- 1Department of Zoology, Stockholm University, Stockholm, Sweden
- 2Department of Ecology and Evolutionary Biology, Brown University, Providence, RI, United States
- 3Institut de Neurosciences Cognitives et Intégratives d'Aquitaine (CNRS UMR5287), University of Bordeaux, Pessac, France
The insulin/IGF-signaling pathway is central in control of nutrient-dependent growth during development, and in adult physiology and longevity. Eight insulin-like peptides (DILP1–8) have been identified in Drosophila, and several of these are known to regulate growth, metabolism, reproduction, stress responses, and lifespan. However, the functional role of DILP1 is far from understood. Previous work has shown that dilp1/DILP1 is transiently expressed mainly during the pupal stage and the first days of adult life. Here, we study the role of dilp1 in the pupa, as well as in the first week of adult life, and make some comparisons to dilp6 that displays a similar pupal expression profile, but is expressed in fat body rather than brain neurosecretory cells. We show that mutation of dilp1 diminishes organismal weight during pupal development, whereas overexpression increases it, similar to dilp6 manipulations. No growth effects of dilp1 or dilp6 manipulations were detected during larval development. We next show that dilp1 and dilp6 increase metabolic rate in the late pupa and promote lipids as the primary source of catabolic energy. Effects of dilp1 manipulations can also be seen in the adult fly. In newly eclosed female flies, survival during starvation is strongly diminished in dilp1 mutants, but not in dilp2 and dilp1/dilp2 mutants, whereas in older flies, only the double mutants display reduced starvation resistance. Starvation resistance is not affected in male dilp1 mutant flies, suggesting a sex dimorphism in dilp1 function. Overexpression of dilp1 also decreases survival during starvation in female flies and increases egg laying and decreases egg to pupal viability. In conclusion, dilp1 and dilp6 overexpression promotes metabolism and growth of adult tissues during the pupal stage, likely by utilization of stored lipids. Some of the effects of the dilp1 manipulations may carry over from the pupa to affect physiology in young adults, but our data also suggest that dilp1 signaling is important in metabolism and stress resistance in the adult stage.
Introduction
The insulin/IGF signaling (IIS) pathway plays a central role in nutrient-dependent growth control during development, as well as in adult physiology and aging (1–5). More specifically, in mammals, insulin, IGFs, and relaxins act on different types of receptors to regulate metabolism, growth, and reproduction (6–9). This class of peptide hormones has been well conserved over evolution and therefore the genetically tractable fly Drosophila is an attractive model system for investigating IIS mechanisms (1, 10, 11). Eight insulin-like peptides (DILP1–8), each encoded on a separate gene, have been identified in Drosophila (11–14). The genes encoding these DILPs display differential temporal and tissue-specific expression profiles, suggesting that they have different functions (12, 13, 15–17). Specifically, DILP1, 2, 3, and 5 are mainly expressed in median neurosecretory cells located in the dorsal midline of the brain, designated insulin-producing cells (IPCs) (12, 17–20). The IPC-derived DILPs can be released into the open circulation from axon terminations in the corpora cardiaca, the anterior aorta, the foregut, and the crop. Genetic ablation of the IPCs reduces growth and alters metabolism, and results in increased resistance to several forms of stress and prolongs lifespan (19, 21).
The functions of the individual DILPs produced by the IPCs may vary depending on the stage of the Drosophila life cycle. Already, the temporal expression patterns hint that DILP1–3 and 5 play different roles during development. Thus, whereas DILP2 and 5 are relatively highly expressed during larval and adult stages, DILP1 and 6 are almost exclusively expressed during pupal stages under normal conditions (16, 22).
DILP1 is unique among the IPC-produced peptides since it can be detected primarily during the pupal stage (a non-feeding stage) and the first few days of adult life when residual larval/pupal fat body is present (16, 17). Furthermore, in female flies kept in adult reproductive diapause, where feeding is strongly reduced, dilp1/DILP1 expression is also high (17). The temporal expression profile of dilp1/DILP1 resembles that of dilp6/DILP6 although the latter peptide is primarily produced by the fat body, not IPCs (16, 22). Since DILP6 was shown to regulate growth of adult tissues during pupal development (16, 22), we asked whether also DILP1 plays a role in growth control. It is known that overexpression of several of the DILPs is sufficient to increase body growth through an increase in cell size and cell number, and especially DILP2 produces a substantial increase in body weight (12, 23, 24). In contrast, not all single dilp mutants display a decreased body mass. The dilp1, dilp2, and dilp6 single mutants display slightly decreased body weight (11, 16, 22), whereas the dilp3, dilp4, dilp5, and dilp7 single mutants display normal body weight (11). However, a triple mutation of dilp2, 3, and 5 causes a drastically reduced body weight, and a dilp1–4,5 mutation results in a further reduction (11, 25). Note that several of the above studies do not show bona fide effects on cell or organismal growth (e.g., volume or cell numbers/sizes); they only provide body mass data.
There is a distinction between how DILPs act in growth regulation. DILPs other than DILP1 and DILP6 promote growth primarily during the larval stages (both feeding and wandering stages) when their expression is high (12, 23). This nutrient-dependent growth is relatively well-understood and is critical for production of the steroid hormone ecdysone and thereby developmental timing and induction of developmental transitions such as larval molts and pupariation (26–30). The growth in the pupal stage, which primarily affects imaginal discs and therefore adult tissues, is far less studied [see Slaidina et al. (16) and Okamoto et al. (31)]. In this study, we investigate the role of dilp1/DILP1 in growth regulation in Drosophila in comparison to dilp6/DILP6. For this, we determine both bona fide size of body and/or wings and provide wet weights, and thus can distinguish between growth and increase of body mass. We found that mutation of dilp1 diminishes body weight (but not body size), whereas ectopic dilp1 expression promotes organismal growth by increasing both weight and size during the pupal stage, similar to dilp6. Thus, we cannot unequivocally show a role of dilp1 in organismal growth, but it does regulate body mass, suggesting that dilp1 affects metabolism and energy stores. Determination of metabolic rate (MR) and respiratory quotient (RQ) as well as triacylglyceride (TAG) levels during late pupal development provides evidence that dilp1 and dilp6 increase the MR and that the associated increased metabolic cost is fueled by increased lipid catabolism.
Since dilp1/DILP1 levels are high the first week of adult life, we also investigated the role of dilp1 mutation and overexpression on early adult physiology, including metabolism stress resistance and fecundity. Interestingly, the newly eclosed dilp1 mutant flies are less resistant to starvation than controls and dilp2 mutants. Thus, dilp1 acts differently from other dilps for which it has been shown that reduced signaling increases survival during starvation (21). Also, early egg laying and female fecundity are affected by dilp1 overexpression, and in general, dilp1 manipulations produce more prominent effects in female flies.
Taken together, our data suggest that ectopic expression of dilp1/DILP1 promotes growth of adult tissues during the pupal stage, and that this process mainly utilizes stored lipids to fuel the increased MR. The DILP1 signaling also affects the metabolism in the young adult fly, and we see sex dimorphic effects of altered signaling on stress responses and fecundity.
Methods
Fly Lines and Husbandry
Parental flies were reared and maintained at 18°C with 12:12 light:dark cycle on food based on a recipe from Bloomington Drosophila Stock Center (BDSC) (https://bdsc.indiana.edu/information/recipes/bloomfood.html). The experimental flies were reared and maintained at 25°C, with 12:12 light:dark cycle on an agar-based diet with 10% sugar and 5% dry yeast.
The following Gal4 lines were used in this study: dilp2-Gal4 [(19) from E. Rulifson, Stanford, CA], ppl-Gal4 [(32) from M. J. Pankratz, Bonn, Germany], To-Gal4 [(33) from B. Dauwalder, Houston, TX], c929-Gal4 [(34) from Paul H. Taghert], yw; UAS-dilp6, and yw; UAS-dilp2;+ [(23) from H. Stocker, Zürich, Switzerland]. Several UAS-dilp1 lines were produced for a previous study (35), and two of them, UAS-dilp1 (II) and UAS-dilp1 (III), were used here. UAS-dilp1-RNAi flies were from Vienna Drosophila Resource Center (VDRC), Vienna, Austria. As controls, we used w1118 or yw obtained from BDSC, crossed to Gal4 and UAS lines. All flies (except yw; UAS-dilp6, and yw; UAS-dilp2; +) were backcrossed to w1118 for at least 6 generations.
We used a double null mutation of dilp1/dilp2 that was previously generated by homologous recombination and verified as described by Post et al. (35). Also, single dilp1 and dilp2 null mutants were employed. We refer to these three null mutants as dilp1, dilp2, and dilp1/dilp2 mutants for simplicity. As described earlier (35), these were obtained from BDSC and a residual w+ marker was Cre excised followed by chromosomal exchange to remove yw markers on chromosomes 2 and X.
To generate a recombinant dilp6;;dilp1 double mutant, the dilp1 and dilp668 mutants (11) were used for crossing with a double balancer fly, 4E10D/FM7,dfd;;Vno/TM3,dfd, obtained from Dr. Vasilios Tsarouhas (Stockholm University). The efficiency of the dilp6;;dilp1 double mutant was validated by qPCR.
Antisera and Immunocytochemistry
For immunolabeling, tissues from larvae or female adults were dissected in chilled 0.1 M phosphate buffered saline (PBS). They were then fixed for 4 h in ice-cold 4% paraformaldehyde (PFA) in PBS, and subsequently rinsed in PBS three times for 1 h. Incubation with primary antiserum was performed for 48 h at 4°C with gentle agitation. After rinse in PBS with 0.25% Triton-X 100 (PBS-Tx) four times, the tissues were incubated with secondary antibody for 48 h at 4°C. After a thorough wash in PBS-Tx, tissues were mounted in 80% glycerol with 0.1 M PBS.
The following primary antisera were used: Rabbit or guinea pig antiserum to part of the C-peptide of DILP1 diluted 1:10,000 (17). Rabbit antisera to A-chains of DILP2 and DILP3 (36) and part of the C-peptide of DILP5 (37) all at a dilution of 1:2,000, mouse anti-green fluorescent protein (GFP) at 1:000 (RRID: AB_221568, Invitrogen, Carlsbad, CA). The following secondary antisera were used: goat anti-rabbit Alexa 546, goat anti-rabbit Alexa 488, and goat anti-mouse Alexa 488 (all from Invitrogen). Cy3-tagged goat anti-guinea pig antiserum (Jackson ImmunoResearch, West Grove, PA). All were used at a dilution of 1:1,000.
Image Analysis
Images were captured with a Zeiss LSM 780 confocal microscope (Jena, Germany) using 10 ×, 20 ×, and 40 × oil immersion objectives. The projections of z-stacks were processed using Fiji (https://imagej.nih.gov/ij/). The cell body outlines were extracted manually and the staining intensity was determined using ImageJ (https://imagej.nih.gov/ij/). The background intensity for all samples was recorded by randomly selecting three small regions near the cell body of interest. The final intensity value of the cell bodies was determined by subtracting the background intensity.
Images of pupae, adult flies, and fly wings were captured with a Leica EZ4HD light microscope (Wetzlar, Germany). The size of the adult fly body and wings was determined using Fiji. The pupal volume (v) was calculated using the equation v = 4/3 π (L/2) × (l/2)2, in which L = length and l = width (38). Thorax length was measured from the posterior tip of the scutellum to the base of the most anterior point of the humeral bristle.
Pupariation Time, Egg to Pupae Viability, and Adult Body Weight
To determine time to pupariation, 6- to 7-day-old adult females were crossed in the evening. The following morning, adult flies were transferred to vials with fresh food on which they were allowed to lay eggs for 4 h. Two hours after the initiation of egg laying was considered time “0,” and thereafter, the number of pupae was monitored at 6- or 12-h intervals. To investigate the viability of egg to pupae formation, one pair of 6- to 7-day-old adult flies was allowed to lay eggs for 24 h, after which the total number of eggs was counted. Subsequently, the total number of pupae was counted and the viability of egg to pupae was determined as pupa number/egg number × 100%. The body weight (wet weight) of single adult flies was determined using a Mettler Toledo MT5 microbalance (Columbus, USA). The number of eggs of stage 10–14 in ovaries was counted in 3-day-old flies.
Starvation and Desiccation Survival Assay
Newly eclosed and mated 6- to 7-day-old adults were used for starvation and desiccation resistance experiments. For newly eclosed flies, we collected virgin flies every 4 h, to be used for starvation experiments. The flies were kept in vials containing 5 ml of 0.5% aqueous agarose (A2929, Sigma-Aldrich) for starvation and empty vials for desiccation. The number of dead flies was counted at least every 12 h until all the flies were dead. At least 110 flies from three replicates were used for the analysis.
Capillary Feeding (CAFE) Assay
Food intake was measured using a slightly modified CAFE assay following Ja et al. (39). In brief, female flies were placed into 1.5-ml Eppendorf microcentrifuge tubes with an inserted capillary tube (5 μl, Sigma-Aldrich) containing 5% sucrose, 2% yeast extract, and 0.1% propionic acid. To estimate evaporation, three food-filled capillaries were inserted in identical tubes without flies. The final food intake was determined by calculating the decrease in food level minus the average decrease in the three control capillaries. Food consumption was measured daily and calculated cumulatively over 4 consecutive days. For this assay, we used 8–10 flies in each of three biological replicates.
