- 1Department of Nuclear Medicine, Tongji Hospital, Tongji Medical College, Huazhong University of Science and Technology, Wuhan, China
- 2Department of Biology, Massachusetts Institute of Technology, Cambridge, MA, United States
- 3Department of Endocrinology, Internal Medicine, Tongji Hospital, Tongji Medical College, Huazhong University of Science and Technology, Wuhan, China
Brown fat and beige fat are known as thermogenic fat due to their contribution to non-shivering thermogenesis in mammals following cold stimulation. Beige fat is unique due to its origin and its development in white fat. Subsequently, both brown fat and beige fat have become viable targets to combat obesity. Over the last few decades, most therapeutic strategies have been focused on the canonical pathway of thermogenic fat activation via the β3-adrenergic receptor (AR). Notwithstanding, administering β3-AR agonists often leads to side effects including hypertension and particularly cardiovascular disease. It is thus imperative to search for alternative therapeutic approaches to combat obesity. In this review, we discuss the current challenges in the field with respect to stimulating brown/beige fat thermogenesis. Additionally, we include a summary of other newly discovered pathways, including non-AR signaling- and non-UCP1-dependent mechanisms, which could be potential targets for the treatment of obesity and its related metabolic diseases.
Introduction
In recent years, obesity has become an ever-growing public health crisis. Its related diseases include type 2 diabetes, hypertension, cardiovascular disease, and cancer. The treatments for obesity have been shown to be minimally effective and often come with a slew of side effects. Generally, the production of heat is accompanied by a concomitant increase in the lipolysis of triglycerides and the oxidation of fatty acids (1). Thus, stimulating thermogenesis is a useful tool with which to combat obesity. In addition to shivering thermogenesis, non-shivering thermogenesis plays an important role in energy homeostasis. It was originally thought to occur only in newborn humans as a means to maintain their body temperatures as there exists abundant brown fat in their body. However, in 2007, brown fat was discovered in adult humans using 18F-fluorodeoxyglucose positron emission tomography/computed tomography (18FFDG-PET/CT)-based imaging (2). Importantly, the activity of brown fat in humans is negatively correlated to body mass index (BMI) and positively correlated to glucose tolerance as well as insulin sensitivity (3). Thus, non-shivering thermogenesis has become an area of interest as a means to promote more robust basal metabolism and consequently reduce the prevalence of diseases caused by a surplus of energy stores.
Canonically, the metabolic effect of brown fat is mediated by the activation of β-adrenergic signaling and the regulatory effect of uncoupling protein 1 (UCP1). The former is mediated by norepinephrine which is released from the sympathetic nerve terminals, and the latter contributes to the generation of heat through the mitochondria (4). As a result, most efforts to induce brown fat thermogenesis in mammals have focused on developing β3-adrenergic receptor (AR) agonists. However, β3-AR is not specific to adipose tissue, and its global activation oftentimes leads to deleterious side effects. For this reason, recent efforts in the field have focused on better understanding the mechanisms of brown fat activation that bypass ARs.
Brown/Beige Adipose Tissue Biology
Brown/Beige Adipose Tissue
In a healthy adult human, as much as 20–35% of the body weight is composed of white adipose tissue (WAT) (5), located predominantly in the subcutaneous and the visceral regions of the body. However, during disease states such as obesity, BMI can be above 30 kg/m2. WAT serves as the main energy store for the body, while brown adipose tissue (BAT) dissipates energy into heat via non-shivering thermogenesis (6–8).
In humans, BAT is located primarily in the cervical, supra-clavicular, supra-adrenal, and para-spinal regions (2). Morphologically, brown adipocytes are composed of multilocular small droplets and abundant mitochondria, which play a crucial role in non-shivering thermogenesis. BAT innervation by the sympathetic nervous system is important for its development and activation (9). Classically, following cold exposure, norepinephrine is released from the sympathetic nervous system. It then binds to the β3-AR in brown adipocytes, leading to an activation of adenylyl cyclase, an increase in cAMP levels, and the activation of protein kinase A (PKA). This, in turn, induces lipolysis in brown adipocytes. Moreover, UCP1, a mitochondrial membrane protein expressed primarily in BAT, has been shown to play a key role in the process of non-shivering thermogenesis. It uncouples the respiratory chain of oxidative phosphorylation within the mitochondria, leading to a production of transmembrane proton flow and generation of heat. Prolonged β3-adrenergic stimulation has been demonstrated to be necessary for sustained thermogenic activity (10).
Beige adipocytes were defined by the Spiegelman group in 2012 (11). However, brown-like adipocytes in mice was described as early as 1984 by Young et al. (12). The cells were found to be distributed in WAT after cold exposure or adrenergic stimulation. Furthermore, beige adipocytes appear morphologically similar to brown adipocytes, express UCP1, and also generate heat in the form of non-shivering thermogenesis (13, 14). They are innervated by the sympathetic nervous system as well (14). Indeed the density of noradrenergic fibers dramatically increases in murine WAT depot after cold stimulation or transgenic overexpression of protein PR domain containing 16 (PRDM16), which is a main regulator of brown adipogenesis (15). This indicates the importance of sympathetic stimulus in the development of beige adipocytes. The presence of beige adipocytes in humans is supported not only by 18FFDG-PET/CT imaging but also by anatomical and transcriptome profiling, revealing that the supra-clavicular region of 18FFDG-positive depots mainly consists of beige adipocytes (16), while the cervical region consists of classical brown adipocytes (17).
Targeting Brown/Beige Fat Thermogenesis
While skeletal muscle-mediated shivering thermogenesis consumes a great deal of energy in cold, non-shivering thermogenesis contributes to energy expenditure even at low levels of cold stimulation. It has been shown that both BAT and skeletal muscle play a role in non-shivering thermogenesis (18, 19). Under mild cold conditions, UCP1-based thermogenesis in BAT and sarcolipin-based thermogenesis in skeletal muscle work synergistically. When either thermogenic processes is impaired, the other is upregulated to maintain temperature homeostasis in mice (20). However, the mechanism of this functional crosstalk between BAT and skeletal muscle remains unclear. Furthermore, during prolonged cold exposure, muscle shivering intensity decreases while BAT activity increases (21). This suggests a pivotal role of BAT in thermogenesis under thermal stress. Therefore, increasing BAT mass and activity by stimulating its development and adrenergic response can be strategies to combat obesity in mammals.
Crucially, scientists have discovered that classical brown adipocytes share a common progenitor with skeletal myocytes (22). It has been shown that PRDM16, peroxisome proliferator-activated receptor γ (PPARγ), and CCAAT/enhancer-binding protein β (C/EBPβ) are master regulators of brown adipogenesis. PRDM16 has been shown to control the switch between skeletal myoblasts and brown adipocytes (22). Moreover, it binds directly to PPARγ to stimulate brown adipogenesis. C/EBPβ has been shown to play a crucial role in BAT development as well (23), binding to PRDM16 and initiating the switch from myoblast to BAT differentiation (24). Additionally, data indicate that PRDM16 binds to many other regulatory factors including peroxisome proliferator-activated receptor γ-coactivator 1α (PGC1α), PGC1β, euchromatic histone-lysine N-methyltransferase 1 (EHMT1), C-terminal-binding proteins (CtBPs), and early B cell factor-2 (EBF2). It likely forms a complex with these factors to regulate brown/beige adipocyte development (25–28). Although active BAT has been detected by 18FFDG-PET/CT imaging in adult humans after cold stimulation, it has primarily been found in people who are young and lean, with a lower BMI (3). Numerous studies have indicated that BAT activity is inversely related to BMI (8, 29–31). This may also likely be attributed to the increase in cold insulation and the subsequent protection of heat loss associated with higher adiposity. This paradox presents a challenge in simply targeting BAT to treat obese patients.
