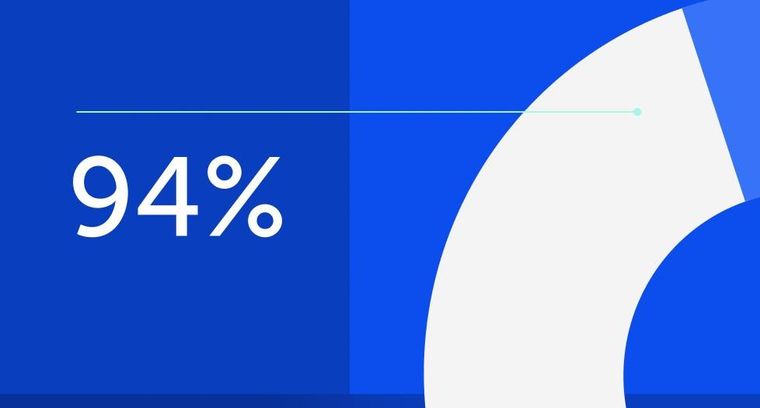
94% of researchers rate our articles as excellent or good
Learn more about the work of our research integrity team to safeguard the quality of each article we publish.
Find out more
REVIEW article
Front. Endocrinol., 18 January 2021
Sec. Bone Research
Volume 11 - 2020 | https://doi.org/10.3389/fendo.2020.578477
This article is part of the Research TopicBone Inside-Out and Outside-In Signals: Control of Body HomeostasisView all 21 articles
Osteocytes make up 90–95% of the cellular content of bone and form a rich dendritic network with a vastly greater surface area than either osteoblasts or osteoclasts. Osteocytes are well positioned to play a role in bone homeostasis by interacting directly with the matrix; however, the ability for these cells to modify bone matrix remains incompletely understood. With techniques for examining the nano- and microstructure of bone matrix components including hydroxyapatite and type I collagen becoming more widespread, there is great potential to uncover novel roles for the osteocyte in maintaining bone quality. In this review, we begin with an overview of osteocyte biology and the lacunar–canalicular system. Next, we describe recent findings from in vitro models of osteocytes, focusing on the transitions in cellular phenotype as they mature. Finally, we describe historical and current research on matrix alteration by osteocytes in vivo, focusing on the exciting potential for osteocytes to directly form, degrade, and modify the mineral and collagen in their surrounding matrix.
Embedded within the mineralized matrix of bone, osteocytes, a cell population of growing importance in bone biology and medicine, find great longevity despite their apparently isolated location. Osteocytes are increasingly recognized as cells that govern the process of bone remodeling by directing bone forming osteoblasts and bone resorbing osteoclasts. While these actions play an important role in determining the location and time-course of bone remodeling, osteocytes themselves are positioned to readily access immense quantities of bone tissue. Making up over 90% of the cellular content of bone, osteocytes form a rich network of dendrites that communicate with roughly 50 neighboring osteocytes, resulting in a total surface area that greatly exceeds that of osteoblasts and osteoclasts combined. Therefore, any stimulus that triggers osteocytes to directly interact with the bone matrix could have a great positive or negative impact on the overall integrity of bone. In this review, we begin with a brief discussion of how osteocytes direct the activities of osteoblasts and osteoclasts. Next, we cover the important role of the lacunar-canalicular network (LCN) in osteocyte communication and remodeling. Finally, we discuss the exciting potential for osteocytes to directly modify the organic and inorganic components of the bone matrix, which may form an important basis for future treatment strategies aimed at improving bone mass and tissue quality.
At the end of their period of bone formation, late-stage osteoblasts are directed via unknown cues to either undergo apoptosis or terminal differentiation (1, 2). One option is for osteoblasts to differentiate into quiescent bone lining cells, which cover the bone surface and are thought to mediate remodeling in localized bone areas (1). Some osteoblasts further differentiate into osteocytes. Late osteoblasts transition to early osteocytes by forming dendrites via upregulation of the gene E11/gp38, or podoplanin (3). Upregulation of MT1-MMP, a metalloproteinase that cleaves collagen, is also required for osteocyte dendrite formation and maintains cell viability throughout differentiation (4, 5). These findings may suggest that osteocyte embedding is an active, proteolytic process, in contrast to initial studies that suggested osteocyte embedding is a process of passive entrapment within the matrix (6, 7). Recently, however, studies utilizing intravital imaging have suggested that there may be multiple mechanisms for osteocyte embedding that involve some combination of the above processes, as well as lacunar reshaping prior to differentiation (8). Once embedded, the osteocyte begins its role in coordinating the actions of osteoblasts and osteoclasts as a part of the rich osteocyte network.
The activities of osteoblasts and osteoclasts are highly regulated by signals originating from osteocytes, although the mechanisms by which signals reach these cells are poorly understood. Osteoblasts are responsible for new bone formation, which primarily occurs on trabecular and cortical bone surfaces (9). Bone formation is notably induced by the Wnt signaling pathway. The canonical pathway involves Wnt binding to low-density lipoprotein receptor-related protein 5/6 (Lrp5/6) and its co-receptor, Frizzled (10). This binding inhibits the intracellular activity of glycogen synthase kinase 3 (GSK3) and its complex consisting of Axin and adenomatous polyposis coli (APC), which results in hypophosphorylation of the transcription factor β-catenin (11). Translocation of intact β-catenin to the nucleus results in the expression of genes that enhance osteoblast survival and bone formation activity. Osteocytes are an important regulator of this process via the secretion of Sclerostin (Sost). Sost is a potent suppressor of Wnt signaling by binding Lrp5/6, competitively inhibiting Wnt binding (12). This results in uninhibited phosphorylation of β-catenin and its subsequent degradation by the proteasome. Sclerostin has also been shown to inhibit bone morphogenic protein (BMP)-related bone formation (13). In humans, mutations in Sost result in sclerosteosis, a condition characterized by increased bone formation resulting in high bone mass and cranial neuropathies due to nerve compression (14). Therefore, osteocytes have the potential to control when and where bone formation occurs and interfering with this process can have dramatic effects on human health.
Interestingly, osteocytes also mediate the process of bone resorption by osteoclasts. One method of regulation is through osteocyte secretion of receptor activator of nuclear factor kappa B ligand (RANKL) through their dendrites, which binds the RANK receptor on osteoclast precursors and drives their differentiation into mature osteoclasts (15). RANKL expression by osteocytes is essential for trabecular bone remodeling and is secreted by osteocytes in regions of osteocytic apoptosis (16, 17). Additionally, osteocytes secrete osteoprotegerin (OPG), a molecule that competes with RANKL for the RANK receptor (18). This interaction suppresses osteoclast activity and is the basis for the anti-resorptive osteoporosis drug denosumab (19, 20). Frequently, the overall secreted RANKL/OPG ratio is measured in in vitro and in vivo models, and in humans, to approximate the degree of osteoclastogenesis in the bone (21). Therefore, osteocytes can modify the total content and activity of mature osteoblasts and osteoclasts, demonstrating their important regulatory role in the process of bone remodeling.
Repair of bone microdamage has also been shown to be dependent on the coordinated actions of osteocytes and osteoclasts. Microdamage, or small cracks or breaks in the bone, trigger osteocyte apoptosis and induce intracortical remodeling, a process that is atypical in rodent cortical bone (22). Further, regions of bone remodeling colocalize with regions of osteocyte apoptosis in the context of microdamage or estrogen deficiency (22, 23). In vitro studies have demonstrated that apoptotic osteocytes stimulate their neighbors to release RANKL, which acts as a chemotactic signal for osteoclasts to migrate into the regions of apoptosis and remodel the bone (24). Therefore, osteocytes also utilize osteoclasts to repair regions of microdamage through the controlled release of RANKL while preserving undamaged regions of the bone.
