- 1Instituto de Inmunología, Genética y Metabolismo (INIGEM), Universidad de Buenos Aires, CONICET, Buenos Aires, Argentina
- 2Instituto de Investigaciones Biomédicas en Retrovirus y Sida (INBIRS), Universidad de Buenos Aires, CONICET, Buenos Aires, Argentina
The human immunodeficiency virus type 1 (HIV)/AIDS pandemic represents the most significant global health challenge in modern history. This infection leads toward an inflammatory state associated with chronic immune dysregulation activation that tilts the immune-skeletal interface and its deep integration between cell types and cytokines with a strong influence on skeletal renewal and exacerbated bone loss. Hence, reduced bone mineral density is a complication among HIV–infected individuals that may progress to osteoporosis, thus increasing their prevalence of fractures. Highly active antiretroviral therapy (HAART) can effectively control HIV replication but the regimens, that include tenofovir disoproxil fumarate (TDF), may accelerate bone mass density loss. Molecular mechanisms of HIV-associated bone disease include the OPG/RANKL/RANK system dysregulation. Thereby, osteoclastogenesis and osteolytic activity are promoted after the osteoclast precursor infection, accompanied by a deleterious effect on osteoblast and its precursor cells, with exacerbated senescence of mesenchymal stem cells (MSCs). This review summarizes recent basic research data on HIV pathogenesis and its relation to bone quality. It also sheds light on HAART-related detrimental effects on bone metabolism, providing a better understanding of the molecular mechanisms involved in bone dysfunction and damage as well as how the HIV-associated imbalance on the gut microbiome may contribute to bone disease.
Introduction
According to UNAIDS, 37.9 million people worldwide are currently living with HIV/AIDS and about 22 million are on highly active antiretroviral therapy (HAART). The life expectancy of HIV-infected individuals treated with HAART is nearly normal, with a decreased incidence in AIDS-related morbidity and mortality (1).
Low bone mineral density (BMD) has frequently been observed among HIV-infected individuals, likely leading to osteopenia and osteoporosis with a high prevalence of fractures compared with the general population (2).
In HIV-infected patients, bone loss is primarily enhanced by two pivotal factors: HIV infection and its direct consequences, and HAART, mainly during the first years of treatment (3–8). The contribution of each one is still controversial. The evidence of reduced bone mass in treatment-naïve patients indicates that the virus alone directly affects bone homeostasis (9–14). Moreover, some reports indicate that low BMD is not completely attributable to HIV infection alone or HIV infection plus treatments with HAART (15–23).
The bone as part of the skeletal system interacts with immune cells in the bone marrow, interacting with each other in a significant mutual influence (24). Recently, the molecular mechanisms involved in the homeostatic interactions between bone and immune cells has been elucidated (25–27), which HIV appears to be able to disturb.
Microbiota Contribution in HIV Interaction With Bone
HIV Proteins
HIV genes encode regulatory, auxiliary, and structural proteins. The regulatory proteins include the HIV trans-activator (Tat) involved in the regulation of the reverse transcription of the viral genome, and the regulator of expression of virion proteins (Rev) responsible for the synthesis of major viral proteins. The auxiliary HIV proteins comprise the negative factor (Nef), which is implicated in multiple functions during the viral replication cycle, including, among other functions, the lentivirus protein R (Vpr) responsible for nuclear import of the pre-integration complex. It is also comprised of the viral infectivity factor (Vif) required to synthesize infectious viruses in several human cells and the virus protein U (Vpu) the main role of which is the successful release of virions from infected cells. The structural proteins included the group-specific antigen (p55 gag polyprotein), a polyprotein which is processed by viral proteases during maturation to matrix protein (p17), capsid protein (p24), spacer peptide 1 (p2), nucleocapsid protein (p7), spacer peptide 2 (p1), and P6 protein. Other structural proteins involve the polymerase (Pol) and the envelope protein (gp160) that is post-translationally processed to produce the surface glycoprotein (gp120) and gp41 that mediate binding to the CD4 receptor, and envelope fusion to target cells, respectively (28).
Interaction of HIV and Its Proteins With Bone Cells
Among many of the viral pathogenic mechanisms, HIV regulatory, auxiliary, and structural proteins play critical roles during cell-host interaction and thus have shown significant impacts on bone in experimental studies, promoting changes in the balance of bone formation and resorption. It is important to highlight that the HIV-induced detrimental effects on cells are not only a consequence of the active viral replication and the role of infectious virions but are also caused by several HIV proteins that are released to extracellular media which could induce bystander harmful effects, such as apoptosis, oxidative stress, mitochondrial dysfunctions, or autophagy alterations, on surrounding cells (29, 30).
Mesenchymal stem cells (MSCs) are multipotent precursors able to differentiate toward multiple tissue lineages such as adipocytes, chondroblasts, and osteoblasts (31, 32). As MSCs express CD4 receptors and CCR5 and CXCR4 coreceptors, these cells are likely susceptible to HIV infection, although integrated proviruses are rarely found and productive infection has not yet been documented (33). Nonetheless, hematopoietic progenitor cells (HPCs) in the bone marrow of HIV-infected individuals have been regarded as a persistent HIV reservoir (34).
Differentiation of MSCs ex vivo into both osteoblasts and adipocytes depicted a dichotomy upon exposure to the serum source, since those in contact with a high HIV viral load preferentially acquired a proadipogenic phenotype whereas those in contact with low viral load serum were induced toward an osteogenic condition. This phenomenon may involve Tat protein, which inhibits the transcription factor COUP TF-I (chicken ovalbumin upstream promoter transcription factor), thus favoring adipocyte differentiation while preventing osteoblast development.
To command the balance of bone resorption and formation, osteoblasts produce a receptor activator factor of nuclear factor-kB ligand (RANKL) that controls the differentiation of osteoclasts (35). Osteocytes -the terminally differentiated form of osteoblast- also produce RANKL to regulate osteoclast activity (36). Under physiological conditions, osteoclastogenesis involves RANKL and macrophage colony-stimulating factor (M-CSF) produced by osteoblast and bone marrow stromal cells (37). M-CSF prompts the expression of RANKL receptor (RANK), on osteoclast precursor which then interacts with RANKL to initiate osteoclasts' differentiation (38). As a counterpart, osteoprotegerin (OPG) is a neutralizing soluble trap receptor expressed by bone marrow stromal cells and osteoblasts able to inhibit the RANKL-RANK interaction (39).
The Tat protein enhances peripheral blood monocyte-derived osteoclast differentiation and activity by RANKL plus M-CSF treatment, which increases both the mRNA transcription of specific osteoclast differentiation markers, such as cathepsin K and calcitonin receptor, and the tartrate-resistant acidic phosphatase (TRAP) expression and activity. Together, these results show that Tat may be considered a viral factor that stimulates osteoclastogenesis and bone resorption activity (11, 40–42). In vitro, Tat and Nef proteins reduce -in a cumulative manner- the number of bone marrow MSCs available to differentiate into osteoblasts by inducing early senescence, associated with increased oxidative stress and mitochondrial dysfunction of these cells. Moreover, Tat, but not Nef, induced an early increase in NF-κB activity and cytokine/chemokine secretion, and reciprocally, Nef- but no Tat-treated cells -have shown early autophagy inhibition (43).
The HIV accessory protein Vpr upregulates the RANKL expression in peripheral mononuclear cells from healthy donors, enhancing osteoclastic activity. This action is synergized by both exogenous and endogenous glucocorticoids as a potent cofactor in bone mineral loss (44). Moreover, Tat and Rev proteins increase monocyte differentiation into osteoclasts, as well as boost osteoclast resorption function by increasing reactive oxygen species and TNF-α production in osteoclast precursors (45, 46).