Since beige fat in humans is gradually recognized (16), scientists have honed on inducing beige adipogenesis to combat a variety of metabolic disorders. Unlike white or classic brown adipocytes, the origin of beige adipocytes is extremely heterogenous. Beige adipocytes have been reported to be transdifferentiated from white adipocytes (32, 33) or directly differentiated from distinct progenitors including PDGFRα+ (34), mural (35, 36), or MyoD+ progenitors (37). Numerous studies indicate that UCP1, one of the main regulators of adaptive thermogenesis, contributes to beige fat development (38–40). Moreover, classical beige adipocytes are governed by PRDM16 as well (41, 42). Deacetylation of PRDM16 and PPARγ by sirtuin 1 (SIRT1) stabilizes the PRDM16/PPARγ complex, contributing to beige adipogenesis (39, 43). Alternatively, SIRT1 is activated and regulated by Ca2+/calmodulin-dependent protein kinase β (CaMKKβ) and AMP-activated protein kinase (AMPK) (44–46), the latter of which plays a role in fatty acid oxidation. Other positive regulators of beige adipogenesis include bone morphogenetic proteins (47) and fibroblast growth factor 21 (48).
For years, targeting the β-adrenergic signaling pathway has been the therapeutic strategy to induce beige adipogenesis and thereby combat obesity. A variety of natural compounds and clinical medications used for treating metabolic diseases, shown in Table 1, have been shown to induce beige fat development. Of note, irisin and berberine are two molecules which show stimulatory effects on beige fat and brown fat in humans (51, 55).
Potential Anti-obesity Drugs and Their Safety
Adrenergic Receptor Agonists
Adrenergic signaling, in particular β3-AR, is a well-established pathway for BAT activation and beige fat development in response to cold temperatures. Common selective β3-AR agonists and antagonists have been summarized in a 2011 review by Bhadada et al. (92). Several β3-AR agonists have been shown to induce thermogenesis (93, 94). However, β3-AR are distributed throughout the body, including in the central nervous system, myocardium, blood vessels, smooth gastrointestinal and skeletal muscles, gallbladder, urinary bladder, prostate, etc. (95). Potential binding of β3-AR agonists with receptors located elsewhere may cause unexpected side effects.
Currently, some β3-AR agonists including mirabegron, vibegron, ritobegron, and solabegron have been extensively investigated. Some have even been approved for clinical use to treat overactive bladders and urinary incontinence (96–98). Although mirabegron has been found to induce BAT activity as measured by 18FFDG-PET/CT (99), increase non-esterified fatty acids by up to 68%, and boost resting energy expenditure by up to 5.8% (100) in humans, no β3-AR agonists has been approved to treat metabolic disorders thus far. The most common off-target binding sites of β3-AR agonists are myocardium and blood vessels (92, 101–103). Notably, it has been indicated that β3-AR stimulation is related to heart failure because of the negative inotropic effect of β3-AR agonists (104, 105). Additionally, different agonists present inconsistent effects on blood vessels (92). Some cause vasodilation, which may give rise to tachycardia, while others promote vasoconstriction, which is associated with high blood pressure. These potentially fatal side effects make β3-AR agonists unsuitable stimulants for thermogenic activity in the clinic.
PPARγ Receptor Agonists
PPAR receptors also play a critical role in regulating whole-body energy homeostasis. These receptors are abundantly expressed in adipose tissue, liver, and skeletal muscle, in addition to immune and gastrointestinal systems, and are known to regulate brown adipogenesis as well as glucose uptake and lipid biosynthesis in WAT (106, 107). PPARγ receptor agonists, such as troglitazone, rosiglitazone, and pioglitazone, have been applied to treat metabolic disorders and type 2 diabetes due to their insulin-sensitizing effects (108). However, due to side effects such as hepatotoxicity, myocardial infarction, bladder cancer, and heart failure, PPARγ receptor agonists have largely been withdrawn from the market (109). Although some PPARγ receptor agonists, such as pioglitazone, have been shown to cause weight gain in humans (110, 111), studies have indicated that rosiglitazone may induce beige fat development in mice through the activation of the SIRT1–PRDM16 pathway (41, 43). This suggests that PPARγ receptor agonists may be leveraged to combat obesity. Yet due to the potentially fatal side effects mentioned above, their clinical use remains problematic. Currently, several dual-acting PPARγ agonists have been synthesized. Promising studies have shown that certain PPARγ agonists may be beneficial in treating metabolic disorders with minimal off-target effects (112).
Non-canonical Mechanisms Involved in Non-shivering Thermogenesis
AR activation triggers the process of non-shivering thermogenesis in response to cold, as shown in Figure 1, while mitochondrial membrane protein UCP1 is the key driver of heat production in BAT. The UCP1 levels in beige fat are lower than in BAT. This has previously led to the misconception that the contribution of beige fat in the regulation of whole-body energy balance is marginal (113). However, UCP1 knockout mice without functional BAT can gradually adapt to and survive cold temperatures by increasing their recruitment of beige fat (114, 115). This suggests that UCP1 may be dispensable for beige fat induction. This phenotype suggests that other UCP1-independent mechanisms are involved in beige fat-regulated energy homeostasis. Furthermore, several studies have identified other pathways which activate BAT or induce beige adipogenesis, independent of ARs signaling (37, 116, 117). Here we describe a few novel mechanisms that have recently been implicated in the thermogenic regulation of BAT and beige fat (Figure 1).
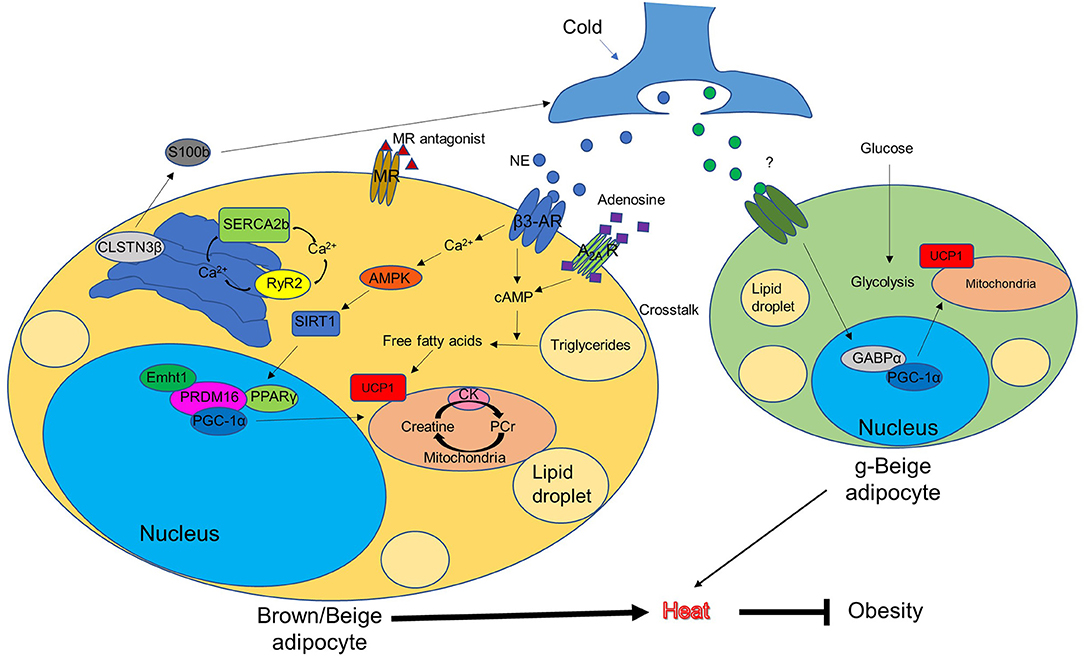
Figure 1. Conventional and unconventional mechanisms of brown/beige thermogenesis (potential approaches to combat obesity). AMPK, AMP-activated protein kinase; AR, adrenergic receptor; CK, creatine kinase; CLSTN3β, calsyntenin3β; Ehmt1, euchromatic histone-lysine N-methyltransferase 1; GABPα, GA-binding protein α; g-beige adipocyte, glycolytic-beige adipocyte; MR, mineralocorticoid receptor; NE, norepinephrine; PCr, phosphocreatine; PPARγ, peroxisome proliferator-activated receptor gamma; PGC-1α, PPARγ coactivator-1α; PRDM16, protein PR domain containing 16; RyR2, ryanodine receptor 2; SERCA2b, sarco/endoplasmic reticulum Ca2+-ATPase 2b; SIRT1, sirtuin 1; UCP1, uncoupling protein 1.