The process of remodeling is slow and deliberate, but evidence demonstrating decreased whole body bone mineral content in lactating women suggests that rapid changes in systemic mineral demands must be met by liberating mineral from the bone (25). Furthermore, bone matrix components must rapidly reform to maintain bone strength when mineral demands are lifted. Indeed, weaning triggers osteoclast apoptosis and a decrease in RANKL levels within the bone while osteoblastic activity remains elevated, favoring bone formation (26). However, considering that the resorption and formation processes by osteoclasts and osteoblasts, respectively, primarily occur on bone surfaces, it is logical that osteocytes may utilize their large surface area to release bone mineral during lactation and reconstruct their surrounding matrix after weaning. Therefore, due to their large population, extensive network, and sprawling surface area, researchers have begun investigating the osteocyte as a potential candidate for rapid bone alterations when subjected to stimuli that alter bone formation and resorption (27). The remainder of this review will focus on observations from in vitro and in vivo studies examining the potential for osteocytes to control the structure and composition of bone by modulating the activity of osteoblasts and osteoclasts and by direct interaction with the extracellular matrix.
Osteocyte cells are embedded in the mineralized matrix in protected lacunae which surround the cell body. The cells are connected to one another by dendritic cell processes which reside in canaliculi. Together, these form the lacunar–canalicular network (LCN). This interconnected network of cells may be relevant to mechanical sensing and is important for signaling and solute transport (28). As extracellular fluid flows through the LCN, the osteocytes release chemicals such as nitric oxide, prostaglandin, and other factors (29). Additionally, the level of mechanical stimuli is related to osteocyte apoptosis which promotes osteoclastogenesis and is a mechanism by which osteocytes regulate bone repair and shape (28). Loading enhances fluid flow and the shape of the LCN may affect how fluid flows through the system, as observed by the use of injected tracers, where there is an increase in labeled osteocytes with loading (30). Ciani et al. saw an increase in the percentage of osteocytes labeled with an injected tracer in loaded tibiae compared to non-loaded tibiae of rats. However, this only occurred in cancellous bone, not cortical bone (30). This increase of fluid flow with loading has also been speculated with numerical methods. Multiple groups have attempted to quantify the forces placed on the LCN using finite element analysis (FEA) and numerical models which indicate that the shape of the network influences the shear stress the cell is exposed to (31–33). The model in Gatti et al. indicated that vascular porosity plays a role as well, with idealized models showing a decrease in fluid velocity with an increase in vascular porosity (33). Using a fluid–structure interaction model to model a single cell, Joukar et al. indicated cells in rounded lacunae experienced less shear stress than elliptical ones under different modes of loading (32). The overall organization and shape of the LCN affect the ability of the osteocyte to sense stimuli, communicate with other cells, and effectively modulate bone quality.
The LCN can be imaged multiple ways, in two and three dimensions to provide quantitative measures of the LCN and osteocyte shape and organization. Two dimensional methods include scanning electron microscopy (11) with silver staining or quantitative backscatter imaging. Three dimensional methods allow for data analysis on connections between the osteocytes. These include high resolution micro-computed tomography, second harmonic generation, and confocal microscopy when combined with staining (34). In addition to the LCN shape, the osteocyte cell can be imaged using a combination of staining and confocal microscopy. Recent research has utilized green fluorescence protein (GFP)-labeled osteocytes and other dyes to image cellular aspects of the osteocyte such as the cytoskeleton along with the dendritic connections in relation to the surrounding collagen (35). This approach has yielded some evidence that vesicles may be released by the osteocyte as it embeds itself within the LCN and that collagen may be produced by the osteocyte. It is important to note that quantifying the number of lacunae is not the same as quantifying occupied lacunae (36). After an osteocyte dies, the lacunae will remain empty until it is gradually filled with mineralized debris.
Quantitative analysis of the LCN structure must be done to determine if alterations to the shape and organization of the network are occurring. Lacunar area or volume, lacunar density, canalicular length, and canalicular density are some of the measurements that can be made to quantify changes. The LCN can also be quantified in a manner similar to quantification of the connectivity of the trabecular bone network. This is essentially a measure of how many connections would have to be broken to separate the network into two (37). Additionally, the LCN can be analyzed in terms of connectomics. In this analysis, the LCN is considered as a system of nodes linked together by edges. Nodes can be either lacunae or places where at least three canaliculi connect. This analysis could be useful to determine how the organization of the LCN affects the osteocyte’s ability to communicate with other cells and respond to loading. Nodal centers with higher numbers of connections may indicate fewer and more utilized routes of communication. Connectomics analysis has been reviewed in depth elsewhere (38). Less work has been done using connectomics analysis, but there have been some studies that have utilized this technique. Mabilleau et al. have indicated that high fat diet caused an increase in node degree in mice (39) Additionally, connectomics analysis has been used to analyze differences in the LCN structure between sheep and mouse bone, albeit on a limited number of samples (40). The network of sheep bone was more regularly organized but less connected than mouse bone, but properties such as edges per node and edge length were similar between species.
Changes to the LCN have been observed based on the organization of the surrounding matrix, during aging, disease, and in response to environmental factors. More spherical lacunae are likely to be found in woven bone versus the more organized lamellar bone (41). Osteocytes have been seen to elongate perpendicular to the long axis of bones in amphibians, reptiles, and mammals (42). High fat diet caused an increase in lacunar area in mice (39). The LCN also changes with aging, as lacunae become flatter and the canaliculi become more interconnected with maturity, a trend that reverses once bone is aged (43). There are changes to the LCN in osteogenesis imperfecta (OI) as OI mice have been observed to have more spherical lacunae with more canaliculi than wild-type mice (44). Mechanical unloading also results in changes to the LCN. Sciatic neurectomy to immobilize one limb in growing rats resulted in lower lacunar density and volume (45). Similarly, growing mice were found to have a reduced cell volume and number of processes with sciatic neurectomy in both cortical and cancellous bone (46). It is important to note that these experiments were both done in growing rodents. Immobilized female patients had a lower osteocyte density and reduced connectivity than postmenopausal controls (47). Fluid flow as determined by finite element analysis (FEA) was shown to decrease in ovariectomized rats that had lower lacunar density and porosity (33).
The LCN may have a direct effect on bone quality. In cases of spaceflight where the lacunar volume was shown to decrease and become more spherical, nanoindentation indicated that the hardness and stiffness of the matrix also decreased (48). Another study used nanoindentation to assess the area close to (1 to 5 µm) and further away from the lacunae (16 to 20 µm) in ovariectomized rats with treatment. While there were no differences between treatment groups (PTH, alendronate, raloxifene, PTH and alendronate, and PTH and raloxifene), Young’s modulus was lower in the perilacunar region compared to the area further away (49). Modulus was also higher further away from the lacunae and canaliculi in healthy 4-month old female rats (50). Mounting evidence supports that actions coordinated by osteocytes in the LCN directly impact matrix quality.
The pericellular matrix (PCM) surrounds the osteocyte and separates the cell from the walls of the lacunae and canaliculi. This matrix contains proteoglycans and hyaluronic acid and may amplify the impacts of mechanical loading to allow osteocyte to sense more load than what would be calculated by tissue strain alone. Tethering elements between the matrix wall and the cells that could amplify force through shear drag forces in response to fluid flow were first postulated with computational modeling (51) prior to transverse elements between the matrix wall and cell being visually confirmed with TEM imaging (52). Perlecan has been speculated to form the tethering elements in the PCM. MLO-Y4 cells express perlecan protein and immunogold labeling indicated the presence of perlecan along the osteocyte bodies and walls of the canliculi (53). Perlecan deficient mice have shown higher solute diffusivity, but lacked the anabolic response to in vivo tibial loading (54) indicating its importance for mechanical sensing (54). Additionally, integrins have been speculated to form part of the PCM and affect the osteocyte response to mechanical stimulation. TEM images have indicated the canalicular walls may have protrusions into the pericellular space (55, 56). A theoretical model incorporating tethering elements along with focal adhesion complexes mathematically predicted a high amplification of strain that was an order of magnitude higher than previous strain amplification models (56). This focal adhesion complex has been speculated to be β3-integrin as immunohistochemistry has indicated the presence of β3-integrin along the walls of canaliculi of murine cortical bone (55). In vitro, inhibition of αvβ3 integrin attachment sites in MLO-Y4 cells reduced the Ca2+ response to probe stimulation (57). Structured Illumination Super Resolution Microscopy has found membrane proteins associated with mechanotransduction to be colocalized with β3-integrin foci in vivo, though this did not find a colocalization with connexin 43 (58). The PCM may also alter with age. Osteocytes isolated from aged mice were able to produce less PCM than osteocytes from young mice in vitro. Aged cells also had fewer plasma membrane disruptions than young cells in response to fluid shear stress, indicating the mechanical response may be dependent on the PCM (59). This is an aspect of osteocyte control of the environment that needs to be further studied.
