- 1Endocrine Unit, Department of Medicine and Surgery, University of Insubria, ASST dei Sette Laghi, Varese, Italy
- 2Immunology and General Pathology Laboratory, Department of Biotechnology and Life Sciences, University of Insubria, Varese, Italy
- 3IRCCS MultiMedica, Milan, Italy
Graves' disease (GD) is a common autoimmune cause of hyperthyroidism, which is eventually related to the generation of IgG antibodies stimulating the thyrotropin receptor. Clinical manifestations of the disease reflect hyperstimulation of the gland, causing thyrocyte hyperplasia (goiter) and excessive thyroid hormone synthesis (hyperthyroidism). The above clinical manifestations are preceded by still partially unraveled pathogenic actions governed by the induction of aberrant phenotype/functions of immune cells. In this review article we investigated the potential contribution of natural killer (NK) cells, based on literature analysis, to discuss the bidirectional interplay with thyroid hormones (TH) in GD progression. We analyzed cellular and molecular NK-cell associated mechanisms potentially impacting on GD, in a view of identification of the main NK-cell subset with highest immunoregulatory role.
The autoimmune thyroid disorder, known as Graves' disease (GD), is the most frequent cause of hyperthyroidism in iodine sufficient areas (1). Production of autoantibodies against the TSH-receptor (TRAb) represents the ultimate step for disease progression (2). Therefore, identification of the major drivers involved in triggering and progression of the disease, still represents an unmet need (1). There is a large consensus that identification of all potential factors involved in the pathogenesis of GD might favor the development of a more efficient treatment strategy, as well as of prevention approaches (3). This would be of paramount importance in view of the current lack of an effective pharmacological therapy for GD (4–6).
Natural killer (NK) cells, whose has been initially defined in virus clearance and defense against tumors, represent a highly heterogenous cell population. More recently, they have been shown to be involved in autoimmune disorders with both pathogenic and regulatory roles (7). While it is widely accepted that abnormalities in the adaptive immune response underpin autoreactivity and autoimmune diseases, it is also clear that other effector cells within the innate immunity compartment can act as relevant players. The major aim of this narrative review was to discuss the potential involvement of NK cells in the pathogenesis of Graves' disease and to speculate on potential future treatment/prevention strategies, based on NK cells as a target and/or as a tool for therapy.
Current Understanding of the Pathogenesis of Graves' Disease
Although GD can occur at any age and in both genders, it is more frequently observed in women in the 4–5th decade of life (1). The ultimate event is the continuous activation of the TSH-R on thyroid follicular cells by TRAb (8, 9). This dysregulated and continuous thyroid stimulation causes hyperthyroidism and, frequently, thyroid enlargement (goiter) (10, 11). As for other autoimmune disorders, GD likely results from the breakdown in the immune tolerance mechanisms, both at systemic (peripheral blood) and local (tissue) levels (8, 9). Failure of T regulatory (T reg) cell activity, proliferation of autoreactive T and B cells, and enhanced presentation of TSH-R (due to increased HLA-D affinity for TSH-R, more immunogenic TSH-R haplotype, or increased exposure of TSH-R peptide) drive the development of the disease (12, 13). Interestingly, TRAb has been detected in serum only shortly before diagnosis of GD (8).
Studies of dizygotic and monozygotic twins showed that genetic predisposition plays a relevant role in the development of GD (14, 15). Genetic risk factors for GD include multiple susceptibility genes, such as some HLA haplotypes (e.g., HLA DRB1*3, DQA1*5, DQB1*2), polymorphisms of genes involved in T and B cells regulation [Cytotoxic T-Lymphocyte Antigen 4 (CTLA4), CD40, Protein tyrosine phosphatase non-receptor type 22 (PTPN22), the B cell survival factor (BAFF), Fas-ligand or CD95 and CD3γ], T reg cell functions (FOXp3), and polymorphisms of genes encoding for thyroid peptides (variants of thyroglobulin or TSH-R) (12, 16–21). Recently, a single polymorphism in tumor necrosis factor α (TNFα) gene (rs1800629) was correlated with an increased risk to develop GD (22). GD is a heterogeneous disease, resulting from the combination of various and different gene polymorphisms, actually detectable by pooled genome wide association study (21–25). This would explain the weak overall size effect for genetic markers in genome-wide association studies (16, 21). Precipitating factors, probably inducing epigenetic changes include sex hormones, pregnancy, cigarette smoking, stress, infection, iodine, and other potential environmental factors (17, 26–33).
GD has been historically considered a T helper (Th)2-skewed disorder (34). This was supported by the starring role of B cells and by the features of Th cells infiltrating the thyroid gland, which are T cell clones specific for the TSH-R and mainly harbor Th2 cytokines (34, 35). More recently, Nagayama et al. demonstrated that the induction of immune shifting toward a Th2 phenotype in a GD mouse model was associated with a decrease, rather than an increase, in TRAb synthesis (36). This indirectly suggested a Th1 priority role in the induction of GD (35). In keeping with these findings, several studies showed that thyrostatic treatment with antithyroid drugs progressively induced transition from Th1 to Th2 predominance (37). As elegantly demonstrated by Rapaport and McLachlan, the fact that TRAb antibodies belong to the subclass of IgG, might explain the Th1-Th2 cytokine bias (38). Indeed, different IgG subclasses might coexist in several diseases and could additionally contribute to the pathogenic mechanisms (35–43). While early stage of the humoral immune response involves Th1 cytokines (e.g., IFN [interferon] γ), the prolonged immunization depends on IgG4 antibodies, driven by Th2 cytokines (e.g., interleukin [IL]-4) (39, 40). During a first phase, antigen presenting cells (APCs) and B cells-derived cytokines (IFNγ and TNFα) stimulate thyrocytes to secrete several chemokines, including C-X-C chemokine 10 that can recruit Th cells. Th cells interact with B cells to produce antibodies (1). Finally, intrathyroidal Th2 cells inhibit Th1 responses through the secretion of IL-10, IL-5, and IL-4 (38–46), thus preventing destruction of the thyroid gland, at variance with Hashimoto's thyroiditis. At this stage, thyroid gland might be protected from destruction both by inhibition of macrophages (from Th2 cytokines) and by upregulation of anti-apoptotic mechanisms (BCL-XL)/downregulation of Fas-Fas-ligand interaction (44, 45). Concomitantly, the increased Th2 response leads to an increased production of antibodies.