Adenosine–A2A Receptor Signaling
A 2014 paper from the Pfeifer Lab describes adenosine–A2A receptor signaling in response to sympathetic stimulation, which reduces levels of diet-induced obesity and improves glucose tolerance (116). After sympathetic stimulation by norepinephrine, brown adipocytes themselves release adenosine, which binds to A2A receptors and contributes to energy expenditure. A2A receptor knockout mice exhibit reduced thermogenesis and oxygen consumption in cold conditions compared to wild-type mice. Conversely, A2A agonist treatment increases BAT activation and energy expenditure in mice. This highlights the important role of A2A receptor in the regulation of energy expenditure in BAT. Furthermore, A2A stimulation by either its pharmacological activators or overexpression using lentiviral vector injections protects mice from diet-induced obesity while inducing beige fat development.
Mineralocorticoid Receptor Antagonism
In mice, mineralocorticoid receptor antagonists prevent high fat diet-induced decline in glucose tolerance and induce beige fat development in visceral and inguinal WAT as indicated by an upregulation of brown adipocyte-specific transcripts and increased levels of UCP1. These findings correspond to the results detected by 18FFDG-PET/CT (117). Mineralocorticoid receptor antagonists reduce the autophagic rate in WAT depots. Moreover, when autophagy is repressed using its repressor bafilomycin A1, the effects mimic that of mineralocorticoid receptor antagonists. Furthermore, a more recent study in humans also indicates a positive correlation between mineralocorticoid receptor antagonism and BAT thermogenesis (118), suggesting the potential therapeutic benefit of mineralocorticoid receptor antagonism on obesity.
Calsyntenin3β-S100b Signaling
A recent study from Spiegelman's group has identified a thermogenic adipocyte-specific protein [calsyntenin3β (CLSTN3β)], which is primarily located on the endoplasmic reticulum. This protein promotes sympathetic innervation in adipose tissue in mice (119). Knockout or transgenic overexpression of CLSTN3β in mice impairs or enhances sympathetic innervation in BAT, respectively. CLSTN3β activation leads to the secretion of S100b, a trophic factor which stimulates neurite outgrowth, from the thermogenic adipocytes. S100b deficiency reduces sympathetic innervation in BAT, while the forced expression of S100b rescues the phenotype caused by CLSTN3β ablation. Therefore, selectively targeting CLSTN3β-S100b in thermogenic adipocytes may minimize the off-target side effects in other organs and provide a new therapeutic opportunity for promoting thermogenic anti-obesity effects.
Creatine-Driven Substrate Cycling
Another study from Spiegelman's group has identified arginine/creatine metabolism as a beige fat signature using quantitative mitochondrial proteomics (120). It contributes to beige fat-mediated energy expenditure and thermal homeostasis in mice. Cold exposure stimulates the activity of mitochondrial creatine kinase, which promotes creatine metabolism and in turn, increases ATP demand and induces ADP-dependent mitochondrial respiration in beige fat. Notably, in mice lacking UCP1, creatine metabolism compensatorily induces whole-body energy expenditure in response to cold. Furthermore, researchers identified phosphatase orphan 1 as a regulator of creatine-driven adipocyte respiration. It is concluded that creatine metabolism could be potentially targeted to increase basal energy expenditure.
Sarco/Endoplasmic Reticulum Ca2+-ATPase 2b (SERCA2b)-Mediated Calcium Cycling
Another UCP1-independent signaling pathway in beige fat was described by our group. This novel mechanism involves sarco/endoplasmic reticulum Ca2+-ATPase 2b (SERCA2b)-mediated calcium cycling, which ultimately regulates glucose metabolism (121). Unlike brown adipocytes, beige adipocytes display higher ATP synthesis capacity. In the absence of UCP1, they gain fuel from glucose through multiple metabolic ways including glycolysis, TCA metabolism, and the mitochondrial electron transport chain through the SERCA2b-ryanodine receptor 2 (RyR2) pathway. Of note, the transgenic overexpression of PRDM16 is still able to protect mice from diet-induced obesity in the absence of UCP1. The present study strongly suggests that UCP1 is dispensable in beige fat for non-shivering thermogenesis. SERCA2b-mediated calcium cycling represents an evolutionarily conserved mechanism for maintaining energy homeostasis.
Glycolytic Beige Fat
Our discovery of a distinct form of thermogenic cell was revolutionary in the field of fat biology. This cell, which was termed glycolytic beige adipocyte, exhibits adaptive thermogenesis and energy homeostasis in cold conditions in the absence of β-ARs signaling (37). These unique beige adipocytes are differentiated from MyoD+ progenitors in inguinal WAT. The process is mediated by GA-binding protein α through a myogenic intermediate. To better understand the mechanism by which these cells improved glucose tolerance and increased basal metabolism, we created a glycolytic beige fat-deficient mouse model. We found that glucose uptake, as detected by 18FFDG-PET/CT, in the inguinal WAT of those mice is significantly reduced. Moreover, we noticed a decrease in oxygen consumption rate and extracellular acidification rate in isolated tissues. Glycolytic beige adipocytes are distinct from conventional beige adipocytes in their developmental origin, regulation, and enhanced glucose oxidation. This β-AR-independent pathway has opened up a new path for the treatment of obesity.
Discussion and Prospects
In mammals, brown fat and beige fat play a crucial role in non-shivering thermogenesis and energy homeostasis. Inducing their development or activation is a viable approach to combat obesity. Classic brown fat and beige fat thermogenesis is mediated by β3-AR signaling and UCP1. Previous research has focused on the development of β3-AR agonists or PPARγ agonists to treat metabolic disorders including obesity. However, the clinical outcomes are unsatisfactory due to their deleterious side effects. The added stress from these agonists to the cardiovascular systems is particularly harmful (103, 104, 108).