Due to their preference of remaining embedded within the bone matrix, osteocytes have proven difficult to study when removed from their natural enclosure. Indeed, studies on primary osteocytes have demonstrated complications including low yield, poor viability when grown in 2D culture, and limited dendrite formation. Therefore, most studies to date have utilized immortalized cellular models of osteocytes. These cell lines represent various stages of the osteocyte life cycle, including late transitioning osteoblasts, early osteocytes, late osteocytes, and lines that gradually differentiate through all three stages. Despite being derived from osteocytes, each cell line responds differently to mechanical, endocrine, and paracrine signals. Therefore, we will begin our analysis of osteocyte matrix modeling and remodeling by examining what has been learned using in vitro models.
The most frequently used cell line in osteocyte research is the MLO-Y4 line. These cells were derived from the long bones of female mice and immortalized using an SV40 T-cell antigen coupled to the osteocalcin promoter (60). MLO-Y4 cells are mechanosensitive, as studies utilizing fluid shear stress have demonstrated robust increases in intracellular calcium currents, ATP production, and release of prostaglandin E2 (PGE2) and nitric oxide (NO) (61–63), all of which are essential components of the osteocyte response to mechanical stimulation. Additionally, they express large amounts of connexin 43 (Cx43) and produce a dendritic network. In response to short-term unidirectional and oscillatory fluid flow, MLO-Y4 cells increase RANKL expression while greatly increasing OPG expression, resulting in a decrease in the RANKL/OPG ratio (64, 65). This finding may indicate that osteocytes respond to loading by reducing osteoclast activity through paracrine signaling. Importantly, MLO-Y4 cells do not typically express Sost, a potent inhibitor of bone formation by osteoblasts. This shortcoming is also noted in MLO-A5 cells, a model of late transitioning osteoblasts (66). Interestingly, long-term fluid shear may increase Sost expression in MLO-Y4 cells despite their lack of natural Sost expression, although conflicting evidence exists (67, 68). In terms of anabolic functions, conditioned media taken from MLO-Y4 cultures increases alkaline phosphatase (34) and osteocalcin (OCN) expression in osteoblasts, indicating the presence of additional secreted factors that increase osteoblast activity (69).
Two models of differentiated osteocytes are the Ocy454 and IDG-SW3 cell lines. Each of these lines utilizes an interferon-γ-driven T-cell antigen promoter to induce immortalization followed by temperature-driven differentiation. The mechanical response of Ocy454 cells to fluid shear are more variable than MLO-Y4 cells, with fewer cells demonstrating increased calcium currents with occasional high magnitude calcium waves (70). Unlike MLO-Y4 cells, Ocy454 osteocytes express abundant DMP1 and Sost, and Sost expression can be lowered by fluid shear (68). Increasing the duration of fluid shear gradually increases Sost expression and the RANKL/OPG ratio in both lines (68). Like Ocy454 cells, the IDG-SW3 cell line expresses classic osteocytic genes when they reach maturity. In the early stages of differentiation, IDG-SW3 cells express osteoblastic genes including ALP and type I collagen (col1a1) (71). As they transition into early osteocytes, dentin matrix protein 1 (DMP1), matrix extracellular phosphoglycoprotein (MEPE), and phosphate-regulating neutral endopeptidase (Phex) levels increase (71). Finally, as late osteocytes, IDG-SW3 cells begin expressing high levels of Sost and fibroblast growth factor 23 (FGF23), demonstrating their utility in studying osteocyte paracrine and endocrine signaling (71).
While osteoblasts and osteoclasts are the classic cell types involved with forming and shaping bone, emerging research has demonstrated that many of the cues that drive these cells may also trigger osteocytes to participate in these functions. The process of bone matrix alteration by osteocytes is currently known as perilacunar remodeling (PLR), a concept that is gaining popularity in the bone community. Utilizing the idea that osteocytes also modify their activity in response to cues that would normally change bone mass, we next examine how bone-altering signals may modify osteocyte function to alter their surrounding extracellular matrix using the aforementioned in vitro models.
One of the most important signals the bone receives is from parathyroid hormone (PTH), a peptide hormone secreted from the parathyroid gland in response to low serum calcium. In addition to increasing calcium absorption from the intestine, sustained elevations in PTH are known to cause mineral release from the bone, as seen in hypercalcemia of malignancy and chronic kidney disease (72, 73). Studies using IDG-SW3 cells have demonstrated that PTH upregulates ATPase H+ Transporting V0 Subunit D2 (ATP6V0D2), a proton pump on the cell membrane that acidifies the extracellular environment, indicating that osteocytes can acidify their extracellular environment to degrade mineral (74). PTH-related Peptide (PTHrP) has also been shown to stimulate acidification of the osteocyte extracellular environment by upregulating ATP6V0D2 during lactation, and this process is dependent on intact PTH signaling in osteocytes (75). IDG-SW3 cells naturally upregulate several osteoclastic genes throughout their 28-day differentiation including tartrate-resistant acid phosphatase (TRAP), carbonic anhydrase I and II (CA1/2), and cathepsin K (CTSK), indicating that mature osteocytes are poised to participate in PLR (76). While matrix acidification is required for mineral removal, it also promotes the collagenolytic activity of CTSK, indicating that osteocytes can degrade both mineral and collagen (77, 78). In addition to PTH, Sost signaling has also been shown to upregulate TRAP, CA, and CTSK in neighboring MLO-Y4 osteocytes (79). Therefore, osteocytes may increase the bone resorbing activity of nearby osteocytes in addition to reducing osteoblast activity via Sost signaling (76, 79). Finally, TGFβ also upregulates several osteoclastic genes via the YAP/TAZ signaling pathway in MLO-Y4 and Ocy454 cells. In MLO-Y4 cells, treatment with TGFβ results in extracellular acidification and upregulation of CTSK and matrix metalloproteinase 13 and 14 (MMP13/14) while glucocorticoid treatment decreases MMP13 expression (80). A similar finding was shown in Ocy454 cells, which upregulated CTSK and MMP14, but not MMP13 (78). While these two osteocyte models differ slightly in their responses, they each suggest that osteocytes participate in matrix remodeling by adopting an osteoclast-like phenotype.
As mentioned above, mechanical loading alters osteocyte signaling to osteoblasts and osteoclasts. However, whether loading influences the process of PLR remains unclear. When fluid shear stress is applied to MLO-Y4 cells, increased E11/gp38 expression drives increased dendrite formation and elongation (80). For this process to occur in vivo, however, osteocyte dendrites must express genes that allow them to degrade local mineral and collagen to extend through the bone. Indeed, a recent study seeding IDG-SW3 cells into an MMP-sensitive hydrogel demonstrated increased dendricity, Cx43, and MMPs 2 and 13 throughout differentiation (81). These cells also maintained elevated ALP expression through day 28 of differentiation while ALP expression diminishes in 2D culture. Another study in 3D culture demonstrated that MLO-Y4 cells display increased col1a1 expression over time (82). Therefore, 3D culture models may be necessary to capture the ability for osteocytes to form matrix components. However, studies examining perilacunar modeling and remodeling by osteocytes in 3D cell culture with loading or other physiologic stimuli remain to be performed. Altogether, these in vitro findings suggest that bone-forming osteoblasts can differentiate into mechanosensitive osteocytes that coordinate the activities of osteoblasts and osteoclasts, and eventually gain osteoclastic resorptive abilities. Strikingly, while osteocytes reduce their osteoblastic activity over time, these functions are not entirely lost as they mature in a 3D environment. Therefore, further research probing the ability for osteocytes to form mineral and collagen are imperative to understand the contribution of osteocytes to the microstructure and overall integrity of bone.
