in diabetic retinopathy by absorbing miR-29b and regulating cell apoptosis. Biosci Rep (2017) 37:1–9. doi: 10.1042/BSR20170036
69. Hasegawa K, Wakino S, Simic P, Sakamaki Y, Minakuchi H, Fujimura K, et al. Renal tubular Sirt1 attenuates diabetic albuminuria by epigenetically suppressing Claudin-1 overexpression in podocytes. Nat Med (2013) 19:1496–504. doi: 10.1038/nm.3363
70. Yang S, Zhao L, Han Y, Liu Y, Chen C, Zhan M, et al. Probucol ameliorates renal injury in diabetic nephropathy by inhibiting the expression of the redox enzyme p66Shc. Redox Biol (2017) 13:482–97. doi: 10.1016/j.redox.2017.07.002
71. Zeng S, Wu X, Chen X, Xu H, Zhang T, Xu Y. Hypermethylated in cancer 1 (HIC1) mediates high glucose induced ROS accumulation in renal tubular epithelial cells by epigenetically repressing SIRT1 transcription. Biochim Biophys Acta Gene Regul Mech (2018) 1861:917–27. doi: 10.1016/j.bbagrm.2018.08.002
72. Zhou L, Xu DY, Sha WG, Shen L, Lu GY, Yin X, et al. High glucose induces renal tubular epithelial injury via Sirt1/NF-kappaB/microR-29/Keap1 signal pathway. J Transl Med (2015) 13:352. doi: 10.1186/s12967-015-0710-y
73. Xue M, Li Y, Hu F, Jia YJ, Zheng ZJ, Wang L, et al. High glucose up-regulates microRNA-34a-5p to aggravate fibrosis by targeting SIRT1 in HK-2cells. Biochem Biophys Res Commun (2018) 498:38–44. doi: 10.1016/j.bbrc.2017.12.048
74. Li A, Peng R, Sun Y, Liu H, Peng H, Zhang Z. LincRNA 1700020I14Rik alleviates cell proliferation and fibrosis in diabetic nephropathy via miR-34a-5p/Sirt1/HIF-1alpha signaling. Cell Death Dis (2018) 9:461. doi: 10.1038/s41419-018-0527-8
75. Ge X, Xu B, Xu W, Xia L, Xu Z, Shen L, et al. Long noncoding RNA GAS5 inhibits cell proliferation and fibrosis in diabetic nephropathy by sponging miR-221 and modulating SIRT1 expression. Aging (Albany NY) (2019) 11:8745–59. doi: 10.18632/aging.102249
76. Sun Z, Ma Y, Chen F, Wang S, Chen B, Shi J. miR-133b and miR-199b knockdown attenuate TGF-beta1-induced epithelial to mesenchymal transition and renal fibrosis by targeting SIRT1 in diabetic nephropathy. Eur J Pharmacol (2018) 837:96–104. doi: 10.1016/j.ejphar.2018.08.022
77. Zhang Y, Chang B, Zhang J, Wu X. LncRNA SOX2OT alleviates the high glucose-induced podocytes injury through autophagy induction by the miR-9/SIRT1 axis. Exp Mol Pathol (2019) 110:104283. doi: 10.1016/j.yexmp.2019.104283
78. Wang Y, Zheng ZJ, Jia YJ, Yang YL, Xue YM. Role of p53/miR-155-5p/sirt1 loop in renal tubular injury of diabetic kidney disease. J Transl Med (2018) 16:146. doi: 10.1186/s12967-018-1486-7
79. Zhang T, Chi Y, Kang Y, Lu H, Niu H, Liu W, et al. Resveratrol ameliorates podocyte damage in diabetic mice via SIRT1/PGC-1alpha mediated attenuation of mitochondrial oxidative stress. J Cell Physiol (2019) 234:5033–43. doi: 10.1002/jcp.27306
80. Xue H, Li P, Luo Y, Wu C, Liu Y, Qin X, et al. Salidroside stimulates the Sirt1/PGC-1alpha axis and ameliorates diabetic nephropathy in mice. Phytomedicine (2019) 54:240–7. doi: 10.1016/j.phymed.2018.10.031