Natural Killer Cells and Their Role in Autoimmunity
NK cells are large granular lymphocytes (LGL), recently classified as a subset of innate lymphoid cells (47). They are classically distinguished from the other mononuclear cells due to the expression of CD56, a molecule mediating homotypic adhesion, and null expression of CD3 (48). Additionally, based on the density of CD16 espression (a low-affinity receptor for the Fc portion of immunoglobulin G) and CD56 surface markers, NK cells could be further distinguished in two major subsets: CD56brightCD16dim/− and CD56dimCD16+cells (49– 51). According to a well-supported theory, NK cell precursors leave the bone marrow, transit through peripheral blood and reach the lymph nodes, where, under the influence of cytokines produced by stromal matrix, they differentiate into CD56+CD16− (49– 53). Maturation process is characterized by the down-regulation of CD56 and the acquisition of CD16 markers, as well as of “killer cell immunoglobulin-like receptors” (KIRs), getting the features of CD56dimCD16+cells (50, 52–54). Therefore, CD56dimCD16+ NKs show high potential of cytotoxicity, due to the high content of cytolytic granules (containing perforin and granzyme), the high expression of KIRs, ILT2 (immunologlobulin-like transcript 2), and CD16 itself (51, 53). Conversely, CD56brightCD16dim/− are more immature cells, characterized by poor cytotoxic ability, high expression of inhibitory receptors (such as NKG2A), high ability to proliferate in response to IL-2 and elevated production of several cytokines, such as IFNγ, TNFα, granulocyte–macrophage colony-stimulating factor, IL-10 and IL-13, depending on the conditions of stimulation (51, 55–58). It is the balance between inhibitory and activating signals, deriving from non-rearranged surface receptors, to dictate whether or not NK cells will kill target cells, engaged during their “patrolling” action (Figure 1A). Inhibitory receptors such as NKG2A, CD161, and inhibitory KIRs prevented the killing of normal cells, through the recognition of “self” molecules belonging to MHC class I. Thus, according to the “missing self-hypothesis,” NK cells recognize and attack target cells presenting low or aberrant MHC class I molecules (59). Furthermore, activating receptors, such as the natural cytotoxic receptors (NKp44, NKp46, NKp30), CD69, activating C-type lectin-like receptors (as the natural killer group 2D receptor) and activating KIRs recognize ligands induced on stressed cells (infected/overactive/transformed cells) and stimulate NK cells activation.
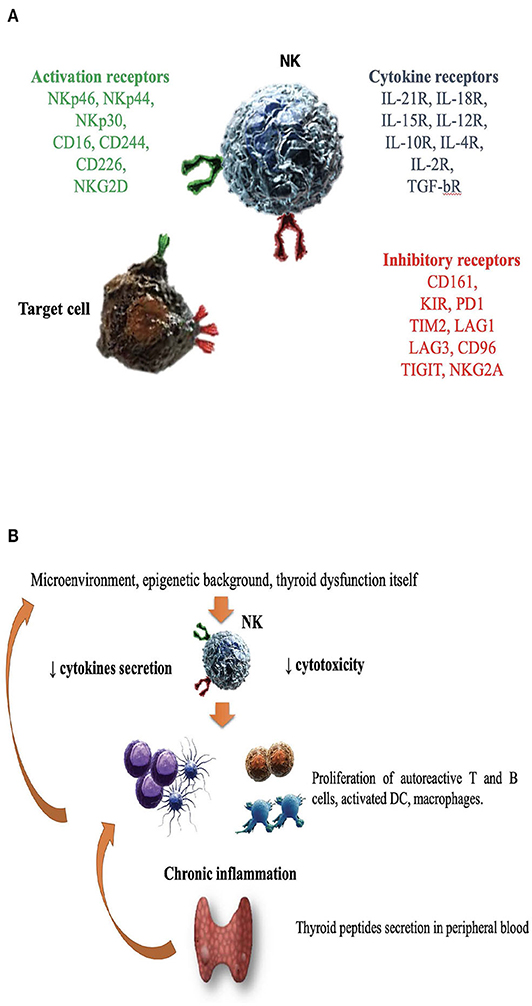
Figure 1. The role of natural killer cells in the pathogenesis of Graves' disease. (A) Enumeration of activating/inhibitory receptors and cytokines receptors, whose signals determined NK cells activity in health and disease. CD, cluster of differentiation; CD16, Fc receptor; CD244, non MHC biding receptor acting as costimulatory ligand for NK cells; CD69, early expressed after NK cell activation; CD96, interacts with nectin and nectin-like proteins; CD161, recognizes the human NKR-P1A antigen; KIR, killer cell immunoglobulin like receptor; LAG1 and LAG3, lymphocyte activation gene 1 and 3; NKp30, NKp44, NKp46, the natural cytotoxic receptors (NCR); NKG2A and NKG2D, natural killer group 2A and 2D; TIGIT, T cell immunoglobulin and ITIM domain; IL (interleukin)-21/18/15/10/12/4/2 R (receptor); TGF-bR, TGF beta receptor family; PD1, programmed cell death protein 1; TIM2, T-cell immunoglobulin and mucin-containing domain 2; (B) Several factors including microenvironment, cytokines milieu, epigenetic background and hyperthyroidism itself might impair NK protective activity. DC, dendritic cells; NK, natural killer cells.
With the advent of the single cell technologies, coupled with RNA sequencing, it has been observed that NK cell heterogeneity, in term of subsets, is more complex (according to the different surface antigens and cytokine milieu) (60). This is not only a gene-restricted but also an environmental (re)-directed process (61–64). Modeling T cell classification, in humans, NK cells could be divided at least in two sets: “NK1,” characterized by the production of IFNγ and the regulatory “NK2” cells (65, 66). The polarization to NK2 phenotype depends on high IL4 levels and is characterized by the high production of “type 2” cytokines (i.g., IL-5, IL-10, and IL-13), the high expression of cytokines receptors and of NKG2A surface marker.
Considering their role in defense against viruses and that viral triggers are often involved in the initiation of several immune disorders, NK cells have been investigated for their role in autoimmunity (65–68). Indeed, CD56bright NK cells may orchestrate the overall immune process, influencing both innate and adaptive immune cells, through the integration of signals from numerous activating and inhibitory receptors. Due to the high plasticity and interaction with other immune and stromal cells, CD56bright NK cells acquire a regulatory role (65–69). In this context, a third subset, called “NK reg” has therefore been suggested and defined, according to surface inducible or constitutive markers such as CD117 (65–73). However, the available studies provided conflicting results, since, under some circumstances, NKs play a protective role, while in others they have been blamed to be pathogenic (7, 62, 68, 69). Likely, their action is correlated to the type of cell becoming the target of attack. In case of whether acquired or inherited dysfunctions, NK cells might participate into the destruction of non-transformed, healthy cells as the first step of the autoimmune process. Conversely, if targets are autoreactive T cells, dendritic cells (DC) or pro-inflammatory macrophages, NKs might act as regulators, dampening the inflammatory process (65, 69–73). Interestingly, NK cell regulatory activity has been demonstrated in several autoimmune diseases, such as multiple sclerosis (MS), experimental colitis or encephalitis (EE) and arthritis (RA), by different strategies such as cytokine release, interaction with ligands of the receptors NKG2D, NKG2A, NKp46 or perforin-mediated T cell death (63, 72, 73). In a mouse model, Ehelers and co-workers demonstrated that high levels of IL-18, which are found in Th1-skewed autoimmune process, induced the expression of CD117 on NKs which, in turn, became able to suppress CD8+T cells (73). In other experiments, CD56bright NK suppressed autologous CD4+ T cells proliferation through the expression of NKp30 and NKp46, granzyme B releasing and immunosuppressive molecule adenosine (72, 73). In experimental models of autoimmune EE, the inhibitory role of NKs on the T effectors proliferation, as well as a direct cytotoxic effect on autoreactive specific T cells, were shown (74). Likewise, Takahashi et al. demonstrated, in MS patients, that CD56bright NK could favor clinical remission, by suppressing the production of IFNγ, by specific autoreactive T effectors and secreting IL-5 (57, 69, 75). Laroni et al. observed that CD56bright NK cells had reduced ability to kill T-cells in MS patients, compared to healthy controls, possibly due to an increased expression of NKG2A (69, 76). Thus, impaired cytotoxicity or the inability to secrete cytolytic granules have been correlated to the escape of proinflammatory cells (both T and B lymphocytes, DC and macrophages) from regulatory mechanisms of controls (77). In other cases, such as RA, loss of NK tolerance (due to decreased inhibitory signals or inappropriate stimulation of activating signals) might favor the development of autoimmune diseases. Different mechanisms have been blamed, such as the presence of antilymphocyte antibodies (78). In other disorders, such as myasthenia gravis and EE, NK cells seem to facilitate initiation and progression of autoimmunity (67, 68). Besides differences in the strains and models used, several factors may influence the specific, and even contradictory, actions of NK cells. Their ability to adapt to different stimuli and different anatomical localization may play an important role. Microenvironment itself may influence NK functions, such as migration and tissue retentions, as it emerged in the complex interaction with DC, influenced by density, maturation state and phenotype of this population (68). Epigenetic modifications strongly influence NK cells all along their life, from development to regulation and differentiation of effector functions (79–81). Epigenetic remodeling, acquired through immunological experiences, might modulate NK functions (61). For instance, gene expression of several genes (including KIRs) is regulated by DNA methylation (hypomethylation or hypermethylation) of their promoters. The interindividual genetic variability in the receptor repertoire, especially of the highly polymorphic KIR gene, influence the recognition of target cells (80). KIRs polymorphisms might influence the engagement with HLA molecules and, as counterpart, functional interaction between co-inherited KIRs (especially inhibitory KIRs) and HLA progressively influence NK education (81). Besides KIRs, other receptors such as NKG2A are involved in NK education (61).
