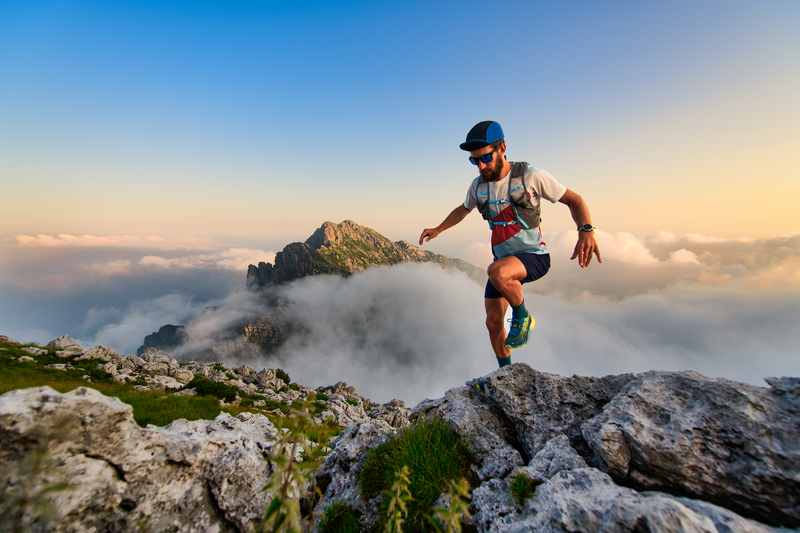
94% of researchers rate our articles as excellent or good
Learn more about the work of our research integrity team to safeguard the quality of each article we publish.
Find out more
MINI REVIEW article
Front. Endocrinol. , 02 October 2020
Sec. Bone Research
Volume 11 - 2020 | https://doi.org/10.3389/fendo.2020.543623
This article is part of the Research Topic Bone Inside-Out and Outside-In Signals: Control of Body Homeostasis View all 21 articles
Bone regeneration has become a research hotspot and therapeutic target in the field of bone and joint medicine. Stem cell-based therapy aims to promote endogenous regeneration and improves therapeutic effects and side-effects of traditional reconstruction of significant bone defects and disorders. Human amniotic mesenchymal stem cells (hAMSCs) are seed cells with superior paracrine functions on immune-regulation, anti-inflammation, and vascularized tissue regeneration. The present review summarized the source and characteristics of hAMSCs and analyzed their roles in tissue regeneration. Next, the therapeutic effects and mechanisms of hAMSCs in promoting bone regeneration of joint diseases and bone defects. Finally, the clinical application of hAMSCs from current clinical trials was analyzed. Although more studies are needed to confirm that hAMSC-based therapy to treat bone diseases, the clinical application prospect of the approach is worth investigating.
Large bone defects and disorders, either congenital or acquired, severely affect the patients' appearance and function (1). Moreover, the incidence of these bone diseases is high and has been on the rise in the past decade. In terms of bone defects, approximately more than 350 million people suffer from fractures, about 46 million people have head injuries, and 20 million people are subject to spinal injuries. Meanwhile, more than 300 million people have been diagnosed with osteoarthritis while about 20 million people have got rheumatoid arthritis around the world. Periodontal disease, which tends to cause severe alveolar bone loss and tooth loss, also has a high prevalence rate of about 800 million (2). Currently, the reconstruction of the bone defects mainly depends on autologous tissue transplantation due to various factors, such as biocompatibility and histocompatibility (3). However, this strategy has limited applications due to a shortage of harvest sites, incomplete integration into the defects, and risk of disease transfer (4). In addition to various defects, bone disorders also include joint diseases associated with an autoimmune disorder, such as rheumatoid arthritis (RA) (5), osteoarthritis (OA) (6), and ankylosing spondylitis (AS) (7). Typically, these joint diseases are treated by drugs (for example, glucocorticoid, immunosuppressive agents, non-steroidal anti-inflammatory drugs, and disease modifying antirheumatic drug) to reduce the symptoms and improve the joint function; however, therapeutic interventions are essential in the advanced stage characterized by loss of articular cartilage, subchondral sclerosis, osteophyte formation, and joint capsule thickening (8). Nowadays, tissue engineering is gaining increasing attention and is expected to resolve these clinical issues.
Different types of scaffold, bioactive factors, and seed cells are the three major elements of tissue engineering. Thus, finding an ideal scaffold to replace the autologous bone translation in the treatment of bone disorder is under intensive research. Nowadays, the scaffold matrix used for bone tissue engineering includes inorganic material, polymers, and their composites (9). In addition, bioactive factors, such as bone morphogenetic protein-2 (BMP-2), fibroblast growth factor-2 (FGF-2), vascular endothelial growth factor (VEGF), and epidermal growth factor (EGF), also play a vital role in bone rebuilding (10). Among those growth factors, BMP-2 and FGF-2 have been utilized to promote bone regeneration and angiogenesis in clinical practice (11, 12). Currently, stem cell-based tissue regeneration has some curative effects (13), but the effect of seed cells in repairing the bone disorders is yet controversial.
Endogenous regeneration, proposed in recent years, focuses on the stimulation and regulation of endogenous factors to achieve in situ tissue regeneration by applying bioactive factors locally (14). Stem cells possess robust biological potential with respect to self-renewal, multidirectional differentiation, and paracrine functions (15, 16). These might act as bioactive factors to activate the endogenous regeneration by local or systematic applications (14, 17). The homeostasis of tissues and organs relies on the coordination and regulation of the nervous, endocrine, and immune systems (18). The endocrine system is a complex network of hormone-producing cells and tissues, which secrete a variety of hormones to act on distant and/or adjacent target cells through endocrine, paracrine, autocrine, or intracrine mechanisms to exert biological activities (19). In addition to enteroendocrine cells, other tissues and cells, such as retinal ganglion cells (RGCs) (20), bone (21), and muscle (22), have paracrine and endocrine functions to maintain homeostasis. Growth hormone (GH) can be expressed in RGCs, and retinal GH has a paracrine role in ocular development and vision (20). It has been widely accepted that stem cells could secrete a variety of bioactive factors which regulate immune state of the body and local microenvironment of tissue regeneration (23). These mechanisms of stem cell-based therapy are to some extent similar to those of some hormones, such as GH. Based on these similarities, stem cell-based therapy might exert a positive effect on promoting endogenous bone regeneration.
Stem cells are divided into embryonic stem cells and adult stem cells (16). The embryonic stem cells for stem cell therapy shows high tumorigenicity and ethical problems in the application process (24). Adult stem cells, including mesenchymal stem cells (MSCs), are undifferentiated cells found in various tissues and organs (25). Nowadays, researchers can isolate MSCs from bone marrow (bone-marrow mesenchymal stem cells, BMSCs) (26), fat (adipose-derived stem cells, AdSCs) (27), peripheral blood (peripheral blood-derived mesenchymal stem cells, PMSCs) (28), umbilical cord blood (umbilical cord blood-derived mesenchymal stem cells, CB-MSCs) (29), and other tissues (30–32) for tissue engineering, immune-regulation, and anti-inflammation. However, it is also unknown which source of stem cells is better for promoting tissue regeneration after transplantation.