Quantitative Real-Time PCR (qPCR)
Total RNA was extracted from whole bodies of middle or late pupal stages of Drosophila by using Trizol-chloroform (Sigma-Aldrich). Quality and concentration of the RNA were determined with a NanoDrop 2000 spectrophotometer (Thermo Scientific). The concentration of the RNA was adjusted to 400 ng/μl. A total of 2 μg RNA was used for cDNA synthesis. The cDNA syntheses were performed by using random hexamer primer (Thermo Scientific) and RevertAid reverse transcriptase (Thermo Scientific). The cDNA products were then diluted 10 times and applied for qPCR using a StepOnePlusTM instrument (Applied Biosystems, USA) and SensiFAST SYBR Hi-ROX Kit (Bioline) following the protocol from the manufacturer. The mRNA abundance was normalized to ribosomal protein (rp49) levels in the same samples. Relative expression values were determined by the 2−ΔΔCT method (40). The sequences of primers used for qPCR were those used previously (17, 35, 41):
dilp1 F: CGGAAACCACAAACTCTGCG
dilp1 R:CCCAGCAAGCTTTCACGTTT
dilp2 F: AGCAAGCCTTTGTCCTTCATCTC
dilp2 R: ACACCATACTCAGCACCTCGTTG
dilp3 F: TGTGTGTATGGCTTCAACGCAATG
dilp3 R: CACTCAACAGTCTTTCCAGCAGGG
dilp6 F: CCCTTGGCGATGTATTTCCCAACA
dilp6 R: CCGACTTGCAGCACAAATCGGTTA
rp49 F: ATCGGTTACGGATCGAACAA
rp49 R: GACAATCTCCTTGCGCTTCT.
Metabolite Quantification
Glycogen and TAG levels were assayed as previously described (35, 42, 43). For glycogen assays, 5–6 adult female flies per sample were homogenized in PBS and quantified using the Infinity Glucose Hexokinase reagent by spectrophotometry. For TAG assays, 5–6 adult female flies per sample were homogenized in PBS + 0.05% TBS-T and quantified using the Infinity Triglycerides reagent by spectrophotometry. The fly lysate protein levels were determined by BCA assay (Thermo Fisher) and metabolite levels were normalized to protein level.
To measure the amount of TAG during late pupal stages, 6 replicates with 4 pupae in each were collected and then homogenized in PBS + 0.05% Triton X-100 with a tissuelyser II from Qiagen. The TAG levels were determined with a Liquick Cor-TG diagnostic kit (Cormay, Poland) using a linear regression coefficient from a standard curve made with 2.2 μg/μl TAG standard (Cormay, Poland). Absorbance of samples was measured at 550 nm with a micro-plate reader (Thermo scientific). Data are expressed as micrograms of TAG related to protein levels. Protein levels were determined using a Bradford assay according to Diop et al. (44).
Dynamic Injection Respirometry
Carbon dioxide (CO2) production and oxygen (O2) consumption of individual pupae of both sexes were measured during pupal development at 25°C to assess MR as described previously (45). Pupae were placed in 1-ml syringes (i.e., respirometry chambers) that were filled with air scrubbed of CO2 with ascarite (Acros Organics, USA) that then passed through filtered acidified water (pH <4.5, checked weekly), closed with three-way luer valves, and kept for roughly 24 h at 25°C with 12:12 light:dark cycle. An empty syringe served as control. CO2 production was measured using a Sable Systems (Las Vegas, NV, USA) differential respirometry setup. Two independent lines of outdoor air scrubbed of H2O and CO2, using drierite (WA Hammond Drierite, USA) and ascarite scrubbers, respectively, were pushed at a steady rate of 150 ml min−1 using as SS-4 pump (Sable Systems) and two separate mass flow controllers (840 Series; Sierra Instruments Inc., California, USA). The syringes containing pupae were placed after the mass valve controllers in the first line (sample) and 0.45 ml pushed into the airflow. The push rate was recorded through a second flow meter downstream of the syringe and approximated a flow rate of 162 ml min−1 downstream of the syringe. The line was then scrubbed of H2O with magnesium perchlorate (Sigma-Aldrich) and entered the sample line of a Li-7000 CO2 analyzer (LiCor, Lincoln, NE, USA). The second line (reference) proceeded the same way, mimicking the exact length of the sample line (including an empty measurement chamber), entering the reference line of the CO2 analyzer. The lines then proceeded through a second set of ascarite CO2 scrubbers and entered an Oxzilla FC-2 O2 analyzer (Sable Systems), after which air was ejected. Preliminary measurements were performed to ensure stability of flow rate through either channel by measuring the flow rate of air ejected from the O2 analyzer. After the measurement, pupae were weighed using a Mettler Toledo MT5 microbalance (Columbus, USA) and left at 25°C with 12:12 light:dark cycle until adult eclosion, at which point they were sexed.
Differential CO2 and O2 were calculated by subtracting the output of the reference line from the output of the sample line. For all measurements, sampling rate was 1 Hz. In the program Expedata (version 1.9.10), the raw output was baseline corrected against the reference line value, fractioned and multiplied with flow rate to yield CO2 and O2 in ml min−1 (46). The values were then corrected by subtracting the readings from the empty control syringe from the sample values. MR was calculated by first integrating the fractioned CO2 and O2 (ml min−1) values against time to yield CO2 and O2 in ml produced while pupae were in the syringes. Next, VCO2, and VO2 were corrected by accounting for the fraction of air that was still left in the syringe and the time spent in the syringe using the formula (only calculation for VCO2 is shown) VCO2 = (CO2 × (0.6/0.45))/hours in syringe (46). Then, the RQ was calculated as RQ = VCO2/VO2. RQ values provide an estimate on what energy source is being catabolized to fuel metabolism (47). MR (in watts = joules s−1) was converted from VO2 using the formula MR = (VO2 × (16 + (5.164 × RQ)))/(60 ×60) (46) and finally divided by body weight in mg to yield MR mg−1.
In the present study, we monitored single identified individuals throughout pupal development, and sexed them after eclosion. For the vast majority, eclosion was successful and therefore we could use the true weight of the individual for the calculation above. However, for individuals that failed to eclose properly, we instead used the average weight for that sex and treatment to calculate MR.
Statistical Analysis
All results are presented as means ± SEM. We first investigated normality of data using Shapiro–Wilk's normality test and then used one-way analysis of variance (ANOVA) or Student's t-test, followed by Tukey's multiple comparisons test. Lifespan data were subjected to survival analysis (Log rank tests with Mantel-Cox post-test) and presented as survival curves.
For the respirometry data, we used the natural logarithm of MR mg−1 due to deviations from normality. A factorial two-way ANOVA was used with MR mg−1 or RQ as dependent variable, and sex and treatment as factorial explanatory variables. Non-significant interactions and main effects were removed from final models (48). The respirometry data were analyzed with the IBM SPSS statistics 23.0 (IBM SPSS Inc., Chicago, IL, USA) statistical software package. Prism GraphPad version 6.00 (La Jolla, CA, USA) was used for generating all the graphs.
Results
Mutation of dilp1 Decreases Body Weight
It was previously reported that decreased dilp1 activity reduces adult body weight in Drosophila, but it was not investigated at what developmental stage this occurred or whether the weight decrease was caused by diminished organismal growth (11, 20). This is relevant to ask since dilp1 displays a restricted temporal expression during the Drosophila life cycle (see Figure 1A) and the body mass can increase without cellular/organismal growth. To analyze growth and other effects of dilp1 and possible interactions with its tandem-encoded paralog dilp2, we employed recently generated dilp1, dilp2, and double dilp1-dilp2 null mutants (35). The efficacy of these mutants was confirmed by qPCR in stage 8–9 pupae (about 50% pupal development) and immunolabeling in 1-week-old mated female flies (Supplementary Figure 1). We also asked whether the dilp1 mutants displayed compensatory changes of other dilps in the IPCs or fat body. In dilp1 mutant pupae (stage 8–9), the mRNA levels of dilp2, dilp3, and dilp6 were not altered, but in dilp6 mutants, the dilp1 level was upregulated (Supplementary Figures 1A–C). These findings suggest that only minor (or no) compensatory changes in transcripts of other dilps in dilp1 mutants occur during the mid-pupal stage (later pupal stages were not tested). In 1-week-old female flies, however, immunocytochemistry shows that at the protein level DILP2, but not DILP3, immunofluorescence increased in dilp1 mutants (Supplementary Figures 1D–G). An earlier study showed upregulation also of dilp2 transcript in flies of the same age (35). It should also be noted that the relative expression of dilp1 is 100-fold lower than that of dilp2 (35). Taken together, this suggests that dilp2/DILP2 could provide some compensation for lack of dilp1 at least in young adult flies.
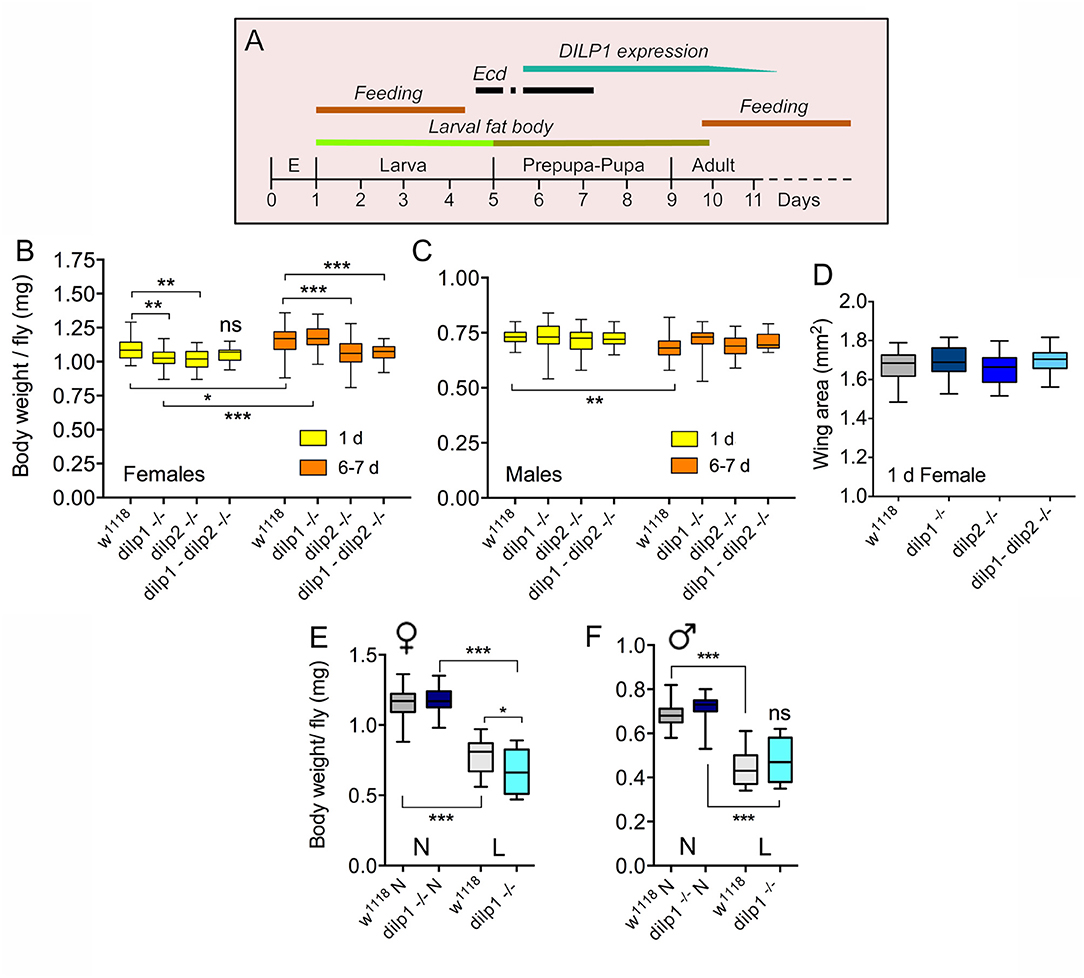
Figure 1. dilp1 mutant flies display reduced body weight, but are not smaller. (A) Expression profile of dilp1/DILP1 in Drosophila. Note that expression of transcript and peptide coincides with the non-feeding pupal stage and the first days of adult life when food intake is reduced (especially day one). It also times with the onset of the second and third ecdysone (Ecd) surges in the early pupa (earlier ecdysone peaks are not shown). E, embryo. (B) Body weight of female flies 1 day and 6–7 days after adult eclosion. dilp1 mutant flies display reduced body weight when 1 day old, but gain substantially the first week. Also, dilp2 mutants weigh less, but do not gain much weight the first week. The double mutants are not significantly affected compared to controls at 1 day, but after 6–7 days, both dilp2 and double mutants weigh less that controls and dilp1 mutants. Data are presented as medians ± range, n = 25–30 flies for each genotype from three independent replicates (*p < 0.05, **p < 0.01, ***p < 0.001, ns, not significant; two-way ANOVA followed by Tukey's test). (C) In male flies, the three mutants display weights similar to controls and controls lose weight the first week. Data are presented as medians ± range, n = 18–30 flies for each genotype from three independent replicates (**p < 0.01, two-way ANOVA followed with Tukey's test). (D) Wing area was used as a proxy for organismal growth. The three mutants did not display altered wing size. Data are presented as medians ± range, n = 16–23 flies for each genotype from three independent replicates (one-way ANOVA followed with Tukey's test). (E,F) Body weight of 7-day-old flies that had been exposed to normal diet (N) or low protein diet (L) during late larval stage. The female dilp1 mutant flies displayed lower body weight than controls after low protein. Data are presented as medians ± range, n = 17–29 flies for each genotype from three replicates (*p < 0.05, ***p < 0.001, one-way ANOVA followed by Tukey's test).