81. Li X, Cai W, Lee K, Liu B, Deng Y, Chen Y, et al. Puerarin attenuates diabetic kidney injury through the suppression of NOX4 expression in podocytes. Sci Rep (2017) 7:14603. doi: 10.1038/s41598-017-14906-8
82. Du YG, Zhang KN, Gao ZL, Dai F, Wu XX, Chai KF. Tangshen formula improves inflammation in renal tissue of diabetic nephropathy through SIRT1/NF-kappaB pathway. Exp Ther Med (2018) 15:2156–64. doi: 10.3892/etm.2017.5621
83. Wang X, Gao Y, Tian N, Zhu Z, Wang T, Xu J, et al. Astragaloside IV represses high glucose-induced mesangial cells activation by enhancing autophagy via SIRT1 deacetylation of NF-kappaB p65 subunit. Drug Des Devel Ther (2018) 12:2971–80. doi: 10.2147/DDDT.S174058
84. Xu J, Liu LQ, Xu LL, Xing Y, Ye S. Metformin alleviates renal injury in diabetic rats by inducing Sirt1/FoxO1 autophagic signal axis. Clin Exp Pharmacol Physiol (2020) 47:599–608. doi: 10.1111/1440-1681.13226
85. Mishra M, Duraisamy AJ, Kowluru RA. Sirt1: A Guardian of the Development of Diabetic Retinopathy. Diabetes (2018) 67:745–54. doi: 10.2337/db17-0996
86. Kowluru RA, Santos JM, Zhong Q. Sirt1, a negative regulator of matrix metalloproteinase-9 in diabetic retinopathy. Invest Ophthalmol Vis Sci (2014) 55:5653–60. doi: 10.1167/iovs.14-14383
87. Zhao S, Li T, Li J, Lu Q, Han C, Wang N, et al. miR-23b-3p induces the cellular metabolic memory of high glucose in diabetic retinopathy through a SIRT1-dependent signalling pathway. Diabetologia (2016) 59:644–54. doi: 10.1007/s00125-015-3832-0
88. Liu HN, Cao NJ, Li X, Qian W, Chen XL. Serum microRNA-211 as a biomarker for diabetic retinopathy via modulating Sirtuin 1. Biochem Biophys Res Commun (2018) 505:1236–43. doi: 10.1016/j.bbrc.2018.10.052
89. Zeng Y, Cui Z, Liu J, Chen J, Tang S. MicroRNA-29b-3p Promotes Human Retinal Microvascular Endothelial Cell Apoptosis via Blocking SIRT1 in Diabetic Retinopathy. Front Physiol (2019) 10:1621. doi: 10.3389/fphys.2019.01621
90. Chen B, Wu L, Cao T, Zheng HM, He T. MiR-221/SIRT1/Nrf2 signal axis regulates high glucose induced apoptosis in human retinal microvascular endothelial cells. BMC Ophthalmol (2020) 20:300. doi: 10.1186/s12886-020-01559-x
91. Tong P, Peng QH, Gu LM, Xie WW, Li WJ. LncRNA-MEG3 alleviates high glucose induced inflammation and apoptosis of retina epithelial cells via regulating miR-34a/SIRT1 axis. Exp Mol Pathol (2019) 107:102–9. doi: 10.1016/j.yexmp.2018.12.003
92. Xiao H, Liu Z. Effects of microRNA217 on high glucoseinduced inflammation and apoptosis of human retinal pigment epithelial cells (ARPE19) and its underlying mechanism. Mol Med Rep (2019) 20:5125–33. doi: 10.3892/mmr.2019.10778
93. Mortuza R, Feng B, Chakrabarti S. miR-195 regulates SIRT1-mediated changes in diabetic retinopathy. Diabetologia (2014) 57:1037–46. doi: 10.1007/s00125-014-3197-9
94. Cui C, Li Y, Liu Y. Down-regulation of miR-377 suppresses high glucose and hypoxia-induced angiogenesis and inflammation in human retinal endothelial cells by direct up-regulation of target gene SIRT1. Hum Cell (2019) 32:260–74. doi: 10.1007/s13577-019-00240-w