Currently, we are focusing on promoting bone regeneration in the oral and maxillofacial regions using human amniotic mesenchymal stem cells (hAMSCs). In this study, we reviewed the source, characteristics, and roles of hAMSCs in bone regeneration, not only in the reconstruction of bone defects but also in the treatment of arthritis. Thus, hAMSCs might be used as an innovative treatment option to promote endogenous bone regeneration.
MSCs are specialized cells with multi-differentiation potentials, which can be activated to differentiate into tissue cells under specific inducing conditions (33, 34). Previous studies have demonstrated that MSCs have abilities of regeneration and immunoregulation (35). The hAMSCs, isolated from the amniotic membrane (AM) of the human term placenta that plays a key role in maintaining maternal-neonatal tolerance, not only share phenotypes similar to typical MSCs, including fibroblast-like morphology, specific surface molecules, and multi-differentiation potential but also have superior immunomodulatory (36–39) and paracrine properties (40, 41). Compared to hAMSCs, most MSCs have inevitable disadvantages on clinical use, including invasive access procedure, host immune response after transplantation, age-related heterogeneity in the quality of MSCs, and extremely low acquisition rate of MSCs (33).
The AM is the innermost layer of the placenta consisting of two sets of cells; one is the amnion epithelial cells that are in direct contact with the amniotic fluid, and the other is the amnion MSCs dispersed in the matrix (42, 43). Since AM is an avascular structure and its epithelial layer can be easily removed by Dispase II, the hAMSCs can be obtained without contamination of endothelial cells and hematopoietic cells (42, 44). Each gram of wet amnion tissue can provide 1.7 ± 0.3 × 106 hAMSCs (45), which are positive for CD44 and CD90 (46, 47). Moreover, the placental tissue becomes a medical waste after childbirth, and hAMSCs can be harvested non-invasively and without ethical controversy (48). In addition, parturients are usually young women, and hence, age-related heterogeneity of hAMSCs might be relatively better than that of stem cells from other sources. The hAMSCs lack the expression of human major histocompatibility complex (MHC) antigens (human leukocyte antigens, HLA), including HLA class I antigens (HLA-DP, HLA-DA, HLA-DR) and HLA class I antigens (HLA-A, HLA-B, HLA-C), showing low immunogenicity (49, 50), while they also exhibit low tumorigenicity due to lack of expression of telomerase (48, 51, 52). Low immunogenicity and low tumorigenicity of hAMSCs render them conducive for allotransplantation to promote tissue regeneration. Also, their paracrine properties have multiple regulatory functions (40). Furthermore, several bioactive factors could be produced by hAMSCs, including immunomodulatory factors that are crucial for the resolution of inflammation (44, 53) and growth and angiogenic factors that are critical for tissue remodeling (41). These exogenous molecules have been shown to be important in inducing endogenous regeneration.
Arthritis is a characteristic of rheumatic diseases, which are chronic, intractable, and musculoskeletal system diseases, such as RA, OA, AS, and juvenile idiopathic arthritis (JIA) (54). Although the pathological characteristics of these joint disorders are different, the joint symptoms are associated with abnormal autoimmune function, inflammatory cell infiltration, and joint structural lesions (5). Stem cells, including hAMSCs, have been introduced to arthritis models, such as rat, to improve the treatment by inhibiting inflammation, regulating the status of autoimmunity, and promoting tissue regeneration (Table 1) (55, 64, 65).
RA is a chronic, autoimmune, inflammatory joint disease characterized by hyperplasia of the synovial membrane and infiltration of immune and inflammatory cells. The synovial cell fibrosis, excessive production of inflammatory cytokines, and osteoclast appearance led to joint destruction and disability (5). The immunomodulatory and anti-inflammatory properties of hAMSCs indicated the therapeutic potential for the treatment of RA. In the classic rat arthritis model for human RA, hAMSCs significantly ameliorated the severity of arthritis and decreased the histopathological changes due to dramatic inhibition of the production of proinflammatory cytokines, such as interferon-γ (IFN-γ) and tumor necrosis factor-α (TNF-α) (55). For a T cell-mediated disease, such as RA, the therapeutic effects of hAMSCs are crucial because they could remarkably restore the CD4+/CD8+ T-cell ratio and inhibit the response of T-cells (55). In addition, hAMSCs suppressed the antigen-specific Th1/Th17 activation and stimulated the generation of CD4+CD25+FoxP3+ Treg cells (56). In mice with collagen-induced arthritis (CIA), systemic infusion of hAMSCs markedly reduces Th1-driven autoimmunity and inflammation, as shown by decreased production of TNF-α, IFN-γ, and some interleukins (IL-2 and IL-17) and increased production of IL-10 and activation of cyclooxygenase 1/2 (COX1/2) (56).
OA is another chronic joint disease with an incidence as high as 40% (66). It is a degenerative disease characterized by progressive cartilage degradation, subchondral bone remodeling, osteophyte formation, and synovitis (67). Synovial NK cells and macrophages secrete abnormally large amounts of perforins, granzymes, and pro-inflammatory cytokines (TNF-α, IL-1β) to induce and aggravate the synovial inflammation and bone/cartilage resorption (57), while activation of CD4+Th1 cells contributes to the development of inflammation (68). The hAMSCs might be beneficial for OA as they inhibit the proliferation of T-cells in vitro (58), polarize M2 macrophages in the condition with the hallmarks of RA in vitro (59), and promote bone/cartilage regeneration in rabbits (69, 70). The conditioned medium of hAMSCs (hAMSC-CM) was reported to remedy tissue fibrosis by lowering the levels of T-cells and macrophages, leading to a decline in pro-inflammatory cytokines (60). When hAMSCs were introduced to the OA model established by coculturing the OA patients' cartilage and synovium, the M1:M2 percentage ratio of synovial macrophages was decreased significantly; also, the concentrations of IL-1β and matrix metalloproteinase-13 (MMP-13) was declined, and macrophage-mediated cartilage destruction was effectively abrogated (59).
In addition, hAMSCs not only secreted active factors with therapeutic effects routinely but also produced abundant cytokines in specific environments. Under identical inflammatory conditions, hAMSCs produce more anti-inflammatory cytokines than AdSCs, such as IL-10 (61). Under three-dimensional (3D) culture conditions, hAMSCs spheroids could secrete considerable amounts of angiogenic and immunosuppressive factors while remaining viable and multipotent (62). The production of some cytokines by hAMSCs vary under different conditions of different oxygen tension. Consequent to exposure to 20% oxygen culture condition, hAMSCs secrete abundant IL-6 as a response to changes in the mitochondrial function, but the content of intracellular reactive oxygen species (ROS) remained unaltered (63). These properties of hAMSCs rendered the stem cell-based therapy applicable and also provided valuable guidance for bone regeneration.