To determine a possible role of dilp1 and dilp2 in organismal growth during development, we initially monitored the body weight (wet weight) of dilp1, dilp2, and dilp1/dilp2 double mutants. First, we measured the body weight in both recently eclosed and, for comparison, 6- to 7-day-old adult mated dilp1 mutant flies. In female flies, the newly eclosed dilp1 mutants displayed a decrease in body weight compared to controls (Figure 1B). However, this difference in body weight was no longer detectable in 6- to 7-day-old mated flies kept under normal feeding conditions; a significant weight increase was observed in both controls (w1118) and dilp1 mutant flies, but not in dilp2 and double mutants (Figure 1B). Also, dilp2 mutant female flies have significantly lower body weight than controls 1 day after emergence, but in contrast to dilp1 mutants, they did not increase the weight over 6–7 days of feeding (Figure 1B), possibly indicating that dilp2 affects egg development. We will get back to these effects on “older” flies in a later section. Interestingly, the weight of dilp1/dilp2 double mutants was not significantly affected compared to the single mutants (and control) and no weight increase was seen the first week, except in control flies (Figure 1B). Thus, there was no additive effect of the two mutations in females. In male flies, none of the mutant flies displayed altered body weight (Figure 1C). The effects of different genotypes on flyweight are shown in Table 1.
To determine whether decreased organismal growth was responsible for the lower body weight, we measured wing size in 1-day-old female mutant flies and found no significant difference to controls (Figure 1D). Thus, the decreased weight of the flies does not seem to reflect a significant decrease in organismal size. We cannot exclude that the lack of a growth phenotype in the dilp1 mutants is caused by compensatory action of other DILPs. It was shown in a previous study that 1-week-old dilp1 mutant flies display a 2-fold increased expression of dilp6 transcript (17) that might compensate for the loss of dilp1.
What is the role of dilp1 during pupal development? In a study of dilp6, it was shown that if third instar larvae (after reaching critical size) were put on a low-protein diet, they emerged as adults with lower body mass (wet weight) and that this was accentuated in dilp6 mutants (16). This suggests that dilp6 is important for metabolism and to assure growth of adult tissues under low protein conditions. We, thus, performed a similar experiment with dilp1 mutant larvae kept on normal food or low protein diet. Flies emerging from larvae on restricted protein indeed displayed significantly lower body weight and female dilp1 mutants weighed less than controls under protein starvation (Figure 1E). In male flies, this latter effect was not seen in the mutants (Figure 1F). This sex difference might indicate that part of the female weight loss is caused by diminished egg or ovary development.
We then asked whether mutation of both dilp1 and dilp6 would result in a further decrease of body weight and generated a recombinant dilp1/dilp6 mutant. Using qPCR, we found that these flies displayed virtually no detectable dilp1 and dilp6 RNA (Supplementary Figure 2A). The weights of dilp1/dilp6 mutants were significantly reduced compared to controls (Supplementary Figure 2B). However, their weights were not diminished more than those of the single dilp1 and dilp6 mutants, suggesting that there was no additive effect caused by loss of both dilps.
Overexpression of dilp1 Promotes Organismal Growth
Having shown effects of the dilp1 null mutation on adult fly weight, we next explored the outcome of overexpressing dilp1, either in IPCs, or more broadly, in fat body, or using a neuroendocrine cell Gal4 driver, c929. The fat body expression represents fully ectopic dilp1 expression (gain of function) since we could not detect dilp1/DILP1 in the fat body at any stage in wild-type flies; we rely here on the capacity of the fat body to produce and release DILP1 similar to DILP6. For the overexpression, we used several UAS-dilp1 lines [see Post et al. (35)]. These UAS-dilp1 lines were verified by DILP1 immunolabeling after expression with several Gal4 drivers (Supplementary Figures 3A–D) and by qPCR in stage 8–9 pupae (Supplementary Figures 4A–F). Overexpression of dilp1 in the fat body, using the fat body-specific pumpless (ppl) and takeout (to) Gal4 drivers, and in IPCs (dilp2-Gal4) results in a drastic upregulation of dilp1 RNA (Supplementary Figures 4A,D), but has no effect on dilp2 and dilp6 expression (Supplementary Figures 4B,C,E,F), except a minor decrease in dilp2 for ppl-Gal4 (Supplementary Figure 4B). At the protein level, dilp1 overexpression resulted in upregulation of DILP2 and DILP5 immunolevels in IPCs and a minor downregulation of DILP3 of 1-week-old adult female flies (Supplementary Figures 5A–E). One line, UAS-dilp1 (III), was selected for subsequent experiments since it generated the strongest DILP1 immunolabeling.
To determine whether manipulations of dilp1 affect the body mass during pupal development, we monitored wet weights of flies on the first day after eclosion (1-day-old flies). First, we used a dilp2-Gal4 driver to express dilp1 in the IPCs and detected no significant increase in body weight of female flies (Supplementary Figure 2A; Table 1), but a slight increase in males (Supplementary Figure 2B; Table 1). We next expressed dilp1 in the fat body, the insect functional analog of the liver and white adipocytes in mammals, and the source of secreted DILP6 (16, 31, 49–51). The fat body displays nutrient sensing capacity and is an important tissue for regulation of growth and metabolism in Drosophila either by secreted DILP6 or via other factors acting on IPCs to affect DILP secretion (16, 31, 51–55). To investigate the effect of ectopic dilp1 expression in the fat body, we used the ppl and to Gal4 drivers. The efficiency of the drivers was confirmed by DILP1 immunostaining of larval fat body of ppl>dilp1 and to>dilp1 flies, but not in the control flies (Supplementary Figure 3D). In ppl>dilp1 flies, we also found DILP1 labeling in the nephrocytes (not shown), which are highly endocytotic cells located close to the heart (56). The immunoreactive DILP1 is likely to have accumulated from the circulation after release from the fat body since the ppl-Gal4 is not expressed in the nephrocytes.
We found that ectopic expression of dilp1 in the fat body (ppl>dilp1), but not in IPCs (dilp2>dilp1), increased the body weight of females (Figure 2A; Table 1) as well as males (Supplementary Figure 6C; Table 1). We suggest that dilp1 overexpression in IPCs, which already express the peptide, does not necessarily lead to increased release of DILP1; the amount of release is likely to be tightly controlled and is not affected by the size of the stored pool of peptide. In contrast, knockdown of dilp1 in IPCs (dilp2>dilp1-RNAi), leads to a decrease in body weight (Figure 2A).
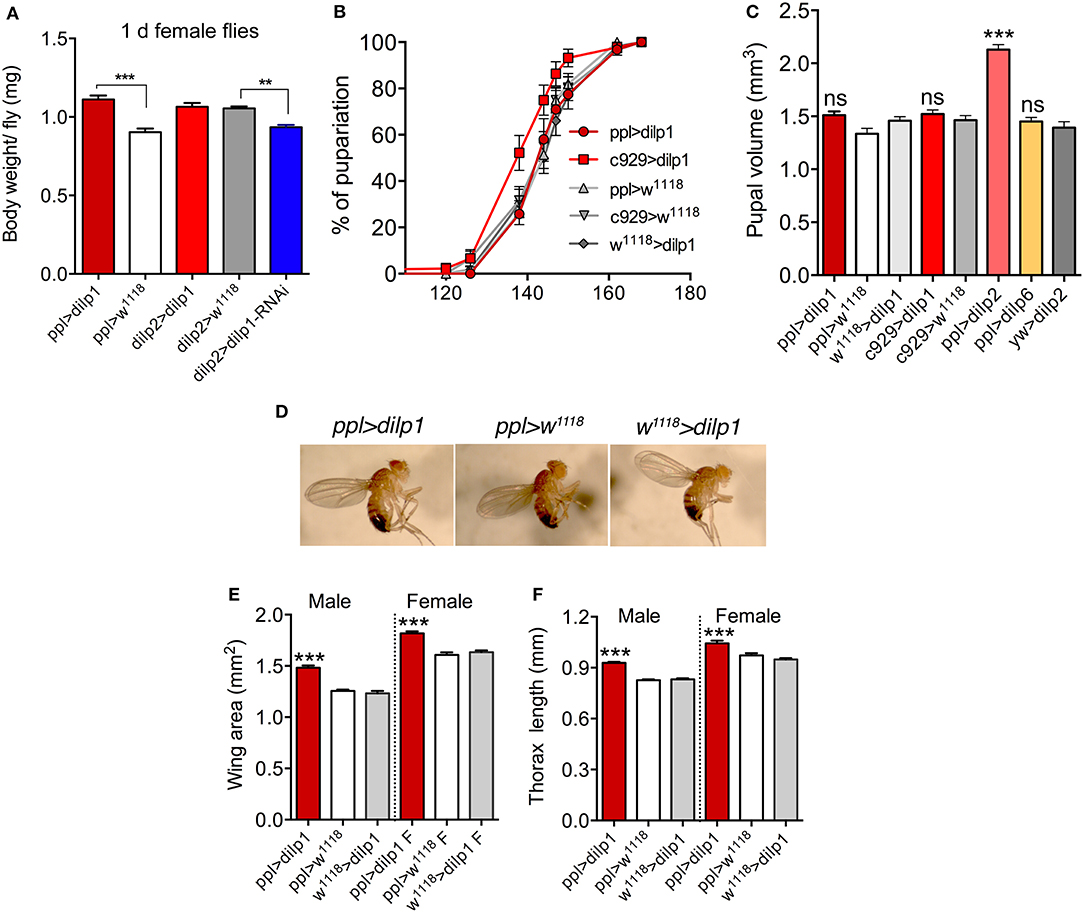
Figure 2. Overexpression of dilp1 affects growth during pupal stage. (A) One-day-old female flies weigh more than controls in ppl>dilp1 flies, but not in dilp2>dilp1. Knockdown of dilp1 by dilp2>dilp1-RNAi leads to decreased body weight. Data are presented as means ± S.E.M, n = 20–27 flies for each genotype from three independent replicates (**p < 0.01, ***p < 0.001, unpaired Student's t-test). (B) Overexpression of dilp1 in fat body (ppl-Gal4) or neuroendocrine cells (c929-Gal4) does not affect time to pupariation (larval development). Data are presented as means ± S.E.M, n = 138–147 flies for each genotype from three independent replicates [assessed by Log-rank (Mantel-Cox) test]. (C) Overexpression of dilp1 using ppl-Gal4 or c929-Gal4 does not affect pupal volume (proxy for larval growth). Also dilp6 overexpression has no effect, whereas dilp2 expression triggers a significant increase in pupal volume. Data are presented as means ± S.E.M, n = 15–32 flies for each genotype from three independent replicates (***p < 0.001, ns, not significant; one-way ANOVA followed with Tukey's test). (D) Images of flies overexpressing dilp1 in the fat body and controls. (E,F) Overexpression of dilp1 in fat body results in flies with increased wing area (E), and length of thorax (F) as proxies for organismal growth. Data are presented as means ± S.E.M (***p < 0.001, one way ANOVA followed with Tukey's test); in H n = 17–24 flies and in I n = 9–17 flies from three independent replicates.
Before monitoring further effects of dilp1 overexpression in the fat body on regulation of adult body weight and organismal size, we wanted to determine whether dilp1 has an effect on larval development and/or growth. We therefore measured the time from egg to pupariation and size of pupae to determine whether dilp1 overexpression affected timing of larval development and growth during this stage. Using the ppl-Gal4 driver, we did not observe any effect on the time from egg to pupa compared to controls (Figure 2B). Pupal volume, as a measurement of larval growth, was not altered by ppl-Gal4>dilp1 (Figure 2C), suggesting that the larval growth was not affected. As expected (16, 31), overexpression of dilp6 also had no effect on pupal size (Figure 2C). However, as shown earlier for ubiquitously expressed dilp2 (23), dilp2 expression in the fat body generated a strong increase in pupal volume, suggesting that growth occurred during the larval stage (Figure 2C). Driving dilp1 with the c929 Gal4 line, which directs expression to several hundred dimm-expressing peptidergic neurons including IPCs and other neurosecretory cells (57), we also did not observe any effect on time to pupariation or pupal volume (Figures 2B,C). Taken together, our data suggest the ectopic dilp1 does not affect larval growth or developmental time, whereas dilp2, as shown earlier, does affect larval growth (developmental time was not monitored). Since only one receptor (dInR) is known for DILP1, DILP2, and DILP6 in Drosophila, the differential action of these peptides on larval growth could perhaps be explained by stage-specific and differential control by insulin/IGF-binding proteins such as secreted decoy of insulin receptor (SDR), acid-labile subunit (ALS), and imaginal morphogenesis protein-Late 2 (Imp-L2), which are known to inhibit the action of DILPs and affect growth (55, 58–61).