In an in vivo humanized mice and ex vivo human joint tissue study, Raynaud-Messina et al. have contributed to our current understanding of the HIV-induced bone loss mechanisms. For the first time, the authors demonstrated that HIV infects osteoclast precursors even at different stages of osteoclastogenesis, either via cell-free viruses or, more efficiently, through transfer from infected T cells. These infected precursor cells have been proposed as HIV reservoirs that display a greater migratory capacity and exhibit the enhanced ability to recruit and concentrate in the bones where the viral infection alters the bone resorption machinery. HIV can enlarge podosomes and enhance the osteolytic activity of the bone resorption apparatus, also known as the “sealing zone” (SZ). The virus is also able to increase the TRAP secretion by osteoclasts, leading to demineralization and degradation of larger bone extensions. These viral-directed actions are Nef-mediated and are abundantly produced and secreted during the early phase of viral replication. Such Nef-mediated actions occur through the activation of Src, which regulates podosomes into the SZ (47).
Soluble HIV-structural proteins are also mediators of cytopathogenic effects. These proteins may act as part of the viral particle or as bystander effect mediators after their release from productively infected cells (48). Both, p55-gag and gp120 were found to reduce calcium deposition, alkaline-phosphatase activity, levels of secreted BMP-2, -7, and RANKL, as well as the expression and activity of the pro-osteogenic transcription factor runt-related transcription factor 2 (RUNX2) in human osteoblasts. The levels of osteocalcin were also significantly reduced by p55-gag treatment, while gp120 also increased the pro-adipogenic transcription factor and peroxisome proliferator-activated receptor γ (PPARγ) activity. The ability of MSCs to develop into functioning osteoblasts was also affected by the presence of HIV proteins, with p55-gag inducing a decrease in osteogenesis, while rev induced an increase (49). A positive feedback loop exists between RANKL production and HIV replication, which may be relevant to both the pathophysiology of HIV-linked osteopenia and the control of HIV replication (50).
Furthermore, HIV gp120 can trigger in vitro osteoblast apoptosis induction mediated by the up-regulation of TNF–α (51). In these cells, gp120 enhances the expression of Dickkopf-1 (Dkk1), the antagonist of the Wnt, significantly reducing the intracytosolic and intranuclear β-catenin expression, the alkaline phosphatase activity, and the cell proliferation (52).
In HIV-infected individuals, B and T lymphocytes have exhibited several signs of dysfunction with an impact on bone homeostasis. They are sources of OPG and, consequently, their dysfunction contributes to viral-induced bone loss. Hence, there is a higher frequency of RANKL-expressing B cells (resting memory and exhausted tissue-like memory B cells) expanded as a consequence of inflammation and a lower frequency of OPG-expressing B cells (resting memory B cells) in HIV-infected compared to HIV-uninfected individuals, thus resulting in a lower RANKL/OPG ratio that correlates with total hip BMD, T-, and Z-scores in the HIV-infected participants (14).
Similarly, T-cell OPG production was also significantly lower in CD4 T-cell-sufficient HIV-infected individuals (>200 cells/μl) but not in those with lower cell counts. It was coupled with moderately higher T-cell RANKL production, resulting in a significantly higher T-cell RANKL/OPG ratio. Such a T-cell RANKL/OPG lowered ratio correlated significantly with BMD-derived z-scores at the hip, lumbar spine, and femur neck (53). Moreover, as a bystander effect, such an abnormal RANKL expression by T cells is mimicked when these cells are exposed to soluble gp120 (Figure 1) (54).
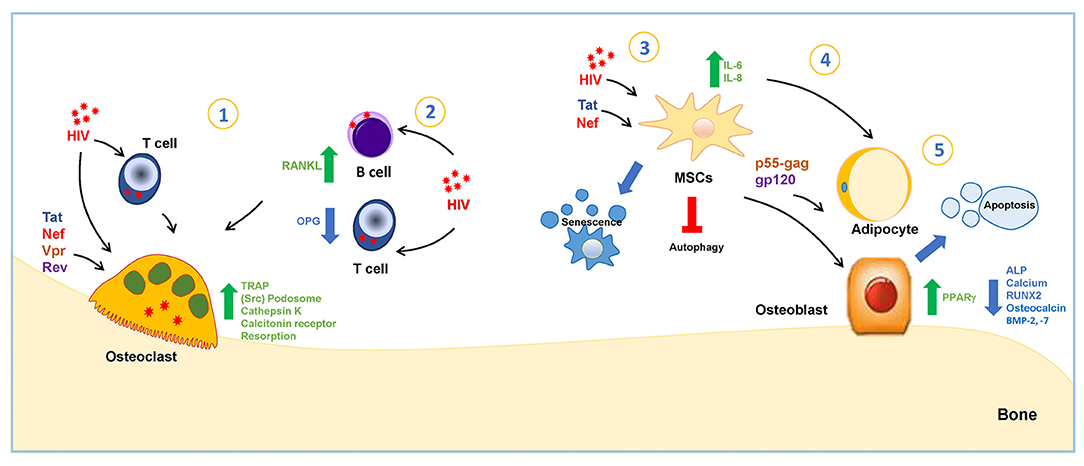
Figure 1. HIV and viral proteins' interaction with bone cells. 1-HIV infects osteoclasts via cell-free viruses or by cell-to-cell transfer from infected T cells. The infection increases the tartrate-resistant acidic phosphatase (TRAP) secretion by osteoclasts. The viral protein Tat increases mRNA transcription of cathepsin K, calcitonin receptor, TRAP, and Nef-regulate podosomes through activation of Src. Vpr upregulates RANKL expression, stimulating osteoclastogenesis. Tat and Rev increase osteoclastogenesis. 2-HIV infection induces an increase in RANKL-expression and the reduction of OPG-expression in B and T cells. 3-HIV proteins Tat and Nef reduce the number of bone marrow MSCs by inducing early senescence. Tat stimulates MSC to secrete IL-6 and IL-8, and Nef induces the inhibition of autophagy. 4-Human serum with a high HIV viral load preferentially acquired a proadipogenic phenotype in a mechanism dependent on Tat protein, while those in contact with a low viral load serum were induced toward osteogenic conditions. 5-p55-gag and gp120 stimulate osteoblast apoptosis and reduce alkaline-phosphatase activity (ALP), calcium deposition, the runt-related transcription factor 2 (RUNX-2), and Bone morphogenic protein-2 and−7 (BMP-2−7), and p55-gag also reduces osteocalcin levels, and gp120 induces the increase in peroxisome proliferator-activated receptor γ (PPARγ).
HIV-Related Gut Microbiome Alterations and Its Relationship With Bone Loss
Recently, the gut microbiota has been reported to have an influence on bone metabolism, attracting attention as a prospective new target to balance BMD. The basis of this evidence is mainly concentrated on its involvement in modulating the interface between the immune system and bone cells (55, 56).
As an early event, the gut microbiome in HIV-infected individuals exhibits different compositions compared to uninfected individuals (57, 58). Among them, the bacterial composition is altered on its diversity, genes, and functional capabilities, that are either pro-inflammatory or potentially pathogenic and whose abundance correlated with immune status (59, 60). T-cell depletion is pronounced at the gut-associated lymphoid tissue (GALT) promptly after HIV infection, followed by an increase in the barrier permeability and microbial translocation with increased LPS levels (61). This context induces an innate immune activation leading to a shift toward a pro-inflammatory cytokine environment with osteoclastogenesis and bone resorption enhancement (62, 63).