The hAMSCs, as a kind of stem cells, can be induced to osteogenic differentiation to form refracted crystal-like nodules which could be indicated by alkaline phosphatase staining or/and alizarin red S staining (71–73). The expression of osteogenesis-related genes and proteins, such as alkaline phosphatase (ALP), runt-related transcription factor 2 (Runx2), osteopontin (OPN), and osteocalcin (OCN), was significantly enhanced in osteo-induced hAMSCs (74–76); also, their osteogenic differentiation capacity is superior to other MSCs derived from chorionic membrane and decidua (77, 78). However, the comparison of the osteogenic capacity of hAMSCs and BMSCs revealed that BMSCs are more likely to differentiate into osteoblasts in vitro and seem to be appropriate for bone regeneration (79). Nevertheless, the capacity of hAMSCs might not be inferior to that of BMSCs in improving bone regeneration in vivo. Several studies have reported that transplanted MSCs might play therapeutic roles by paracrine signaling rather than becoming target tissue cells directly (80–82). In recent years, several studies have focused on hAMSCs promoting tissue regeneration, based on their functions of anti-inflammation, pro-angiogenesis, and chemotaxis(Table 2) (38, 83, 96). To the best of our knowledge, hAMSCs secrete more cytokines than BMSCs, including interleukins (IL-6 and IL-8), C-X-C motif chemokine ligand-1/5 (CXCL1 and CXCL5), Angiogenin (ANG), hepatocyte growth factor (HGF), and fibroblast growth factor-7 (FGF-7) (83). These paracrine properties of hAMSCs make them suitable for the restoration of bone defects (40, 83).
Fracture healing is a complex, well-orchestrated, regenerative process that is coordinated by a variety of cell types, including inflammatory cells, vascular endothelial cells, MSCs, and fibroblasts (97). In the various stages of the bone healing process, inflammation and angiogenesis precede osteogenesis, thereby indicating that controlling inflammation and promoting angiogenesis in an early stage might speed up the subsequent bone formation and ultimately bone remodeling (97). Therefore, we proposed to introduce hAMSCs to bone defects' microenvironment and stimulate and accelerate the endogenous vascularized bone regeneration. Several studies have shown that hAMSCs enhance the osteogenic differentiation of AdSCs, BMSCs, and promote the tube-formation of human umbilical vein endothelial cells (HUVECs) (84–89). When hAMSCs are co-cultured with BMSCs in a transwell system, ALP activity of BMSCs and the expression of osteogenic markers, including OCN and Runx2, were upregulated (90). Conversely, in the co-culture, hAMSCs reverse the inhibition of oxidative stress-induced osteogenic differentiation of caused by hydrogen peroxide, which in turn, inhibits the inflammatory response in vivo (91). When hAMSCs are co-cultured with HUVECs, high levels of Collagen-1 (COL1), ANG, and VEGF were detected in the co-culture medium, and capillary-like tube structures were observed in HUVEC tube-formation assay (92). Interestingly, a correlation was established between the high expression of lncRNA H19 and the pro-angiogenic functions of hAMSCs (93).
Based on the in vitro data, the researchers applied hAMSCs to animal bone defect models. Ranzoni et al. injected hAMSCs intraperitoneally into mice that suffered from osteogenesis imperfecta (OI). The transplanted mice had improved bone structural quality, high mineral density, and better mineralization, while the genes related to osteogenesis were upregulated and those associated with inflammation, TGF-β, and osteoclast differentiation were downregulated (94). The β-tricalcium phosphate (TCP) scaffolds containing xenograft hAMSCs have been reported to improve regeneration of Wistar rats' skull defects. The xenograft cells did not cause obvious immune response in the transplanted rats (95). In our recent studies, we comprehensively analyzed the survival of hAMSCs after transplantation in nude mice and the specific mechanism of hAMSCs in promoting bone tissue regeneration. It had been confirmed that hAMSCs could be survival in bone defects for at least 2 weeks after transplantation. Although hAMSCs survived in vivo, they did not seem to transform into osteoblasts. In specimens from early bone defect healing, hAMSCs polarized macrophages to M2 that could secrete pro-angiogenic and osteogenic cytokines, such as BMP-2 and VEGF (83). Moreover, we also found that hAMSCs promote extracellular matrix remodeling (98). Combined with these functions, we believed hAMSCs could start endogenous vascularized bone regeneration. And in terms of the ultimate osteogenic effect, our findings showed that hAMSCs accelerated new bone formation not only in bone defects but promoted rapid osseointegration of dental implants (69, 83).
Stem cell therapies exert their effects on a wide range of diseases and injuries, including immune disorders (99), various neural disorders or injuries (100), myocardial injury (101), pulmonary diseases (102), diabetes (103), cancer treatments (104), and bone/cartilage degenerative disorders or injuries (105). Several types of stem cells have been subjected to clinical trials (106). Stem cells derived from the human placenta have been reported to be in clinical trials for a variety of therapeutic applications (107). In a single-center, non-randomized, intravenous dose-escalation phase Ib trial, patients with idiopathic pulmonary fibrosis received intravenous administration of placental MSCs from unrelated donors. Previous data demonstrated that placental MSC therapy is feasible and has a satisfactory short-term safety profile in idiopathic pulmonary fibrosis (108). Clinical trials using placental MSCs to treat OA, Crohn's disease, and multiple sclerosis (MS) have shown that the cells were well-tolerated, and cell therapy was dose-related (109–111). Amnion is a part of the placenta that has been used in clinical trials to treat skin and corneal burns (112, 113). These clinical trials suggested that the amniotic membrane accelerates recovery via inhibiting inflammation and releasing growth factors. These therapeutic effects could also be found in hAMSC-CM. In the subsequent clinical trials using this conditioned media, the bioactive cytokines, such as VEGF, FGFs, and keratinocyte growth factor (KGF) were identified and were found to promote ulcer healing (114) and improved photoaging (115) when the medium was locally injected into the lesions. Currently, there are no reports on the clinical trials using hAMSCs on bone regeneration; however, BMSCs (116, 117), AdSCs (118), and dental pulp stem cells (DPSCs) (119) had shown to be safe, feasible, and effective (120). Based on the data of the clinical trials, we speculated that hAMSCs could be studied in the clinical trials of bone regeneration for future applications.
Although hAMSCs have become an alternative source of stem cells in regenerative medicine and tissue engineering due to their advantages such as easy gain, sufficient quantity, and superior properties, the application from laboratory research to clinical practice in the future needs further exploration. We still need to carry out further studies on hAMSCs acquisition, storage, and transportation to form standardized standards to maintain and improve the therapeutic potential of hAMSCs, so as to ensure the clinical application effects. It has been well-known that autologous MSCs represent the primary sources considered safe for transplantation and minimization of immunological risk. Preclinical studies should confirm the safety and immunological risk of allogenic hAMSCs for transplantation by comparing with autologous MSCs, and then the mechanisms of hAMSCs in promoting skeletal system diseases in vivo are also needed to further elucidate, which are important to determine the indications, timing, dosage, and accurate administration of hAMSC-based therapy. For the treatment of bone regeneration and other bone disorders, more efforts should be made to optimize the therapeutic effects of hAMSC-based therapy by combining with other biomaterials and bioactive factors. Despite these challenges, it is no doubt that MSC-based therapy has a promising clinical application prospect. Since previous studies have demonstrated the hAMSCs with excellent MSC properties, hAMSC-based therapy is worthy to be further studied in depth and finally put into clinical practice.
JL and ZZ helped to draft the manuscript. FJ and YX contributed to the conception and supervision of the study. JW contributed to the consultation and revision of the manuscript. All authors contributed to the article and approved the submitted version.