95. Ke N, Pi LH, Liu Q, Chen L. Long noncoding RNA SNHG7 inhibits high glucose-induced human retinal endothelial cells angiogenesis by regulating miR-543/SIRT1 axis. Biochem Biophys Res Commun (2019) 514:503–9. doi: 10.1016/j.bbrc.2019.04.141
96. Peng QH, Tong P, Gu LM, Li WJ. Astragalus polysaccharide attenuates metabolic memory-triggered ER stress and apoptosis via regulation of miR-204/SIRT1 axis in retinal pigment epithelial cells. Biosci Rep (2020) 40:1–15. doi: 10.1042/BSR20192121
97. Tu Y, Zhu M, Wang Z, Wang K, Chen L, Liu W, et al. Melatonin inhibits Muller cell activation and pro-inflammatory cytokine production via upregulating the MEG3/miR-204/Sirt1 axis in experimental diabetic retinopathy. J Cell Physiol (2020) 235:8724–35. doi: 10.1002/jcp.29716
98. Milne JC, Lambert PD, Schenk S, Carney DP, Smith JJ, Gagne DJ, et al. Small molecule activators of SIRT1 as therapeutics for the treatment of type 2 diabetes. Nature (2007) 450:712–6. doi: 10.1038/nature06261
99. Pacholec M, Bleasdale JE, Chrunyk B, Cunningham D, Flynn D, Garofalo RS, et al. SRT1720, SRT2183, SRT1460, and resveratrol are not direct activators of SIRT1. J Biol Chem (2010) 285:8340–51. doi: 10.1074/jbc.M109.088682
100. Beher D, Wu J, Cumine S, Kim KW, Lu SC, Atangan L, et al. Resveratrol is not a direct activator of SIRT1 enzyme activity. Chem Biol Drug Des (2009) 74:619–24. doi: 10.1111/j.1747-0285.2009.00901.x
Keywords: diabetes mellitus, diabetic complications, SIRT1, epigenetics, deacetylation
Citation: Wang(a) J, Wang S, Wang(b) J, Xiao M, Guo Y, Tang Y, Zhang J and Gu J (2021) Epigenetic Regulation Associated With Sirtuin 1 in Complications of Diabetes Mellitus. Front. Endocrinol. 11:598012. doi: 10.3389/fendo.2020.598012
Received: 23 August 2020; Accepted: 27 November 2020;
Published: 18 January 2021.
Edited by:
Hao Wu, Shandong University, ChinaReviewed by:
Madhu Khullar, Post Graduate Institute of Medical Education and Research (PGIMER), IndiaCopyright © 2021 Wang(a), Wang, Wang(b), Xiao, Guo, Tang, Zhang and Gu. This is an open-access article distributed under the terms of the Creative Commons Attribution License (CC BY). The use, distribution or reproduction in other forums is permitted, provided the original author(s) and the copyright owner(s) are credited and that the original publication in this journal is cited, in accordance with accepted academic practice. No use, distribution or reproduction is permitted which does not comply with these terms.
*Correspondence: Junlian Gu, anVubGlhbl9ndUBzZHUuZWR1LmNu
Disclaimer: All claims expressed in this article are solely those of the authors and do not necessarily represent those of their affiliated organizations, or those of the publisher, the editors and the reviewers. Any product that may be evaluated in this article or claim that may be made by its manufacturer is not guaranteed or endorsed by the publisher.
Research integrity at Frontiers
Learn more about the work of our research integrity team to safeguard the quality of each article we publish.