Osteocyte modification of the mineral in the surrounding matrix has been observed in cases where PLR removes mineral such as in lactation (83) and hibernation (84), and lack of PLR can result in hypermineralization such as in the case of exposure to microgravity (48). This has been supported by changes to lacunar area. In the case of lactation, it has been suggested that the osteocyte can also replace the mineral in its surrounding bone if recovery after weaning is allowed, as double fluorochrome labeling has indicated new mineral formation around the osteocyte (75). The osteocyte can alter the overall porosity of bone by either removing or adding mineral to its lacunae. The osteocyte network appears to influence the quality of the mineral as well. Using small angle X-ray scattering (SAXS) combined with confocal microscopy, a study showed that in areas with a high density of osteocytes that were well aligned, the mineral platelet thickness and particle orientation was higher than is less organized areas (85). The mineral thickness and particle orientation were lower in the areas closer to the lacunae themselves, indicating that the osteocytes may control the quality of the mineral over time (85). In another study looking at mice that underwent treadmill running, the mineral to matrix ratio (MMR) of the matrix surrounding the osteocyte was lower than the MMR of the matrix further away, indicating the osteocyte altering its bone matrix (86). Interestingly, mice that underwent treadmill running and showed lower MMR in their perilacunar region had higher post-yield work in bending tests of their tibiae, indicating that PLR may improve bone’s overall mechanical properties (86). An effect on mechanical properties has also been observed elsewhere as the elastic modulus as measured by microindentation of the bone decreased with lactation (87). Thus, changes to the mineral by the osteocyte may affect overall bone quality.
Type I collagen is the most prevalent organic component of the bone extracellular matrix and provides the tissue with tensile ductility and fracture toughness by limiting crack formation and propagation (88–90). Collagen is primarily produced by osteoblasts during bone formation alongside mineral. The helical structure of collagen is composed of Gly-X-Y repeats where X and Y are typically proline and hydroxyproline, respectively (91). Collagen consists of two pro-α1 and one pro-α2 peptide chains that are translated by ribosomes embedded within the endoplasmic reticulum (ER) membrane. Next, post-translational modifications including hydroxylation of proline and lysine residues and glycosylation of some prolines occurs within the ER. The chains twist into a triple helix and are shuttled to the Golgi apparatus as procollagen. Upon secretion from the osteoblast, the N- and C-terminal domains are cleaved, forming tropocollagen. Finally, tropocollagen strands self-assemble into fibrils and neighboring tropocollagen molecules are crosslinked at their hydroxylysine residues by lysyl oxidase, stabilizing the fibrillar structure (92).
The overall quality of collagen is dependent on the correct level of post-translational modifications, proper crosslinking, incorporation into the bone, and alignment within the bone tissue. Importantly, the alignment of collagen fibrils is related to the types of loads that each bone experiences. During physiologic loading of the lower limb, the anterior portion of the femur and tibia typically experiences tension while the posterior portion is under compression (93). Studies utilizing polarized light microscopy have determined that collagen fibrils tend to be aligned perpendicular to transverse sections of bones under tension while they are aligned parallel to transverse sections in compressive regions. Intriguingly, collagen fibrils tend to align with the major axis of osteocytes and their lacunae (41). Additionally, it has been shown that osteoblasts initially secrete disorganized collagen that eventually aligns with the osteoblast major axis or the axis under the greatest mechanical strain (94, 95).
While osteoblasts follow mechanical cues from their environment to determine collagen orientation and placement, mechanosensory cues from osteocytes may also be required to instruct osteoblast collagen deposition. Further, it stands that osteocytes themselves may be responsible for forming and aligning collagen in the perilacunar region. One of the earliest studies examining this possibility placed bones from egg-laying hens in media containing radiolabeled proline, a highly prevalent amino acid in all collagens (96). In hens fed a calcium-rich diet after egg laying, it was reported that osteoblasts and osteocytes demonstrated widespread uptake of proline, indicating that osteocytes may replenish matrix collagen following lactation (97). Modern intravital imaging studies have also demonstrated that early osteocytes may be able to synthesize parts of the collagen matrix surrounding their lacunae while also exerting mechanical forces on the existing collagen matrix (8). Eventually, this process results in a collagenous matrix that aligns with the major axis of osteocyte lacunae, but whether this process is mechanically driven remains unknown.
As discussed earlier, in vitro models of osteocytes have the capacity to degrade collagen in response to catabolic stimuli including PTH. The importance of this finding has also been established in vivo, as lactating mice fail to resorb mineral from their lacunae if collagen degrading genes including CTSK and MMP-13 are knocked out in osteocytes (98, 99). Therefore, collagen degradation is an essential step in perilacunar remodeling. Additionally, MMP-13 expression by osteocytes is critical to maintenance of bone fracture toughness, or the ability of bone to resist crack formation and propagation, a property that is highly dependent on proper collagen incorporation and crosslinking (99, 100). There are implications that TGF-β may be involved as well. It has been demonstrated that inhibiting TGF-β receptor pharmacologically in mice resulted in a reduction of gene expression of genes associated with PLR and reduced canaliculi length (76). The same study examined a knock-out mouse of osteocyte specific TGF-β receptor in bone which resulted in a similar decline in PLR gene expression and decrease in canalicular length and lacunar–canalicular area. Fracture resistance was notably lower in the knock-out mice (76). Taken together, there is striking preliminary evidence to warrant a deeper investigation of the osteocytes ability to modify, align, and produce collagen within their lacunae. Future work may require the use of 3D scaffolds in order to capture these effects in vitro while in vivo studies will likely benefit from the use of emerging techniques to analyze bone composition and mechanical properties on the microscale in animal models of post-lactation recovery, space flight, and other instances of mineral challenge. Furthermore, while collagen is the most prevalent protein in bone, genetic knockouts of non-collagenous proteins (NCPs) including biglycan, fibrillin-2, and bone sialoprotein among others have demonstrated altered microarchitecture and/or reduced mechanical properties (101–103). Biglycan in particular is required for proper collagen assembly into organized fibrils, and knockouts resemble the phenotype of Ehlers–Danlos syndrome (104). However, material tests such as fracture toughness testing and tissue-level analyses have largely not been performed on these genetic models. While NCPs are known to impact bone formation, whether osteocytes can interact with and alter NCPs, or whether NCPs control the ability for osteocytes to model and remodel their surrounding matrix remains largely unknown. Taken together, understanding the full extent of the osteocytes capabilities will require a combination of robust cellular models, modern imaging modalities, and tissue-level analyses that can distinguish material, structural, and compositional properties on the micro- and nano-scales in and around the LCN, enhancing our ability to devise new treatments for bone diseases.
The osteocyte has a profound effect on the bone matrix through signaling to osteoblasts and osteoclasts and by directly modifying its environment (Figure 1). The structure of the LCN relates to the structure and quality of the surrounding matrix. There is also in vitro and in vivo evidence indicating the osteocyte can directly modify mineral and collagen in its surroundings. Thus, the osteocyte must be considered when examining the effects of disease and treatments on the bone matrix.
Figure 1 Role of Osteocytes in Modifying Bone Matrix Properties. (A) Mechanical loading and wearing are known to increased bone formation, and osteocytes are known to play a critical role in each. While a definite pathway leading to bone formation by osteocytes remains to be discovered, a small amount of bone formation by osteocytes could have a profound effect on bone material properties. (B) Perilacunar remodeling by osteocytes occurs in response to unloading and lactation, processes in which PTH is a major player. This results in decreased pH in the perilacunar space and increased expression of collagenolytic enzymes. Figure created with BioRender.com.