Next, we found that organismal size, estimated by wing size (Figures 2D,E) and thorax length (Figures 2D,F), increased after ectopic expression of dilp1 in the fat body. Thus, overexpression of dilp1 does not just lead to a weight increase, but to actual growth of the organism. Since we see no effect of dilp1 expression on developmental time or pupal volume, but register increased body weight and size of adults, we propose that ectopic dilp1, like dilp6, promotes growth of adult tissues during the pupal stage. This stage also correlates with the temporal expression pattern of dilp1/DILP1 (17).
To substantiate the data obtained with the dilp2 and ppl-Gal4 drivers, we also tested ectopic dilp1 expression using another fat body driver, to-Gal4, and a neuroendocrine cell driver, c929. With to-Gal4>dilp1, we also noted an increase in weight of recently emerged female and male flies (Supplementary Figures 6D,E; Table 1), but no change in body size except a minor increase in thorax length in females (Supplementary Figures 6F,G). The female to>dilp1 flies increased further in weight the first 6–7 days of adult life, but not later (Supplementary Figure 6D), whereas the males did not (Supplementary Figure 6E). Furthermore, with the to-Gal4 driver, there was no increase in pupal volume, supporting that dilp1 does not affect larval growth (Supplementary Figure 6H).
Ectopic expression of dilp1 in neuroendocrine cells by means of the c929-Gal4 increased adult body weight (Supplementary Figure 7A), but had no effect on wing area in males and females (Supplementary Figure 7B), suggesting that the dilp1 expression (and/or systemic release) was not strong enough to produce major effects. As mentioned, expressing dilp1 in cells already producing it (IPCs) may not yield increased release and an ensuing phenotype, and additional neurosecretory cells in the c929 line may not release enough DILP1.
MR and RQ in Pupae of Different Genotypes
To investigate a possible role of dilp1 in metabolism and utilization of nutrients during pupal development, metamorphosis, and adult tissue growth, we determined MR and RQ in pupae of different genotypes. First we characterized the metabolic trajectory in control pupae (w1118) by measuring cumulative MR daily throughout pupal development (Figure 3A). These data show the exponential MR curve typical for developing insects, including D. melanogaster (62). To minimize handling stress, we chose to investigate only the end of pupal development in more detail and measured MR and RQ in 4-day-old pupae (that is the cumulative MR between hours 96 and 120 after pupation). For this experiment, we only obtained useful data for ppl-Ga4 overexpression animals since the mutant flies displayed high mortality in the respirometry setup and therefore numbers of data points obtained were small (not shown). Instead, we monitored the effect of dilp1 gain of function by expression in the fat body using ppl-Gal4 (to match other gain of function experiments). As can be seen in Figures 3B,C, the ppl>dilp1 and ppl>dilp6 differed significantly from the controls in the respirometry assay. The MR was higher and RQ was lower in the dilp1 and dilp6 overexpression flies than in the control flies. RQ values, around 0.6 in both overexpression lines, suggest pure lipid metabolism (47), and lipids are known to be a major or sole fuel during metamorphosis of insects (45, 62–64). Our findings strongly suggest that dilp1 and dilp6 affect metabolism (especially of lipids) in the pupa, probably by acting on the residual larval fat body that is present throughout pupal development and the first few days of adult life (65, 66).
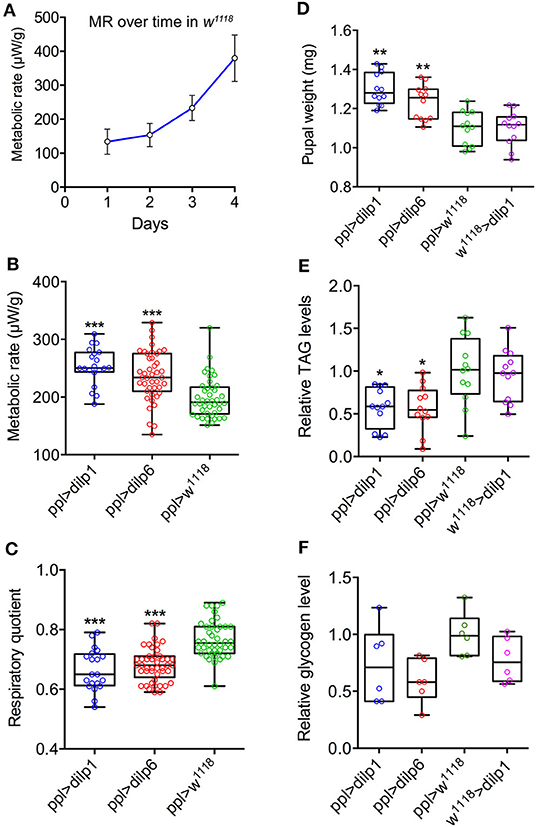
Figure 3. Metabolic rate trajectories and respiratory quotients (RQs) during pupal development respond to dilp1 and dilp6 overexpression in the fat body. (A) Metabolic rate in w1118 flies increased exponentially as a function of time. For the ensuing overexpression analysis, we studied the period 96–120 h after pupation. Data are presented as means ± S.E.M, n = 20–47 flies from three independent replicates. (B) Metabolic rate was significantly elevated during this period in dilp1 and dilp6 overexpression flies (ppl-Gal4) when compared to w1118 flies. Data are presented as means ± S.E.M, n = 20–47 flies for each genotype from three independent replicates (***p < 0.001, compared to w1118 flies, as assessed by two-way ANOVA followed with Tukey's test). Data are from both males and females as no difference was found in the ANOVA for sex. (C) RQ, reflecting catabolic energy substrate, was significantly lower in the overexpression flies when compared to the control flies and indicates a shift from mixed fuel catabolism (RQ = 0.7–0.8) to predominantly lipid catabolism (RQ < 0.7). Data are presented as means ± S.E.M, n = 20–47 flies for each genotype from three independent replicates (***p < 0.001, compared to w1118 flies, as assessed by one-way ANOVA followed with Tukey's test). Data are from both males and females as no difference was found in the ANOVA for sex. (D) Four-day-old pupae (mixed male and female) were weighed (wet weight) before extraction and TAG determination. Overexpression of dilp1 and dilp6 both resulted in increased pupal weight. (E) Levels of TAG were measured in the pupae used for weighing in D. Overexpression of each dilp resulted in decreased TAG levels. (F) Glycogen levels in 4-day-old pupae (no significant changes). In (D,E), 12 replicates per genotype with 4 pupae in each replicate (each data point represents 4 pupae); in (F), 6 replicates per genotype with 4 pupae in each replicate (*p < 0.05, **p < 0.01, one-way ANOVA followed by Tukey's test).
TAG and Carbohydrates in Pupae of Different Genotypes
To determine whether lipids indeed fuel growth of adult tissues in 4-day-old pupae, we determined TAG levels after overexpression of dilp1 and dilp6 in fat body (ppl-Gal4). Pupae of both genotypes displayed increased weight (Figure 3D) and also significantly reduced TAG levels (Figure 3E), compared to controls of the same age. The levels of glycogen were not significantly altered in pupae after ectopic expression of dilp1 and dilp6 (Figure 3F) and neither were glucose levels (Supplementary Figure 8).
Effects of dilp1 Manipulations on Metabolism and Body Mass in Newly Eclosed and Young Flies
We have shown that dilp1/DILP1 expression is prominent in pupae as well as during the first 5–7 days of adulthood (17). What is the role of the peptide in young flies? To investigate whether dilp1/dilp1 signaling affects adult metabolism, we monitored the levels of TAGs, glycogen, and glucose in recently emerged and 3-day-old dilp mutant and dilp1-overexpressing female flies (Figure 4). In newly eclosed dilp1 mutant flies, glycogen was significantly lowered, whereas glucose and glycogen were diminished in dilp2 mutants, while in the dilp1/dilp2 double mutants, all three compounds were decreased (Figures 4A–C). In the 3-day-old flies, dilp1 and double mutants displayed reduced glycogen, whereas in dilp1/dilp2 double mutants, TAG was increased (Figures 4D–F).
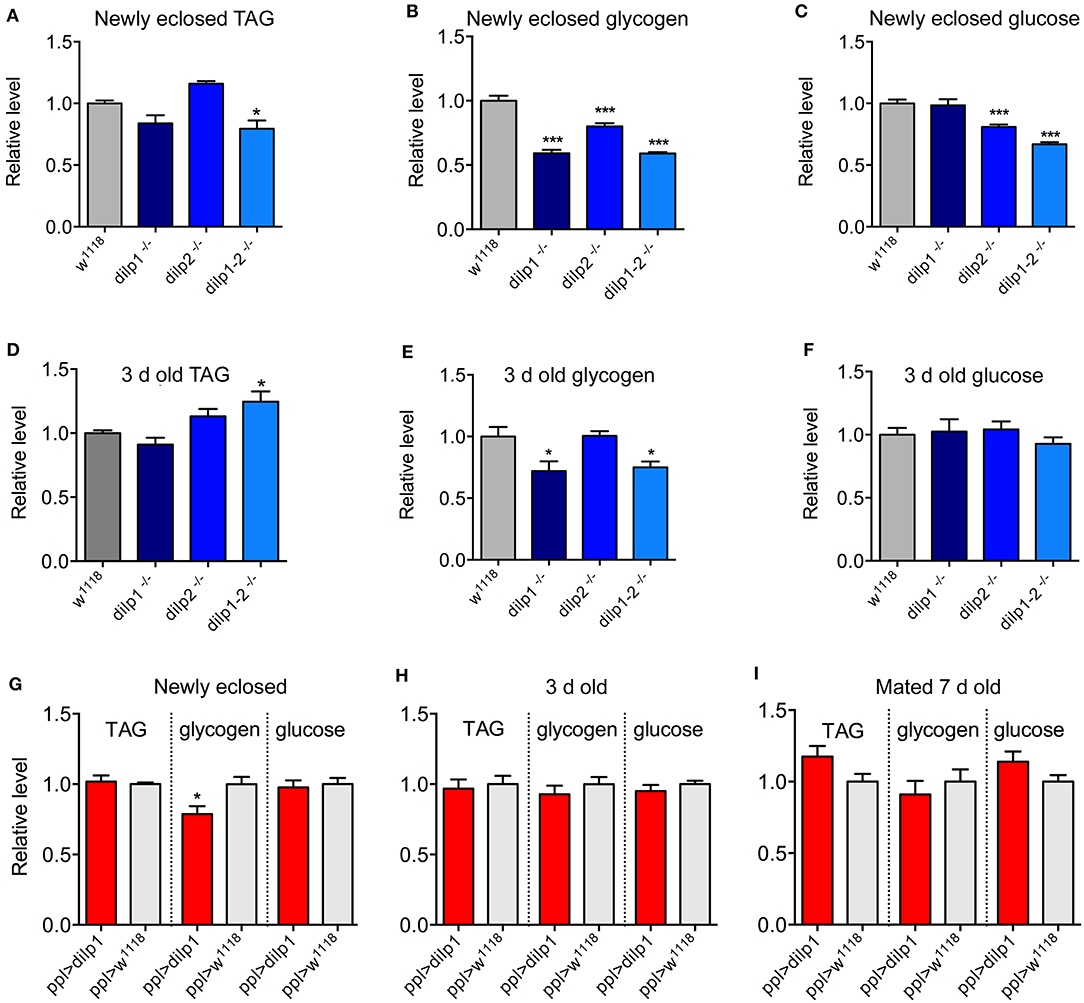
Figure 4. Contents of TAG, glycogen, and glucose in female mutant flies and after ectopic dilp1 expression. (A–C) Contents of TAG and carbohydrates in recently emerged mutants and controls. Note that for dilp1 mutants, only glycogen was diminished, whereas for dilp1-2 mutants, all three compounds were decreased. Eight replicates per genotype with 5–6 flies in each replicate (*p < 0.05, ***p < 0.001, one-way ANOVA followed by Tukey's test). (D–F) In 3-day-old flies, glycogen was also reduced in dilp1 mutants and double mutants. Eight replicates per genotype with 5–6 flies in each replicate (*p < 0.05, ***p < 0.001, one-way ANOVA followed by Tukey's test). (G–I) Overexpression of dilp1 in fat body (ppl-Gal4) only affected glycogen levels in newly emerged flies. Six to eight replicates per genotype with 5–6 flies in each. Data are presented as means ± S.E.M (*p < 0.05, ***p < 0.001, compared to w1118 flies, as assessed by unpaired Student's t-test).