The Link Between Leukocytes and Thyroid Hormones
A possible link between THs and the immune system was already suggested more than 40 years ago, by the discovery that Staphylococcus-stimulated lymphocytes might de novo synthesize a TSH-like substance (immunoreactive TSH, i-TSH), similar to the pituitary-released form and possibly involved in autoimmune thyroid disorders (AITD) (82). Further experiments progressively demonstrated that bone marrow hematopoietic cells, lymphocytes, DC and even intestinal epithelial cells, could synthesize TSH (83). The role of extra-pituitary TSH remains to be clarified. It was speculated that, as pituitary TSH, i-TSH might stimulate the synthesis of TH, which, in turn, might influence the immune system (indirect effect). Several papers showed that immune cells harbor essential elements required for THs metabolism and action. For example, both neutrophils and DC express T3 (the active form of TH) transporters (MCT10 in human) and type 2 and 3 deiodinases (involved in THs synthesis) (84–86). Indeed, it has been widely demonstrated that THs interact with hematopoietic cells (85–90) at different levels. T3 might affect target immune cells by binding both to nuclear receptors (thyroid hormones receptors TRα and TRβ) and membrane receptors (86–90). For example, TH and especially T3 can influence maturation of DCs (84, 85). DC phenotype was studied in thyroidectomized patients before and after levothyroxine supplementation, showing that THs induce an increase in DCs number and influence their functions (91). A research group from Cordoba demonstrated that T3 induce DCs activation through Akt and NF-kB pathways, driving the immune response toward a Th1 phenotype (92, 93). Further support to the regulatory role of TH came from experiments showed that daily administration of T4 was followed by the complete restoration of the immune competence in thyroidectomized mice (94). Furthermore, T4 treatment in mice enhanced the NKs cytotoxic activity against classical target cells, amplifying their responsiveness to cytokines and modulating NK metabolic properties (95). Some years later, Provinciali et al. demonstrated that, after T4 pre-treatment, the peak of NK cytotoxic activity was achieved using half the optimal IFNγ concentration (96). Additional experiments strengthen the hypothesis of a paracrine TSH-pathway (97–99). TSH-R is expressed on myeloid and lymphoid cells (100, 101). By its stimulation, TSH (both the immune and the pituitary released forms) may act as a cytokine-like regulatory molecule and induce the secretion of several cytokines, such as TNFα (102, 103). In vitro studies showed that TSH, combined to classical cytokines (as IL-2, IL-12, IL-1β), acts as co-stimulus improving lymphocytes and NKs proliferative response to even low dose of mitogens (103, 104). Todd et al. demonstrated that TSH was able to enhance the expression of MHC class II in thyroid cells treated with IFNγ (105). Accordingly, Dorshkind et al. demonstrated that THs induce the synthesis of cytokines and the expression of IL-2 receptor in NK cells (106). Indeed, while both T3 and FT4 boosted the IFNγ response in mice (107, 108), T4 amplified both IFNγ and IL-2 (96).
Based on the bidirectional relationship between TH and the immune system (96), Kmiec et al. postulated that in the elderly the reduction of TH with aging might be involved in the impairment of NK activity by T3 administration; they found a direct correlation between serum T3 levels and NK activity, in spite of conserved proportion of circulating NK cells (109, 110). Indeed, NK cell activity was selectively improved by T3 administration in those subjects having T3 levels in the slower range.
Natural Killer Cells and Graves' Disease
From a mutual perspective, thyroid function might orchestrate the immune response and, conversely, dysfunction of the immune system might favor the development of thyroid disorders. Several studies investigated the potential contribution of NKs in the development and/or progression of GD, but results are still inconclusive and sometimes conflicting. Table 1 reports the available data on this issue (111–123). Researchers from Osaka University observed that the total percentage of LGL, including NK-like cells, was decreased in untreated GD patients compared to euthyroid GD patients on antithyroid drug therapy and to controls; in addition, the proportion of LGL was inversely correlated to T4 and T3 levels (110–112, 123). Thus, while normal THs levels are crucial to maintain an adequate activity of the immune system, supraphysiological THs levels exerted a detrimental effect, mimicking starvation, and increased cortisol secretion (121, 124–126). Immunocomplexes able to suppress NK cell activity, as in other autoimmune disorders (e.g., RA), were considered as a possible cause of this phenomenon (76, 127). According to a different hypothesis, the decrease of NK cells might be the primary immunological abnormality in the pathogenesis of GD (111, 127).
Solerte et al. reported that both spontaneous and IL-2/IFNβ-modulated NK cells cytotoxicity (NKCC), as well as spontaneous and IL-2 induced TNFα release were decreased in NK cells from 13 GD patients compared to 15 controls (121). Both cytokines secretion and cytotoxicity were promptly normalized by co-incubating NKs with DHEAS (dehydroepiandrosterone sulfate), supporting the concept of a concomitant effect of other endocrine axes (121, 128, 129). Studies from the University of Miami comparing thyrotoxic mice (due to levothyroxine treatment) to euthyroid or hypothyroid (due to antithyroid drug treatment) control mice observed a reduced secretion of cytolytic granules (113). Similar results were obtained from the same group in humans (see Table 1) (114), with a reduction in cytotoxicity, studied by release assay for NK cell cytotoxicity against K562 tumor target cells.
Considering that NK cell activity is affected by age (130), a study compared NKCC in AITD patients with age and gender-matched healthy controls, demonstrating an impaired NK cell activity in AITD (120). As previously outlined, the integration of activating and inhibitory signals from NK surface regulates NK cells effector functions, such as cytokine secretion and NKCC. In a study of 28 newly onset GD patients, Zhang et al. observed a reduction of NK cells expressing both activating (NKG2D, NKG2C, NKp30) and inhibitory receptors (NKG2A) compared to matched healthy controls (122). Additionally, NKG2A+ NKs were inversely related to TRAb levels, while NKG2D+ NKs were inversely related to serum free T4 levels (122), supporting the role of dysfunctional NK cells. Figure 1B illustrates the hypothesis that in case of dysfunctional impairment, NK cells lose their ability to protect from the development of GD. Other studies (115, 117, 119, 120, 122, 131, 132), with some exceptions (116, 118) generally agreed on the impairment of NK activity in GD and reported that restoration of euthyroidism by antithyroid drug treatment (especially propylthiouracil) could improve NK functionality (133, 134).