AC, JD, and JW planned and wrote the document. All authors contributed to the article and approved the submitted version.
This work was supported by the National Institutes of Health [JW: AR072609; AC: AR065971; JD: DK121399].
The authors declare that the research was conducted in the absence of any commercial or financial relationships that could be construed as a potential conflict of interest.
The reviewer CW declared a shared affiliation with one of the authors, JD, to the handling editor at time of review.
1. Manolagas SC. Birth and Death of Bone Cells: Basic Regulatory Mechanisms and Implications for the Pathogenesis and Treatment of Osteoporosis*. Endocrine Rev (2000) 21:115–37. doi: 10.1210/er.21.2.115
2. Jilka RL, Weinstein RS, Bellido T, Parfitt AM, Manolagas SC. Osteoblast Programmed Cell Death (Apoptosis): Modulation by Growth Factors and Cytokines. J Bone Mineral Res (1998) 13:793–802. doi: 10.1359/jbmr.1998.13.5.793
3. Wetterwald A, Hofstetter W, Cecchini MG, Lanske B, Wagner C, Fleisch H, et al. Characterization and cloning of the E11 antigen, a marker expressed by Rat Osteoblasts and Osteocytes. Bone (1996) 18:125–32. doi: 10.1016/8756-3282(95)00457-2
4. Karsdal MA, Andersen TA, Bonewald L, Christiansen C. Matrix Metalloproteinases (MMPs) Safeguard Osteoblasts from Apoptosis during Transdifferentiation into Osteocytes: MT1-MMP Maintains Osteocyte Viability. DNA Cell Biol (2004) 23:155–65. doi: 10.1089/104454904322964751
5. Holmbeck K, Bianco P, Pidoux I, Inoue S, Billinghurst RC, Wu W, et al. The metalloproteinase MT1-MMP is required for normal development and maintenance of osteocyte processes in bone. J Cell Sci (2005) 118:147. doi: 10.1242/jcs.01581
6. Palumbo C, Palazzini S, Zaffe D, Marotti G. Osteocyte differentiation in the tibia of newborn rabbit: an ultrastructural study of the formation of cytoplasmic processes. Acta Anat (Basel) (1990) 137:350–8. doi: 10.1159/000146907
7. Franz-Odendaal TA, Hall BK, Witten PE. Buried alive: how osteoblasts become osteocytes. Dev Dyn (2006) 235:176–90. doi: 10.1002/dvdy.20603
8. Shiflett LA, Tiede-Lewis LM, Xie Y, Lu Y, Ray EC, Dallas SL. Collagen Dynamics During the Process of Osteocyte Embedding and Mineralization. Front Cell Dev Biol (2019) 7:178. doi: 10.3389/fcell.2019.00178
9. Eriksen EF. Normal and Pathological Remodeling of Human Trabecular Bone: Three Dimensional Reconstruction of the Remodeling Sequence in Normals and in Metabolic Bone Disease*. Endocrine Rev (1986) 7:379–408. doi: 10.1210/edrv-7-4-379
10. Wodarz A, Nusse R. MECHANISMS OF WNT SIGNALING IN DEVELOPMENT. Annu Rev Cell Dev Biol (1998) 14:59–88. doi: 10.1146/annurev.cellbio.14.1.59
11. Li VS, Ng SS, Boersema PJ, Low TY, Karthaus WR, Gerlach JP, et al. Wnt Signaling through Inhibition of β-Catenin Degradation in an Intact Axin1 Complex. Cell (2012) 149:1245–56. doi: 10.1016/j.cell.2012.05.002
12. Poole KES, Van Bezooijen RL, Loveridge N, Hamersma H, Papapoulos SE, Löwik CW, et al. Sclerostin is a delayed secreted product of osteocytes that inhibits bone formation. FASEB J (2005) 19:1842–4. doi: 10.1096/fj.05-4221fje
13. Bezooijen R, Dijke P.t., Papapoulos SE, Löwik CW. SOST/sclerostin, an osteocyte-derived negative regulator of bone formation. Cytokine Growth Factor Rev (2005) 16:319–27. doi: 10.1016/j.cytogfr.2005.02.005
14. Truswell AS. OSTEOPETROSIS WITH SYNDACTYLY. J Bone Joint Surg (1958) British volume 40-B:208–18. doi: 10.1302/0301-620X.40B2.208
15. Honma M, Ikebuchi Y, Kariya Y, Hayashi M, Hayashi N, Aoki S, et al. RANKL subcellular trafficking and regulatory mechanisms in osteocytes. J Bone Mineral Res (2013) 28:1936–49. doi: 10.1002/jbmr.1941
16. Nakashima T, Hayashi M, Fukunaga T, Kurata K, Oh-hora M, Feng JQ, et al. Evidence for osteocyte regulation of bone homeostasis through RANKL expression. Nat Med (2011) 17:1231–4. doi: 10.1038/nm.2452
17. Xiong J, Onal M, Jilka RL, Weinstein RS, Manolagas SC, O’Brien CA. Matrix-embedded cells control osteoclast formation. Nat Med (2011) 17:1235–41. doi: 10.1038/nm.2448
18. Lacey DL, Timms E, Tan HL, Kelley MJ, Dunstan CR, Burgess T, et al. Osteoprotegerin Ligand Is a Cytokine that Regulates Osteoclast Differentiation and Activation. Cell (1998) 93:165–76. doi: 10.1016/S0092-8674(00)81569-X
19. Delmas PD. Clinical Potential of RANKL Inhibition for the Management of Postmenopausal Osteoporosis and Other Metabolic Bone Diseases. J Clin Densitometry (2008) 11:325–38. doi: 10.1016/j.jocd.2008.02.002
20. Boyle WJ, Simonet WS, Lacey DL. Osteoclast differentiation and activation. Nature (2003) 423:337–42. doi: 10.1038/nature01658
21. Hofbauer LC, Schoppet M. Clinical Implications of the Osteoprotegerin/RANKL/RANK System for Bone and Vascular Diseases. JAMA (2004) 292:490–5. doi: 10.1001/jama.292.4.490
22. Kennedy OD, Lendhey M, Mauer P, Philip A, Basta-Pljakic J, Schaffler MB. Microdamage induced by in vivo Reference Point Indentation in mice is repaired by osteocyte-apoptosis mediated remodeling. Bone (2017) 95:192–8. doi: 10.1016/j.bone.2016.11.029
23. Emerton KB, Hu B, Woo AA, Sinofsky A, Hernandez C, Majeska RJ, et al. Osteocyte apoptosis and control of bone resorption following ovariectomy in mice. Bone (2010) 46:577–83. doi: 10.1016/j.bone.2009.11.006
24. McCutcheon S, Majeska RJ, Spray DC, Schaffler MB, Vazquez M. Apoptotic Osteocytes Induce RANKL Production in Bystanders via Purinergic Signaling and Activation of Pannexin Channels. J Bone Miner Res (2020) 35:966–77. doi: 10.1002/jbmr.3954
25. Hopkinson JM, Butte NF, Ellis K, Smith EOB. Lactation Delays Postpartum Bone Mineral Accretion and Temporarily Alters Its Regional Distribution in Women. J Nutr (2000) 130:777–83. doi: 10.1093/jn/130.4.777
26. Ardeshirpour L, Dann P, Adams DJ, Nelson T, VanHouten J, Horowitz MC, et al. Weaning Triggers a Decrease in Receptor Activator of Nuclear Factor-κB Ligand Expression, Widespread Osteoclast Apoptosis, and Rapid Recovery of Bone Mass after Lactation in Mice. Endocrinology (2007) 148:3875–86. doi: 10.1210/en.2006-1467
27. Marotti G, Ferretti M, Remaggi F, Palumbo C. Quantitative evaluation on osteocyte canalicular density in human secondary osteons. Bone (1995) 16:125–8. doi: 10.1016/8756-3282(95)80022-I
28. Wang B, Zhou X, Price C, Li W, Pan J, Wang L. Quantifying load-induced solute transport and solute-matrix interaction within the osteocyte lacunar-canalicular system. J Bone Mineral Res (2013) 28:1075–86. doi: 10.1002/jbmr.1804