Using ppl-Ga4 to overexpress dilp1, we found that the only effect was a reduction of glycogen in recently eclosed flies; at 3 or 7 days of age, no effect was noted (Figures 4G–I). Thus, it appears that intact dilp1 signaling is required for mobilization of glycogen stores in newly emerged and young flies. The finding that both dilp1 mutants and flies with dilp1 overexpression display decreased glycogen may suggest that any change of dilp signaling offsets glycogen homeostasis.
Next we asked whether dilp1 has an effect on body mass and food ingestion in adult flies. Hence, we first determined the wet weight of mated 6- to 7-day-old flies and found that it was significantly increased in ppl>dilp1 flies compared to the controls in both female (Figure 5A; Table 1) and male flies (Figure 5B). We furthermore noted increased weight for ppl>dilp2 and ppl>dilp6 flies (Figures 5A,B; Table 1). Comparing 1-day-old female flies with 6- to 7-day-old ones, it is clear that all genotypes display increased body mass, but at each time point, the ppl>dilp1 flies increase significantly more than controls (Figure 5C). This weight increase in all genotypes of females may be related to ovary growth, since male flies do not increase their weight the first week; they instead weigh less (Supplementary Figure 6C). However, since male ppl>dilp1 flies also display higher body mass than controls at both time points, it is suggestive that dilp1 plays a role in regulation of other aspects of body mass.
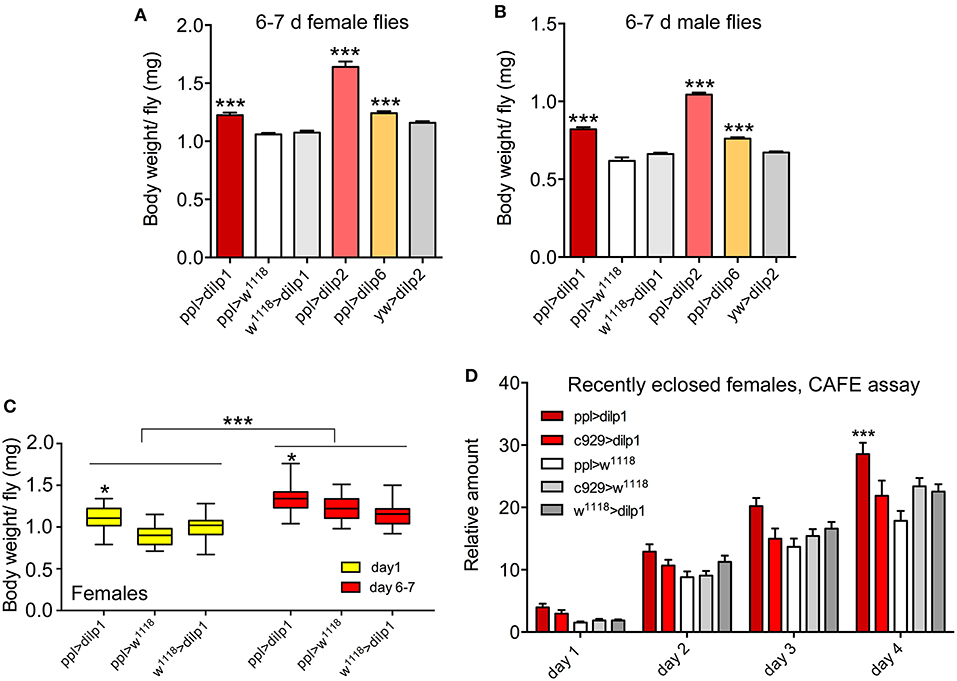
Figure 5. Overexpression of dilp1 affects body mass and feeding in young adult flies. (A, B) Overexpression of dilp1, dilp2, and dilp6 in fat body (ppl-Gal4) all lead to adult flies (1 week old) with increased body weight in both females and males. Data are presented as means ± S.E.M, n = 24–30 flies for each genotype from three independent replicates. Except for ppl>dilp2, 13 flies were used (*p < 0.05, one-way ANOVA followed with Tukey's test). (C) Body weight of 6- to 7-day female flies is increased for all genotypes compared to 1-day flies and the ppl>dilp1 flies weigh more than controls at both time points. Data are presented as medians ± range, n = 23–27 flies for each genotype from three independent replicates (*p < 0.05, ***p < 0.001, two-way ANOVA followed with Tukey's test). (D) Food intake (CAFE assay) is increased over 4 days (cumulative data shown) in flies overexpressing dilp1 in fat body, but not in neuroendocrine cells (c929-Gal4). Data are presented as means ± S.E.M, n = 15–30 flies for each genotype from three independent replicates (*p < 0.05, two-way ANOVA followed with Tukey's test).
As a comparison, dilp2>dilp1 had only minor effects on body weight of female flies; only in 6- to 7-day-old dilp2>dilp1 flies was there an increase compared to the controls of the same age (Supplementary Figure 6A; Table 1), whereas in males, a significant increase in weight was noted at both ages for dilp2>dilp1 compared to controls, and a loss of weight over the next 6 days for all genotypes (Supplementary Figure 6B). Using another fat body Gal4-driver, to-Gal4, to express dilp1, we obtained results similar to ppl-Gal4 (Supplementary Figures 6D,E). Monitoring body mass another week (13–14 days) in to>dilp1 flies, we found that, in males, dilp1-overexpressing flies are still heavier than controls, whereas in females, the different genotypes weigh the same (Supplementary Figures 6D,E). Thus, these experiments indicate that there is a sex difference in the body mass profile over the first week that might reflect egg development. However, dilp1 overexpression leads to increased body mass in both sexes compared to controls in both 1-day and 6- to 7-day-old flies.
It was suggested that dilp6 promotes growth of adult tissues during pupal development by utilizing nutrients stored in the larval fat body, which is carried into the pupa (16). This may be the case also for dilp1, and if so, newly eclosed dilp1-overexpressing flies should have less energy stores in the residual larval fat body. Also, the increased body mass over the first week requires additional nutrients. To test this, we monitored feeding in recently emerged dilp1-overexpressing flies (ppl>dilp1) and controls. Indeed, flies overexpressing dilp1 displayed increased food ingestion over the first 4 days after adult emergence compared to controls (Figure 5D), suggesting that these flies were in extra need of nutrients.
Effects of dilp1 on Adult Stress Resistance and Fecundity
Genetic ablation of the IPCs, which produce DILP1, 2, 3, and 5, results in increased starvation resistance in adult flies (21). Thus, we asked whether alterations of dilp1 expression have effects on adult physiology such as survival during starvation or desiccation (also referred to as starvation and desiccation resistance). We investigated the starvation resistance in newly emerged, 3-day-old and 1-week-old female dilp1, dilp2, and dilp1/dilp2 mutant flies (all virgins). The newly eclosed dilp1 mutant flies display strongly reduced survival during starvation and the dilp1/dilp2 mutants increased survival compared to control flies, whereas the starvation resistance of dilp2 mutants is similar to the controls (Figure 6A; Table 2). In 3-day-old virgin flies, the dilp1 and dilp1/dilp2 mutants display reduced survival during starvation, whereas the dilp2 mutants perform similar to the controls (Figure 6B; Table 2). In a previous study (35), it was shown that 6- to 7-day-old female flies display a similar response to starvation: the dilp1/dilp2 mutants exhibit the strongest reduction in survival, followed by dilp1 mutants that also are much less stress tolerant, whereas the performance of dilp2 mutants and control flies is very similar (see Table 2). Here, we also tested 6- to 7-day-old male flies and found that they survived starvation in a manner different from females with dilp2 and double mutants displaying diminished stress resistance, whereas dilp1 mutants survive similar to controls (Supplementary Figure 9A). Thus, a difference between sexes was detected in metabolic stress responses of the different mutants that might suggest a link between dilp1 and egg development in females. We also see a change in the effects of dilp1 mutation over age in female flies that may reflect the switch from larval to adult fat body, as well as ovary maturation.
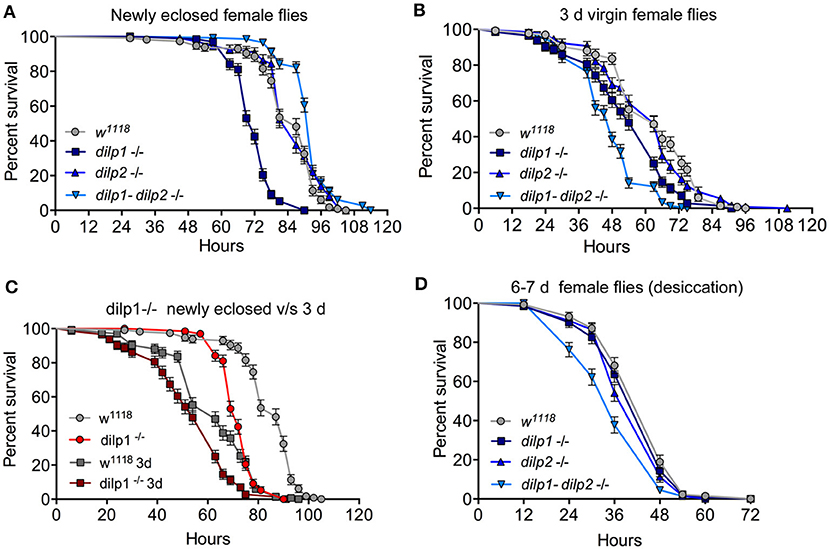
Figure 6. Effects of mutated dilp genes on adult responses to starvation and desiccation change in early adult life. (A) In newly eclosed female flies, dilp1 mutant flies display reduced survival during starvation (p < 0.001) compared to the other mutants and control. The double mutant is significantly more resistant (p < 0.001). n = 109–147 flies for each genotype from three independent replicates. (B) In 3-day-old virgin female flies, dilp1-dilp2 double mutants are the least starvation resistant (p < 0.001) followed by the dilp1 mutants; n = 129–148 flies for each genotype from three independent replicates. (C) Comparison between newly eclosed and 3-day-old flies exposed to starvation. Both mutants and controls survive longer as recently eclosed flies and mutants perform worse than controls at each time point (p < 0.001). n = 114–144 flies from three independent replicates. (D) When exposed to desiccation, 6- to 7-day-old female double mutants are less resistant than the other genotypes (p < 0.001), n = 132–135 flies from three independent replicates. Data are presented in survival curves and the error bars show S.E.M., as assessed by log-rank (Mantel–Cox) test.
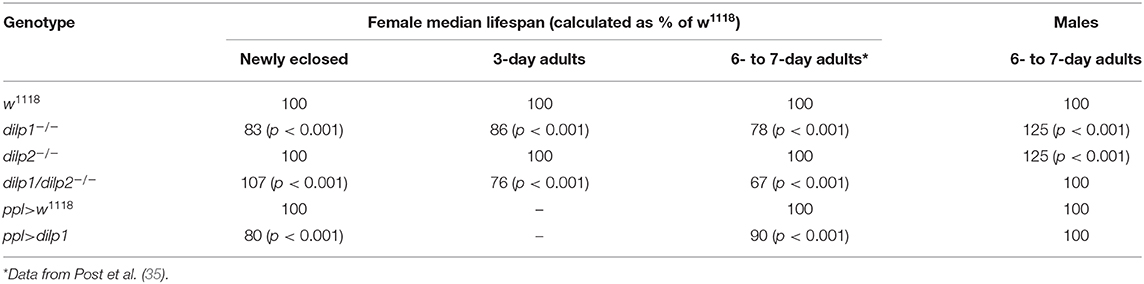
Table 2. Median lifespans of dilp1 mutant and dilp1 overexpressing female and male flies exposed to starvation display a sex dimorphism.
As seen above, our data suggest a change in the response to loss of dilp1 and dilp1/dilp2 function in starvation resistance over the first week of adult life. It is known that newly eclosed wild-type flies are more resistant to starvation than slightly older flies (66). Thus, we compared the survival during starvation in recently emerged and 3-day-old virgin flies. As seen in Figure 6C (based on data in Figures 6A,B), recently eclosed control flies (w1118) indeed exhibit increased starvation resistance compared to controls that were tested when 3 days old. Also, the dilp1 mutant flies are more starvation resistant when tested as newly eclosed than as older flies, and the mutants perform less well than controls at both ages (Figure 6D). However, the most drastic change within the first week is that dilp1 mutants yield the strongest reduction in starvation resistance as newly eclosed flies, and then in 3-day and 6- to 7-day-old flies, the dilp1/dilp2 mutants are the ones with the lowest stress resistance. Thus, a change in the role of dilp1 seems to occur as the fly matures during the first few days of adult life. To provide additional evidence that dilp1 impairs starvation resistance, we performed dilp1-RNAi using a dilp2-Gal4 driver. The efficiency of the dilp2>dilp1-RNAi was tested by qPCR (Supplementary Figure 10A) where a strong decrease in dilp1, but not dilp2 or dilp6, was seen. The dilp1-RNAi in IPCs resulted in newly eclosed flies that displayed reduced survival during starvation (Supplementary Figure 10B), similar to dilp1 mutant flies.