Alternative pathways which bypass canonical thermogenic regulators are of great interest. Surprisingly, UCP1 knockout mice and β-AR knockout mice are able to acclimate to cold environments (114, 115). This suggests that other compensatory pathways, independent of UCP1 or β-AR, are involved in regulating whole-body thermogenesis and energy homeostasis. Pathways associated with this acclimation, shown in Figure 1, include: two non-AR-dependent pathways mediated by other thermogenic cell-expressing receptors, such as A2A receptors and mineralocorticoid receptors, whose activation by adenosine or inhibition by its antagonists contribute to energy expenditure; the thermogenic adipocyte-specific CLSTN3β-S100b signaling pathway, which regulates thermogenesis through promoting the sympathetic innervation of the thermogenic adipose tissue; two distinct UCP1-independent pathways in beige fat, including creatine-driven substrate cycling and SERCA2b-RyR2 signaling, which compensate for the loss of UCP1 and contribute to energy expenditure; and a subtype of beige fat, originating from MyoD+ progenitors, which is required for thermal regulation in the absence of β-ARs signaling.
It is important to note that these signaling pathways may only be a small part of the mechanisms involved in the regulation of BAT and beige fat on thermogenesis. Particularly, the role of beige fat in heat generation seems to be extremely multifaceted and, as such, is an active area of research. Notably, our group has identified glycolytic beige fat, marking for the first time that a subtype of beige fat has been described. We believe that multiple subtypes of beige fat with distinct origins and unique biological characterizations may exist. It is likely that there exists a robust crosstalk between different thermogenic cell types to maintain energy balance under different conditions. A better understanding of the plasticity of beige fat as well as of brown fat will likely provide new discoveries on metabolic adaptation and thus new therapeutic approaches to combat metabolic disorders including obesity.
Author Contributions
RP and YC wrote the manuscript. RP, XZ, PM, and YC edited the manuscript.
Funding
This work was supported by a grant from Tongji Hospital in Huazhong University of Science and Technology (Grant No. 2201103295 to YC).
Conflict of Interest
The authors declare that the research was conducted in the absence of any commercial or financial relationships that could be construed as a potential conflict of interest.
The handling editor declared a past co-authorship with the authors YC.
References
1. Dawkins MJ, Scopes JW. Non-shivering thermogenesis and brown adipose tissue in the human new-born infant. Nature. (1965) 206:201–2. doi: 10.1038/206201b0
2. Nedergaard J, Bengtsson T, Cannon B. Unexpected evidence for active brown adipose tissue in adult humans. Am J Physiol Endocrinol Metab. (2007) 293:E444–52. doi: 10.1152/ajpendo.00691.2006
3. Matsushita M, Yoneshiro T, Aita S, Kameya T, Sugie H, Saito M. Impact of brown adipose tissue on body fatness and glucose metabolism in healthy humans. Int J Obes. (2014) 38:812–7. doi: 10.1038/ijo.2013.206
4. Rodriguez-Cuenca S, Pujol E, Justo R, Frontera M, Oliver J, Gianotti M, et al. Sex-dependent thermogenesis, differences in mitochondrial morphology and function, and adrenergic response in brown adipose tissue. J Biol Chem. (2002) 277:42958–63. doi: 10.1074/jbc.M207229200
5. Gallagher D, Visser M, Sepulveda D, Pierson RN, Harris T, Heymsfield SB. How useful is body mass index for comparison of body fatness across age, sex, and ethnic groups? Am J Epidemiol. (1996) 143:228–39. doi: 10.1093/oxfordjournals.aje.a008733
6. Rothwell NJ, Stock MJ. A role for brown adipose tissue in diet-induced thermogenesis. Nature. (1979) 281:31–5. doi: 10.1038/281031a0
7. Fischer AW, Schlein C, Cannon B, Heeren J, Nedergaard J. Intact innervation is essential for diet-induced recruitment of brown adipose tissue. Am J Physiol Endocrinol Metab. (2019) 316:E487–503. doi: 10.1152/ajpendo.00443.2018
8. Cypess AM, Lehman S, Williams G, Tal I, Rodman D, Goldfine AB, et al. Identification and importance of brown adipose tissue in adult humans. N Engl J Med. (2009) 360:1509–17. doi: 10.1056/NEJMoa0810780
9. Steiner G, Loveland M, Schonbaum E. Effect of denervation on brown adipose tissue metabolism. Am J Physiol. (1970) 218:566–70. doi: 10.1152/ajplegacy.1970.218.2.566
10. Young P, Wilson S, Arch JR. Prolonged beta-adrenoceptor stimulation increases the amount of GDP-binding protein in brown adipose tissue mitochondria. Life Sci. (1984) 34:1111–7. doi: 10.1016/0024-3205(84)90081-X
11. Wu J, Bostrom P, Sparks LM, Ye L, Choi JH, Giang AH, et al. Beige adipocytes are a distinct type of thermogenic fat cell in mouse and human. Cell. (2012) 150:366–76. doi: 10.1016/j.cell.2012.05.016
12. Young P, Arch JR, Ashwell M. Brown adipose tissue in the parametrial fat pad of the mouse. FEBS Lett. (1984) 167:10–4. doi: 10.1016/0014-5793(84)80822-4
13. Petrovic N, Walden TB, Shabalina IG, Timmons JA, Cannon B, Nedergaard J. Chronic peroxisome proliferator-activated receptor gamma (PPARgamma) activation of epididymally derived white adipocyte cultures reveals a population of thermogenically competent, UCP1-containing adipocytes molecularly distinct from classic brown adipocytes. J Biol Chem. (2010) 285:7153–64. doi: 10.1074/jbc.M109.053942
14. Seale P, Conroe HM, Estall J, Kajimura S, Frontini A, Ishibashi J, et al. Prdm16 determines the thermogenic program of subcutaneous white adipose tissue in mice. J Clin Invest. (2011) 121:96–105. doi: 10.1172/JCI44271
15. Murano I, Barbatelli G, Giordano A, Cinti S. Noradrenergic parenchymal nerve fiber branching after cold acclimatisation correlates with brown adipocyte density in mouse adipose organ. J Anat. (2009) 214:171–8. doi: 10.1111/j.1469-7580.2008.01001.x
16. Jespersen NZ, Larsen TJ, Peijs L, Daugaard S, Homoe P, Loft A, et al. A classical brown adipose tissue mRNA signature partly overlaps with brite in the supraclavicular region of adult humans. Cell Metab. (2013) 17:798–805. doi: 10.1016/j.cmet.2013.04.011
17. Cypess AM, White AP, Vernochet C, Schulz TJ, Xue R, Sass CA, et al. Anatomical localization, gene expression profiling and functional characterization of adult human neck brown fat. Nat Med. (2013) 19:635–9. doi: 10.1038/nm.3112