Conclusion and Future Perspectives
It is now clear the immune system, both the innate and the adaptive components, are crucial host-related orchestrators of disorder induction/insurgence and progression. Alterations of immune cell phenotype and functions, as a consequence of chronic inflammation, are shared features between cancers, cardiovascular, neurological, and autoimmune diseases. In the new era of immunotherapy, most of the efforts are addressed to cancer, as supported by the vast literature and clinical trials (135). This rapidly developing field suggests the same attention should be dedicated also to autoimmunity, that still requires a better understanding of the cellular and molecular events occurring during autoimmune disorders, including GD. Unveiling these mechanisms and events is required to identify new immunological cellular biomarkers, trace disease progression, and design new targeted therapeutic strategies for autoimmunity. In this scenario, re-education/manipulation of NK cells appear as a promising strategy, as confirmed by the growing interest in CAR-NK cells (136).
Author Contributions
DG, LM, EP, and AB conceived the manuscript. All the authors took part in manuscript writing and editing. EP, LB, LM, and AB supervised the final version of the manuscript.
Funding
This work was partly supported by University of Insubria intramural grant FAR 2019 and MIUR Funding for Basic Research Activities FFABR 2017 to LM and by grants from the Ministry of Education, University and Research (MIUR, Roma) to LB and from the University of Insubria to LB and EP. AB has received funding from AIRC under MFAG 2019- ID 22818-PI. AB is supported by Italian Ministry of Health Ricerca Corrente-IRCCS MultiMedica. DG was supported by a University of Insubria Ph.D. scholarship in Experimental and Translational Medicine, whereas MG was a recipient of Ph.D. course in Life Sciences and Biotechnology at the University of Insubria.
Conflict of Interest
The authors declare that the research was conducted in the absence of any commercial or financial relationships that could be construed as a potential conflict of interest.
References
1. Smith TJ, Hegedüs L. Graves' disease. N Engl J Med. (2016) 375:1552–65. doi: 10.1056/NEJMra1510030
2. Davies TF, Latif R. Editorial: TSH receptor and autoimmunity. Front Endocrinol. (2019) 10:19. doi: 10.3389/fendo.2019.00019
3. Tanda ML, Piantanida E, Lai A, Lombardi V, Dalle Mule I, Liparulo L, et al. Thyroid autoimmunity and environment. Horm Metab Res. (2009) 41:436–42. doi: 10.1055/s-0029-1215568
4. Masiello E, Veronesi G, Gallo D, Premoli P, Bianconi E, Rosetti S, et al. Antithyroid drug treatment for Graves' disease: baseline predictive models of relapse after treatment for a patient-tailored management. J Endocrinol Invest. (2018) 41:1425–32. doi: 10.1007/s40618-018-0918-9
5. Piantanida E, Lai A, Sassi L, Gallo D, Spreafico E, Tanda ML, et al. Outcome prediction of treatment of Graves' hyperthyroidism with antithyroid drugs. Horm Metab Res. (2015) 47:767–72. doi: 10.1055/s-0035-1555759
6. Bartalena L, Piantanida E, Tanda ML. Can a patient-tailored treatment approach for Graves' disease reduce mortality? Lancet Diabetes Endocrinol. (2019) 7:245–6. doi: 10.1016/S2213-8587(19)30057-9
7. Zitti B, Bryceson YT. Natural killer cells in inflammation and autoimmunity. Cytokine Growth Factor Rev. (2018) 42:37–46. doi: 10.1016/j.cytogfr.2018.08.001
8. Morshed SA, Latif R, Davies TF. Delineating the autoimmune mechanisms in Graves' disease. Immunol Res. (2012) 54:191–203. doi: 10.1007/s12026-012-8312-8
9. Antonelli A, Fallahi P, Elia G, Ragusa F, Paparo SR, Ruffilli I, et al. Graves' disease: clinical manifestations, immune pathogenesis (cytokines and chemokines) and therapy. Best Pract Res Clin Endocrinol Metab. (2020). 34:101388. doi: 10.1016/j.beem.2020.101388
10. Bartalena L, Masiello E, Magri F, Veronesi G, Bianconi E, Zerbini F, et al. The phenotype of newly diagnosed Graves' disease in Italy in recent years is milder than in the past: results of a large observational longitudinal study. J Endocrinol Invest. (2016) 39:1445–51. doi: 10.1007/s40618-016-0516-7
11. Valente WA, Vitti P, Rotella CM, Vaughan MM, Aloj SM, Grollman EF, et al. Antibodies that promote thyroid growth. A distinct population of thyroid stimulating autoantibodies. N Engl J Med. (1983) 309:1028–34. doi: 10.1056/NEJM198310273091705
12. McLachlan S, Rapoport B. Breaking tolerance to thyroid antigens: changing concepts in thyroid autoimmunity. Endocr Rev. (2014) 35:59–151. doi: 10.1210/er.2013-1055
13. Chen Z, Wang Y, Ding X, Zhang M, He M, Zhao Y, et al. The proportion of peripheral blood tregs among the CD4+ T cells of autoimmune thyroid disease patients: a meta-analysis. Endocr J. (2020) 67:317–26. doi: 10.1507/endocrj.EJ19-0307
14. Brix TH, Kyvik KO, Christensen K, Hegedüs L. Evidence for a major role of heredity in Graves' disease: a population-based study of two danish twin cohorts. J Clin Endocrinol Metab. (2001) 86:930–34. doi: 10.1210/jcem.86.2.7242
15. Brix TH, Hegedüs L. Twin studies as a model for exploring the aetiology of autoimmune thyroid disease. Clin Endocrinol. (2012) 76:457–64. doi: 10.1111/j.1365-2265.2011.04318.x
16. Vejrazkova D, Vcelak J, Vaclavikova E, VanKova M, Zajickova K, Duskova M, et al. Genetic predictors of the development and recurrence of Graves' disease. Physiol Res. (2018) 67:S431–9. doi: 10.33549/èhysiolres.934018
17. Antonelli A, Ferrari SM, Ragusa F, Ragusa F, Paparo SR, Ruffilli I, et al. Graves' disease: epidemiology, genetic and environmental risk factors and viruses. Best Pract Res Clin Endocrinol Metab. (2020) 4:101387. doi: 10.1016/j.beem.2020.101387
18. Pujol-Borrell R, Álvarez-Sierra D, Jaraquemada D, Marín-Sánchez A, Colobran R. Central tolerance mechanisms to TSHR in Graves' disease: contributions to understand the genetic association. Horm Metab Res. (2018) 50:863–70. doi: 10.1055/a-0755-7927
19. Latif R, Mezei M, Morshed SA, Ma R, Ehrlich R, Davies TF. A modifying autoantigen in Graves' disease. Endocrinology. (2019) 160:1008–20. doi: 10.1210/en.2018-01048
20. Watanabe A, Inoue N, Watanabe M, Yamanoto M, Ozaki H, Hidaka Y, et al. Increases of CD80 and CD86 Expression on peripheral blood cells and their gene polymorphisms in autoimmune thyroid disease. Immunol Invest. (2020) 49:191–203. doi: 10.1080/08820139.2019.1688343
21. Lane LC, Allinson KR, Campbell K, Bhatnagar I, Ingoe L, Razvi S, et al. Analysis of BAFF gene polymorphisms in UK Graves' disease patients. Clin Endocrinol. (2019) 90:170–4. doi: 10.1111/cen.13872
22. Tu Y, Fan G, Zeng T, Cai X, Kong W. Association of TNF-α promoter polymorphism and Graves' disease: an updated systematic review and meta-analysis. Biosci Rep. (2018) 38:BSR20180143. doi: 10.1042/BSR20180143
23. Truja T, Kutz A, Fischli S, Meier C, Mueller B, Recher M, et al. Is Graves' disease a primary immunodeficiency? New immunological perspectives on an endocrine disease. BMC Med. (2017) 15:174. doi: 10.1186/s12916-017-0939-9
24. Shao X, Wang B, Mu K, Li, Li L Q, Jia X, et al. Key gene co-expression modules and functional pathways involved in the pathogenesis of Graves' disease. Mol Cell Endocrinol. (2018) 474:252–9. doi: 10.1016/j.mce.2018.03.015
25. Khong JJ, Burdon KP, Lu Y, Laurie K, Leonardos L, Baird PN, et al. Pooled genome wide association detects association upstream of FCRL3 with Graves' disease. BMC Genomics. (2016) 17:939. doi: 10.1186/s12864-016-3276-z
26. Croce L, Di Dalmazi G, Orsolini F, Virili C, Brigante G, Gianetti E, et al. Graves' disease and the post-partum period: an intriguing relationship. Front Endocrinol. (2019) 10:853. doi: 10.3389/fendo.2019.00853
27. Papanastasiou L, Vatalas IA, Koutras DA, Mastorakos G. Thyroid autoimmunity in the current iodine environment. Thyroid. (2007) 17:729–39. doi: 10.1089/thy.2006.0299
28. Vestegard P. Smoking and thyroid disorders–a meta-analysis. Eur J Endocrinol. (2002) 146:153–61. doi: 10.1530/eje.0.1460153
29. Kim SJ, Kim MJ, Yoon SG, Myong JP, Yo HW, Chai YJ, et al. Impact of smoking on thyroid gland: dose-related effect of urinary cotinine levels on thyroid function and thyroid autoimmunity. Sci Rep. (2019) 9:4213. doi: 10.1038/s41598-019-40708-1
30. Gallo D, Cattaneo SAM, Piantanida E, Mortara L, Merletti F, Nisi M, et al. Severità del morbo di basedow di prima diagnosi: ruolo dei linfociti T reg e dei micronutrienti. Dati preliminari di uno studio osservazionale pilota. Lettere GIC. (2019) 28:15–19.