Since chronic immune activation with progressive immune suppression impacts on the gut microbiome, a differential contribution of gut bacteria and their molecular agents (metabolites and proteins) is desirable to promote immune recovery in HIV-infected individuals. Hence, after characterizing the interplay between the active gut microbiota and the host, it is plausible to reduce inflammation and recover the immune–skeletal interface (64–66).
The HAART treatment effect on gut microbiota in HIV patients is uncertain (67). One hypothesis is that HIV treatment stimulates the restoration of normal microbial flora (68). However, some studies show a minimal effect of HAART on the restoration of normal microbial flora (68–70) while others reveal a negative impact (71).
Role of Antiretroviral Therapy on Bone Tissue Metabolism
The widespread accessibility of HAART has changed HIV from a life-limiting condition to one with a near-normal life expectancy. Unexpectedly, throughout such a therapy, the bone loss promoted by HIV-infection may continue unabated. However, among HIV-infected individuals on HAART, the presence of osteoporosis appears to be about three times higher than those uninfected (3–8). Although, far from consensus, other reports have estimated up to a 6% decrease in BMD upon HAART treatment initiation for a 2-year period, but then the BMD remains unchanged despite continuing therapy (72–74).
As mentioned above, in naïve immunosuppressed HIV-infected individuals a decrease in BMD is observed. Paradoxically, when these individuals are on HAART they achieve their immune reconstitution by CD4+ T cell repopulation (75, 76). These reports offer evidence of stable or increasing BMD with plausible early, but small and not sustained, loss of BMD that accompanies the initiation of HAART, and without accelerated bone loss in the medium term (77–82).
The gender of the HIV-infected individual also influences the BMD reduction grade. Among HAART-treated patients, it appears to be more accentuated in women than in men (83, 84), but is at a level similar to that observed initially during menopause (85).
Several studies have directly emphasized HIV factors associated with low BMD: duration of infection, HIV viral burden, and a more advanced HIV disease (86–88). In this regard, data presented in a sub-study of the Strategy for Management of Antiretroviral Therapy (SMART) study demonstrated a low level of bone turnover markers but higher BMD when HAART is interrupted, thus inferring a higher HIV RNA level and lower CD4+ T cell counts (89). In contrast, Grund et al. have reported that continuous HAART was associated with significant reductions in BMD with no changes or increases in BMD observed in those on intermittent ART (90). Similarly, longitudinal data collected from randomized control trials have insinuated that the initiation of HAART at higher viral RNA and lower CD4+ T cell counts at baseline were associated with more pronounced reductions in BMD (88). Such low pre-treatment CD4 counts were reported as a strong and independent risk factor for loss of BMD during treatment. However, loss of bone continues for up to 2 years after HAART initiation and the extent of immune reconstitution was not related to BMD improvement (88). In conjunction, these data suggest that important roles are played directly by HIV and/or indirectly by the immune response in BMD loss.
The effect of HAART on BMD seems to be influenced by the specific type of treatment. Low BMD has been associated with regimens such as nucleoside analog reverse-transcriptase inhibitors (NRTIs) (74, 91, 92). Individuals exposed to tenofovir disoproxil fumarate (TDF)-based treatment in particular exhibited a more accentuated BMD loss compared to individuals on other regimens, such as lamivudine (3TC) and emtricitabine (FTC), or those who have been switched to two-drug regimens (74, 91–97). However, others have reported contradictory findings regarding TDF-therapy duration and BMD loss, even after long-term exposure to the drug (98).
The underlying mechanisms by which antiretroviral drugs promote BMD loss are still controversial. The mechanism to NRTIs-mediated BMD loss may be promoted by elevated lactic acid concentration in the blood leading to calcium hydroxyapatite loss, especially in the trabecular bone, due to the labile of calcium storage (99). Regarding the underlying mechanisms that may be related to TDF-associated lower BMD, mitochondrial toxicity, hyperphosphaturia secondary to tubular dysfunction, and renal osteodystrophy have been considered (92, 100–102). Despite the bone loss, there are contradictory findings about phosphate metabolism abnormalities observed among HAART-treated individuals who can present higher phosphate blood levels and lower bone density (86). These data offer supportive information to avoid the use of TDF and its replacement with bone-friendly regimens among the HIV-infected population with fracture risks (103).
Besides the BMD reduction related to NRTIs, available data regarding protease inhibitors (PIs) remain contradictory (104). On the one hand, increased bone turnover, accelerated bone loss, and a higher prevalence of reduced BMD have been reported (3, 72, 92, 105–107), whereas other studies showed opposed results (9, 10, 73, 108). Detrimental effects on BMD are in line with in vitro observations evaluating the effect of different PIs on osteoblast activity (109). For example, pharmacologic levels of two PIs that are clinically linked to osteopenia, ritonavir (RTV) and saquinavir (SQV) but not indinavir (IDV) and nelfinavir (NFV), abolish the interferon-γ-mediated degradation of the RANKL signaling adapter protein TRAF6 (tumor necrosis factor receptor-associated protein 6) in proteasomes. Moreover, under inflammatory conditions, interferon-γ promotes bone loss mainly by up-regulating the activity of macrophages, leading to T cell activation and osteoclastogenic cytokine production (110).
RTV appears as an osteoclast-activating agent that promotes the proliferation and activation of osteoclasts in vitro (111, 112) and ex vivo studies (113), causing increased bone absorption.
Importantly, most of these in vitro direct effects of PIs on bone cells did not resemble the in vivo observations collected from patients on HAART. RTV, SQV, and fosamprenavir (FPV) appear to improve the BMD in vitro rather than the loss observed in vivo, by decreasing RANKL and increasing OPG secretion (54, 109). The impact on BMD loss was also reported in several in vivo studies which also observed a strong difference in bone loss according to PI discontinued and continued schemes between patients (72, 92, 107). RTV -but not IDV- at a greater than normal concentration was able to inhibit osteoclast function and suppress osteoclastogenesis in vitro and in vivo by impairing RANKL-induced signaling (114). However, RTV at plasma concentration, as a PI-boosting drug, favors the differentiation of blood monocytes into osteoclasts by up-regulating the production of transcripts for osteoclast growth factors using the non-canonical Wnt proteins 5B and 7B as well as activated promoters of nuclear factor-kappaB signaling, but suppressing genes involved in canonical Wnt signaling. Additionally, RTV blocks the cytoplasmic-to-nuclear translocation of β-catenin, the molecular node of the Wnt signaling pathway, in association with enhanced β-catenin ubiquitination (111, 112). In vivo, among RTV-treated patients, its discontinuation resulted in a slower decrease in BMD (107), and the bone mineral loss appeared in a time-dependent manner irrespective of dosage (107). Other PIs, such as IDV and NFV, have been shown to have a negative impact on osteoblasts by impairing its alkaline phosphatase activity and calcium deposition. Lastly, in vivo and in vitro studies demonstrate that PIs atazanavir (ATV) and lopinavir (LPV) also decrease BMD by impairing the MSCs differentiation to osteoblasts (72, 92, 115).