18. Aydin J, Shabalina IG, Place N, Reiken S, Zhang SJ, Bellinger AM, et al. Nonshivering thermogenesis protects against defective calcium handling in muscle. FASEB J. (2008) 22:3919–24. doi: 10.1096/fj.08-113712
19. Enerback S, Jacobsson A, Simpson EM, Guerra C, Yamashita H, Harper ME, et al. Mice lacking mitochondrial uncoupling protein are cold-sensitive but not obese. Nature. (1997) 387:90–4. doi: 10.1038/387090a0
20. Bal NC, Singh S, Reis FCG, Maurya SK, Pani S, Rowland LA, et al. Both brown adipose tissue and skeletal muscle thermogenesis processes are activated during mild to severe cold adaptation in mice. J Biol Chem. (2017) 292:16616–25. doi: 10.1074/jbc.M117.790451
21. Blondin DP, Daoud A, Taylor T, Tingelstad HC, Bezaire V, Richard D, et al. Four-week cold acclimation in adult humans shifts uncoupling thermogenesis from skeletal muscles to brown adipose tissue. J Physiol. (2017) 595:2099–113. doi: 10.1113/JP273395
22. Seale P, Bjork B, Yang W, Kajimura S, Chin S, Kuang S, et al. PRDM16 controls a brown fat/skeletal muscle switch. Nature. (2008) 454:961–7. doi: 10.1038/nature07182
23. Tanaka T, Yoshida N, Kishimoto T, Akira S. Defective adipocyte differentiation in mice lacking the C/EBPbeta and/or C/EBPdelta gene. EMBO J. (1997) 16:7432–43. doi: 10.1093/emboj/16.24.7432
24. Kajimura S, Seale P, Kubota K, Lunsford E, Frangioni JV, Gygi SP, et al. Initiation of myoblast to brown fat switch by a PRDM16-C/EBP-beta transcriptional complex. Nature. (2009) 460:1154–8. doi: 10.1038/nature08262
25. Seale P, Kajimura S, Yang W, Chin S, Rohas LM, Uldry M, et al. Transcriptional control of brown fat determination by PRDM16. Cell Metab. (2007) 6:38–54. doi: 10.1016/j.cmet.2007.06.001
26. Ohno H, Shinoda K, Ohyama K, Sharp LZ, Kajimura S. EHMT1 controls brown adipose cell fate and thermogenesis through the PRDM16 complex. Nature. (2013) 504:163–7. doi: 10.1038/nature12652
27. Kajimura S, Seale P, Tomaru T, Erdjument-Bromage H, Cooper MP, Ruas JL, et al. Regulation of the brown and white fat gene programs through a PRDM16/CtBP transcriptional complex. Genes Dev. (2008) 22:1397–409. doi: 10.1101/gad.1666108
28. Rajakumari S, Wu J, Ishibashi J, Lim HW, Giang AH, Won KJ, et al. EBF2 determines and maintains brown adipocyte identity. Cell Metab. (2013) 17:562–74. doi: 10.1016/j.cmet.2013.01.015
29. van Marken Lichtenbelt WD, Vanhommerig JW, Smulders NM, Drossaerts JM, Kemerink GJ, Bouvy ND, et al. Cold-activated brown adipose tissue in healthy men. N Engl J Med. (2009) 360:1500–8. doi: 10.1056/NEJMoa0808718
30. Saito M, Okamatsu-Ogura Y, Matsushita M, Watanabe K, Yoneshiro T, Nio-Kobayashi J, et al. High incidence of metabolically active brown adipose tissue in healthy adult humans: effects of cold exposure and adiposity. Diabetes. (2009) 58:1526–31. doi: 10.2337/db09-0530
31. Zingaretti MC, Crosta F, Vitali A, Guerrieri M, Frontini A, Cannon B, et al. The presence of UCP1 demonstrates that metabolically active adipose tissue in the neck of adult humans truly represents brown adipose tissue. FASEB J. (2009) 23:3113–20. doi: 10.1096/fj.09-133546
32. Barbatelli G, Murano I, Madsen L, Hao Q, Jimenez M, Kristiansen K, et al. The emergence of cold-induced brown adipocytes in mouse white fat depots is determined predominantly by white to brown adipocyte transdifferentiation. Am J Physiol Endocrinol Metab. (2010) 298:E1244–53. doi: 10.1152/ajpendo.00600.2009
33. Frontini A, Vitali A, Perugini J, Murano I, Romiti C, Ricquier D, et al. White-to-brown transdifferentiation of omental adipocytes in patients affected by pheochromocytoma. Biochim Biophys Acta. (2013) 1831:950–9. doi: 10.1016/j.bbalip.2013.02.005
34. Lee YH, Petkova AP, Granneman JG. Identification of an adipogenic niche for adipose tissue remodeling and restoration. Cell Metab. (2013) 18:355–67. doi: 10.1016/j.cmet.2013.08.003
35. Long JZ, Svensson KJ, Tsai L, Zeng X, Roh HC, Kong X, et al. A smooth muscle-like origin for beige adipocytes. Cell Metab. (2014) 19:810–20. doi: 10.1016/j.cmet.2014.03.025
36. Vishvanath L, MacPherson KA, Hepler C, Wang QA, Shao M, Spurgin SB, et al. Pdgfrbeta+ mural preadipocytes contribute to adipocyte hyperplasia induced by high-fat-diet feeding and prolonged cold exposure in adult mice. Cell Metab. (2016) 23:350–9. doi: 10.1016/j.cmet.2015.10.018
37. Chen Y, Ikeda K, Yoneshiro T, Scaramozza A, Tajima K, Wang Q, et al. Thermal stress induces glycolytic beige fat formation via a myogenic state. Nature. (2019) 565:180–5. doi: 10.1038/s41586-018-0801-z
38. Rachid TL, Penna-de-Carvalho A, Bringhenti I, Aguila MB, Mandarim-de-Lacerda CA, Souza-Mello V. Fenofibrate (PPARalpha agonist) induces beige cell formation in subcutaneous white adipose tissue from diet-induced male obese mice. Mol Cell Endocrinol. (2015) 402:86–94. doi: 10.1016/j.mce.2014.12.027
39. Baskaran P, Krishnan V, Ren J, Thyagarajan B. Capsaicin induces browning of white adipose tissue and counters obesity by activating TRPV1 channel-dependent mechanisms. Br J Pharmacol. (2016) 173:2369–89. doi: 10.1111/bph.13514
40. Bargut TCL, Souza-Mello V, Aguila MB, Mandarim-de-Lacerda CA. Browning of white adipose tissue: lessons from experimental models. Horm Mol Biol Clin Investig. (2017) 31. doi: 10.1515/hmbci-2016-0051
41. Ohno H, Shinoda K, Spiegelman BM, Kajimura S. PPARgamma agonists induce a white-to-brown fat conversion through stabilization of PRDM16 protein. Cell Metab. (2012) 15:395–404. doi: 10.1016/j.cmet.2012.01.019
42. Lo KA, Sun L. Turning WAT into BAT: a review on regulators controlling the browning of white adipocytes. Biosci Rep. (2013) 33:711–19. doi: 10.1042/BSR20130046
43. Qiang L, Wang L, Kon N, Zhao W, Lee S, Zhang Y, et al. Brown remodeling of white adipose tissue by SirT1-dependent deacetylation of PPARgamma. Cell. (2012) 150:620–32. doi: 10.1016/j.cell.2012.06.027
44. Passariello CL, Gottardi D, Cetrullo S, Zini M, Campana G, Tantini B, et al. Evidence that AMP-activated protein kinase can negatively modulate ornithine decarboxylase activity in cardiac myoblasts. Biochim Biophys Acta. (2012) 1823:800–7. doi: 10.1016/j.bbamcr.2011.12.013
45. Lau AW, Liu P, Inuzuka H, Gao D. SIRT1 phosphorylation by AMP-activated protein kinase regulates p53 acetylation. Am J Cancer Res. (2014) 4:245–55.