31. Marinò M, Menconi F, Rotondo Dottore G, Leo M, Marcocci C. Selenium in Graves' hyperthyroidism and orbitopathy. Ophthalmic Plast Reconstr Surg. (2018) 34:S105–10. doi: 10.1097/IOP.0000000000001136
32. Gallo D, Mortara L, Gariboldi MB, Cattaneo SAM, Rosetti S, Gentile L, et al. Immunomodulatory effect of vitamin D and its potential role in the prevention and treatment of thyroid autoimmunity: a narrative review. J Endocrinol Invest. (2020) 43:413–29. doi: 10.1007/s40618-019-01123-5
33. Ponzetto A, Figura N. Clinical phenotype of Graves' disease. J Endocrinol Invest. (2020) doi: 10.1007/s40618-020-01214-8
34. McIver B, Morris JC. The pathogenesis of Graves' disease. Endocrinol Metab Clin North Am. (1998) 27:73–89. doi: 10.1016/s0889-8529(05)70299-1
35. Nicholson LB, Kuchroo VK. Manipulation of the Th1/Th2 balance in autoimmune disease. Curr Opin Immunol. (1996) 8:837–42. doi: 10.3109/08916939608995329
36. Nagayama Y, Mizuguchi H, Hayakawa T, Niwa M, McLachlan SM, Rapoport B. Prevention of autoantibody-mediated graves'-like hyperthyroidism in mice with IL-4, a Th2 cytokine. J Clin Invest. (2003) 170:3522–7. doi: 10.4049/jimmunol.170.7.3522
37. Yoshihisa I, Atsushi M, Naoto S, Yoshimasa A. Changes in expression of T-helper (Th) 1- and Th2-associated chemokine receptors on peripheral blood lymphocytes and plasma concentrations of their ligands, interferon-inducible protein-10 and thymus and activation-regulated chemokine, after antithyroid drug administration in hyperthyroid patients with Graves' disease. Eur J Endocrinol. (2007) 156:623–30. doi: 10.1530/EJE-07-0019
38. Rapoport B, McLachlan SM. Graves' hyperthyroidism is antibody-mediated but is predominantly a Th1-type cytokine disease. J Clin Endocrinol Metab. (2014) 99:4060–61. doi: 10.1210/jc.2014-3011
39. Giusti C. The Th1 chemokine MIG in Graves' disease: a narrative review of the literature. Clin Ter. (2019) 170:e285–90. doi: 10.7417/CT.2019.2149
40. Toellner KM, Luther SA, Sze DM, Choy RK, Taylor DR, MacLennan IC, et al. T helper 1 (Th1) and Th2 characteristics start to develop during T cell priming and are associated with an immediate ability to induce immunoglobulin class switching. J Exp Med. (1998) 187:1193–204. doi: 10.1084/jem.187.8.1193
41. Lu X, Peng S, Wang X, Shan Z, Teng W. Decreased expression of FcγRII in active Graves' disease patients. J Clin Lab Anal. (2019) 33:e22904. doi: 10.1002/jcla.22904
42. Mullins RJ, Cohen SB, Webb LM, Chernajovsky Y, Dayan CM, Londei M, et al. Identification of thyroid stimulating hormone receptor-specific T cells in Graves' disease thyroid using autoantigen-transfected epstein-barr virus-transformed B cell lines. J Clin Invest. (1995) 96:30–37. doi: 10.1172/JCI118034
43. Koneczny I. A new classification system for IgG4 autoantibodies. Front Immunol. (2018) 9:97. doi: 10.3389/fimmu.2018.00097
44. Fountoulakis S, Vartholomatos G, Kolaitis N, Frillingos S., Philippou G, Tsatsoulis A. Differential expression of Fas system apoptotic molecules in peripheral lymphocytes from patients with Graves' disease and hashimoto's thyroiditis. Eur J Endocrinol. (2008) 158:853–9. doi: 10.1530/EJE-08-0092
45. Stassi G, de Maria R. Autoimmune thyroid disease: new models of cell death in autoimmunity. Nat Rev Immunol. (2002) 2:195–204. doi: 10.1038/nri750
46. Fallahi P, Ferrari SM, Ragusa F, Ruffini I, Elia G, Paparo SR, et al. Th1 chemokines in autoimmune endocrine disorders. J Clin Endocrinol Metab. (2020) 105:dgz289. doi: 10.1210/clinem/dgz289
47. Sciumé G. Innate lymphocytes: development, homeostasis, and disease. Cytokine Growth Factor Rev. (2018) 42:1–4. doi: 10.1016/j.cytogfr.2018.08.002
48. Crinier A, Narni-Mancinelli E, Ugolini S, Vivier E. Snapshot: natural killer cells. Cell. (2020) 180:1280.e1. doi: 10.1016/j.cell2020.02.029
49. Cichocki F, Grzywacz B, Miller JS. Human NK cell development: one road or many? Front Immunol. (2019) 10:2078. doi: 10.3389/fimmu.2019.02078
50. Chan A, Hong DL, Atzberger A, Briquemont B, Iserentant G, Ollert M, et al. CD56bright human NK cells differentiate into CD56dim cells: role of contact with peripheral fibroblasts. J Immunol. (2007) 179:89–94. doi: 10.4049/jimmunol.179.1.89
51. Sivori S, Vacca P, Del Zotto G, Munari E, Migari MC, Moretta L. Human NK cells: surface receptors, inhibitory checkpoints, translational applications. Cell Mol Immunol. (2019) 16:430–41. doi: 10.1038/s41423-019-0206-4