This study was jointly supported by the National Natural Science Foundation of China (81670966, 81900960), Young Elite Scientists Sponsorship Program by CAST, 2018QNRC001, the Natural Science Foundation of Jiangsu Province (SBK2018042855), Qing Lan Project and the Priority Academic Program Development of Jiangsu Higher Education Institutions (PAPD, 2018-87).
The authors declare that the research was conducted in the absence of any commercial or financial relationships that could be construed as a potential conflict of interest.
1. Chen FM, Liu X. Advancing biomaterials of human origin for tissue engineering. Prog Polym Sci. (2016) 53:86–168. doi: 10.1016/j.progpolymsci.2015.02.004
2. Disease GBD, Injury I, Prevalence C. Global, regional, and national incidence, prevalence, and years lived with disability for 354 diseases and injuries for 195 countries and territories, 1990-2017: a systematic analysis for the global burden of disease study 2017. Lancet. (2018) 392:1789–858. doi: 10.1016/S0140-6736(18)32279-7
3. Atala A, Kasper FK, Mikos AG. Engineering complex tissues. Sci Transl Med. (2012) 4:160rv12. doi: 10.1126/scitranslmed.3004890
4. Kolk A, Handschel J, Drescher W, Rothamel D, Kloss F, Blessmann M, et al. Current trends and future perspectives of bone substitute materials - from space holders to innovative biomaterials. J Craniomaxillofac Surg. (2012) 40:706–18. doi: 10.1016/j.jcms.2012.01.002
5. McInnes IB, Schett G. The pathogenesis of rheumatoid arthritis. N Engl J Med. (2011) 365:2205–19. doi: 10.1056/NEJMra1004965
6. Berenbaum F. Osteoarthritis as an inflammatory disease (osteoarthritis is not osteoarthrosis!). Osteoarthritis Cartilage. (2013) 21:16–21. doi: 10.1016/j.joca.2012.11.012
7. Braun J, Sieper J. Ankylosing spondylitis. Lancet. (2007) 369:1379–90. doi: 10.1016/S0140-6736(07)60635-7
8. Lin YJ, Anzaghe M, Schulke S. Update on the pathomechanism, diagnosis, and treatment options for rheumatoid arthritis. Cells. (2020) 9:880. doi: 10.3390/cells9040880
9. Qu HW, Fu HY, Han ZY, Sun, Y. Biomaterials for bone tissue engineering scaffolds: a review. RSC Adv. (2019) 26252–62. doi: 10.1039/c9ra05214c
10. Kowalczewski CJ, Saul JM. Biomaterials for the delivery of growth factors and other therapeutic agents in tissue engineering approaches to bone regeneration. Front Pharmacol. (2018) 9:513. doi: 10.3389/fphar.2018.00513
11. Caballe-Serrano J, Abdeslam-Mohamed Y, Munar-Frau A, Fujioka-Kobayashi M, Hernandez-Alfaro F, Miron R. Adsorption and release kinetics of growth factors on barrier membranes for guided tissue/bone regeneration: a systematic review. Arch Oral Biol. (2019) 100:57–68. doi: 10.1016/j.archoralbio.2019.02.006
12. Kuroda Y, Kawai T, Goto K, Matsuda S. Clinical application of injectable growth factor for bone regeneration: a systematic review. Inflamm Regen. (2019) 39:20. doi: 10.1186/s41232-019-0109-x
13. Badylak SF, Nerem RM. Progress in tissue engineering and regenerative medicine. Proc Natl Acad Sci USA. (2010) 107:3285–6. doi: 10.1073/pnas.1000256107
14. Chen FM, Wu LA, Zhang M, Zhang R, Sun HH. Homing of endogenous stem/progenitor cells for in situ tissue regeneration: promises, strategies, and translational perspectives. Biomaterials. (2011) 32:3189–209. doi: 10.1016/j.biomaterials.2010.12.032
15. Weissman IL. Stem cells: units of development, units of regeneration, and units in evolution. Cell. (2000) 100:157–68. doi: 10.1016/S0092-8674(00)81692-X
16. Zakrzewski W, Dobrzynski M, Szymonowicz M, Rybak Z. Stem cells: past, present, and future. Stem Cell Res Ther. (2019) 10:68. doi: 10.1186/s13287-019-1165-5
17. Ebrahimi M, Botelho M. Adult stem cells of orofacial origin: current knowledge and limitation and future trend in regenerative medicine. Tissue Eng Regen Med. (2017) 14:719–733. doi: 10.1007/s13770-017-0078-6
18. Kotas ME, Medzhitov R. Homeostasis, inflammation, and disease susceptibility. Cell. (2015) 160:816–27. doi: 10.1016/j.cell.2015.02.010
19. Gribble FM, Reimann F. Enteroendocrine cells: chemosensors in the intestinal epithelium. Annu Rev Physiol. (2016) 78:277–99. doi: 10.1146/annurev-physiol-021115-105439
20. Harvey S, Martinez-Moreno CG. Growth hormone and ocular dysfunction: endocrine, paracrine or autocrine etiologies? Growth Horm IGF Res. (2016) 29:28–32. doi: 10.1016/j.ghir.2016.03.004
21. Han Y, You X, Xing W, Zhang Z, Zou W. Paracrine and endocrine actions of bone-the functions of secretory proteins from osteoblasts, osteocytes, and osteoclasts. Bone Res. (2018) 6:16. doi: 10.1038/s41413-018-0019-6
22. Giudice J, Taylor JM. Muscle as a paracrine and endocrine organ. Curr Opin Pharmacol. (2017) 34:49–55. doi: 10.1016/j.coph.2017.05.005
23. Murphy MB, Moncivais K, Caplan AI. Mesenchymal stem cells: environmentally responsive therapeutics for regenerative medicine. Exp Mol Med. (2013) 45:e54. doi: 10.1038/emm.2013.94
24. Deb KD, Sarda K. Human embryonic stem cells: preclinical perspectives. J Transl Med. (2008) 6:7. doi: 10.1186/1479-5876-6-7
26. Bianco P, Riminucci M, Gronthos S, Robey PG. Bone marrow stromal stem cells: nature, biology, and potential applications. Stem Cells. (2001) 19:180–92. doi: 10.1634/stemcells.19-3-180
27. Gimble JM, Katz AJ, Bunnell BA. Adipose-derived stem cells for regenerative medicine. Circ Res. (2007) 100:1249–60. doi: 10.1161/01.RES.0000265074.83288.09
28. Kang HJ, Kim HS, Zhang SY, Park KW, Cho HJ, Koo BK, et al. Effects of intracoronary infusion of peripheral blood stem-cells mobilised with granulocyte-colony stimulating factor on left ventricular systolic function and restenosis after coronary stenting in myocardial infarction: the MAGIC cell randomised clinical trial. Lancet. (2004) 363:751–6. doi: 10.1016/s0140-6736(04)15689-4
29. Wagner W, Wein F, Seckinger A, Frankhauser M, Wirkner U, Krause U, et al. Comparative characteristics of mesenchymal stem cells from human bone marrow, adipose tissue, and umbilical cord blood. Exp Hematol. (2005) 33:1402–16. doi: 10.1016/j.exphem.2005.07.003