29. Robling AG, Bonewald LF. The Osteocyte: New Insights. Annu Rev Physiol (2020) 82:485–506. doi: 10.1146/annurev-physiol-021119-034332
30. Ciani C, Sharma D, Doty SB, Fritton SP. Ovariectomy enhances mechanical load-induced solute transport around osteocytes in rat cancellous bone. Bone (2014) 59:229–34. doi: 10.1016/j.bone.2013.11.026
31. Fan L, Pei S, Lucas Lu X, Wang L. A multiscale 3D finite element analysis of fluid/solute transport in mechanically loaded bone. Bone Res (2016) 4:16032. doi: 10.1038/boneres.2016.32
32. Joukar A, Niroomand-Oscuii H, Ghalichi F. Numerical simulation of osteocyte cell in response to directional mechanical loadings and mechanotransduction analysis: Considering lacunar-canalicular interstitial fluid flow. Comput Methods Progr Biomed (2016) 133:133–41. doi: 10.1016/j.cmpb.2016.05.019
33. Gatti V, Azoulay EM, Fritton SP. Microstructural changes associated with osteoporosis negatively affect loading-induced fluid flow around osteocytes in cortical bone. J Biomechanics (2018) 66:127–36. doi: 10.1016/j.jbiomech.2017.11.011
34. Schneider P, Meier M, Wepf R, Müller R. Towards quantitative 3D imaging of the osteocyte lacuno-canalicular network. Bone (2010) 47:848–58. doi: 10.1016/j.bone.2010.07.026
35. Kamel-ElSayed SA, Tiede-Lewis LM, Lu Y, Veno PA, Dallas SL. Novel approaches for two and three dimensional multiplexed imaging of osteocytes. Bone (2015) 76:129–40. doi: 10.1016/j.bone.2015.02.011
36. Qiu S, Rao DS, Palnitkar S, Parfitt AM. Reduced Iliac Cancellous Osteocyte Density in Patients With Osteoporotic Vertebral Fracture. J Bone Mineral Res (2003) 18:1657–63. doi: 10.1359/jbmr.2003.18.9.1657
37. Odgaard A, Gundersen HJG. Quantification of connectivity in cancellous bone, with special emphasis on 3-D reconstructions. Bone (1993) 14:173–82. doi: 10.1016/8756-3282(93)90245-6
38. Weinkamer R, Kollmannsberger P, Fratzl P. Towards a Connectomic Description of the Osteocyte Lacunocanalicular Network in Bone. Curr Osteoporosis Rep (2019) 17:186–94. doi: 10.1007/s11914-019-00515-z
39. Mabilleau G, Perrot R, Flatt PR, Irwin N, Chappard D. High fat-fed diabetic mice present with profound alterations of the osteocyte network. Bone (2016) 90:99–106. doi: 10.1016/j.bone.2016.06.008
40. Kollmannsberger P, Kerschnitzki M, Repp F, Wagermaier W, Weinkamer R, Fratzl P. The small world of osteocytes: connectomics of the lacuno-canalicular network in bone. New J Phys (2017) 19:073019. doi: 10.1088/1367-2630/aa764b
41. Kerschnitzki M, Wagermaier W, Roschger P, Seto J, Shahar R, Duda GN, et al. The organization of the osteocyte network mirrors the extracellular matrix orientation in bone. J Struct Biol (2011) 173:303–11. doi: 10.1016/j.jsb.2010.11.014
42. Cao L, Moriishi T, Miyazaki T, Iimura T, Hamagaki M, Nakane A, et al. Comparative morphology of the osteocyte lacunocanalicular system in various vertebrates. J Bone Mineral Metab (2011) 29:662–70. doi: 10.1007/s00774-011-0268-6
43. Okada S, Yoshida S, Ashrafi SH, Schraufnagel DE. The Canalicular Structure of Compact Bone in the Rat at Different Ages. Microscopy Microanalysis (2002) 8:104–15. doi: 10.1017/S1431927601020037
44. Carriero A, Doube M, Vogt M, Busse B, Zustin J, Levchuk A, et al. Altered lacunar and vascular porosity in osteogenesis imperfecta mouse bone as revealed by synchrotron tomography contributes to bone fragility. Bone (2014) 61:116–24. doi: 10.1016/j.bone.2013.12.020
45. Britz HM, Carter Y, Jokihaara J, Leppänen OV, Järvinen TLN, Belev G, et al. Prolonged unloading in growing rats reduces cortical osteocyte lacunar density and volume in the distal tibia. Bone (2012) 51:913–9. doi: 10.1016/j.bone.2012.08.112
46. Sugawara Y, Kamioka H, Ishihara Y, Fujisawa N, Kawanabe N, Yamashiro T. The early mouse 3D osteocyte network in the presence and absence of mechanical loading. Bone (2013) 52:189–96. doi: 10.1016/j.bone.2012.09.033
47. Rolvien T, Milovanovic P, Schmidt FN, von Kroge S, Wölfel EM, Krause M, et al. Long-Term Immobilization in Elderly Females Causes a Specific Pattern of Cortical Bone and Osteocyte Deterioration Different From Postmenopausal Osteoporosis. J Bone Mineral Res N/A (2020) 35(7):1343–51. doi: 10.1002/jbmr.3970
48. Gerbaix M, Gnyubkin V, Farlay D, Olivier C, Ammann P, Courbon G, et al. One-month spaceflight compromises the bone microstructure, tissue-level mechanical properties, osteocyte survival and lacunae volume in mature mice skeletons. Sci Rep (2017) 7:2659. doi: 10.1038/s41598-017-09608-0
49. Stern AR, Yao X, Wang Y, Berhe A, Dallas M, Johnson ML, et al. Effect of osteoporosis treatment agents on the cortical bone osteocyte microenvironment in adult estrogen-deficient, osteopenic rats. Bone Rep (2018) 8:115–24. doi: 10.1016/j.bonr.2018.02.005
50. Zhang S, Bach-Gansmo FL, Xia D, Besenbacher F, Birkedal H, Dong M. Nanostructure and mechanical properties of the osteocyte lacunar-canalicular network-associated bone matrix revealed by quantitative nanomechanical mapping. Nano Res (2015) 8:3250–60. doi: 10.1007/s12274-015-0825-8
51. Weinbaum S, Cowin SC, Zeng Y. A model for the excitation of osteocytes by mechanical loading-induced bone fluid shear stresses. J Biomechanics (1994) 27:339–60. doi: 10.1016/0021-9290(94)90010-8
52. You L-D, Weinbaum S, Cowin SC, Schaffler MB. Ultrastructure of the osteocyte process and its pericellular matrix. Anatomical Record Part A: Discov Mol Cell Evolutionary Biol (2004) 278A:505–13. doi: 10.1002/ar.a.20050
53. Thompson WR, Modla S, Grindel BJ, Czymmek KJ, Kirn-Safran CB, Wang L, et al. Perlecan/Hspg2 deficiency alters the pericellular space of the lacunocanalicular system surrounding osteocytic processes in cortical bone. J Bone Mineral Res (2011) 26:618–29. doi: 10.1002/jbmr.236
54. Wang B, Lai X, Price C, Thompson WR, Li W, Quabili TR, et al. Perlecan-Containing Pericellular Matrix Regulates Solute Transport and Mechanosensing Within the Osteocyte Lacunar-Canalicular System. J Bone Mineral Res (2014) 29:878–91. doi: 10.1002/jbmr.2105
55. McNamara LM, Majeska RJ, Weinbaum S, Friedrich V, Schaffler MB. Attachment of Osteocyte Cell Processes to the Bone Matrix. Anatomical Record (2009) 292:355–63. doi: 10.1002/ar.20869