Finally, in addition to immune cells, the HIV-coreceptor CCR5 has been involved in the regulation of the function of bone cells by directly modulating osteoclastogenesis and the communication between osteoclasts and osteoblasts (116–118). In this regard, epidemiological evidence suggests that the functional loss of CCR5 is correlated with a lower incidence of bone-destructive diseases as well as of HIV transmission. Using a CCR5-deficient murine model, the osteoclasts appeared dysfunctional in their cellular locomotion and bone-resorption activity, which is associated with the disarrangement of podosomes and adhesion complex molecules including Pyk2. Such an experimental model exhibited an osteoporosis-resistance induced by RANKL (119). These data are in line with a previous study showing the CCR5-antagonist Maraviroc associated with a lower degree of bone loss in the hip and lumbar spine of HIV-infected individuals, as an example of a CCR5-antagonist treatment that might help to improve bone health among HIV-infected patients (120).
In conclusion, important progress has been made in our understanding of the effect of antiretroviral drugs on bone health in HIV-infected people. Such advances have enriched our ability to apply treatment to diminish aging-associated complications, such as osteoporosis and fractures.
Concluding Remarks
During HIV infection and its progression to AIDS, bone loss occurs and HAART likely contributes -at least in part- to this comorbidity, involving both factors associated with disease reversal and direct skeletal effects. Although the clinical and imaging characterization of HIV bone pathology has been well-documented, the pathogenic mechanisms of bone loss have only been partially elucidated at present.
Irrespective of the mechanisms involved, diagnostic and therapeutic measures are necessary to delay the onset of bone disease in HIV patients to prevent a significant new threat to the health of the HIV/AIDS population.
Author Contributions
MD and JQ conceived the idea and drafted the manuscript. All authors contributed to the article and approved the submitted version.
Funding
This work was supported by grant from Agencia Nacional of Promoción Científica y Tecnológica (ANPCYT, Argentina) PICT 2015-0316.
Conflict of Interest
The authors declare that the research was conducted in the absence of any commercial or financial relationships that could be construed as a potential conflict of interest.
References
1. Back D, Marzolini C. The challenge of HIV treatment in an era of polypharmacy. J Int AIDS Soc. (2020) 23:e25449. doi: 10.1002/jia2.25449
2. Cotter AG, Mallon PW. The effects of untreated and treated HIV infection on bone disease. Curr Opinion HIV AIDS. (2014) 9:17–26. doi: 10.1097/COH.0000000000000028
3. Brown TT, Qaqish RB. Antiretroviral therapy and the prevalence of osteopenia and osteoporosis: a meta-analytic review. Aids. (2006) 20:2165–74. doi: 10.1097/QAD.0b013e32801022eb
4. Powderly WG. Osteoporosis and bone health in HIV. Current HIV/AIDS Reports. (2012) 9:218–22. doi: 10.1007/s11904-012-0119-7
5. Maffezzoni F, Porcelli T, Karamouzis I, Quiros-Roldan E, Castelli F, Mazziotti G, et al. Osteoporosis in human immunodeficiency virus patients - an emerging clinical concern. Eur Endocrinol. (2014) 10:79–83. doi: 10.17925/EE.2014.10.01.79
6. Cervero M, Torres R, Agud JL, Alcazar V, Jusdado JJ, Garcia-Lacalle C, et al. Prevalence of and risk factors for low bone mineral density in Spanish treated HIV-infected patients. PLoS ONE. (2018) 13:e0196201. doi: 10.1371/journal.pone.0196201
7. Calmy A, Chevalley T, Delhumeau C, Toutous-Trellu L, Spycher-Elbes R, Ratib O, et al. Long-term HIV infection and antiretroviral therapy are associated with bone microstructure alterations in premenopausal women. Osteoporos Int. (2013) 24:1843–52. doi: 10.1007/s00198-012-2189-1
8. Dalla Grana E, Rigo F, Lanzafame M, Lattuada E, Suardi S, Mottes M, et al. Relationship between vertebral fractures, bone mineral density, and osteometabolic profile in HIV and hepatitis B and C-infected patients treated with ART. Front Endocrinol. (2019) 10:302. doi: 10.3389/fendo.2019.00302
9. Arnsten JH, Freeman R, Howard AA, Floris-Moore M, Santoro N, Schoenbaum EE. HIV infection and bone mineral density in middle-aged women. Clin Infect Diseases. (2006) 42:1014–20. doi: 10.1086/501015
10. Bruera D, Luna N, David DO, Bergoglio LM, Zamudio J. Decreased bone mineral density in HIV-infected patients is independent of antiretroviral therapy. Aids. (2003) 17:1917–23. doi: 10.1097/00002030-200309050-00010
11. Gibellini D, Borderi M, De Crignis E, Cicola R, Vescini F, Caudarella R, et al. RANKL/OPG/TRAIL plasma levels and bone mass loss evaluation in antiretroviral naive HIV-1-positive men. J Med Virol. (2007) 79:1446–54. doi: 10.1002/jmv.20938
12. Teichmann J, Stephan E, Lange U, Discher T, Friese G, Lohmeyer J, et al. Osteopenia in HIV-infected women prior to highly active antiretroviral therapy. J Infect. (2003) 46:221–7. doi: 10.1053/jinf.2002.1109
13. Wattanachanya L, Jantrapakde J, Avihingsanon A, Ramautarsing R, Kerr S, Trachunthong D, et al. Antiretroviral-naive HIV-infected patients had lower bone formation markers than HIV-uninfected adults. AIDS Care. (2020) 32:984–93. doi: 10.1080/09540121.2019.1622631
14. Titanji K, Vunnava A, Sheth AN, Delille C, Lennox JL, Sanford SE, et al. Dysregulated B cell expression of RANKL and OPG correlates with loss of bone mineral density in HIV infection. PLoS Pathog. (2014) 10:e1004497. doi: 10.1371/journal.ppat.1004497
15. Grijsen ML, Vrouenraets SM, Steingrover R, Lips P, Reiss P, Wit FW, et al. High prevalence of reduced bone mineral density in primary HIV-1-infected men. Aids. (2010) 24:2233–8. doi: 10.1097/QAD.0b013e32833c93fe
16. Grijsen ML, Vrouenraets SM, Wit FW, Stolte IG, Prins M, Lips P, et al. Low bone mineral density, regardless of HIV status, in men who have sex with men. J Infect Dis. (2013) 207:386–91. doi: 10.1093/infdis/jis687
17. Bolland MJ, Grey A, Reid IR. Skeletal health in adults with HIV infection. Lancet Diabetes Endocrinol. (2015) 3:63–74. doi: 10.1016/S2213-8587(13)70181-5
18. Jimenez B, Sainz T, Diaz L, Mellado MJ, Navarro ML, Rojo P, et al. Low bone mineral density in vertically HIV-infected children and adolescents: risk factors and the role of t-cell activation and senescence. Pediatric Infect Disease J. (2017) 36:578–83. doi: 10.1097/INF.0000000000001506