52. Romagnani C, Juelke K, Falco M, Morandi B, D'Agostino A, Costa R, et al. CD56brightCD16- killer Ig-like receptor- NK cells display longer telomeres and acquire features of CD56dim NK cells upon activation. J Immunol. (2008) 178:4947–55. doi: 10.4049/jimmunol.178.8.4947
53. Bozzano F, Perrone C, Moretta L, De Maria A. NK cell precursors in human bone marrow in health and inflammation. Front Immunol. (2019) 10:2045. doi: 10.3389/fimmu.2019.02045
54. Moretta L. Dissecting CD56dim human NK cells. Blood. (2010) 116:3689–91. doi: 10.1182/blood-2010-09-303057
55. Poli A, Michel T, Thérésine M, Andrès E, Hentges F, Zimmer J. CD56bright natural killer (NK) cells: an important NK cell subset. Immunology. (2009) 126:458–65. doi: 10.1111/j.1365-2567.2008.03027.x
56. Michel T, Poli A, Cuapio A, Briquemont T, Iserentat G, Ollert M, et al. Human CD56bright NK cells: an update. J Immunol. (2016) 196:2923–31. doi: 10.4049/jimmunol.1502570
57. Morandi F, Horenstein AL, Chillemi A, Quarona V, Chiesa S, Imperatori A, et al. CD56bright CD16-NK cells produce adenosine through a CD38-mediated pathway and act as regulatory cells inhibiting autologous CD4+ T cell proliferation. J Immunol. (2015) 195:965–72. doi: 10.4049/jimmunol.1500591
58. Melsen JE, Lugthart G, Lankester AC, Schilham MW. Human circulating and tissue-resident CD56(bright) natural killer cell populations. Front Immunol. (2016) 7:262. doi: 10.3389/fimmu.2016.00262
59. Pegram HJ, Andrews DM, Smyth MJ, Darcy PK, Kershaw MH Activating and inhibitory receptors of natural killer cells. Immunol Cell Biol. (2011) 89:216–24. doi: 10.1038/icb.2010.78
60. Freud AG, Mundy-Bosse BL, Yu J, Caligiuri MA. The broad spectrum of human natural killer cell diversity. Immunity. (2017) 47:820–33. doi: 10.1016/j.immuni.2017.10.008
61. Boudreau JE, Hsu KC. Natural killer cell education in human health and disease. Curr Opin Immunol. (2018) 50:102–11. doi: 10.1016/j.coi.2017.11.003
62. Varchetta S, Oliviero B, Mavilio D, Mondelli MU. Different combinations of cytokines and activating receptor stimuli are required for human natural killer cell functional diversity. Cytokine. (2013) 62:58–63. doi: 10.1016/j.cyto.2013.02.018
63. Kumar S. Natural killer cell cytotoxicity and its regulation by inhibitory receptors. Immunology. (2018) 154:383–93. doi: 10.1111/imm.12921
64. Collins PL, Cella M, Porter SI, Li S, Gurewitz G, Hong HS, et al. Gene regulatory programs conferring phenotypic identities to human NK cells. Cell. (2019) 176:348–60.e12. doi: 10.1016/j.cell.2018.11.045
65. Zhang C, Zhang J, Tian Z. The regulatory effect of natural killer cells: do “NK-reg cells” exist? Cell Mol Immunol. (2006) 3:241–54.
66. Deniz G, Akdis M, Aktas E, Blaser K, Akdis C. Human NK1 and NK2 subsets determined by purification of IFN-γ-secreting and IFN-γ-nonsecreting NK cells. Eur J Immunol. (2002) 32:879–84. doi: 10.1002/1521-4141(200203)32:3<879::AID-IMMU879>3.0.CO;2-2
67. Gianchecchi E, Delfino DV, Fierabracci A. NK cells in autoimmune diseases: linking innate and adaptive immune responses. Autoimmun Rev. (2018) 17:142–54. doi: 10.1016/j.autrev.2017.11.018
68. Poggi A, Zocchi MR. NK cell autoreactivity and autoimmune diseases. Front Immunol. (2014) 5:27. doi: 10.3389/fimmu.2014.00027
69. Laroni A, Armentani E, Kerlero de Rosbo N, Ivaldi F, Marcenaro E, Sivori S, et al. Dysregulation of regulatory CD56bright NK cells/T cells interactions in multiple sclerosis. J Autoimmun. (2016) 72:8–18.doi: 10.1016/j.jaut.2016.04.003
70. Johansson S, Berg L, Hall H, Höglund P. NK cells: elusive players in autoimmunity. Trends Immunol. (2005) 26:613–18. doi: 10.1016/j.it.2005.08.008
71. Ferlazzo G, Morandi B. Cross-talks between natural killer cells and distinct subsets of dendritic cells. Front Immunol. (2014) 5:159. doi: 10.3389/fimmu.2014.00159
72. Crome SQ, Lang PA, Lang KS, Ohashi PS. Natural killer cells regulate diverse T cell responses. Trends Immunol. (2013) 34:342–9. doi: 10.1016/j.it.2013.03.002
73. Ehlers M, Papewalis C, Stenzel W, Jacobs B, Meyer KL, Deenen R, et al. Immunoregulatory natural killer cells suppress autoimmunity by down-regulating antigen-specific CD8+ T cells in mice. Endocrinology. (2012) 153:4367–79. doi: 10.1210/en.2012-1247
74. Smeltz RB, Wolf NA, Swanborg RH. Inhibition of autoimmune T cell responses in the DA rat by bone marrow-derived NK cells in vitro: implications for autoimmunity. J Immunol. (1999) 163:1390–97.