Next, we investigated the effect of the mutations on the flies' response to desiccation (dry starvation). One-week-old flies were put in empty vials and survival was recorded. Female dilp1/dilp2 mutants were more sensitive to desiccation than controls and both of the single mutants (Figure 6D). In males, the double mutants also displayed higher mortality during desiccation, whereas the two single mutants were more resistant than controls (Supplementary Figure 9B). Thus, there is a sex dimorphism in how the different mutants respond to both desiccation and starvation, and in female dilp1 mutants, desiccation resistance seems not to be affected, in contrast to starvation resistance. This difference in response to desiccation may contribute to the sex dimorphism in wet weight after manipulating dilp1 signaling.
What about effects of dilp1 gain of function on stress tolerance? When overexpressing dilp1 with the fat body driver ppl-Gal4 newly eclosed and 6- to 7-day-old female flies become less resistant to starvation compared to parental controls (Figures 7A,B). However, in 6- to 7-day-old male flies, there is no difference between controls and flies with ectopic dilp1, using ppl- and c929-Gal4 drivers (Supplementary Figures 10C,D). We furthermore investigated starvation resistance in flies overexpressing dilp1 in IPCs (dilp2>dilp1) and in most neuroendocrine cells (c929>dilp1) and found that, in just eclosed female flies, overexpression reduced survival (Figures 7C,E), whereas in 1-week-old flies, all genotypes displayed the same survival (Figures 7D,F). Thus, in females, it appears as if both knockout and overexpression of dilp1 reduce starvation resistance in recently eclosed flies. It was shown earlier that conditional knockdown of dilp6 by RNAi during the pupal stage resulted in newly eclosed flies with increased survival during starvation (16), suggesting that the effects of dilp6 and dilp1 mutation are different.
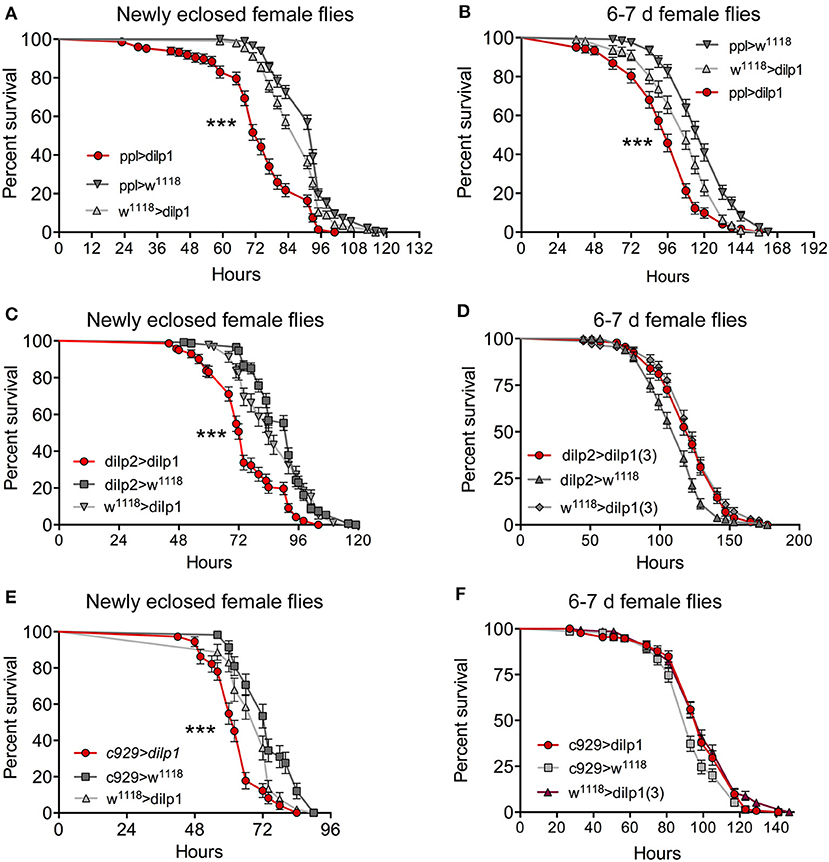
Figure 7. Overexpression of dilp1 in the fat body affects starvation resistance in adult flies. (A, B) In recently eclosed (A) and 6- to 7-day-old (B) female flies, overexpression of dilp1 (with ppl-Gal4) leads to a decrease in survival during starvation n = 147–201 flies per genotype from three independent replicates [***p < 0.001, as assessed by log-rank (Mantel–Cox) test]. (C,D) Expressing dilp1 in IPCs with a dilp2-Gal4 driver also diminishes starvation survival in recently eclosed flies, n = 92–148 flies from three independent replicates [***p < 0.001, as assessed by log-rank (Mantel–Cox) test], but not in 6- to 7-day flies (n = 122–132 flies from three independent replicates). (E,F) Using c929 to drive dilp1 in recently eclosed and 6- to 7-day-old adult flies altered starvation resistance only in the recently eclosed ones [***p < 0.001 as assessed by log-rank (Mantel–Cox) test, n = 132–135 flies per genotype from three independent replicates].
After ectopic expression of dilp1 in the fat body, there was an increase in food intake (cumulative data) in 1-week-old flies over 4 days (Figure 8A), suggesting that metabolism is altered also in older flies. Since the effect of dilp1 manipulations seems stronger in female flies, we asked whether fecundity is affected by overexpression of dilp1. An earlier study showed that dilp1 mutant flies are not deficient in number of eggs laid, or the viability of offspring (egg to pupal viability), although the dilp1/dilp2 double mutants displayed a reduction in viability of these eggs (35). Here, we expressed dilp1 in fat body (ppl-Gal4) and detected an increase in number of eggs laid over 24 h in 6- to 7-day-old flies (Figure 8B). Both ppl-Gal4- and c929-Gal4-driven dlip1 decreased the viability of eggs laid as monitored by numbers of eggs that developed into pupae (Figure 8C). As a comparison, we noted no difference in number of eggs retained in ovaries in 3-day-old dilp1 mutant flies (Figure 8D) similar to the 6- to 7-day-old flies studied previously (35).
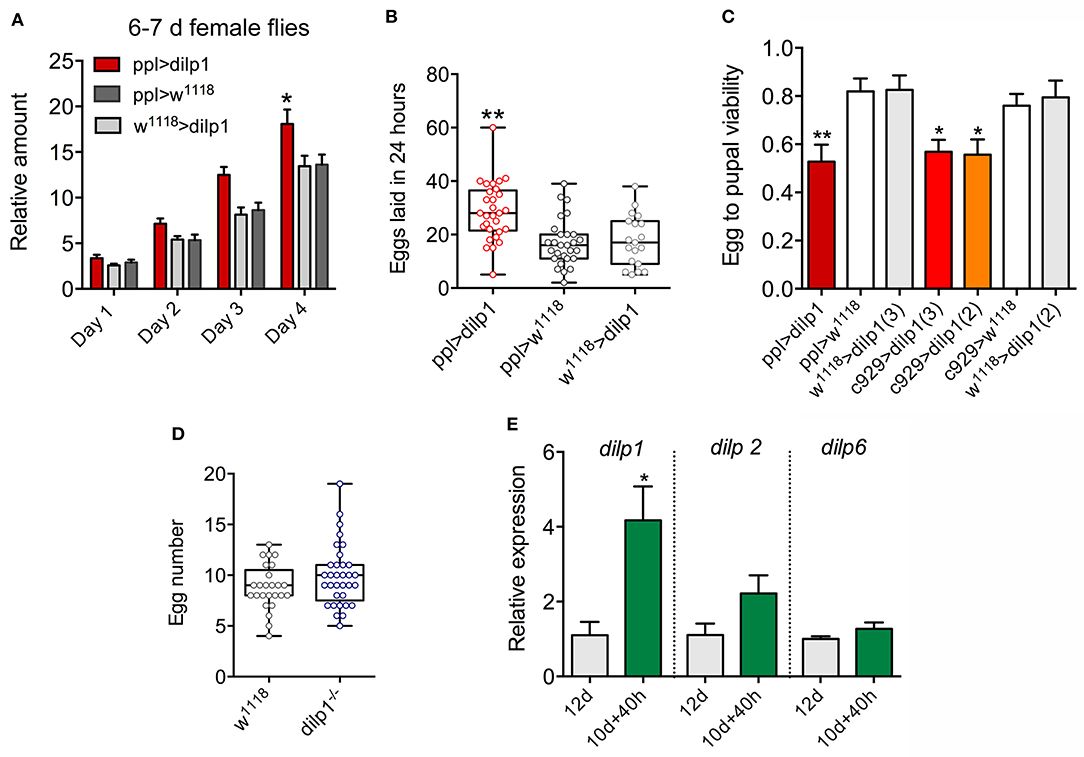
Figure 8. Overexpression of dilp1 affects food intake and fecundity. (A) In CAFE assay, the dilp1 overexpressing flies (6- to 7-day-old females) display increased food intake over 4 days (cumulative data shown). Data are presented as means ± S.E.M, n = 23–24 flies from three independent replicates (*p < 0.05, two-way ANOVA followed by Tukey's test). (B) Number of eggs laid in 24 h by 6- to 7-day-old flies. We analyzed 19–29 pairs of flies from 3 replicates (**p < 0.01, one-way ANOVA followed by Tukey's test). (C) The egg to pupal viability is diminished in flies with dilp1 expressed in fat body (ppl-Gal4) and neuroendocrine cells [c929-Gal4, using two different UAS-dilp1 (2 and 3)]. Data are presented as means ± S.E.M; more than 276 eggs from 6 replicates were monitored (*p < 0.05, unpaired Student's t-test). (D) Number of eggs in ovaries of 3-day-old flies is not affected in dilp1 mutants. A total of 25–33 flies from three replicates were analyzed. (E) dilp1 mRNA is upregulated during starvation for 40 h in 10-day-old adult w1118 flies, compared to 12-day-old flies fed normal food, as monitored by qPCR. No effect was seen on dilp2 and dilp6 levels. Data are presented as means ± S.E.M.; 3 replicates with 10 flies in each replicates were monitored (*p < 0.05, unpaired Student's t-test).
In flies older than 7 days kept under normal laboratory conditions, dilp1/DILP1 expression is barely detectable. Thus, we next asked whether there is any physiological trigger of increased dilp1 expression in older adult flies, except for diapause (17) and experimental ones such as ectopic expression of sNPF or knockdown of dilp6, dilp2 and dilp2,3,5 (17, 35, 67). Here, we found that 40 h starvation of 10-day-old flies (w1118) leads to a significant increase in dilp1, but not in dilp2 or dilp6 (Figure 8E). Thus, at a time (12 days) when dilp1 is barely detectable under normal conditions, there is a 4-fold upregulation during starvation, further suggesting that the peptide indeed plays a role also in older adult flies (and its function is uncoupled from its pupal role).
Discussion
Our study shows that dilp1 gain of function stimulates adult tissue growth and increases MR during the pupal stage, and also affects adult physiology, especially during the first days of adult life. These stages correspond to the time when dilp1 is normally expressed (16, 17, 31). The gain of function experiments herein suggest that the developmental role of ectopic dilp1 could be similar to that of dilp6 (16), namely, to stimulate growth of adult tissues during pupal development. We furthermore show that in the adult fly, dilp1 is upregulated during starvation and genetic gain and loss of function of dilp1 signaling diminishes the flies' survival under starvation conditions in a sex-specific manner. These novel findings, combined with previous data that demonstrated high levels of dilp1 during adult reproductive diapause (17) and the role of dilp1 as a pro-longevity factor during aging (35), suggest a wide-ranging importance of this signaling system. Not only does dilp1 expression correlate with stages of non-feeding (or reduced feeding), these stages are also associated with lack of reproductive activity and encompass the pupa, newly eclosed flies, and diapausing flies. Under normal conditions, the transient expression of dilp1/DILP1 during the first few days of adult life may be associated with a metabolic transition [remodeling from larval to adult fat body; (68)] and the process of sexual maturation (gonad growth and differentiation). Our data also suggest that dilp1 affects physiology more prominently in young female flies than in males, which might be associated with ovary maturation.
It is also interesting to note that the diminished starvation resistance in dilp1 and dilp1/dilp2 mutants is opposite to the phenotype seen after IPC ablation, mutation of dilp1-4, or diminishing IIS by other genetic interventions (11, 21, 69, 70). Thus, in recently eclosed flies, dilp1 appears to promote starvation resistance rather than diminishing it. Furthermore, the decreased survival during starvation in female dilp1 mutants is the opposite of that shown in dilp6 mutants (16), indicating that dilp1 action is different from the other insulin-like peptides tested.