30. Carlotti F, Zaldumbide A, Loomans CJ, van Rossenberg E, Engelse M, de Koning EJ, et al. Isolated human islets contain a distinct population of mesenchymal stem cells. Islets. (2010) 2:164–73. doi: 10.4161/isl.2.3.11449
31. Huang F, Chen M, Chen W, Gu J, Yuan J, Xue Y, et al. Human gingiva-derived mesenchymal stem cells inhibit xeno-graft-versus-host disease via CD39-CD73-Adenosine and IDO Signals. Front Immunol. (2017) 8:68. doi: 10.3389/fimmu.2017.00068
32. De Bari C, Dell'Accio F, Tylzanowski P, Luyten FP. Multipotent mesenchymal stem cells from adult human synovial membrane. Arthritis Rheum. (2001) 44:1928–42. doi: 10.1002/1529-0131(200108)44:8<1928::AID-ART331>3.0.CO;2-P
33. Pittenger MF, Mackay AM, Beck SC, Jaiswal RK, Douglas R, Mosca JD, et al. Multilineage potential of adult human mesenchymal stem cells. Science. (1999) 284:143–7. doi: 10.1126/science.284.5411.143
34. Berebichez-Fridman R, Montero-Olvera PR. Sources and clinical applications of mesenchymal stem cells: state-of-the-art review. Sultan Qaboos Univ Med J. (2018) 18:e264–e-77. doi: 10.18295/squmj.2018.18.03.002
35. Dimarino AM, Caplan AI, Bonfield TL. Mesenchymal stem cells in tissue repair. Front Immunol. (2013) 4:201. doi: 10.3389/fimmu.2013.00201
36. Magatti M, Caruso M, De Munari S, Vertua E, De D, Manuelpillai U, et al. Human amniotic membrane-derived mesenchymal and epithelial cells exert different effects on monocyte-derived dendritic cell differentiation and function. Cell Transplant. (2015) 24:1733–52. doi: 10.3727/096368914X684033
37. Magatti M, Vertua E, Cargnoni A, Silini A, Parolini O. The immunomodulatory properties of amniotic cells: the two sides of the coin. Cell Transplant. (2018) 27:31–44. doi: 10.1177/0963689717742819
38. Silini AR, Magatti M, Cargnoni A, Parolini O. Is immune modulation the mechanism underlying the beneficial effects of amniotic cells and their derivatives in regenerative medicine? Cell Transplant. (2017) 26:531–9. doi: 10.3727/096368916X693699
39. Insausti CL, Blanquer M, Garcia-Hernandez AM, Castellanos G, Moraleda JM. Amniotic membrane-derived stem cells: immunomodulatory properties and potential clinical application. Stem Cells Cloning. (2014) 7:53–63. doi: 10.2147/SCCAA.S58696
40. Ling L, Feng X, Wei T, Wang Y, Wang Y, Wang Z, et al. Human amnion-derived mesenchymal stem cell (hAD-MSC) transplantation improves ovarian function in rats with premature ovarian insufficiency (POI) at least partly through a paracrine mechanism. Stem Cell Res Ther. (2019) 10:46. doi: 10.1186/s13287-019-1136-x
41. Li JY, Ren KK, Zhang WJ, Xiao L, Wu HY, Liu QY, et al. Human amniotic mesenchymal stem cells and their paracrine factors promote wound healing by inhibiting heat stress-induced skin cell apoptosis and enhancing their proliferation through activating PI3K/AKT signaling pathway. Stem Cell Res Ther. (2019) 10:247. doi: 10.1186/s13287-019-1366-y
42. Parolini O, Alviano F, Bagnara GP, Bilic G, Buhring HJ, Evangelista M, et al. Concise review: isolation and characterization of cells from human term placenta: outcome of the first international workshop on placenta derived stem cells. Stem Cells. (2008) 26:300–11. doi: 10.1634/stemcells.2007-0594
43. Antoniadou E, David AL. Placental stem cells. Best Pract Res Clin Obstet Gynaecol. (2016) 31:13–29. doi: 10.1016/j.bpobgyn.2015.08.014
44. Lindenmair A, Hatlapatka T, Kollwig G, Hennerbichler S, Gabriel C, Wolbank S, et al. Mesenchymal stem or stromal cells from amnion and umbilical cord tissue and their potential for clinical applications. Cells. (2012) 1:1061–88. doi: 10.3390/cells1041061
45. Bilic G, Zeisberger SM, Mallik AS, Zimmermann R, Zisch AH. Comparative characterization of cultured human term amnion epithelial and mesenchymal stromal cells for application in cell therapy. Cell Transplant. (2008) 17:955–68. doi: 10.3727/096368908786576507
46. Wei X, Sun G, Zhao X, Wu Q, Chen L, Xu Y, et al. Human amnion mesenchymal stem cells attenuate atherosclerosis by modulating macrophage function to reduce immune response. Int J Mol Med. (2019) 44:1425–35. doi: 10.3892/ijmm.2019.4286
47. Koike C, Zhou K, Takeda Y, Fathy M, Okabe M, Yoshida T, et al. Characterization of amniotic stem cells. Cell Reprogram. (2014) 16:298–305. doi: 10.1089/cell.2013.0090
48. Parolini O, Soncini M, Evangelista M, Schmidt D. Amniotic membrane and amniotic fluid-derived cells: potential tools for regenerative medicine? Regen Med. (2009) 4:275–91. doi: 10.2217/17460751.4.2.275
49. Bailo M, Soncini M, Vertua E, Signoroni PB, Sanzone S, Lombardi G, et al. Engraftment potential of human amnion and chorion cells derived from term placenta. Transplantation. (2004) 78:1439–48. doi: 10.1097/01.TP.0000144606.84234.49
50. Evangelista M, Soncini M, Parolini O. Placenta-derived stem cells: new hope for cell therapy? Cytotechnology. (2008) 58:33–42. doi: 10.1007/s10616-008-9162-z
51. Miki T, Lehmann T, Cai H, Stolz DB, Strom SC. Stem cell characteristics of amniotic epithelial cells. Stem Cells. (2005) 23:1549–59. doi: 10.1634/stemcells.2004-0357
52. Meng MY, Li L, Wang WJ, Liu FF, Song J, Yang SL, et al. Assessment of tumor promoting effects of amniotic and umbilical cord mesenchymal stem cells in vitro and in vivo. J Cancer Res Clin Oncol. (2019) 145:1133–46. doi: 10.1007/s00432-019-02859-6
53. Farhadihosseinabadi B, Farahani M, Tayebi T, Jafari A, Biniazan F, Modaresifar K, et al. Amniotic membrane and its epithelial and mesenchymal stem cells as an appropriate source for skin tissue engineering and regenerative medicine. Artif Cells Nanomed Biotechnol. (2018) 46:431–40. doi: 10.1080/21691401.2018.1458730
54. Narazaki M, Tanaka T, Kishimoto T. The role and therapeutic targeting of IL-6 in rheumatoid arthritis. Expert Rev Clin Immunol. (2017) 13:535–51. doi: 10.1080/1744666X.2017.1295850
55. Shu J, He XJ, Li H, Liu X, Qiu XM, Zhou TL, et al. The beneficial effect of human amnion mesenchymal cells in inhibition of inflammation and induction of neuronal repair in EAE mice. J Immunol Res. (2018) 2018:5083797. doi: 10.1155/2018/5083797