56. Wang Y, McNamara LM, Schaffler MB, Weinbaum S. A model for the role of integrins in flow induced mechanotransduction in osteocytes. Proc Natl Acad Sci U S A (2007) 104:15941–6. doi: 10.1073/pnas.0707246104
57. Thi MM, Suadicani SO, Schaffler MB, Weinbaum S, Spray DC. Mechanosensory responses of osteocytes to physiological forces occur along processes and not cell body and require αVβ3 integrin. Proc Natl Acad Sci U S A (2013) 110:21012–7. doi: 10.1073/pnas.1321210110
58. Cabahug-Zuckerman P, Stout RF Jr, Majeska RJ, Thi MM, Spray DC, Weinbaum S, et al. Potential role for a specialized β3 integrin-based structure on osteocyte processes in bone mechanosensation. J Orthopaedic Res (2018) 36:642–52. doi: 10.1002/jor.23792
59. Hagan ML, Yu K, Zhu J, Vinson BN, Roberts RL, Montesinos Cartagena M, et al. Decreased pericellular matrix production and selection for enhanced cell membrane repair may impair osteocyte responses to mechanical loading in the aging skeleton. Aging Cell (2020) 19:e13056–6. doi: 10.1111/acel.13056
60. Kato Y, Windle JJ, Koop BA, Mundy GR, Bonewald LF. Establishment of an Osteocyte-like Cell Line, MLO-Y4. J Bone Mineral Res (1997) 12:2014–23. doi: 10.1359/jbmr.1997.12.12.2014
61. Kamel MA, Picconi JL, Lara-Castillo N, Johnson ML. Activation of β-catenin signaling in MLO-Y4 osteocytic cells versus 2T3 osteoblastic cells by fluid flow shear stress and PGE2: Implications for the study of mechanosensation in bone. Bone (2010) 47:872–81. doi: 10.1016/j.bone.2010.08.007
62. Rath AL, Bonewald LF, Ling J, Jiang JX, Van Dyke ME, Nicolella DP. Correlation of cell strain in single osteocytes with intracellular calcium, but not intracellular nitric oxide, in response to fluid flow. J Biomechanics (2010) 43:1560–4. doi: 10.1016/j.jbiomech.2010.01.030
63. Genetos DC, Kephart CJ, Zhang Y, Yellowley CE, Donahue HJ. Oscillating fluid flow activation of gap junction hemichannels induces atp release from MLO-Y4 osteocytes. J Cell Physiol (2007) 212:207–14. doi: 10.1002/jcp.21021
64. You L, Temiyasathit S, Lee P, Kim CH, Tummala P, Yao W, et al. Osteocytes as mechanosensors in the inhibition of bone resorption due to mechanical loading. Bone (2008) 42:172–9. doi: 10.1016/j.bone.2007.09.047
65. Li J, Rose E, Frances D, Sun Y, You L. Effect of oscillating fluid flow stimulation on osteocyte mRNA expression. J Biomech (2012) 45:247–51. doi: 10.1016/j.jbiomech.2011.10.037
66. Yang D, Gronthos S, Isenmann S, Morris HA, Atkins GJ. The Late Osteoblast/Preosteocyte Cell Line MLO-A5 Displays Mesenchymal Lineage Plasticity In Vitro and In Vivo. Stem Cells Int (2019) 2019:9838167. doi: 10.1155/2019/9838167
67. Li X, Liu C, Li P, Li S, Zhao Z, Chen Y, et al. Connexin 43 is a potential regulator in fluid shear stress-induced signal transduction in osteocytes. J Orthopaedic Res (2013) 31:1959–65. doi: 10.1002/jor.22448
68. Spatz JM, Wein MN, Gooi JH, Qu Y, Garr JL, Liu S, et al. The Wnt Inhibitor Sclerostin Is Up-regulated by Mechanical Unloading in Osteocytes in Vitro. J Biol Chem (2015) 290:16744–58. doi: 10.1074/jbc.M114.628313
69. Heino TJ, Hentunen TA, Väänänen HK. Conditioned medium from osteocytes stimulates the proliferation of bone marrow mesenchymal stem cells and their differentiation into osteoblasts. Exp Cell Res (2004) 294:458–68. doi: 10.1016/j.yexcr.2003.11.016
70. Xu LH, Shao H, Ma Y-HV, You L. OCY454 Osteocytes as an in Vitro Cell Model for Bone Remodeling Under Mechanical Loading. J Orthopaedic Res (2019) 37:1681–9. doi: 10.1002/jor.24302
71. Woo SM, Rosser J, Dusevich V, Kalajzic I, Bonewald LF. Cell line IDG-SW3 replicates osteoblast-to-late-osteocyte differentiation in vitro and accelerates bone formation in vivo. J Bone Mineral Res (2011) 26:2634–46. doi: 10.1002/jbmr.465
72. Stewart AF. Hypercalcemia Associated with Cancer. N Engl J Med (2005) 352:373–9. doi: 10.1056/NEJMcp042806
73. Levin A, Bakris GL, Molitch M, Smulders M, Tian J, Williams LA, et al. PTH, calcium, and phosphorus in patients with chronic kidney disease: Results of the study to evaluate early kidney disease. Kidney Int (2007) 71:31–8. doi: 10.1038/sj.ki.5002009
74. Jähn K, Kelkar S, Zhao H, Xie Y, Tiede-Lewis LM, Dusevich V, et al. Osteocytes Acidify Their Microenvironment in Response to PTHrP In Vitro and in Lactating Mice In Vivo. J Bone Mineral Res (2017) 32:1761–72. doi: 10.1002/jbmr.3167
75. Qing H, Ardeshirpour L, Divieti Pajevic P, Dusevich V, Jähn K, Kato S, et al. Demonstration of osteocytic perilacunar/canalicular remodeling in mice during lactation. J Bone Miner Res (2012) 27:1018–29. doi: 10.1002/jbmr.1567
76. Dole NS, Mazur CM, Acevedo C, Lopez JP, Monteiro DA, Fowler TW, et al. Osteocyte-Intrinsic TGF-β Signaling Regulates Bone Quality through Perilacunar/Canalicular Remodeling. Cell Rep (2017) 21:2585–96. doi: 10.1016/j.celrep.2017.10.115
77. Hou WS, Brömme D, Zhao Y, Mehler E, Dushey C, Weinstein H, et al. Characterization of novel cathepsin K mutations in the pro and mature polypeptide regions causing pycnodysostosis. J Clin Invest (1999) 103:731–8. doi: 10.1172/JCI653
78. Bossard MJ, Tomaszek TA, Thompson SK, Amegadzie BY, Hanning CR, Jones C, et al. Proteolytic activity of human osteoclast cathepsin K. Expression, purification, activation, and substrate identification. J Biol Chem (1996) 271:12517–24. doi: 10.1074/jbc.271.21.12517
79. Fowler TW, Acevedo C, Mazur CM, Hall-Glenn F, Fields AJ, Bale HA, et al. Glucocorticoid suppression of osteocyte perilacunar remodeling is associated with subchondral bone degeneration in osteonecrosis. Sci Rep (2017) 7:44618. doi: 10.1038/srep44618
80. Zhang K, Barragan-Adjemian C, Ye L, Kotha S, Dallas M, Lu Y, et al. E11/gp38 Selective Expression in Osteocytes: Regulation by Mechanical Strain and Role in Dendrite Elongation. Mol Cell Biol (2006) 26:4539. doi: 10.1128/MCB.02120-05
81. Aziz AH, Wilmoth RL, Ferguson VL, Bryant SJ. IDG-SW3 Osteocyte Differentiation and Bone Extracellular Matrix Deposition Are Enhanced in a 3D Matrix Metalloproteinase-Sensitive Hydrogel. ACS Appl Bio Mater (2020) 3:1666–80. doi: 10.1021/acsabm.9b01227
82. Tanaka T, Hoshijima M, Sunaga J, Nishida T, Hashimoto M, Odagaki N, et al. Analysis of Ca2+ response of osteocyte network by three-dimensional time-lapse imaging in living bone. J Bone Mineral Metab (2018) 36:519–28. doi: 10.1007/s00774-017-0868-x
83. Wysolmerski JJ. Osteocytes remove and replace perilacunar mineral during reproductive cycles. Bone (2013) 54:230–6. doi: 10.1016/j.bone.2013.01.025