In Drosophila, the final body size is determined mainly by nutrient-dependent hormonal action during the larval feeding stage (10, 12, 23, 29). However, some regulation of adult body size can also occur after the cessation of the larval feeding stage, and this process is mediated by dilp6 acting on adult tissue growth in the pupa in an ecdysone-dependent manner (16, 31). This is likely a mechanism to ensure growth of adult tissues if the larva is exposed to shortage of nutrition during its feeding stage. Our findings suggest that dilp1 can function as another regulator of growth during the pupal stage. We show here that overexpression of dilp1 promotes organismal growth in the pupa, probably at the cost of stored nutrients derived from the larval feeding stage. This is supported by our RQ data that clearly show a shift from mixed-energy substrate metabolism in control flies toward almost pure lipid catabolism at the end of pupal development in the dilp1 overexpression flies (also seen for dilp6 gain of function in our experiments). Furthermore, TAG (but not carbohydrate) levels in dilp1 overexpression pupae were clearly decreased, which likely reflects the shift in catabolic energy substrate also seen in the RQ using respirometry. It should be noted that insects predominantly use lipids as fuel during metamorphosis (45, 62–64) and dilp1 overexpression increases lipid catabolism. Our study hence suggests that dilp1 can parallel dilp6 (16, 31) in balancing adult tissue growth and storage of nutrient resources during pupal development. This is interesting since dilp6 is an IGF-like peptide that is produced in the nutrient sensing fat body (16, 31), whereas the source of the insulin-like dilp1 is the brain IPCs (17, 20).
In contrast to the dilp1 gain of function, our experiments with dilp1 mutant flies did not show a clear effect on adult body growth, only a decrease in weight. Is this a result of compensation by other DILPs? We showed earlier that young adult dilp1 mutant flies display increased dilp6 and vice versa (17), suggesting feedback between these two peptide hormones in adults. During the pupal stage, this feedback appears less prominent in dilp1 mutants and we detected no effects on dilp2, dilp3, or dilp6 levels. Furthermore, overexpression of dilp1 in fat body or IPCs has no effect on pupal levels of dilp2 and dilp6. Thus, at present, we have no evidence for compensatory changes in other dilps/DILPs in pupae with dilp1 manipulations. However, under normal conditions (in wild-type pupae), dilp6 levels are far higher than those of dilp1 (31) [see also modENCODE_mRNA-Seq_tissues; (71)], which could buffer the effects of changes in dilp1 signaling.
DILPs and IIS are involved in modulating responses to starvation, desiccation, and oxidative stress in Drosophila [see Grönke et al. (11), Broughton et al. (21), and Nässel and Vanden Broeck (55)]. Flies with ablated IPCs or genetically diminished IIS display increased resistance to several forms of stress, including starvation (11, 21). Conversely, overexpression of dilp2 increases mortality in Drosophila (24). We found that young dilp1 mutant flies displayed diminished starvation resistance. In both recently eclosed and 3-day-old flies, mutation of dilp1 decreased survival during starvation (but not in 6- to 7-day-old ones).
Action of dilp1 in the adult fly is also linked to reproductive diapause in females, where feeding is strongly reduced (72), and both peptide and transcript are upregulated (17). Related to this, we found here that dilp1 mRNA is upregulated during starvation in 12-day-old flies. Furthermore, it was shown that expression of dilp1 (dilp1 rescue) increases lifespan in dilp1/dilp2 double mutants, suggesting that loss of dilp2 induces dilp1 as a factor that promotes longevity (35). Thus, dilp1 activity is beneficial also during adult life, even though its expression under normal conditions is very low (16, 17, 31). This pro-longevity effect of dilp1 is in contrast to dilp2, 3, and 5 and the mechanisms behind this effect are of great interest to unveil.
A previous study showed that in wild-type (Canton S) Drosophila, DILP1 expression in young adults is sex-dimorphic with higher levels in females (17). In line with this, we show here that starvation resistance in young flies is diminished only in female dilp1 mutant and dilp1 overexpression flies. Thus, taken together, we found earlier that dilp1 displays a sex-specific expression (17) and here we show female-specific function in young adult Drosophila. It is tempting to speculate that the more prominent role of dilp1 in female flies is linked to metabolism associated with reproductive physiology and early ovary maturation, which is also reflected in the dilp1 upregulation during reproductive diapause (17). In fact, we show here that egg-laying increased after dilp1 overexpression, and an earlier study demonstrated a decreased egg laying in dilp1 mutant flies (17). Part of the sex dimorphic effects on body weight of young adults after dilp1 manipulations might be a result of a differential role of dilp1 in water homeostasis.
We show here that IPC-derived dilp1 displays several similarities to the fat body-produced dilp6, including temporal expression pattern, growth promotion, effects on adult stress resistance and lifespan. Additionally, dilp1 may play a role in regulation of nutrient utilization and metabolism during the first few days of adult life, especially in females. At this time, larval fat body is still present and utilized as energy fuel/nutrient store (66) and this source also contributes to egg development (73). Curiously, there is a change in the action of DILP1 between the pupal and adult stages from being able to stimulate growth (agonist of dInR, like DILP6) in pupae, to acting in a manner opposite to DILP2, DILP6, and other DILPs in adults in regulation of lifespan and stress responses [see also Post et al. (35)]. Only one dInR is known so far (excluding the G protein-coupled receptors for the relaxin-like DILP7 and DILP8). Thus, the mechanisms behind this apparent switch in function of DILP1 signaling remain an open question. One possibility is that DILP1 acts via different signaling pathways downstream the dInR in pupae and adults. An obvious difference between these two stages is the presence of larval-derived fat body in the pupa and during the first few days of adults and its replacement by functional adult fat body in later stages (51, 66). Perhaps dInR-mediated action differs in these types of fat body when activated by DILP1. Another possibility is stage-specific expression of insulin/IGF-binding proteins such as SDR, ALS, and Imp-L2, mentioned earlier, that could affect the activity of DILP1 in particular [see Arquier et al. (58), Honegger et al. (59), and Okamoto et al. (60, 61)].
In the future, it would be interesting to investigate whether DILP1 acts differently on larval/pupal and adult fat body, or act on different downstream signaling in the two stages of the life cycle. Also, the possibility that dilp1 and dilp6 interact to regulate growth and metabolism in Drosophila is worth pursuing.
Data Availability Statement
All datasets generated for this study are included in the article/Supplementary Material.
Author Contributions
SL: conceptualization, performed experiments, interpreted data, and wrote paper. SP and PL: performed experiments and interpreted data. JV: contributed unpublished reagents. MT: conceptualization, contributed reagents, and supervision. DN: conceptualization, interpreted data, contributed reagents, obtained funding, wrote paper, and supervised study. All authors read, edited, and finally approved manuscript.
Funding
This work was funded by the Swedish Research Council (Vetenskapsrådet), grant number 2015-04626 (DN); the National Institutes of Health (NIH), grant number NIH R37 AG024360 (MT); and CNRS, internal funding (JV).
Conflict of Interest
The authors declare that the research was conducted in the absence of any commercial or financial relationships that could be construed as a potential conflict of interest.
Acknowledgments
This manuscript has been released as a preprint at bioRxiv (74). Stocks obtained from the Bloomington Drosophila Stock Center (NIH P40OD018537) were used in this study, and we thank the Bloomington Drosophila Stock Center (Bloomington, IN), the Vienna Drosophila Resource Center (Vienna, Austria), and Drs. M. J. Pankratz, B. Dauwalder, V. Monnier, P. H. Taghert, V. Tsarouhas, and H. Stocker for flies.
Supplementary Material
The Supplementary Material for this article can be found online at: https://www.frontiersin.org/articles/10.3389/fendo.2020.00180/full#supplementary-material
References
1. Garofalo RS. Genetic analysis of insulin signaling in Drosophila. Trends Endocrinol Metab. (2002) 13:156–62. doi: 10.1016/S1043-2760(01)00548-3
2. Barbieri M, Bonafe M, Franceschi C, Paolisso G. Insulin/IGF-I-signaling pathway: an evolutionarily conserved mechanism of longevity from yeast to humans. Am J Physiol Endocrinol Metab. (2003) 285:E1064–71. doi: 10.1152/ajpendo.00296.2003
3. Edgar BA. How flies get their size: genetics meets physiology. Nat Rev Genet. (2006) 7:907–16. doi: 10.1038/nrg1989
4. Kaletsky R, Murphy CT. The role of insulin/IGF-like signaling in C. elegans longevity and aging. Dis Model Mech. (2010) 3:415–9. doi: 10.1242/dmm.001040
5. Antonova Y, Arik AJ, Moore W, Riehle MR, Brown MR. Insulin-like peptides: structure, signaling, and function. In: Gilbert LI, editor. Insect Endocrinology. New York, NY: Elsevier/Academic Press (2012). p. 63–92. doi: 10.1016/B978-0-12-384749-2.10002-0
6. Froesch ER, Zapf J. Insulin-like growth factors and insulin: comparative aspects. Diabetologia. (1985) 28:485–93. doi: 10.1007/BF00281982
7. Baker J, Liu JP, Robertson EJ, Efstratiadis A. Role of insulin-like growth-factors in embryonic and postnatal-growth. Cell. (1993) 75:73–82. doi: 10.1016/S0092-8674(05)80085-6
8. Watanabe Y, Miyamoto Y, Matsuda T, Tanaka M. Relaxin-3/INSL7 regulates the stress-response system in the rat hypothalamus. J Mol Neurosci. (2011) 43:169–74. doi: 10.1007/s12031-010-9468-0
9. Tokarz VL, Macdonald PE, Klip A. The cell biology of systemic insulin function. J Cell Biol. (2018) 217:2273–89. doi: 10.1083/jcb.201802095
10. Oldham S, Hafen E. Insulin/IGF and target of rapamycin signaling: a TOR de force in growth control. Trends Cell Biol. (2003) 13:79–85. doi: 10.1016/S0962-8924(02)00042-9
11. Grönke S, Clarke DF, Broughton S, Andrews TD, Partridge L. Molecular evolution and functional characterization of Drosophila insulin-like peptides. PLoS Genet. (2010) 6:e1000857. doi: 10.1371/journal.pgen.1000857
12. Brogiolo W, Stocker H, Ikeya T, Rintelen F, Fernandez R, Hafen E. An evolutionarily conserved function of the Drosophila insulin receptor and insulin-like peptides in growth control. Curr Biol. (2001) 11:213–21. doi: 10.1016/S0960-9822(01)00068-9
13. Colombani J, Andersen DS, Leopold P. Secreted peptide Dilp8 coordinates Drosophila tissue growth with developmental timing. Science. (2012) 336:582–5. doi: 10.1126/science.1216689
14. Garelli A, Gontijo AM, Miguela V, Caparros E, Dominguez M. Imaginal discs secrete insulin-like peptide 8 to mediate plasticity of growth and maturation. Science. (2012) 336:579–82. doi: 10.1126/science.1216735
15. Miguel-Aliaga I, Thor S, Gould AP. Postmitotic specification of Drosophila insulinergic neurons from pioneer neurons. PLoS Biol. (2008) 6:e58. doi: 10.1371/journal.pbio.0060058
16. Slaidina M, Delanoue R, Grönke S, Partridge L, Leopold P. A Drosophila insulin-like peptide promotes growth during nonfeeding states. Dev Cell. (2009) 17:874–84. doi: 10.1016/j.devcel.2009.10.009
17. Liu Y, Liao S, Veenstra JA, Nässel DR. Drosophila insulin-like peptide 1 (DILP1) is transiently expressed during non-feeding stages and reproductive dormancy. Sci Rep. (2016) 6:26620. doi: 10.1038/srep26620
18. Cao C, Brown MR. Localization of an insulin-like peptide in brains of two flies. Cell Tissue Res. (2001) 304:317–21. doi: 10.1007/s004410100367
19. Rulifson EJ, Kim SK, Nusse R. Ablation of insulin-producing neurons in flies: growth and diabetic phenotypes. Science. (2002) 296:1118–20. doi: 10.1126/science.1070058
20. Lee KS, Kwon OY, Lee JH, Kwon K, Min KJ, Jung SA, et al. Drosophila short neuropeptide F signalling regulates growth by ERK-mediated insulin signalling. Nat Cell Biol. (2008) 10:468–75. doi: 10.1038/ncb1710
21. Broughton SJ, Piper MD, Ikeya T, Bass TM, Jacobson J, Driege Y, et al. Longer lifespan, altered metabolism, and stress resistance in Drosophila from ablation of cells making insulin-like ligands. Proc Natl Acad Sci USA. (2005) 102:3105–10. doi: 10.1073/pnas.0405775102
22. Okamoto N, Yamanaka N, Satake H, Saegusa H, Kataoka H, Mizoguchi A. An ecdysteroid-inducible insulin-like growth factor-like peptide regulates adult development of the silkmoth Bombyx mori. FEBS J. (2009) 276:1221–32. doi: 10.1111/j.1742-4658.2008.06859.x
23. Ikeya T, Galic M, Belawat P, Nairz K, Hafen E. Nutrient-dependent expression of insulin-like peptides from neuroendocrine cells in the CNS contributes to growth regulation in Drosophila. Curr Biol. (2002) 12:1293–300. doi: 10.1016/S0960-9822(02)01043-6
24. Sato-Miyata Y, Muramatsu K, Funakoshi M, Tsuda M, Aigaki T. Overexpression of dilp2 causes nutrient-dependent semi-lethality in Drosophila. Front Physiol. (2014) 5:147. doi: 10.3389/fphys.2014.00147
25. Zhang H, Liu J, Li CR, Momen B, Kohanski RA, Pick L. Deletion of Drosophila insulin-like peptides causes growth defects and metabolic abnormalities. Proc Natl Acad Sci USA. (2009) 106:19617–22. doi: 10.1073/pnas.0905083106
26. McBrayer Z, Ono H, Shimell M, Parvy JP, Beckstead RB, Warren JT, et al. Prothoracicotropic hormone regulates developmental timing and body size in Drosophila. Dev Cell. (2007) 13:857–71. doi: 10.1016/j.devcel.2007.11.003
27. Mirth CK, Riddiford LM. Size assessment and growth control: how adult size is determined in insects. Bioessays. (2007) 29:344–55. doi: 10.1002/bies.20552
28. Walkiewicz MA, Stern M. Increased insulin/insulin growth factor signaling advances the onset of metamorphosis in Drosophila. PLoS ONE. (2009) 4:e5072. doi: 10.1371/journal.pone.0005072
29. Rewitz KF, Yamanaka N, O'Connor MB. Developmental checkpoints and feedback circuits time insect maturation. Curr Top Dev Biol. (2013) 103:1–33. doi: 10.1016/B978-0-12-385979-2.00001-0
30. Yamanaka N, Rewitz KF, O'Connor MB. Ecdysone control of developmental transitions: lessons from Drosophila research. Ann Rev Entomol. (2013) 58:497–516. doi: 10.1146/annurev-ento-120811-153608
31. Okamoto N, Yamanaka N, Yagi Y, Nishida Y, Kataoka H, O'Connor MB, et al. A fat body-derived IGF-like peptide regulates postfeeding growth in Drosophila. Dev Cell. (2009) 17:885–91. doi: 10.1016/j.devcel.2009.10.008
32. Zinke I, Kirchner C, Chao LC, Tetzlaff MT, Pankratz MJ. Suppression of food intake and growth by amino acids in Drosophila: the role of pumpless, a fat body expressed gene with homology to vertebrate glycine cleavage system. Development. (1999) 126:5275–84.