56. Parolini O, Souza-Moreira L, O'Valle F, Magatti M, Hernandez-Cortes P, Gonzalez-Rey E, et al. Therapeutic effect of human amniotic membrane-derived cells on experimental arthritis and other inflammatory disorders. Arthritis Rheumatol. (2014) 66:327–39. doi: 10.1002/art.38206
57. Huss RS, Huddleston JI, Goodman SB, Butcher EC, Zabel BA. Synovial tissue-infiltrating natural killer cells in osteoarthritis and periprosthetic inflammation. Arthritis Rheum. (2010) 62:3799–805. doi: 10.1002/art.27751
58. Pianta S, Bonassi Signoroni P, Muradore I, Rodrigues MF, Rossi D, Silini A, et al. Amniotic membrane mesenchymal cells-derived factors skew T cell polarization toward Treg and downregulate Th1 and Th17 cells subsets. Stem Cell Rev Rep. (2015) 11:394–407. doi: 10.1007/s12015-014-9558-4
59. Topoluk N, Steckbeck K, Siatkowski S, Burnikel B, Tokish J, Mercuri J. Amniotic mesenchymal stem cells mitigate osteoarthritis progression in a synovial macrophage-mediated in vitro explant coculture model. J Tissue Eng Regen Med. (2018) 12:1097–110. doi: 10.1002/term.2610
60. Cargnoni A, Piccinelli EC, Ressel L, Rossi D, Magatti M, Toschi I, et al. Conditioned medium from amniotic membrane-derived cells prevents lung fibrosis and preserves blood gas exchanges in bleomycin-injured mice-specificity of the effects and insights into possible mechanisms. Cytotherapy. (2014) 16:17–32. doi: 10.1016/j.jcyt.2013.07.002
61. Borem R, Madeline A, Bowman M, Gill S, Tokish J, Mercuri J. Differential effector response of amnion- and adipose-derived mesenchymal stem cells to inflammation; implications for intradiscal therapy. J Orthop Res. (2019) 37:2445–56. doi: 10.1002/jor.24412
62. Miceli V, Pampalone M, Vella S, Carreca AP, Amico G, Conaldi PG. Comparison of immunosuppressive and angiogenic properties of human amnion-derived mesenchymal stem cells between 2d and 3d culture systems. Stem Cells Int. (2019) 2019:7486279. doi: 10.1155/2019/7486279
63. Banerjee A, Lindenmair A, Steinborn R, Dumitrescu SD, Hennerbichler S, Kozlov AV, et al. Oxygen tension strongly influences metabolic parameters and the release of interleukin-6 of human amniotic mesenchymal stromal cells in vitro. Stem Cells Int. (2018) 2018:9502451. doi: 10.1155/2018/9502451
64. Chahal J, Gomez-Aristizabal A, Shestopaloff K, Bhatt S, Chaboureau A, Fazio A, et al. Bone marrow mesenchymal stromal cell treatment in patients with osteoarthritis results in overall improvement in pain and symptoms and reduces synovial inflammation. Stem Cells Transl Med. (2019) 8:746–57. doi: 10.1002/sctm.18-0183
65. Park KH, Mun CH, Kang MI, Lee SW, Lee SK, Park YB. Treatment of collagen-induced arthritis using immune modulatory properties of human mesenchymal stem cells. Cell Transplant. (2016) 25:1057–72. doi: 10.3727/096368915X687949
66. Safiri S, Kolahi AA, Smith E, Hill C, Bettampadi D, Mansournia MA, et al. Global, regional and national burden of osteoarthritis 1990-2017: a systematic analysis of the global burden of disease study 2017. Ann Rheum Dis. (2020) 79:819–28. doi: 10.1136/annrheumdis-2019-216515
67. Glyn-Jones S, Palmer AJ, Agricola R, Price AJ, Vincent TL, Weinans H, et al. Osteoarthritis. Lancet. (2015) 386:376–87. doi: 10.1016/S0140-6736(14)60802-3
68. Li YS, Luo W, Zhu SA, Lei GH. T cells in osteoarthritis: alterations and beyond. Front Immunol. (2017) 8:356. doi: 10.3389/fimmu.2017.00356
69. Yin L, Zhou ZX, Shen M, Chen N, Jiang F, Wang SL. The human amniotic mesenchymal stem cells (hamscs) improve the implant osseointegration and bone regeneration in maxillary sinus floor elevation in rabbits. Stem Cells Int. (2019) 2019:9845497. doi: 10.1155/2019/9845497
70. You Q, Liu Z, Zhang J, Shen M, Li Y, Jin Y, et al. Human amniotic mesenchymal stem cell sheets encapsulating cartilage particles facilitate repair of rabbit osteochondral defects. Am J Sports Med. (2020) 48:599–611. doi: 10.1177/0363546519897912
71. Topoluk N, Hawkins R, Tokish J, Mercuri J. Amniotic mesenchymal stromal cells exhibit preferential osteogenic and chondrogenic differentiation and enhanced matrix production compared with adipose mesenchymal stromal cells. Am J Sports Med. (2017) 45:2637–46. doi: 10.1177/0363546517706138
72. Li Y, Liu Z, Tang Y, Feng W, Zhao C, Liao J, et al. Schnurri-3 regulates BMP9-induced osteogenic differentiation and angiogenesis of human amniotic mesenchymal stem cells through Runx2 and VEGF. Cell Death Dis. (2020) 11:72. doi: 10.1038/s41419-020-2279-5
73. Li Y, Liu Z, Tang Y, Fan Q, Feng W, Luo C, et al. Three-dimensional silk fibroin scaffolds enhance the bone formation and angiogenic differentiation of human amniotic mesenchymal stem cells: a biocompatibility analysis. Acta Biochim Biophys Sin. (2020) 52:590–602. doi: 10.1093/abbs/gmaa042
74. Meesuk L, Tantrawatpan C, Kheolamai P, Manochantr S. The immunosuppressive capacity of human mesenchymal stromal cells derived from amnion and bone marrow. Biochem Biophys Rep. (2016) 8:34–40. doi: 10.1016/j.bbrep.2016.07.019
75. Leyva-Leyva M, Barrera L, Lopez-Camarillo C, Arriaga-Pizano L, Orozco-Hoyuela G, Carrillo-Casas EM, et al. Characterization of mesenchymal stem cell subpopulations from human amniotic membrane with dissimilar osteoblastic potential. Stem Cells Dev. (2013) 22:1275–87. doi: 10.1089/scd.2012.0359
76. Fan X, Li L, Ye Z, Zhou Y, Tan WS. Regulation of osteogenesis of human amniotic mesenchymal stem cells by sodium butyrate. Cell Biol Int. (2018) 42:457–69. doi: 10.1002/cbin.10919
77. Shen C, Yang C, Xu S, Zhao H. Comparison of osteogenic differentiation capacity in mesenchymal stem cells derived from human amniotic membrane (AM), umbilical cord (UC), chorionic membrane (CM), and decidua (DC). Cell Biosci. (2019) 9:17. doi: 10.1186/s13578-019-0281-3