19. Jones S, Restrepo D, Kasowitz A, Korenstein D, Wallenstein S, Schneider A, et al. Risk factors for decreased bone density and effects of HIV on bone in the elderly. Osteoporos Int. (2008) 19:913–8. doi: 10.1007/s00198-007-0524-8
20. Knobel H, Guelar A, Vallecillo G, Nogues X, Diez A. Osteopenia in HIV-infected patients: is it the disease or is it the treatment? Aids. (2001) 15:807–8. doi: 10.1097/00002030-200104130-00022
21. Starup-Linde J, Rosendahl SB, Storgaard M, Langdahl B. Management of osteoporosis in patients living with HIV-A systematic review and meta-analysis. J Acquired Immune Deficiency Syndromes. (2020) 83:1–8. doi: 10.1097/QAI.0000000000002207
22. Yin M, Dobkin J, Brudney K, Becker C, Zadel JL, Manandhar M, et al. Bone mass and mineral metabolism in HIV+ postmenopausal women. Osteoporos Int. (2005) 16:1345–52. doi: 10.1007/s00198-005-1845-0
23. Tebas P, Powderly WG, Claxton S, Marin D, Tantisiriwat W, Teitelbaum SL, et al. Accelerated bone mineral loss in HIV-infected patients receiving potent antiretroviral therapy. Aids. (2000) 14:F63–7. doi: 10.1097/00002030-200003100-00005
24. Tsukasaki M, Takayanagi H. Osteoimmunology: evolving concepts in bone-immune interactions in health and disease. Nat Rev Immunol. (2019) 19:626–42. doi: 10.1038/s41577-019-0178-8
25. Takayanagi H. Osteoimmunology - bidirectional dialogue and inevitable union of the fields of bone and immunity. Proc Jpn Acad Ser B Phys Biol Sci. (2020) 96:159–69. doi: 10.2183/pjab.96.013
27. Takayanagi H, Ogasawara K, Hida S, Chiba T, Murata S, Sato K, et al. T-cell-mediated regulation of osteoclastogenesis by signalling cross-talk between RANKL and IFN-gamma. Nature. (2000) 408:600–5. doi: 10.1038/35046102
28. German Advisory Committee Blood SAoPTbB. Human immunodeficiency virus (HIV). Transfus Med Hemother. (2016) 43:203–22. doi: 10.1159/000445852
29. Liu Z, Xiao Y, Torresilla C, Rassart E, Barbeau B. Implication of different HIV-1 genes in the modulation of autophagy. Viruses. (2017) 9:389. doi: 10.3390/v9120389
30. Welch JL, Stapleton JT, Okeoma CM. Vehicles of intercellular communication: exosomes and HIV-1. J Gen Virol. (2019) 100:350–66. doi: 10.1099/jgv.0.001193
31. Verma S, Rajaratnam JH, Denton J, Hoyland JA, Byers RJ. Adipocytic proportion of bone marrow is inversely related to bone formation in osteoporosis. J Clin Pathol. (2002) 55:693–8. doi: 10.1136/jcp.55.9.693
32. Kim BS, Kim JS, Sung HM, You HK, Lee J. Cellular attachment and osteoblast differentiation of mesenchymal stem cells on natural cuttlefish bone. J Biomed Mater Res A. (2012) 100:1673–9. doi: 10.1002/jbm.a.34113
33. Nazari-Shafti TZ, Freisinger E, Roy U, Bulot CT, Senst C, Dupin CL, et al. Mesenchymal stem cell derived hematopoietic cells are permissive to HIV-1 infection. Retrovirology. (2011) 8:3. doi: 10.1186/1742-4690-8-3
34. McNamara LA, Onafuwa-Nuga A, Sebastian NT, Riddell Jt, Bixby D, Collins KL. CD133+ hematopoietic progenitor cells harbor HIV genomes in a subset of optimally treated people with long-term viral suppression. J Infect Dis. (2013) 207:1807–16. doi: 10.1093/infdis/jit118
35. Karner CM, Long F. Wnt signaling and cellular metabolism in osteoblasts. Cell Mol Life Sci. (2017) 74:1649–57. doi: 10.1007/s00018-016-2425-5
36. Chen H, Senda T, Kubo KY. The osteocyte plays multiple roles in bone remodeling and mineral homeostasis. Med Mol Morphol. (2015) 48:61–8. doi: 10.1007/s00795-015-0099-y
37. Lampiasi N, Russo R, Zito F. The Alternative Faces of Macrophage Generate Osteoclasts. Biomed Res Int. (2016) 2016:9089610. doi: 10.1155/2016/9089610
38. Yasuda H, Shima N, Nakagawa N, Yamaguchi K, Kinosaki M, Mochizuki S, et al. Osteoclast differentiation factor is a ligand for osteoprotegerin/osteoclastogenesis-inhibitory factor and is identical to TRANCE/RANKL. Proc Natl Acad Sci USA. (1998) 95:3597–602. doi: 10.1073/pnas.95.7.3597
39. Grundt A, Grafe IA, Liegibel U, Sommer U, Nawroth P, Kasperk C. Direct effects of osteoprotegerin on human bone cell metabolism. Biochem Biophys Res Commun. (2009) 389:550–5. doi: 10.1016/j.bbrc.2009.09.026
40. Cotter EJ, Chew N, Powderly WG, Doran PP. HIV type 1 alters mesenchymal stem cell differentiation potential and cell phenotype ex vivo. AIDS Res Hum Retroviruses. (2011) 27:187–99. doi: 10.1089/aid.2010.0114
41. Gibellini D, De Crignis E, Ponti C, Borderi M, Clo A, Miserocchi A, et al. HIV-1 Tat protein enhances RANKL/M-CSF-mediated osteoclast differentiation. Biochem Biophys Res Commun. (2010) 401:429–34. doi: 10.1016/j.bbrc.2010.09.071
42. Caldwell RL, Gadipatti R, Lane KB, Shepherd VL. HIV-1 TAT represses transcription of the bone morphogenic protein receptor-2 in U937 monocytic cells. J Leukoc Biol. (2006) 79:192–201. doi: 10.1189/jlb.0405194
43. Beaupere C, Garcia M, Larghero J, Feve B, Capeau J, Lagathu C. The HIV proteins Tat and Nef promote human bone marrow mesenchymal stem cell senescence and alter osteoblastic differentiation. Aging Cell. (2015) 14:534–46. doi: 10.1111/acel.12308
44. Fakruddin JM, Laurence J. HIV-1 Vpr enhances production of receptor of activated NF-kappaB ligand (RANKL) via potentiation of glucocorticoid receptor activity. Arch Virol. (2005) 150:67–78. doi: 10.1007/s00705-004-0395-7
45. Chew N, Tan E, Li L, Lim R. HIV-1 tat and rev upregulates osteoclast bone resorption. J Int AIDS Soc. (2014) 17(4 Suppl. 3):19724. doi: 10.7448/IAS.17.4.19724
46. Agidigbi TS, Kim C. Reactive oxygen species in osteoclast differentiation and possible pharmaceutical targets of ROS-mediated osteoclast diseases. Int J Mol Sci. (2019) 20:3576. doi: 10.3390/ijms20143576
47. Raynaud-Messina B, Bracq L, Dupont M, Souriant S, Usmani SM, Proag A, et al. Bone degradation machinery of osteoclasts: an HIV-1 target that contributes to bone loss. Proc Natl Acad Sci USA. (2018) 115:E2556–65. doi: 10.1073/pnas.1713370115
48. Ran X, Ao Z, Trajtman A, Xu W, Kobinger G, Keynan Y, et al. HIV-1 envelope glycoprotein stimulates viral transcription and increases the infectivity of the progeny virus through the manipulation of cellular machinery. Sci Rep. (2017) 7:9487. doi: 10.1038/s41598-017-10272-7