84. McGee-Lawrence ME, Stoll DM, Mantila ER, Fahrner BK, Carey HV, Donahue SW. Thirteen-lined ground squirrels (<em<Ictidomys tridecemlineatus</em<) show microstructural bone loss during hibernation but preserve bone macrostructural geometry and strength. J Exp Biol (2011) 214:1240. doi: 10.1242/jeb053520
85. Kerschnitzki M, Kollmannsberger P, Burghammer M, Duda GN, Weinkamer R, Wagermaier W, et al. Architecture of the osteocyte network correlates with bone material quality. J Bone Mineral Res (2013) 28:1837–45. doi: 10.1002/jbmr.1927
86. Gardinier JD, Al-Omaishi S, Morris MD, Kohn DH. PTH signaling mediates perilacunar remodeling during exercise. Matrix Biol (2016) 52-54:162–75. doi: 10.1016/j.matbio.2016.02.010
87. Kaya S, Basta-Pljakic J, Seref-Ferlengez Z, Majeska RJ, Cardoso L, Bromage TG, et al. Lactation-Induced Changes in the Volume of Osteocyte Lacunar-Canalicular Space Alter Mechanical Properties in Cortical Bone Tissue. J Bone Mineral Res (2017) 32:688–97. doi: 10.1002/jbmr.3044
88. Rho J-Y. Kuhn-Spearing, Liisa, Zioupos, Peter, Mechanical properties and the hierarchical structure of bone. Med Eng Phys (1998) 20:92–102. doi: 10.1016/S1350-4533(98)00007-1
89. Burton B, Gaspar A, Josey D, Tupy J, Grynpas MD, Willett TL. Bone embrittlement and collagen modifications due to high-dose gamma-irradiation sterilization. Bone (2014) 61:71–81. doi: 10.1016/j.bone.2014.01.006
90. Cabral WA, Perdivara I, Weis M, Terajima M, Blissett AR, Chang W, et al. Abnormal Type I Collagen Post-translational Modification and Crosslinking in a Cyclophilin B KO Mouse Model of Recessive Osteogenesis Imperfecta. PLoS Genet (2014) 10:e1004465. doi: 10.1371/journal.pgen.1004465
91. Ramshaw JAM, Shah NK, Brodsky B. Gly-X-Y Tripeptide Frequencies in Collagen: A Context for Host–Guest Triple-Helical Peptides. J Struct Biol (1998) 122:86–91. doi: 10.1006/jsbi.1998.3977
92. Wu M, Cronin K, Crane JS. Collagen Synthesis, Biochemistry. Treasure Island, FL: StatPearls Publishing LLC (2020).
93. Yang P-F, Sanno M, Ganse B, Koy T, Brüggemann G-P, Müller LP, et al. Torsion and Antero-Posterior Bending in the In Vivo Human Tibia Loading Regimes during Walking and Running. PLoS One (2014) 9:e94525. doi: 10.1371/journal.pone.0094525
94. Jones SJ, Boyde A, Pawley JB. Osteoblasts and collagen orientation. Cell Tissue Res (1975) 159:73–80. doi: 10.1007/BF00231996
95. Matsugaki A, Fujiwara N, Nakano T. Continuous cyclic stretch induces osteoblast alignment and formation of anisotropic collagen fiber matrix. Acta Biomater (2013) 9:7227–35. doi: 10.1016/j.actbio.2013.03.015
96. Krane SM. The importance of proline residues in the structure, stability and susceptibility to proteolytic degradation of collagens. Amino Acids (2008) 35:703. doi: 10.1007/s00726-008-0073-2
97. Zambonin Zallone A, Teti A, Primavera MV, Pace G. Mature osteocytes behaviour in a repletion period: the occurrence of osteoplastic activity. Basic Appl Histochem (1983) 27:191–204. 6196018.
98. Lotinun S, Ishihara Y, Nagano K, Kiviranta R, Carpentier VT, Neff L, et al. Cathepsin K–deficient osteocytes prevent lactation-induced bone loss and parathyroid hormone suppression. J Clin Invest (2019) 129:3058–71. doi: 10.1172/JCI122936
99. Tang SY, Herber R-P, Ho SP, Alliston T. Matrix metalloproteinase–13 is required for osteocytic perilacunar remodeling and maintains bone fracture resistance. J Bone Miner Res (2012) 27:1936–50. doi: 10.1002/jbmr.1646
100. McNerny EMB, Gong B, Morris MD, Kohn DH. Bone Fracture Toughness and Strength Correlate With Collagen Cross-Link Maturity in a Dose-Controlled Lathyrism Mouse Model. J Bone Mineral Res (2015) 30:455–64. doi: 10.1002/jbmr.2356
101. Xu T, Bianco P, Fisher LW, Longenecker G, Smith E, Goldstein S, et al. Targeted disruption of the biglycan gene leads to an osteoporosis-like phenotype in mice. Nat Genet (1998) 20:78–82. doi: 10.1038/1746
102. Arteaga-Solis E, Sui-Arteaga L, Kim M, Schaffler MB, Jepsen KJ, Pleshko N, et al. Material and mechanical properties of bones deficient for fibrillin-1 or fibrillin-2 microfibrils. Matrix Biol (2011) 30:188–94. doi: 10.1016/j.matbio.2011.03.004
103. Malaval L, Wade-Guéye NM, Boudiffa M, Fei J, Zirngibl R, Chen F, et al. Bone sialoprotein plays a functional role in bone formation and osteoclastogenesis. J Exp Med (2008) 205:1145–53. doi: 10.1084/jem.20071294
104. Corsi A, Xu T, Chen XD, Boyde A, Liang J, Mankani M, et al. Phenotypic effects of biglycan deficiency are linked to collagen fibril abnormalities, are synergized by decorin deficiency, and mimic Ehlers-Danlos-like changes in bone and other connective tissues. J Bone Miner Res (2002) 17:1180–9. doi: 10.1359/jbmr.2002.17.7.1180
Keywords: perilacunar remodeling, lacunocanalicular network, extracellular matrix, collagen, mineral, mechanical loading
Citation: Creecy A, Damrath JG and Wallace JM (2021) Control of Bone Matrix Properties by Osteocytes. Front. Endocrinol. 11:578477. doi: 10.3389/fendo.2020.578477
Received: 30 June 2020; Accepted: 01 December 2020;
Published: 18 January 2021.
Edited by:
Deborah Veis, Washington University School of Medicine in St. Louis, United StatesReviewed by:
Laoise Maria McNamara, National University of Ireland Galway, IrelandCopyright © 2021 Creecy, Damrath and Wallace. This is an open-access article distributed under the terms of the Creative Commons Attribution License (CC BY). The use, distribution or reproduction in other forums is permitted, provided the original author(s) and the copyright owner(s) are credited and that the original publication in this journal is cited, in accordance with accepted academic practice. No use, distribution or reproduction is permitted which does not comply with these terms.
*Correspondence: Joseph M. Wallace, am13YWxsYUBpdXB1aS5lZHU=
†These authors have contributed equally to this work
Disclaimer: All claims expressed in this article are solely those of the authors and do not necessarily represent those of their affiliated organizations, or those of the publisher, the editors and the reviewers. Any product that may be evaluated in this article or claim that may be made by its manufacturer is not guaranteed or endorsed by the publisher.
Research integrity at Frontiers
Learn more about the work of our research integrity team to safeguard the quality of each article we publish.