78. Ma J, Wu J, Han L, Jiang X, Yan L, Hao J, et al. Comparative analysis of mesenchymal stem cells derived from amniotic membrane, umbilical cord, and chorionic plate under serum-free condition. Stem Cell Res Ther. (2019) 10:19. doi: 10.1186/s13287-018-1104-x
79. Schmelzer E, McKeel DT, Gerlach JC. Characterization of human mesenchymal stem cells from different tissues and their membrane encasement for prospective transplantation therapies. Biomed Res Int. (2019) 2019:6376271. doi: 10.1155/2019/6376271
80. Liu S, Liu D, Chen C, Hamamura K, Moshaverinia A, Yang R, et al. MSC transplantation improves osteopenia via epigenetic regulation of notch signaling in lupus. Cell Metab. (2015) 22:606–18. doi: 10.1016/j.cmet.2015.08.018
81. Li H, Shen S, Fu H, Wang Z, Li X, Sui X, et al. Immunomodulatory functions of mesenchymal stem cells in tissue engineering. Stem Cells Int. (2019) 2019:9671206. doi: 10.1155/2019/9671206
82. Gnecchi M, Danieli P, Malpasso G, Ciuffreda MC. Paracrine mechanisms of mesenchymal stem cells in tissue repair. Methods Mol Biol. (2016) 1416:123–46. doi: 10.1007/978-1-4939-3584-0_7
83. Jiang F, Zhang W, Zhou M, Zhou Z, Shen M, Chen N, et al. Human amniotic mesenchymal stromal cells promote bone regeneration via activating endogenous regeneration. Theranostics. (2020) 10:6216–30. doi: 10.7150/thno.45249
84. Zhang C, Du Y, Yuan H, Jiang F, Shen M, Wang Y, et al. HAMSCs/HBMSCs coculture system ameliorates osteogenesis and angiogenesis against glucolipotoxicity. Biochimie. (2018) 152:121–33. doi: 10.1016/j.biochi.2018.06.028
85. Wang Y, Wu H, Shen M, Ding S, Miao J, Chen N. Role of human amnion-derived mesenchymal stem cells in promoting osteogenic differentiation by influencing p38 MAPK signaling in lipopolysaccharide -induced human bone marrow mesenchymal stem cells. Exp Cell Res. (2017) 350:41–9. doi: 10.1016/j.yexcr.2016.11.003
86. Wang J, Miao J, Meng X, Chen N, Wang Y. Expression of long noncoding RNAs in human bone marrow mesenchymal stem cells cocultured with human amnionderived mesenchymal stem cells. Mol Med Rep. (2017) 16:6683–9. doi: 10.3892/mmr.2017.7465
87. Zhang C, Yu L, Liu S, Wang Y. Human amnion-derived mesenchymal stem cells promote osteogenic and angiogenic differentiation of human adipose-derived stem cells. PLoS ONE. (2017) 12:e0186253. doi: 10.1371/journal.pone.0186253
88. Wang Y, Du Y, Yuan H, Pan Y, Wu J, Du X, et al. Human amnion-derived mesenchymal stem cells enhance the osteogenic differentiation of human adipose-derived stem cells by promoting adiponectin excretion via the APPL1-ERK1/2 signaling pathway. IUBMB Life. (2020) 72:296–304. doi: 10.1002/iub.2165
89. Ma X, Bian Y, Yuan H, Chen N, Pan Y, Zhou W, et al. Human amnion-derived mesenchymal stem cells promote osteogenic differentiation of human bone marrow mesenchymal stem cells via H19/miR-675/APC axis. Aging. (2020) 12:103277. doi: 10.18632/aging.103277
90. Wang Y, Yin Y, Jiang F, Chen N. Human amnion mesenchymal stem cells promote proliferation and osteogenic differentiation in human bone marrow mesenchymal stem cells. J Mol Histol. (2015) 46:13–20. doi: 10.1007/s10735-014-9600-5
91. Wang Y, Ma J, Du Y, Miao J, Chen N. Human amnion-derived mesenchymal stem cells protect human bone marrow mesenchymal stem cells against oxidative stress-mediated dysfunction via erk1/2 mapk signaling. Mol Cells. (2016) 39:186–94. doi: 10.14348/molcells.2016.2159
92. Bian Y, Du Y, Wang R, Chen N, Du X, Wang Y, et al. A comparative study of HAMSCs/HBMSCs transwell and mixed coculture systems. IUBMB Life. (2019) 71:1048–55. doi: 10.1002/iub.2074
93. Yuan Z, Bian Y, Ma X, Tang Z, Chen N, Shen M. LncRNA H19 knockdown in human amniotic mesenchymal stem cells suppresses angiogenesis by associating with EZH2 and Activating Vasohibin-1. Stem Cells Dev. (2019) 28:781–90. doi: 10.1089/scd.2019.0014
94. Ranzoni AM, Corcelli M, Hau KL, Kerns JG, Vanleene M, Shefelbine S, et al. Counteracting bone fragility with human amniotic mesenchymal stem cells. Sci Rep. (2016) 6:39656. doi: 10.1038/srep39656
95. Tsuno H, Yoshida T, Nogami M, Koike C, Okabe M, Noto Z, et al. Application of human amniotic mesenchymal cells as an allogeneic transplantation cell source in bone regenerative therapy. Mater Sci Eng C. (2012) 32:2452–8. doi: 10.1016/j.msec.2012.07.021
96. Manuelpillai U, Moodley Y, Borlongan CV, Parolini O. Amniotic membrane and amniotic cells: potential therapeutic tools to combat tissue inflammation and fibrosis? Placenta. (2011) 32(Suppl. 4):S320–5. doi: 10.1016/j.placenta.2011.04.010
97. Bahney CS, Zondervan RL, Allison P, Theologis A, Ashley JW, Ahn J, et al. Cellular biology of fracture healing. J Orthop Res. (2019) 37:35–50. doi: 10.1002/jor.24170
98. Jiang F, Ma J, Liang Y, Niu Y, Chen N, Shen M. Amniotic mesenchymal stem cells can enhance angiogenic capacity via MMPs in vitro and in vivo. Biomed Res Int. (2015) 2015:324014. doi: 10.1155/2015/324014
99. Prasad VK, Lucas KG, Kleiner GI, Talano JA, Jacobsohn D, Broadwater G, et al. Efficacy and safety of ex vivo cultured adult human mesenchymal stem cells (Prochymal) in pediatric patients with severe refractory acute graft-versus-host disease in a compassionate use study. Biol Blood Marrow Transplant. (2011) 17:534–41. doi: 10.1016/j.bbmt.2010.04.014
100. Gupta N, Henry RG, Strober J, Kang SM, Lim DA, Bucci M, et al. Neural stem cell engraftment and myelination in the human brain. Sci Transl Med. (2012) 4:155ra137. doi: 10.1126/scitranslmed.3004373
101. Hare JM, Fishman JE, Gerstenblith G, DiFede Velazquez DL, Zambrano JP, Suncion VY, et al. Comparison of allogeneic vs autologous bone marrow-derived mesenchymal stem cells delivered by transendocardial injection in patients with ischemic cardiomyopathy: the POSEIDON randomized trial. JAMA. (2012) 308:2369–79. doi: 10.1001/jama.2012.25321
102. Chang YS, Ahn SY, Yoo HS, Sung SI, Choi SJ, Oh WI, et al. Mesenchymal stem cells for bronchopulmonary dysplasia: phase 1 dose-escalation clinical trial. J Pediatr. (2014) 164:966–72.e6. doi: 10.1016/j.jpeds.2013.12.011