46. Peng Y, Rideout DA, Rakita SS, Gower WR Jr, You M, Murr MM, et al. Does LKB1 mediate activation of hepatic AMP-protein kinase (AMPK) and sirtuin1 (SIRT1) after Roux-en-Y gastric bypass in obese rats? J Gastrointest Surg. (2010) 14:221–8. doi: 10.1007/s11605-009-1102-5
47. Gustafson B, Hammarstedt A, Hedjazifar S, Hoffmann JM, Svensson PA, Grimsby J, et al. BMP4 and BMP antagonists regulate human white and beige adipogenesis. Diabetes. (2015) 64:1670–81. doi: 10.2337/db14-1127
48. Huang Z, Zhong L, Lee JTH, Zhang J, Wu D, Geng L, et al. The FGF21-CCL11 axis mediates beiging of white adipose tissues by coupling sympathetic nervous system to type 2 immunity. Cell Metab. (2017) 26:493–508.e4. doi: 10.1016/j.cmet.2017.08.003
49. Jimenez-Aranda A, Fernandez-Vazquez G, Campos D, Tassi M, Velasco-Perez L, Tan DX, et al. Melatonin induces browning of inguinal white adipose tissue in Zucker diabetic fatty rats. J Pineal Res. (2013) 55:416–23. doi: 10.1111/jpi.12089
50. Zhang Z, Zhang H, Li B, Meng X, Wang J, Zhang Y, et al. Berberine activates thermogenesis in white and brown adipose tissue. Nat Commun. (2014) 5:5493. doi: 10.1038/ncomms6493
51. Wu L, Xia M, Duan Y, Zhang L, Jiang H, Hu X, et al. Berberine promotes the recruitment and activation of brown adipose tissue in mice and humans. Cell Death Dis. (2019) 10:468. doi: 10.1038/s41419-019-1706-y
52. Yamashita Y, Wang L, Wang L, Tanaka Y, Zhang T, Ashida H. Oolong, black and pu-erh tea suppresses adiposity in mice via activation of AMP-activated protein kinase. Food Funct. (2014) 5:2420–9. doi: 10.1039/C4FO00095A
53. Rossato M, Granzotto M, Macchi V, Porzionato A, Petrelli L, Calcagno A, et al. Human white adipocytes express the cold receptor TRPM8 which activation induces UCP1 expression, mitochondrial activation and heat production. Mol Cell Endocrinol. (2014) 383:137–46. doi: 10.1016/j.mce.2013.12.005
54. Zhang Y, Li R, Meng Y, Li S, Donelan W, Zhao Y, et al. Irisin stimulates browning of white adipocytes through mitogen-activated protein kinase p38 MAP kinase and ERK MAP kinase signaling. Diabetes. (2014) 63:514–25. doi: 10.2337/db13-1106
55. Zhang Y, Xie C, Wang H, Foss RM, Clare M, George EV, et al. Irisin exerts dual effects on browning and adipogenesis of human white adipocytes. Am J Physiol Endocrinol Metab. (2016) 311:E530–41. doi: 10.1152/ajpendo.00094.2016
56. Mu Q, Fang X, Li X, Zhao D, Mo F, Jiang G, et al. Ginsenoside Rb1 promotes browning through regulation of PPARgamma in 3T3-L1 adipocytes. Biochem Biophys Res Commun. (2015) 466:530–5. doi: 10.1016/j.bbrc.2015.09.064
57. Kim K, Nam KH, Yi SA, Park JW, Han JW, Lee J. Ginsenoside Rg3 induces browning of 3T3-L1 adipocytes by activating AMPK signaling. Nutrients. (2020) 12. doi: 10.3390/nu12020427
58. Wang J, Sun GJ, Ding J, Zhang JX, Cui Y, Li HR, et al. WY14643 combined with all-trans retinoic acid acts via p38 MAPK to induce “browning” of white adipocytes in mice. Genet Mol Res. (2015) 14:6978–84. doi: 10.4238/2015.June.26.6
59. Wang S, Liang X, Yang Q, Fu X, Rogers CJ, Zhu M, et al. Resveratrol induces brown-like adipocyte formation in white fat through activation of AMP-activated protein kinase (AMPK) alpha1. Int J Obes. (2015) 39:967–76. doi: 10.1038/ijo.2015.23
60. Wang S, Wang X, Ye Z, Xu C, Zhang M, Ruan B, et al. Curcumin promotes browning of white adipose tissue in a norepinephrine-dependent way. Biochem Biophys Res Commun. (2015) 466:247–53. doi: 10.1016/j.bbrc.2015.09.018
61. Zhang X, Tian Y, Zhang H, Kavishwar A, Lynes M, Brownell AL, et al. Curcumin analogues as selective fluorescence imaging probes for brown adipose tissue and monitoring browning. Sci Rep. (2015) 5:13116. doi: 10.1038/srep13116
62. Nishikawa S, Aoyama H, Kamiya M, Higuchi J, Kato A, Soga M, et al. Artepillin C, a typical Brazilian propolis-derived component, induces brown-like adipocyte formation in C3H10T1/2 cells, primary inguinal white adipose tissue-derived adipocytes, and mice. PLoS ONE. (2016) 11:e0162512. doi: 10.1371/journal.pone.0162512
63. Chang YY, Su HM, Chen SH, Hsieh WT, Chyuan JH, Chao PM. Roles of peroxisome proliferator-activated receptor alpha in bitter melon seed oil-corrected lipid disorders and conversion of alpha-eleostearic acid into rumenic acid in C57BL/6J mice. Nutrients. (2016) 8:805. doi: 10.3390/nu8120805
64. Simopoulos A. The FTO gene, browning of adipose tissue and omega-3 fatty acids. J Nutrigenet Nutrigenomics. (2016) 9:123–6. doi: 10.1159/000448617
65. Simopoulos AP. An increase in the omega-6/omega-3 fatty acid ratio increases the risk for obesity. Nutrients. (2016) 8:128. doi: 10.3390/nu8030128
66. Song NJ, Choi S, Rajbhandari P, Chang SH, Kim S, Vergnes L, et al. Prdm4 induction by the small molecule butein promotes white adipose tissue browning. Nat Chem Biol. (2016) 12:479–81. doi: 10.1038/nchembio.2081
67. Liu D, Bordicchia M, Zhang C, Fang H, Wei W, Li JL, et al. Activation of mTORC1 is essential for beta-adrenergic stimulation of adipose browning. J Clin Invest. (2016) 126:1704–16. doi: 10.1172/JCI83532
68. Laiglesia LM, Lorente-Cebrian S, Prieto-Hontoria PL, Fernandez-Galilea M, Ribeiro SM, Sainz N, et al. Eicosapentaenoic acid promotes mitochondrial biogenesis and beige-like features in subcutaneous adipocytes from overweight subjects. J Nutr Biochem. (2016) 37:76–82. doi: 10.1016/j.jnutbio.2016.07.019
69. Zhang X, Zhang QX, Wang X, Zhang L, Qu W, Bao B, et al. Dietary luteolin activates browning and thermogenesis in mice through an AMPK/PGC1alpha pathway-mediated mechanism. Int J Obes. (2016) 40:1841–9. doi: 10.1038/ijo.2016.108
70. Abdul-Rahman O, Kristof E, Doan-Xuan QM, Vida A, Nagy L, Horvath A, et al. AMP-activated kinase (AMPK) activation by AICAR in human white adipocytes derived from pericardial white adipose tissue stem cells induces a partial beige-like phenotype. PLoS ONE. (2016) 11:e0157644. doi: 10.1371/journal.pone.0157644
71. Kim HL, Jung Y, Park J, Youn DH, Kang J, Lim S, et al. Farnesol has an anti-obesity effect in high-fat diet-induced obese mice and induces the development of beige adipocytes in human adipose tissue derived-mesenchymal stem cells. Front Pharmacol. (2017) 8:654. doi: 10.3389/fphar.2017.00654