49. Cotter EJ, Malizia AP, Chew N, Powderly WG, Doran PP. HIV proteins regulate bone marker secretion and transcription factor activity in cultured human osteoblasts with consequent potential implications for osteoblast function and development. AIDS Res Hum Retroviruses. (2007) 23:1521–30. doi: 10.1089/aid.2007.0112
50. Fakruddin JM, Laurence J. Interactions among human immunodeficiency virus (HIV)-1, interferon-gamma and receptor of activated NF-kappa B ligand (RANKL): implications for HIV pathogenesis. Clin Exp Immunol. (2004) 137:538–45. doi: 10.1111/j.1365-2249.2004.02568.x
51. Gibellini D, De Crignis E, Ponti C, Cimatti L, Borderi M, Tschon M, et al. HIV-1 triggers apoptosis in primary osteoblasts and HOBIT cells through TNFalpha activation. J Med Virol. (2008) 80:1507–14. doi: 10.1002/jmv.21266
52. Butler JS, Dunning EC, Murray DW, Doran PP, O'Byrne JM. HIV-1 protein induced modulation of primary human osteoblast differentiation and function via a Wnt/beta-catenin-dependent mechanism. J Orthop Res. (2013) 31:218–26. doi: 10.1002/jor.22196
53. Titanji K, Vunnava A, Foster A, Sheth AN, Lennox JL, Knezevic A, et al. T-cell receptor activator of nuclear factor-kappaB ligand/osteoprotegerin imbalance is associated with HIV-induced bone loss in patients with higher CD4+ T-cell counts. Aids. (2018) 32:885–94. doi: 10.1097/QAD.0000000000001764
54. Fakruddin JM, Laurence J. HIV envelope gp120-mediated regulation of osteoclastogenesis via receptor activator of nuclear factor kappa B ligand (RANKL) secretion and its modulation by certain HIV protease inhibitors through interferon-gamma/RANKL cross-talk. J Biol Chem. (2003) 278:48251–8. doi: 10.1074/jbc.M304676200
55. Yan J, Charles JF. Gut microbiome and bone: to build, destroy, or both? Curr Osteoporos Rep. (2017) 15:376–84. doi: 10.1007/s11914-017-0382-z
56. Locantore P, Del Gatto V, Gelli S, Paragliola RM, Pontecorvi A. The interplay between immune system and microbiota in osteoporosis. Mediators Inflamm. (2020) 2020:3686749. doi: 10.1155/2020/3686749
57. Mudd JC, Brenchley JM. Gut mucosal barrier dysfunction, microbial dysbiosis, and their role in HIV-1 disease progression. J Infect Dis. (2016) 214 (Suppl. 2):S58–66. doi: 10.1093/infdis/jiw258
58. Tuddenham SA, Koay WLA, Zhao N, White JR, Ghanem KG, Sears CL, et al. The impact of human immunodeficiency virus infection on gut microbiota alpha-diversity: an individual-level meta-analysis. Clin Infect Diseases. (2020) 70:615–27. doi: 10.1093/cid/ciz258
59. Vazquez-Castellanos JF, Serrano-Villar S, Latorre A, Artacho A, Ferrus ML, Madrid N, et al. Altered metabolism of gut microbiota contributes to chronic immune activation in HIV-infected individuals. Mucosal Immunol. (2015) 8:760–72. doi: 10.1038/mi.2014.107
60. Alzahrani J, Hussain T, Simar D, Palchaudhuri R, Abdel-Mohsen M, Crowe SM, et al. Inflammatory and immunometabolic consequences of gut dysfunction in HIV: parallels with IBD and implications for reservoir persistence and non-AIDS comorbidities. EBioMedicine. (2019) 46:522–31. doi: 10.1016/j.ebiom.2019.07.027
61. Storm-Larsen C, Stiksrud B, Eriksen C, Nowak P, Holm K, Thalme A, et al. Microbial translocation revisited: targeting the endotoxic potential of gut microbes in HIV-infected individuals. Aids. (2019) 33:645–53. doi: 10.1097/QAD.0000000000002087
62. McGinty T, Mirmonsef P, Mallon PW, Landay AL. Does systemic inflammation and immune activation contribute to fracture risk in HIV? Current Opinion HIV AIDS. (2016) 11:253–60. doi: 10.1097/COH.0000000000000275
63. Gootenberg DB, Paer JM, Luevano JM, Kwon DS. HIV-associated changes in the enteric microbial community: potential role in loss of homeostasis and development of systemic inflammation. Curr Opin Infect Dis. (2017) 30:31–43. doi: 10.1097/QCO.0000000000000341
64. Serrano-Villar S, Rojo D, Martinez-Martinez M, Deusch S, Vazquez-Castellanos JF, Bargiela R, et al. Gut bacteria metabolism impacts immune recovery in HIV-infected individuals. EBioMedicine. (2016) 8:203–16. doi: 10.1016/j.ebiom.2016.04.033
65. Wang Z, Usyk M, Sollecito CC, Qiu Y, Williams-Nguyen J, Hua S, et al. Altered gut microbiota and host metabolite profiles in HIV-infected women. Clin Infect Diseases. (2019). doi: 10.1093/cid/ciz1117. [Epub ahead of print].
66. Lu W, Feng Y, Jing F, Han Y, Lyu N, Liu F, et al. Association between gut microbiota and CD4 recovery in HIV-1 infected patients. Front Microbiol. (2018) 9:1451. doi: 10.3389/fmicb.2018.01451
67. Nowak RG, Bentzen SM, Ravel J, Crowell TA, Dauda W, Ma B, et al. Rectal microbiota among HIV-uninfected, untreated HIV, and treated HIV-infected in Nigeria. Aids. (2017) 31:857–62. doi: 10.1097/QAD.0000000000001409
68. Nowak P, Troseid M, Avershina E, Barqasho B, Neogi U, Holm K, et al. Gut microbiota diversity predicts immune status in HIV-1 infection. Aids. (2015) 29:2409–18. doi: 10.1097/QAD.0000000000000869
69. Lozupone CA, Rhodes ME, Neff CP, Fontenot AP, Campbell TB, Palmer BE. HIV-induced alteration in gut microbiota: driving factors, consequences, and effects of antiretroviral therapy. Gut Microbes. (2014) 5:562–70. doi: 10.4161/gmic.32132
70. Lozupone CA, Li M, Campbell TB, Flores SC, Linderman D, Gebert MJ, et al. Alterations in the gut microbiota associated with HIV-1 infection. Cell Host Microbe. (2013) 14:329–39. doi: 10.1016/j.chom.2013.08.006
71. Zilberman-Schapira G, Zmora N, Itav S, Bashiardes S, Elinav H, Elinav E. The gut microbiome in human immunodeficiency virus infection. BMC Med. (2016) 14:83. doi: 10.1186/s12916-016-0625-3
72. Duvivier C, Kolta S, Assoumou L, Ghosn J, Rozenberg S, Murphy RL, et al. Greater decrease in bone mineral density with protease inhibitor regimens compared with nonnucleoside reverse transcriptase inhibitor regimens in HIV-1 infected naive patients. Aids. (2009) 23:817–24. doi: 10.1097/QAD.0b013e328328f789
73. Brown TT, McComsey GA, King MS, Qaqish RB, Bernstein BM, da Silva BA. Loss of bone mineral density after antiretroviral therapy initiation, independent of antiretroviral regimen. J Acquired Immune Deficiency Syndromes. (2009) 51:554–61. doi: 10.1097/QAI.0b013e3181adce44
74. Gallant JE, Staszewski S, Pozniak AL, DeJesus E, Suleiman JM, Miller MD, et al. Efficacy and safety of tenofovir DF vs stavudine in combination therapy in antiretroviral-naive patients: a 3-year randomized trial. JAMA. (2004) 292:191–201. doi: 10.1001/jama.292.2.191