33. Dauwalder B, Tsujimoto S, Moss J, Mattox W. The Drosophila takeout gene is regulated by the somatic sex-determination pathway and affects male courtship behavior. Genes Dev. (2002) 16:2879–92. doi: 10.1101/gad.1010302
34. Hewes RS, Park D, Gauthier SA, Schaefer AM, Taghert PH. The bHLH protein Dimmed controls neuroendocrine cell differentiation in Drosophila. Development. (2003) 130:1771–81. doi: 10.1242/dev.00404
35. Post S, Liao S, Yamamoto R, Veenstra JA, Nässel DR, Tatar M. Drosophila insulin-like peptide dilp1 increases lifespan and glucagon-like Akh expression epistatic to dilp2. Aging Cell. (2019) 18:e12863. doi: 10.1111/acel.12863
36. Veenstra JA, Agricola HJ, Sellami A. Regulatory peptides in fruit fly midgut. Cell Tissue Res. (2008) 334:499–516. doi: 10.1007/s00441-008-0708-3
37. Söderberg JA, Birse RT, Nässel DR. Insulin production and signaling in renal tubules of Drosophila is under control of tachykinin-related peptide and regulates stress resistance. PLoS ONE. (2011) 6:e19866. doi: 10.1371/journal.pone.0019866
38. Agrawal N, Delanoue R, Mauri A, Basco D, Pasco M, Thorens B, et al. The Drosophila TNF eiger is an adipokine that acts on insulin-producing cells to mediate nutrient response. Cell Metabolism. (2016) 23:675–84. doi: 10.1016/j.cmet.2016.03.003
39. Ja WW, Carvalho GB, Mak EM, De La Rosa NN, Fang AY, Liong JC, et al. Prandiology of Drosophila and the CAFE assay. Proc Natl Acad Sci USA. (2007) 104:8253–6. doi: 10.1073/pnas.0702726104
40. Livak KJ, Schmittgen TD. Analysis of relative gene expression data using real-time quantitative PCR and the 2(-Delta Delta C(T)) method. Methods. (2001) 25:402–8. doi: 10.1006/meth.2001.1262
41. Kubrak OI, Lushchak OV, Zandawala M, Nässel DR. Systemic corazonin signalling modulates stress responses and metabolism in Drosophila. Open Biol. (2016) 6:160152. doi: 10.1098/rsob.160152
42. Bai H, Kang P, Tatar M. Drosophila insulin-like peptide-6 (dilp6) expression from fat body extends lifespan and represses secretion of Drosophila insulin-like peptide-2 from the brain. Aging Cell. (2012) 11:978–85. doi: 10.1111/acel.12000
43. Tennessen JM, Barry WE, Cox J, Thummel CS. Methods for studying metabolism in Drosophila. Methods. (2014) 68:105–15. doi: 10.1016/j.ymeth.2014.02.034
44. Diop SB, Birse RT, Bodmer R. High fat diet feeding and high throughput triacylglyceride assay in Drosophila melanogaster. J Vis Exp. (2017) 2017:56029. doi: 10.3791/56029
45. Lehmann P, Pruisscher P, Posledovich D, Carlsson MA, Käkelä R, Tang P, et al. Energy and lipid metabolism during direct and diapause development in a pierid butterfly. J Exp Biol. (2016) 219:3049–60. doi: 10.1242/jeb.142687
46. Lighton JRB. Measuring Metabolic Rates: A Manual for Scientists. New York, NY: Oxford University Press (2008). doi: 10.1093/acprof:oso/9780195310610.001.0001
48. Sokal RR, Rohlf FJ. Biometry, the Principles and Practice of Statistics in Biological Research. San Francisco, CA: W.H. Freeman and Company (2003).
49. Sondergaard L. Homology between the mammalian liver and the Drosophila fat-body. Trends Genet. (1993) 9:193. doi: 10.1016/0168-9525(93)90113-V
50. Baker KD, Thummel CS. Diabetic larvae and obese flies - emerging studies of metabolism in Drosophila. Cell Metab. (2007) 6:257–66. doi: 10.1016/j.cmet.2007.09.002
51. Li S, Yu X, Feng Q. Fat body biology in the last decade. Annu Rev Entomol. (2019) 64:315–33. doi: 10.1146/annurev-ento-011118-112007
52. Colombani J, Raisin S, Pantalacci S, Radimerski T, Montagne J, Leopold P. A nutrient sensor mechanism controls Drosophila growth. Cell. (2003) 114:739–49. doi: 10.1016/S0092-8674(03)00713-X
53. Tennessen JM, Thummel CS. Coordinating growth and maturation - insights from Drosophila. Curr Biol. (2011) 21:R750–7. doi: 10.1016/j.cub.2011.06.033
54. Padmanabha D, Baker KD. Drosophila gains traction as a repurposed tool to investigate metabolism. Trends Endocrinol Metabol. (2014) 25:518–27. doi: 10.1016/j.tem.2014.03.011
55. Nässel DR, Vanden Broeck J. Insulin/IGF signaling in Drosophila and other insects: factors that regulate production, release and post-release action of the insulin-like peptides. Cell Mol Life Sci. (2016) 73:271–90. doi: 10.1007/s00018-015-2063-3
56. Helmstadter M, Huber TB, Hermle T. Using the Drosophila nephrocyte to model podocyte function and disease. Front Pediatr. (2017) 5:262. doi: 10.3389/fped.2017.00262
57. Park D, Veenstra JA, Park JH, Taghert PH. Mapping peptidergic cells in Drosophila: where DIMM fits in. PLoS ONE. (2008) 3:e1896. doi: 10.1371/journal.pone.0001896
58. Arquier N, Geminard C, Bourouis M, Jarretou G, Honegger B, Paix A, et al. Drosophila ALS regulates growth and metabolism through functional interaction with insulin-like peptides. Cell Metab. (2008) 7:333–8. doi: 10.1016/j.cmet.2008.02.003
59. Honegger B, Galic M, Kohler K, Wittwer F, Brogiolo W, Hafen E, et al. Imp-L2, a putative homolog of vertebrate IGF-binding protein 7, counteracts insulin signaling in Drosophila and is essential for starvation resistance. J Biol. (2008) 7:10. doi: 10.1186/jbiol72
60. Okamoto N, Nakamori R, Murai T, Yamauchi Y, Masuda A, Nishimura T. A secreted decoy of InR antagonizes insulin/IGF signaling to restrict body growth in Drosophila. Genes Deve. (2013) 27:87–97. doi: 10.1101/gad.204479.112
61. Okamoto N, Yamanaka N. Nutrition-dependent control of insect development by insulin-like peptides. Curr Opin Insect Sci. (2015) 11:21–30. doi: 10.1016/j.cois.2015.08.001
62. Merkey A, Wong CK, Hoshizaki DK, Gibbs AG. Energetics of metamorphosis in Drosophila melanogaster. J Insect Physiol. (2011) 57:1437–45. doi: 10.1016/j.jinsphys.2011.07.013
63. Poulson DF. Oxygen consumption of Drosophila pupae. Zeitschrift für vergleichende Physiologie. (1935) 22:466–72. doi: 10.1007/BF00572720
64. Arrese EL, Soulages JL. Insect fat body: energy, metabolism, and regulation. Annu Rev Entomol. (2010) 55:207–25. doi: 10.1146/annurev-ento-112408-085356
65. Nelliot A, Bond N, Hoshizaki DK. Fat-body remodeling in Drosophila melanogaster. Genesis. (2006) 44:396–400. doi: 10.1002/dvg.20229
66. Aguila JR, Suszko J, Gibbs AG, Hoshizaki DK. The role of larval fat cells in adult Drosophila melanogaster. J Exp Biol. (2007) 210:956–63. doi: 10.1242/jeb.001586
67. Lee KS, You KH, Choo JK, Han YM, Yu K. Drosophila short neuropeptide F regulates food intake and body size. J Biol Chem. (2004) 279:50781–9. doi: 10.1074/jbc.M407842200
68. Zheng H, Yang X, Xi Y. Fat body remodeling and homeostasis control in Drosophila. Life Sci. (2016) 167:22–31. doi: 10.1016/j.lfs.2016.10.019
69. Hwangbo DS, Gershman B, Tu MP, Palmer M, Tatar M. Drosophila dFOXO controls lifespan and regulates insulin signalling in brain and fat body. Nature. (2004) 429:562–6. doi: 10.1038/nature02549
70. Slack C, Werz C, Wieser D, Alic N, Foley A, Stocker H, et al. Regulation of lifespan, metabolism, and stress responses by the Drosophila SH2B protein, Lnk. PLoS Genet. (2010) 6:e1000881. doi: 10.1371/journal.pgen.1000881
71. Brown JB, Boley N, Eisman R, May GE, Stoiber MH, Duff MO, et al. Diversity and dynamics of the Drosophila transcriptome. Nature. (2014) 512:393. doi: 10.1038/nature12962
72. Kubrak OI, Kucerova L, Theopold U, Nässel DR. The sleeping beauty: how reproductive diapause affects hormone signaling, metabolism, immune response and somatic maintenance in Drosophila melanogaster. PLoS ONE. (2014) 9:e113051. doi: 10.1371/journal.pone.0113051
73. O'Brien DM, Min K-J, Larsen T, Tatar M. Use of stable isotopes to examine how dietary restriction extends Drosophila lifespan. Curr Biol. (2008) 18:R155–6. doi: 10.1016/j.cub.2008.01.021
Keywords: insulin signaling, development, metabolism, respirometry, stress responses, adult tissue growth
Citation: Liao S, Post S, Lehmann P, Veenstra JA, Tatar M and Nässel DR (2020) Regulatory Roles of Drosophila Insulin-Like Peptide 1 (DILP1) in Metabolism Differ in Pupal and Adult Stages. Front. Endocrinol. 11:180. doi: 10.3389/fendo.2020.00180
Received: 27 January 2020; Accepted: 13 March 2020;
Published: 21 April 2020.
Edited by:
Ian Orchard, University of Toronto Mississauga, CanadaReviewed by:
Mark R. Brown, University of Georgia, United StatesNaoki Yamanaka, University of California, Riverside, United States
Copyright © 2020 Liao, Post, Lehmann, Veenstra, Tatar and Nässel. This is an open-access article distributed under the terms of the Creative Commons Attribution License (CC BY). The use, distribution or reproduction in other forums is permitted, provided the original author(s) and the copyright owner(s) are credited and that the original publication in this journal is cited, in accordance with accepted academic practice. No use, distribution or reproduction is permitted which does not comply with these terms.
*Correspondence: Dick R. Nässel, ZG5hc3NlbCYjeDAwMDQwO3pvb2xvZ2kuc3Uuc2U=
†ORCID: Sifang Liao orcid.org/0000-0003-2828-6891
Stephanie Post orcid.org/0000-0003-2354-736X
Philipp Lehmann orcid.org/0000-0001-8344-6830
Jan A. Veenstra orcid.org/0000-0002-2783-0018
Marc Tatar orcid.org/0000-0003-3232-6884
Dick R. Nässel orcid.org/0000-0002-1147-7766