103. Liu X, Zheng P, Wang X, Dai G, Cheng H, Zhang Z, et al. A preliminary evaluation of efficacy and safety of Wharton's jelly mesenchymal stem cell transplantation in patients with type 2 diabetes mellitus. Stem Cell Res Ther. (2014) 5:57. doi: 10.1186/scrt446
104. Aboody KS, Najbauer J, Metz MZ, D'Apuzzo M, Gutova M, Annala AJ, et al. Neural stem cell-mediated enzyme/prodrug therapy for glioma: preclinical studies. Sci Transl Med. (2013) 5:184ra59. doi: 10.1126/scitranslmed.3005365
105. Orozco L, Munar A, Soler R, Alberca M, Soler F, Huguet M, et al. Treatment of knee osteoarthritis with autologous mesenchymal stem cells: a pilot study. Transplantation. (2013) 95:1535–41. doi: 10.1097/TP.0b013e318291a2da
106. Trounson A, McDonald C. Stem cell therapies in clinical trials: progress and challenges. Cell Stem Cell. (2015) 17:11–22. doi: 10.1016/j.stem.2015.06.007
107. Abbaspanah B, Momeni M, Ebrahimi M, Mousavi SH. Advances in perinatal stem cells research: a precious cell source for clinical applications. Regen Med. (2018) 13:595–610. doi: 10.2217/rme-2018-0019
108. Chambers DC, Enever D, Ilic N, Sparks L, Whitelaw K, Ayres J, et al. A phase 1b study of placenta-derived mesenchymal stromal cells in patients with idiopathic pulmonary fibrosis. Respirology. (2014) 19:1013–8. doi: 10.1111/resp.12343
109. Mayer L, Pandak WM, Melmed GY, Hanauer SB, Johnson K, Payne D, et al. Safety and tolerability of human placenta-derived cells (PDA001) in treatment-resistant crohn's disease: a phase 1 study. Inflamm Bowel Dis. (2013) 19:754–60. doi: 10.1097/MIB.0b013e31827f27df
110. Lublin FD, Bowen JD, Huddlestone J, Kremenchutzky M, Carpenter A, Corboy JR, et al. Human placenta-derived cells (PDA-001) for the treatment of adults with multiple sclerosis: a randomized, placebo-controlled, multiple-dose study. Mult Scler Relat Disord. (2014) 3:696–704. doi: 10.1016/j.msard.2014.08.002
111. Khalifeh Soltani S, Forogh B, Ahmadbeigi N, Hadizadeh Kharazi H, Fallahzadeh K, Kashani L, et al. Safety and efficacy of allogenic placental mesenchymal stem cells for treating knee osteoarthritis: a pilot study. Cytotherapy. (2019) 21:54–63. doi: 10.1016/j.jcyt.2018.11.003
112. Meller D, Pires RT, Mack RJ, Figueiredo F, Heiligenhaus A, Park WC, et al. Amniotic membrane transplantation for acute chemical or thermal burns. Ophthalmology. (2000) 107:980–9. doi: 10.1016/S0161-6420(00)00024-5
113. Momeni M, Fallah N, Bajouri A, Bagheri T, Orouji Z, Pahlevanpour P, et al. A randomized, double-blind, phase I clinical trial of fetal cell-based skin substitutes on healing of donor sites in burn patients. Burns. (2019) 45:914–22. doi: 10.1016/j.burns.2018.10.016
114. Prakoeswa CRS, Natallya FR, Harnindya D, Thohiroh A, Oktaviyanti RN, Pratiwi KD, et al. The efficacy of topical human amniotic membrane-mesenchymal stem cell-conditioned medium (hAMMSC-CM) and a mixture of topical hAMMSC-CM + vitamin C and hAMMSC-CM + vitamin E on chronic plantar ulcers in leprosy:a randomized control trial. J Dermatolog Treat. (2018) 29:835–840. doi: 10.1080/09546634.2018.1467541
115. Prakoeswa CRS, Pratiwi FD, Herwanto N, Citrashanty I, Indramaya DM, Murtiastutik D, et al. The effects of amniotic membrane stem cell-conditioned medium on photoaging. J Dermatolog Treat. (2019) 30:478–82. doi: 10.1080/09546634.2018.1530438
116. Bajestan MN, Rajan A, Edwards SP, Aronovich S, Cevidanes LHS, Polymeri A, et al. Stem cell therapy for reconstruction of alveolar cleft and trauma defects in adults: a randomized controlled, clinical trial. Clin Implant Dent Relat Res. (2017) 19:793–801. doi: 10.1111/cid.12506
117. Gjerde C, Mustafa K, Hellem S, Rojewski M, Gjengedal H, Yassin MA, et al. Cell therapy induced regeneration of severely atrophied mandibular bone in a clinical trial. Stem Cell Res Ther. (2018) 9:213. doi: 10.1186/s13287-018-0951-9
118. Khojasteh A, Kheiri L, Behnia H, Tehranchi A, Nazeman P, Nadjmi N, et al. Lateral ramus cortical bone plate in alveolar cleft osteoplasty with concomitant use of buccal fat pad derived cells and autogenous bone: phase i clinical trial. Biomed Res Int. (2017) 2017:6560234. doi: 10.1155/2017/6560234
119. Ferrarotti F, Romano F, Gamba MN, Quirico A, Giraudi M, Audagna M, et al. Human intrabony defect regeneration with micrografts containing dental pulp stem cells: a randomized controlled clinical trial. J Clin Periodontol. (2018) 45:841–50. doi: 10.1111/jcpe.12931
Keywords: hAMSCs, paracrine functions, endogenous bone regeneration, arthritis, bone defects, clinical trials
Citation: Li J, Zhou Z, Wen J, Jiang F and Xia Y (2020) Human Amniotic Mesenchymal Stem Cells Promote Endogenous Bone Regeneration. Front. Endocrinol. 11:543623. doi: 10.3389/fendo.2020.543623
Received: 17 March 2020; Accepted: 27 August 2020;
Published: 02 October 2020.
Edited by:
Lilian Irene Plotkin, Indiana University Bloomington, United StatesCopyright © 2020 Li, Zhou, Wen, Jiang and Xia. This is an open-access article distributed under the terms of the Creative Commons Attribution License (CC BY). The use, distribution or reproduction in other forums is permitted, provided the original author(s) and the copyright owner(s) are credited and that the original publication in this journal is cited, in accordance with accepted academic practice. No use, distribution or reproduction is permitted which does not comply with these terms.
*Correspondence: Fei Jiang, bnlrcWpmQG5qbXUuZWR1LmNu; Yang Xia, eGlheWFuZ0Buam11LmVkdS5jbg==
†These authors have contributed equally to this work
Disclaimer: All claims expressed in this article are solely those of the authors and do not necessarily represent those of their affiliated organizations, or those of the publisher, the editors and the reviewers. Any product that may be evaluated in this article or claim that may be made by its manufacturer is not guaranteed or endorsed by the publisher.
Research integrity at Frontiers
Learn more about the work of our research integrity team to safeguard the quality of each article we publish.