75. Ofotokun I, Titanji K, Vikulina T, Roser-Page S, Yamaguchi M, Zayzafoon M, et al. Role of T-cell reconstitution in HIV-1 antiretroviral therapy-induced bone loss. Nat Commun. (2015) 6:8282. doi: 10.1038/ncomms9282
76. Ofotokun I, Titanji K, Vunnava A, Roser-Page S, Vikulina T, Villinger F, et al. Antiretroviral therapy induces a rapid increase in bone resorption that is positively associated with the magnitude of immune reconstitution in HIV infection. Aids. (2016) 30:405–14. doi: 10.1097/QAD.0000000000000918
77. Nolan D, Upton R, McKinnon E, John M, James I, Adler B, et al. Stable or increasing bone mineral density in HIV-infected patients treated with nelfinavir or indinavir. Aids. (2001) 15:1275–80. doi: 10.1097/00002030-200107060-00009
78. Mondy K, Yarasheski K, Powderly WG, Whyte M, Claxton S, DeMarco D, et al. Longitudinal evolution of bone mineral density and bone markers in human immunodeficiency virus-infected individuals. Clin Infect Diseases. (2003) 36:482–90. doi: 10.1086/367569
79. Dolan SE, Kanter JR, Grinspoon S. Longitudinal analysis of bone density in human immunodeficiency virus-infected women. J Clin Endocrinol Metabolism. (2006) 91:2938–45. doi: 10.1210/jc.2006-0127
80. Bolland MJ, Grey AB, Horne AM, Briggs SE, Thomas MG, Ellis-Pegler RB, et al. Bone mineral density remains stable in HAART-treated HIV-infected men over 2 years. Clin Endocrinol. (2007) 67:270–5. doi: 10.1111/j.1365-2265.2007.02875.x
81. Sharma A, Flom PL, Weedon J, Klein RS. Prospective study of bone mineral density changes in aging men with or at risk for HIV infection. Aids. (2010) 24:2337–45. doi: 10.1097/QAD.0b013e32833d7da7
82. Bolland MJ, Grey A, Horne AM, Briggs SE, Thomas MG, Ellis-Pegler RB, et al. Stable bone mineral density over 6 years in HIV-infected men treated with highly active antiretroviral therapy (HAART). Clin Endocrinol. (2012) 76:643–8. doi: 10.1111/j.1365-2265.2011.04274.x
83. Kalayjian RC, Albert JM, Cremers S, Gupta SK, McComsey GA, Klingman KL, et al. Women have enhanced bone loss associated with phosphaturia and CD4+ cell restoration during initial antiretroviral therapy. Aids. (2018) 32:2517–24. doi: 10.1097/QAD.0000000000001995
84. Erlandson KM, Lake JE, Sim M, Falutz J, Prado CM, Domingues da Silva AR, et al. Bone mineral density declines twice as quickly among HIV-infected women compared with men. J Acquired Immune Deficiency Syndromes. (2018) 77:288–94. doi: 10.1097/QAI.0000000000001591
85. Finnerty F, Walker-Bone K, Tariq S. Osteoporosis in postmenopausal women living with HIV. Maturitas. (2017) 95:50–4. doi: 10.1016/j.maturitas.2016.10.015
86. Cotter AG, Powderly WG. Endocrine complications of human immunodeficiency virus infection: hypogonadism, bone disease and tenofovir-related toxicity. Best Pract Res Clin Endocrinol Metab. (2011) 25:501–15. doi: 10.1016/j.beem.2010.11.003
87. Young B, Dao CN, Buchacz K, Baker R, Brooks JT, Investigators HIVOS. Increased rates of bone fracture among HIV-infected persons in the HIV Outpatient Study (HOPS) compared with the US general population, 2000-2006. Clin Infect Diseases. (2011) 52:1061–8. doi: 10.1093/cid/ciq242
88. Grant PM, Kitch D, McComsey GA, Dube MP, Haubrich R, Huang J, et al. Low baseline CD4+ count is associated with greater bone mineral density loss after antiretroviral therapy initiation. Clin Infect Diseases. (2013) 57:1483–8. doi: 10.1093/cid/cit538
89. Hoy J, Grund B, Roediger M, Ensrud KE, Brar I, Colebunders R, et al. Interruption or deferral of antiretroviral therapy reduces markers of bone turnover compared with continuous therapy: the SMART body composition substudy. J Bone Mineral Res. (2013) 28:1264–74. doi: 10.1002/jbmr.1861
90. Grund B, Peng G, Gibert CL, Hoy JF, Isaksson RL, Shlay JC, et al. Continuous antiretroviral therapy decreases bone mineral density. Aids. (2009) 23:1519–29. doi: 10.1097/QAD.0b013e32832c1792
91. Stellbrink HJ, Orkin C, Arribas JR, Compston J, Gerstoft J, Van Wijngaerden E, et al. Comparison of changes in bone density and turnover with abacavir-lamivudine versus tenofovir-emtricitabine in HIV-infected adults: 48-week results from the ASSERT study. Clin Infect Diseases. (2010) 51:963–72. doi: 10.1086/656417
92. McComsey GA, Kitch D, Daar ES, Tierney C, Jahed NC, Tebas P, et al. Bone mineral density and fractures in antiretroviral-naive persons randomized to receive abacavir-lamivudine or tenofovir disoproxil fumarate-emtricitabine along with efavirenz or atazanavir-ritonavir: Aids Clinical Trials Group A5224s, a substudy of ACTG A5202. J Infect Dis. (2011) 203:1791–801. doi: 10.1093/infdis/jir188
93. McComsey GA, Lupo S, Parks D, Poggio MC, De Wet J, Kahl LP, et al. Switch from tenofovir disoproxil fumarate combination to dolutegravir with rilpivirine improves parameters of bone health. Aids. (2018) 32:477–85. doi: 10.1097/QAD.0000000000001725
94. Aurpibul L, Puthanakit T. Review of tenofovir use in HIV-infected children. Pediatric Infect Disease J. (2015) 34:383–91. doi: 10.1097/INF.0000000000000571
95. Negredo E, Domingo P, Perez-Alvarez N, Gutierrez M, Mateo G, Puig J, et al. Improvement in bone mineral density after switching from tenofovir to abacavir in HIV-1-infected patients with low bone mineral density: two-centre randomized pilot study (OsteoTDF study). J Antimicrob Chemotherapy. (2014) 69:3368–71. doi: 10.1093/jac/dku300
96. Bloch M, Tong WW, Hoy J, Baker D, Lee FJ, Richardson R, et al. Switch from tenofovir to raltegravir increases low bone mineral density and decreases markers of bone turnover over 48 weeks. HIV Med. (2014) 15:373–80. doi: 10.1111/hiv.12123
97. Maggiolo F, Rizzardini G, Raffi F, Pulido F, Mateo-Garcia MG, Molina JM, et al. Bone mineral density in virologically suppressed people aged 60 years or older with HIV-1 switching from a regimen containing tenofovir disoproxil fumarate to an elvitegravir, cobicistat, emtricitabine, and tenofovir alafenamide single-tablet regimen: a multicentre, open-label, phase 3b, randomised trial. Lancet HIV. (2019) 6:e655–66. doi: 10.1016/S2352-3018(19)30195-X
98. Cotter AG, Vrouenraets SM, Brady JJ, Wit FW, Fux CA, Furrer H, et al. Impact of switching from zidovudine to tenofovir disoproxil fumarate on bone mineral density and markers of bone metabolism in virologically suppressed HIV-1 infected patients; a substudy of the PREPARE study. J Clin Endocrinol Metab. (2013) 98:1659–66. doi: 10.1210/jc.2012-3686