72. Imran KM, Rahman N, Yoon D, Jeon M, Lee BT, Kim YS. Cryptotanshinone promotes commitment to the brown adipocyte lineage and mitochondrial biogenesis in C3H10T1/2 mesenchymal stem cells via AMPK and p38-MAPK signaling. Biochim Biophys Acta Mol Cell Biol Lipids. (2017) 1862:1110–20. doi: 10.1016/j.bbalip.2017.08.001
73. Jeong MY, Park J, Youn DH, Jung Y, Kang J, Lim S, et al. Albiflorin ameliorates obesity by inducing thermogenic genes via AMPK and PI3K/AKT in vivo and in vitro. Metabolism. (2017) 73:85–99. doi: 10.1016/j.metabol.2017.05.009
74. Kang NH, Mukherjee S, Min T, Kang SC, Yun JW. Trans-anethole ameliorates obesity via induction of browning in white adipocytes and activation of brown adipocytes. Biochimie. (2018) 151:1–13. doi: 10.1016/j.biochi.2018.05.009
75. Parray HA, Lone J, Park JP, Choi JW, Yun JW. Magnolol promotes thermogenesis and attenuates oxidative stress in 3T3-L1 adipocytes. Nutrition. (2018) 50:82–90. doi: 10.1016/j.nut.2018.01.017
76. Samuels JS, Shashidharamurthy R, Rayalam S. Novel anti-obesity effects of beer hops compound xanthohumol: role of AMPK signaling pathway. Nutr Metab. (2018) 15:42. doi: 10.1186/s12986-018-0277-8
77. Mi Y, Liu X, Tian H, Liu H, Li J, Qi G, et al. EGCG stimulates the recruitment of brite adipocytes, suppresses adipogenesis and counteracts TNF-alpha-triggered insulin resistance in adipocytes. Food Funct. (2018) 9:3374–86. doi: 10.1039/C8FO00167G
78. Choi M, Mukherjee S, Kang NH, Barkat JL, Parray HA, Yun JW. L-rhamnose induces browning in 3T3-L1 white adipocytes and activates HIB1B brown adipocytes. IUBMB Life. (2018) 70:563–73. doi: 10.1002/iub.1750
79. Rodriguez Lanzi C, Perdicaro DJ, Landa MS, Fontana A, Antoniolli A, Miatello RM, et al. Grape pomace extract induced beige cells in white adipose tissue from rats and in 3T3-L1 adipocytes. J Nutr Biochem. (2018) 56:224–33. doi: 10.1016/j.jnutbio.2018.03.001
80. Zhang F, Ai W, Hu X, Meng Y, Yuan C, Su H, et al. Phytol stimulates the browning of white adipocytes through the activation of AMP-activated protein kinase (AMPK) alpha in mice fed high-fat diet. Food Funct. (2018) 9:2043–50. doi: 10.1039/C7FO01817G
81. Zou T, Wang B, Yang Q, de Avila JM, Zhu MJ, You J, et al. Raspberry promotes brown and beige adipocyte development in mice fed high-fat diet through activation of AMP-activated protein kinase (AMPK) alpha1. J Nutr Biochem. (2018) 55:157–64. doi: 10.1016/j.jnutbio.2018.02.005
82. Lone J, Parray HA, Yun JW. Nobiletin induces brown adipocyte-like phenotype and ameliorates stress in 3T3-L1 adipocytes. Biochimie. (2018) 146:97–104. doi: 10.1016/j.biochi.2017.11.021
83. Imran KM, Yoon D, Kim YS. A pivotal role of AMPK signaling in medicarpin-mediated formation of brown and beige. Biofactors. (2018) 44:168–79. doi: 10.1002/biof.1392
84. Nagy L, Rauch B, Balla N, Ujlaki G, Kis G, Abdul-Rahman O, et al. Olaparib induces browning of in vitro cultures of human primary white adipocytes. Biochem Pharmacol. (2019) 167:76–85. doi: 10.1016/j.bcp.2019.06.022
85. Palacios-Gonzalez B, Vargas-Castillo A, Velazquez-Villegas LA, Vasquez-Reyes S, Lopez P, Noriega LG, et al. Genistein increases the thermogenic program of subcutaneous WAT and increases energy expenditure in mice. J Nutr Biochem. (2019) 68:59–68. doi: 10.1016/j.jnutbio.2019.03.012
86. Zhang T, Deng B, Zhang R, Qin X, Zhang J, Zhao J. Dietary sea buckthorn pomace induces beige adipocyte formation in inguinal white adipose tissue in lambs. Animals. (2019) 9:193. doi: 10.3390/ani9040193
87. Liu M, Zheng M, Cai D, Xie J, Jin Z, Liu H, et al. Zeaxanthin promotes mitochondrial biogenesis and adipocyte browning via AMPKalpha1 activation. Food Funct. (2019) 10:2221–33. doi: 10.1039/C8FO02527D
88. Kang NH, Mukherjee S, Yun JW. Trans-cinnamic acid stimulates white fat browning and activates brown adipocytes. Nutrients. (2019) 11:577. doi: 10.3390/nu11030577
89. Auger C, Knuth CM, Abdullahi A, Samadi O, Parousis A, Jeschke MG. Metformin prevents the pathological browning of subcutaneous white adipose tissue. Mol Metab. (2019) 29:12–23. doi: 10.1016/j.molmet.2019.08.011
90. Wang J, Zhang L, Dong L, Hu X, Feng F, Chen F. 6-gingerol, a functional polyphenol of ginger, promotes browning through an AMPK-dependent pathway in 3T3-L1 adipocytes. J Agric Food Chem. (2019) 67:14056–65. doi: 10.1021/acs.jafc.9b05072
91. Zou T, Wang B, Li S, Liu Y, You J. Dietary apple polyphenols promote fat browning in high-fat diet-induced obese mice through activation of adenosine monophosphate-activated protein kinase alpha. J Sci Food Agric. (2020) 100:2389–98. doi: 10.1002/jsfa.10248
92. Bhadada SV, Patel BM, Mehta AA, Goyal RK. beta(3) receptors: role in cardoimetabolic disorders. Ther Adv Endocrinol Metab. (2011) 2:65–79. doi: 10.1177/2042018810390259
93. Gong DW, He Y, Karas M, Reitman M. Uncoupling protein-3 is a mediator of thermogenesis regulated by thyroid hormone, beta3-adrenergic agonists, and leptin. J Biol Chem. (1997) 272:24129–32. doi: 10.1074/jbc.272.39.24129
94. Nedergaard J, Cannon B. The 'novel' 'uncoupling' proteins UCP2 and UCP3: what do they really do? Pros and cons for suggested functions. Exp Physiol. (2003) 88:65–84. doi: 10.1113/eph8802502
95. Ursino MG, Vasina V, Raschi E, Crema F, De Ponti F. The beta3-adrenoceptor as a therapeutic target: current perspectives. Pharmacol Res. (2009) 59:221–34. doi: 10.1016/j.phrs.2009.01.002
96. Vonesh E, Gooch KL, Khangulov V, Schermer CR, Johnston KM, Szabo SM, et al. Cardoivascular risk profile in individuals initiating treatment for overactive bladder - challenges and learnings for comparative analysis using linked claims and electronic medical record databases. PLoS ONE. (2018) 13:e0205640. doi: 10.1371/journal.pone.0205640
97. Mitcheson HD, Samanta S, Muldowney K, Pinto CA, Rocha BA, Green S, et al. Vibegron (RVT-901/MK-4618/KRP-114V) administered once daily as monotherapy or concomitantly with tolterodine in patients with an overactive bladder: a multicenter, phase IIb, randomized, double-blind, controlled trial. Eur Urol. (2019) 75:274–82. doi: 10.1016/j.eururo.2018.10.006
98. Keam SJ. Vibegron: first global approval. Drugs. (2018) 78:1835–9. doi: 10.1007/s40265-018-1006-3