75. Takahashi K, Aranami T, Endoh M, Miyake S, Yamamura T. The regulatory role of natural killer cells in multiple sclerosis. Brain. (2004) 127:1917–27. doi: 10.1093/brain/awh219
76. Mimpen M, Smolders J, Hupperts R, Damoiseaux J. Natural killer cells in multiple sclerosis: a review. Immunol Lett. (2020) 222:1–11. doi: 10.1016/j.imlet.2020.02.012
77. Schleinitz N, Vély F, Harlé JR, Vivier E. Natural killer cells in human autoimmune diseases. Immunology. (2010) 131:451–8. doi: 10.1111/j.1365-2567.2010.03360.x
78. Li C, Mu R, Lu XY, He J, Jia RL, Li ZG. Antilymphocyte antibodies in systemic lupus erythematosus: association with disease activity and lymphopenia. J Immunol Res. (2014) 2014:67212. doi: 10.1155/2014/672126
79. Castriconi R, Carrega P, Dondero A, Bellora F, Casu B, Regis S, et al. Molecular mechanisms directing migration and retention of natural killer cells in human tissues. Front Immunol. (2018) 9:2324. doi: 10.3389/fimmu.2018.02324
80. Dastmalchi R, Farazmand A, Noshad S, Mozafari M, Mahmoudi M, Esteghamati A, et al. Polymorphism of killer cell immunoglobulin-like receptors (KIR) and their HLA ligands in Graves' disease. Mol Biol Rep. (2014) 41:5367–74. doi: 10.1007/s11033-014-3408-y
81. Schenk A, Bloch W, Zimmer P. Natural killer cells-an epigenetic perspective of development and regulation. Int J Mol Sci. (2016) 17:326. doi: 10.3390/ijms17030326
82. Smith EM, Phan M, Kruger TE, Coppenhaver DH, Blalock JE. Human lymphocyte production of immunoreactive thyrotropin. Proc Natl Acad Sci USA. (1982) 80:6010–13. doi: 10.1073/pnas.80.19.6010
83. Wang HC, Drago J, Zhou Q, Klein JR. An intrinsic thyrotropin-mediated pathway of TNFα production by bone marrow cells. Blood. (2003) 101:119–23. doi: 10.1182/blood-2002-02-0544
84. Mooij P, Simons PJ, de Haan-Meulman M, de Wit HJ, Drexhage HA. Effect of thyroid hormones and other iodinated compounds on the transition of monocytes into veiled/dendritic cells: role of granulocyte-macrophage colony-stimulating factor, tumour-necrosis factor-alpha and interleukin-6. J Endocrinol. (1994) 140:503–12. doi: 10.1677/joe.0.1400503
85. Montesinos MDM, Pellizas CG. Thyroid hormone action on innate immunity. Front Endocrinol. (2019) 10:486. doi: 10.3389/fendo.2019.00486
86. van Der Spek AH, Fliers E, Boelen A. Thyroid hormone metabolism in innate immune cells. J Endocrinol. (2017) 232:R67–R81. doi: 10.1530/JOE-16-0462
87. De Vito P, Incerpi S, Pedersen JZ, Luly P, Davis FB, Davis PJ. Thyroid hormones as modulators of immune activities at cellular level. Thyroid. (2011) 8:879–90. doi: 10.1089/thy.2010.0429
88. Angelin-Duclos C, Domenget C, Kolbus A, Beug H, Jurdic P, Samarut J. Thyroid hormone T3 acting through the thyroid hormone α receptor is necessary for implementation of erythropoiesis in the neonatal spleen environment in the mouse. Development. (2005) 132:925–34. doi: 10.1243/dev.01648
89. Pallinger E, Kovacs P, Csaba G. Presence of hormones (triiodothyronine, serotonin and histamine) in the immune cells of newborn rats. Cell Biol Int. (2005) 29:826–30. doi: 10.1016/j.cellbi.2005.05.010
90. Csaba G, Kovacs P, Pallinger E. Effect of the inhibition of triiodothyronine (T3) production by thiamazole on the T3 and serotonin content of immune cells. Life Sci. (2011) 76:2043–52. doi: 10.1016/j.lfs.2004.07.031
91. Dedecjus M, Stasiolek M, Brzezinski J, Selmaj K, Lewinski A. Thyroid hormones influence human dendritic cells' phenotype, function, subsets distribution. Thyroid. (2010) 21:533–40. doi: 10.1089/thy.2010.0183
92. Mascanfroni ID, Montesinos, Mdel M, Alamino VA, Susperreguy S, Nicola JP, et al. Nuclear factor (NF)-kappa B-dependent thyroid hormone receptor beta1 expression controls dendritic cell function via Akt signaling. J Biol Chem. (2010) 285:9569–82. doi: 10.1074/jbc.M109.071241
93. Mascanfroni I, Montesinos, Mdel M., Susperreguy S, Cervi L, Ilarregui JM, et al. Control of dendritic cell maturation and function by triiodothyronine. Faseb J. (2008) 22:1032–42. doi: 10.1096/fj.078652com
95. Sharma SD, Tsai V, Proffitt MR. Enhancement of mouse natural killer cell activity by thyroxine. Cell Immunol. (1982) 73:83–97.
96. Provinciali M, Muzzioli M, Fabris N. Thyroxine-dependent modulation of natural killer activity. J Exp Pathol. (1987) 3:617–22.
97. Klein JR, Wang HC. Characterization of a novel set of resident intrathyroidal bone marrow-derived hematopoietic cells: potential for immune-endocrine interactions in thyroid homeostasis. J Exp Biol. (2004) 207:55–65. doi: 10.1242/jeb.00710
98. Kruger TE, Blalock JE. Cellular requirements for thyrotropin enhancement of in vitro antibody production. J Immunol. (1986) 137:197–200.
99. Kruger TE, Smith EM, Harbour DV, Blalock JE. Thyrotropin: an endogenous regulator of the in vitro immune response. J Immunol. (1989) 142:744–7.
100. Bagriacik EU, Klein JR. The thyrotropin (thyroid stimulating hormone) receptor is expressed on murine dendritic cells and on a subset of CD43RB high lymph node T cells: functional role of thyroid stimulating hormone during immune activation. J Immunol. (2000) 164:6158–65. doi: 10.4049/jimmunol.164.12.6158
101. Landucci E, Laurino A, Cinci L, Gencarelli M, Raimondi L. Thyroid hormone, thyroid hormone metabolites and mast cells: a less explored issue. Front Cell Neurosc. (2019) 13:79. doi: 10.3389/fncel.2019.00079
102. Coutelier JP, Kehrl JH, Bellur SS, Kohn LD, Notkins AL, Prabhakar BS. Binding and functional effects of thyroid stimulating hormone to human immune cells. J Clin Immunol. (1990) 10:204–10. doi: 10.1007/bf00918653
103. Whetsell M, Bagriacik EU, Seetharamaiah GS, Prabhakar BS, Klein JR. Neuroendocrine-induced synthesis of bone marrow-derived cytokines with inflammatory immunomodulating properties. Cell Immunol. (1999) 15:159–66. doi: 10.1006/cimm.1998.1444
104. Klein JR. The immune system as a regulator of thyroid hormone activity. Exp Biol Med (Maywood). (2006) 231:229–36. doi: 10.1177/153537020623100301
105. Todd I, Pujol-Borrell R, Hammond LJ, McNally JM, Feldmann M, Bottazzo GF. Enhancement of thyrocyte HLA class II expression by thyroid stimulating hormone. Clin Exp Immunol. (1987) 69:524–31.
106. Dorshkind K, Horseman ND. The roles of prolactin, growth hormone, insulin-like growth factor-I, and thyroid hormones in lymphocyte development and function: insights from genetic models of hormone and hormone receptor deficiency. Endocrine Rev. (2005) 21:292–312. doi: 10.1210/edrv.21.2.0397
107. Provinciali M, Fabris N. Modulation of lymphoid cell sensitivity to interferon by thyroid hormones. J Endocrinol Invest. (1990) 13:187–91. doi: 10.1007/BF03349536
108. Provinciali M, Muzzioli M, Di Stefano G, Fabris N. Recovery of spleen cell natural killer activity by thyroid hormone treatment in old mice. Nat Immun Cell Growth Regul. (1991) 10:226–36.
109. Kmiec Z, Mysliwska J, Rachon D, Kotlarz G, Sworczak K, Mysliwski A. Natural killer activity and thyroid hormone levels in young and elderly persons. Gerontology. (2001) 47:282–8. doi: 10.1159/000052813
110. Lee EK, Sunwoo J. NK cells and thyroid disease. Endocrinol Metab. (2019) 34:132–7. doi: 10.3803/EnM.2019.34.2.132
111. Amino N, Mori H, Iwatani Y, Asari S, Izumiguchi Y. Peripheral K lymphocytes in autoimmune thyroid disease: decrease in Graves' disease and increase in Hashimoto's disease. J Clin End Metab. (1982) 54:587–91. doi: 10.1210/jcem-54-3-587
112. Iwatani Y, Amino N, Kabutomori O, Mori H, Tamaki H, Motoi S, et al. Decrease of peripheral large granular lymphocytes in Graves' disease. Clin Exp Immunol. (1984) 55:239–44.
113. Stein-Streilein J, Zakarija M, Papic M, McKenzie MJ. Hyperthyroxinemic mice have reduced natural killer cell activity. Evidence for a defective trigger mechanism. J Immunol. (1987) 139:2502–7.