99. Carr A, Miller J, Eisman JA, Cooper DA. Osteopenia in HIV-infected men: association with asymptomatic lactic acidemia and lower weight pre-antiretroviral therapy. Aids. (2001) 15:703–9. doi: 10.1097/00002030-200104130-00005
100. Woodward CL, Hall AM, Williams IG, Madge S, Copas A, Nair D, et al. Tenofovir-associated renal and bone toxicity. HIV Med. (2009) 10:482–7. doi: 10.1111/j.1468-1293.2009.00716.x
101. Moyle GJ, Stellbrink HJ, Compston J, Orkin C, Arribas JR, Domingo P, et al. 96-Week results of abacavir/lamivudine versus tenofovir/emtricitabine, plus efavirenz, in antiretroviral-naive, HIV-1-infected adults: ASSERT study. Antiviral Therapy. (2013) 18:905–13. doi: 10.3851/IMP2667
102. Grigsby IF, Pham L, Mansky LM, Gopalakrishnan R, Carlson AE, Mansky KC. Tenofovir treatment of primary osteoblasts alters gene expression profiles: implications for bone mineral density loss. Biochem Biophys Res Commun. (2010) 394:48–53. doi: 10.1016/j.bbrc.2010.02.080
103. Shiau S, Arpadi SM, Yin MT. Bone update: is it still an issue without tenofovir disoproxil fumarate? Current HIV/AIDS Reports. (2020) 1:1–5. doi: 10.1007/s11904-019-00474-1
104. Moran CA, Weitzmann MN, Ofotokun I. The protease inhibitors and HIV-associated bone loss. Curr Opinion HIV AIDS. (2016) 11:333–42. doi: 10.1097/COH.0000000000000260
105. Hirakawa H, Gatanaga H, Ochi H, Fukuda T, Sunamura S, Oka S, et al. Antiretroviral therapy containing HIV protease inhibitors enhances fracture risk by impairing osteoblast differentiation and bone quality. J Infect Dis. (2017) 215:1893–7. doi: 10.1093/infdis/jix246
106. Calmy A, Fux CA, Norris R, Vallier N, Delhumeau C, Samaras K, et al. Low bone mineral density, renal dysfunction, and fracture risk in HIV infection: a cross-sectional study. J Infect Dis. (2009) 200:1746–54. doi: 10.1086/644785
107. Kinai E, Nishijima T, Mizushima D, Watanabe K, Aoki T, Honda H, et al. Long-term use of protease inhibitors is associated with bone mineral density loss. AIDS Res Hum Retroviruses. (2014) 30:553–9. doi: 10.1089/aid.2013.0252
108. Amiel C, Ostertag A, Slama L, Baudoin C, N'Guyen T, Lajeunie E, et al. BMD is reduced in HIV-infected men irrespective of treatment. J Bone Mineral Res. (2004) 19:402–9. doi: 10.1359/JBMR.0301246
109. Gibellini D, Borderi M, de Crignis E, Clo A, Miserocchi A, Viale P, et al. Analysis of the effects of specific protease inhibitors on OPG/RANKL regulation in an osteoblast-like cell line. New Microbiol. (2010) 33:109–15.
110. Gao Y, Grassi F, Ryan MR, Terauchi M, Page K, Yang X, et al. IFN-gamma stimulates osteoclast formation and bone loss in vivo via antigen-driven T cell activation. J Clin Invest. (2007) 117:122–32. doi: 10.1172/JCI30074
111. Modarresi R, Xiang Z, Yin M, Laurence J. WNT/beta-catenin signaling is involved in regulation of osteoclast differentiation by human immunodeficiency virus protease inhibitor ritonavir: relationship to human immunodeficiency virus-linked bone mineral loss. Am J Pathol. (2009) 174:123–35. doi: 10.2353/ajpath.2009.080484
112. Santiago F, Oguma J, Brown AM, Laurence J. Noncanonical Wnt signaling promotes osteoclast differentiation and is facilitated by the human immunodeficiency virus protease inhibitor ritonavir. Biochem Biophys Res Commun. (2012) 417:223–30. doi: 10.1016/j.bbrc.2011.11.089
113. Yin MT, Modarresi R, Shane E, Santiago F, Ferris DC, McMahon DJ, et al. Effects of HIV infection and antiretroviral therapy with ritonavir on induction of osteoclast-like cells in postmenopausal women. Osteoporos Int. (2011) 22:1459–68. doi: 10.1007/s00198-010-1363-6
114. Wang MW, Wei S, Faccio R, Takeshita S, Tebas P, Powderly WG, et al. The HIV protease inhibitor ritonavir blocks osteoclastogenesis and function by impairing RANKL-induced signaling. J Clin Invest. (2004) 114:206–13. doi: 10.1172/JCI15797
115. Hernandez-Vallejo SJ, Beaupere C, Larghero J, Capeau J, Lagathu C. HIV protease inhibitors induce senescence and alter osteoblastic potential of human bone marrow mesenchymal stem cells: beneficial effect of pravastatin. Aging Cell. (2013) 12:955–65. doi: 10.1111/acel.12119
116. Yano S, Mentaverri R, Kanuparthi D, Bandyopadhyay S, Rivera A, Brown EM, et al. Functional expression of beta-chemokine receptors in osteoblasts: role of regulated upon activation, normal T cell expressed and secreted (RANTES) in osteoblasts and regulation of its secretion by osteoblasts and osteoclasts. Endocrinology. (2005) 146:2324–35. doi: 10.1210/en.2005-0065
117. Han JH, Choi SJ, Kurihara N, Koide M, Oba Y, Roodman GD. Macrophage inflammatory protein-1alpha is an osteoclastogenic factor in myeloma that is independent of receptor activator of nuclear factor kappaB ligand. Blood. (2001) 97:3349–53. doi: 10.1182/blood.V97.11.3349
118. Oba Y, Lee JW, Ehrlich LA, Chung HY, Jelinek DF, Callander NS, et al. MIP-1alpha utilizes both CCR1 and CCR5 to induce osteoclast formation and increase adhesion of myeloma cells to marrow stromal cells. Exp Hematol. (2005) 33:272–8. doi: 10.1016/j.exphem.2004.11.015
119. Lee JW, Hoshino A, Inoue K, Saitou T, Uehara S, Kobayashi Y, et al. The HIV co-receptor CCR5 regulates osteoclast function. Nat Commun. (2017) 8:2226. doi: 10.1038/s41467-017-02368-5
Keywords: HIV, HAART, bone, osteoblast, osteoclast
Citation: Delpino MV and Quarleri J (2020) Influence of HIV Infection and Antiretroviral Therapy on Bone Homeostasis. Front. Endocrinol. 11:502. doi: 10.3389/fendo.2020.00502
Received: 07 April 2020; Accepted: 23 June 2020;
Published: 02 September 2020.
Edited by:
Lilian Irene Plotkin, Indiana University Bloomington, United StatesReviewed by:
Marlena Cathorina Kruger, Massey University, New ZealandMohamed El Sayed Abdel Wanis, Sohag University, Egypt
Copyright © 2020 Delpino and Quarleri. This is an open-access article distributed under the terms of the Creative Commons Attribution License (CC BY). The use, distribution or reproduction in other forums is permitted, provided the original author(s) and the copyright owner(s) are credited and that the original publication in this journal is cited, in accordance with accepted academic practice. No use, distribution or reproduction is permitted which does not comply with these terms.
*Correspondence: María Victoria Delpino, bWRlbHBpbm9AZmZ5Yi51YmEuYXI=; Jorge Quarleri, cXVhcmxlcmlAZm1lZC51YmEuYXI=