99. Cypess AM, Weiner LS, Roberts-Toler C, Franquet Elia E, Kessler SH, Kahn PA, et al. Activation of human brown adipose tissue by a beta3-adrenergic receptor agonist. Cell Metab. (2015) 21:33–8. doi: 10.1016/j.cmet.2014.12.009
100. Baskin AS, Linderman JD, Brychta RJ, McGehee S, Anflick-Chames E, Cero C, et al. Regulation of human adipose tissue activation, gallbladder size, and bile acid metabolism by a beta3-adrenergic receptor agonist. Diabetes. (2018) 67:2113–25. doi: 10.2337/db18-0462
101. Arch JR. Challenges in beta(3)-adrenoceptor agonist drug development. Ther Adv Endocrinol Metab. (2011) 2:59–64. doi: 10.1177/2042018811398517
102. Malik M, van Gelderen EM, Lee JH, Kowalski DL, Yen M, Goldwater R, et al. Proarrhythmic safety of repeat doses of mirabegron in healthy subjects: a randomized, double-blind, placebo-, and active-controlled thorough QT study. Clin Pharmacol Ther. (2012) 92:696–706. doi: 10.1038/clpt.2012.181
103. Loh RKC, Formosa MF, La Gerche A, Reutens AT, Kingwell BA, Carey AL. Acute metabolic and cardoivascular effects of mirabegron in healthy individuals. Diabetes Obes Metab. (2019) 21:276–84. doi: 10.1111/dom.13516
104. Cheng HJ, Zhang ZS, Onishi K, Ukai T, Sane DC, Cheng CP. Upregulation of functional beta(3)-adrenergic receptor in the failing canine myocardium. Circ Res. (2001) 89:599–606. doi: 10.1161/hh1901.098042
105. Zhang ZS, Cheng HJ, Onishi K, Ohte N, Wannenburg T, Cheng CP. Enhanced inhibition of L-type Ca2+ current by beta3-adrenergic stimulation in failing rat heart. J Pharmacol Exp Ther. (2005) 315:1203–11. doi: 10.1124/jpet.105.089672
106. Zhou T, Yan X, Wang G, Liu H, Gan X, Zhang T, et al. Evolutionary pattern and regulation analysis to support why diversity functions existed within PPAR gene family members. Biomed Res Int. (2015) 2015:613910. doi: 10.1155/2015/613910
107. Diamant M, Heine RJ. Thiazolidinedoines in type 2 diabetes mellitus: current clinical evidence. Drugs. (2003) 63:1373–405. doi: 10.2165/00003495-200363130-00004
108. Nanjan MJ, Mohammed M, Prashantha Kumar BR, Chandrasekar MJN. Thiazolidinedoines as antidiabetic agents: a critical review. Bioorg Chem. (2018) 77:548–67. doi: 10.1016/j.bioorg.2018.02.009
109. Kung J, Henry RR. Thiazolidinedione safety. Expert Opin Drug Saf. (2012) 11:565–79. doi: 10.1517/14740338.2012.691963
110. Berhanu P, Perez A, Yu S. Effect of pioglitazone in combination with insulin therapy on glycaemic control, insulin dose requirement and lipid profile in patients with type 2 diabetes previously poorly controlled with combination therapy. Diabetes Obes Metab. (2007) 9:512–20. doi: 10.1111/j.1463-1326.2006.00633.x
111. Mattoo V, Eckland D, Widel M, Duran S, Fajardo C, Strand J, et al. Metabolic effects of pioglitazone in combination with insulin in patients with type 2 diabetes mellitus whose disease is not adequately controlled with insulin therapy: results of a six-month, randomized, double-blind, prospective, multicenter, parallel-group study. Clin Ther. (2005) 27:554–67. doi: 10.1016/j.clinthera.2005.05.005
112. Ammazzalorso A, Maccallini C, Amoia P, Amoroso R. Multitarget PPARgamma agonists as innovative modulators of the metabolic syndrome. Eur J Med Chem. (2019) 173:261–73. doi: 10.1016/j.ejmech.2019.04.030
113. Nedergaard J, Cannon B. UCP1 mRNA does not produce heat. Biochim Biophys Acta. (2013) 1831:943–9. doi: 10.1016/j.bbalip.2013.01.009
114. Meyer CW, Willershauser M, Jastroch M, Rourke BC, Fromme T, Oelkrug R, et al. Adaptive thermogenesis and thermal conductance in wild-type and UCP1-KO mice. Am J Physiol Regul Integr Comp Physiol. (2010) 299:R1396–406. doi: 10.1152/ajpregu.00021.2009
115. Ukropec J, Anunciado RP, Ravussin Y, Hulver MW, Kozak LP. UCP1-independent thermogenesis in white adipose tissue of cold-acclimated Ucp1-/- mice. J Biol Chem. (2006) 281:31894–908. doi: 10.1074/jbc.M606114200
116. Gnad T, Scheibler S, von Kugelgen I, Scheele C, Kilic A, Glode A, et al. Adenosine activates brown adipose tissue and recruits beige adipocytes via A2A receptors. Nature. (2014) 516:395–9. doi: 10.1038/nature13816
117. Armani A, Cinti F, Marzolla V, Morgan J, Cranston GA, Antelmi A, et al. Mineralocorticoid receptor antagonism induces browning of white adipose tissue through impairment of autophagy and prevents adipocyte dysfunction in high-fat-diet-fed mice. FASEB J. (2014) 28:3745–57. doi: 10.1096/fj.13-245415
118. Thuzar M, Law WP, Dimeski G, Stowasser M, Ho KKY. Mineralocorticoid antagonism enhances brown adipose tissue function in humans: a randomized placebo-controlled cross-over study. Diabetes Obes Metab. (2019) 21:509–16. doi: 10.1111/dom.13539
119. Zeng X, Ye M, Resch JM, Jedrychowski MP, Hu B, Lowell BB, et al. Innervation of thermogenic adipose tissue via a calsyntenin 3beta-S100b axis. Nature. (2019) 569:229–35. doi: 10.1038/s41586-019-1156-9
120. Kazak L, Chouchani ET, Jedrychowski MP, Erickson BK, Shinoda K, Cohen P, et al. A creatine-driven substrate cycle enhances energy expenditure and thermogenesis in beige fat. Cell. (2015) 163:643–55. doi: 10.1016/j.cell.2015.09.035
Keywords: obesity, brown fat, beige fat, thermogenesis, β-adrenergic signaling, UCP1, calcium cycling, glycolytic beige fat
Citation: Pan R, Zhu X, Maretich P and Chen Y (2020) Combating Obesity With Thermogenic Fat: Current Challenges and Advancements. Front. Endocrinol. 11:185. doi: 10.3389/fendo.2020.00185
Received: 02 December 2019; Accepted: 16 March 2020;
Published: 15 April 2020.
Edited by:
Takeshi Yoneshiro, University of California, San Francisco, United StatesReviewed by:
Alessandra Feraco, San Raffaele Pisana (IRCCS), ItalyKerry Loomes, The University of Auckland, New Zealand
Copyright © 2020 Pan, Zhu, Maretich and Chen. This is an open-access article distributed under the terms of the Creative Commons Attribution License (CC BY). The use, distribution or reproduction in other forums is permitted, provided the original author(s) and the copyright owner(s) are credited and that the original publication in this journal is cited, in accordance with accepted academic practice. No use, distribution or reproduction is permitted which does not comply with these terms.
*Correspondence: Yong Chen, dGoueS5jaGVuQHZpcC4xNjMuY29t