114. Papic M, Stein-Streilein J, Zakarija M, McKenzie JM, Guffee J, Fletcher MA. Suppression of peripheral blood natural killer cell activity by excess thyroid hormone. J Clin Invest. (1987) 79:404–8. doi: 10.1172/JCI112826
115. Wang PW, Luo SF, Huang BY, Lin JD, Huang MJ. Depressed natural killer activity in Graves' disease and during antithyroid medication. Clin Endocrinol. (1988) 28:205–14. doi: 10.111/j.1365-2265.1988.tb03657.x
116. Pedersen BK, Feldt-Rasmussen U, Bech K, Perrild H, Klarlund K, Høier-Madsen M. Characterization of the natural killer cell activity in Hashimoto's and Graves' diseases. Allergy. (1989) 44:477–81. doi: 10.1111/j.1398-9995.1989.tb04186.x
117. Lee MS, Hong WS, Hong SW, Lee JO, Kang TW. Defective response of natural killer activity to thyroxine in Graves' disease. Korean J Intern Med. (1990) 5:93–96. doi: 10.3904/kjim.1990.5.2.93
118. Hidaka Y, Amino N, Iwatani Y, Kaneda T, Nasu M, Mitsuda N, et al. Increase in peripheral natural killer cell activity in patients with autoimmune thyroid disease. Autoimmunity. (1992) 11:239–46. doi: 10.3109/08916939209035161
119. Aust G, Lehmann I, Heberling HJ. Different immunophenotype and autoantibody production by peripheral blood and thyroid-derived lymphocytes in patients with Graves' disease. Exp Clin Endocrinol Diab. (1996) 104:50–58. doi: 10.1055/s-0029-1211422
120. Wenzel BE, Chow A, Baur R, Schleusener H, Wall JR. Natural Killer cell activity in patients with Graves' disease and Hashimoto's thyroiditis. Thyroid. (1998) 8:1019–22. doi: 10.1089/thy.1998.8.1019
121. Solerte S, Precerutti S, Gazzaruso C, Locatelli E, Zamboni M, Schifino N, et al. Defect of a subpopulation of natural killer immune cells in Graves' disease and Hashimoto's thyroiditis: normalizing effect of dehydroepiandrosterone sulfate. Eur J Endocrinol. (2005) 152:703–12. doi: 10.1530/eje.1.01906
122. Zhang Y, Ly G, Lou X, Peng D, Qu X, Yang X, et al. NKG2A expression and impaired function of NK cells in patients with new onset of Graves' disease. Int Immunopharmacol. (2015) 24:133–9. doi: 10.1016/j.intimp.2014.09.020
123. Mori H, Amino N, Iwatani Y, Asari S, Izumiguchi Y, Kumahara Y, et al. Decrease of immunoglobulin G-Fc receptor-bearing T lymphocytes in Graves' disease. J Clin End Metab. (1982) 55:399–402. doi: 10.1210/jcem-55-3-399
124. Gallagher TF, Hellman L, Finkelstein J, Yoshida K, Weitzman ED, Roffward HD, et al. Hyperthyroidism and cortisol secretion in man. J Clin End Metab. (1972) 34:919–27. doi: 10.1210/jcem-34-6-919
125. Bonnyns M, Cano P, Osterland CK, Mckenzie JM. Immune reactions in patients with Graves' disease. Am J Med. (1978) 65:971–7. doi: 10.1016/002-9343(78)90749-0
126. Johnson E, Kamilaris T, Calogero A, Gold P, Chrousos G. Experimentally-induced hyperthyroidism is associated with activation of the rat hypothalamic-pituitary-adrenal axis. Eur J Endocrinol. (2005) 153:177–85. doi: 10.1530/eje.1.01923
127. Yabuhara A, Yang FC, Nakazawa T, Iwasaki Y, Mori T, Koike K. A killing defect of natural killer cells as an underlying immunological abnormality in childhood systemic lupus erythematosus. J Rheumatol. (1996) 23:171–7.
128. Finkelstein JW, Royar RM, Hellman L. Growth hormone secretion in hyperthyroidism. J Clin End Metab. (1974) 38:634–7. doi: 10.1210/jcem-38-4-634
129. Chopra IJ. Gonadal steroids gonadotropins in hyperthyroidism. Med Clin North Am. (1975) 59:1109–21. doi: 10.1016/s0025-7125(16)31961-7
130. Judge SJ, Murphy WJ, Canter RJ. Characterizing the dysfunctional NK cell: assessing the clinical relevance of exhaustion, anergy, and senescence. Front Cell Infect Microbiol. (2020) 10:49. doi: 10.3389/fcimb.2020.00049
131. Marazuela M, Vargas JA, Alvarez-Mon M, Albarran F, Lucas T, Durantez A. Impaired natural killer cell cytotoxicity in peripheral blood mononuclear cells in Graves' disease. Eur J Endocrinol. (1995) 132:175–80. doi: 10.1530/eje.0.1320175
132. Magnusson L, Barcenilla H, Pihl M, Bensing S, Carlsson PO, Casas R, et al. Mass cytometry studies of patients with autoimmune endocrine diseases reveal distinct disease-specific alterations in immune cell subsets. Front Immunol. (2020) 11:288. doi: 10.3389/fimmu.2020.00288
133. Rojano J, Sasián S, Gavilán I, Aguilar M, Escobar L, Girón JA. Serial analysis of the effects of methimazole or radical therapy on circulating CD16/56 subpopulations in Graves' disease. Eur J Endocrinol. (1998) 139:314–16. doi: 10.1530/eje.01390314
134. McGregor AM, Petersen MM, McLachlan SM, Rooke P, Smith BR, Hall R. Carbimazole and autoimmune response in Graves' disease. N Engl J Med. (1980) 7:302–7. doi: 10.1056/NEJM198008073030603
135. Ben-Shmuel A, Biber G, Barda-Saad M. Unleashing natural killer cells in the tumor microenvironment-the next generation of immunotherapy? Front Immunol. (2020) 11:275. doi: 10.3389/fimmu.2020.00275
Keywords: natural killer cells, Graves' disease, autoimmunity, hyperthyroidism, inflammation
Citation: Gallo D, Piantanida E, Gallazzi M, Bartalena L, Tanda ML, Bruno A and Mortara L (2020) Immunological Drivers in Graves' Disease: NK Cells as a Master Switcher. Front. Endocrinol. 11:406. doi: 10.3389/fendo.2020.00406
Received: 11 April 2020; Accepted: 20 May 2020;
Published: 17 July 2020.
Edited by:
Yuji Nagayama, Nagasaki University, JapanReviewed by:
Giampaolo Papi, Local Health Unit of Modena, ItalyTakao Ando, Nagasaki University Hospital, Japan
Copyright © 2020 Gallo, Piantanida, Gallazzi, Bartalena, Tanda, Bruno and Mortara. This is an open-access article distributed under the terms of the Creative Commons Attribution License (CC BY). The use, distribution or reproduction in other forums is permitted, provided the original author(s) and the copyright owner(s) are credited and that the original publication in this journal is cited, in accordance with accepted academic practice. No use, distribution or reproduction is permitted which does not comply with these terms.
*Correspondence: Eliana Piantanida, ZWxpYW5hLnBpYW50YW5pZGEmI3gwMDA0MDt1bmluc3VicmlhLml0; Lorenzo Mortara, bG9yZW56by5tb3J0YXJhJiN4MDAwNDA7dW5pbnN1YnJpYS5pdA==
†These authors have contributed equally to this work