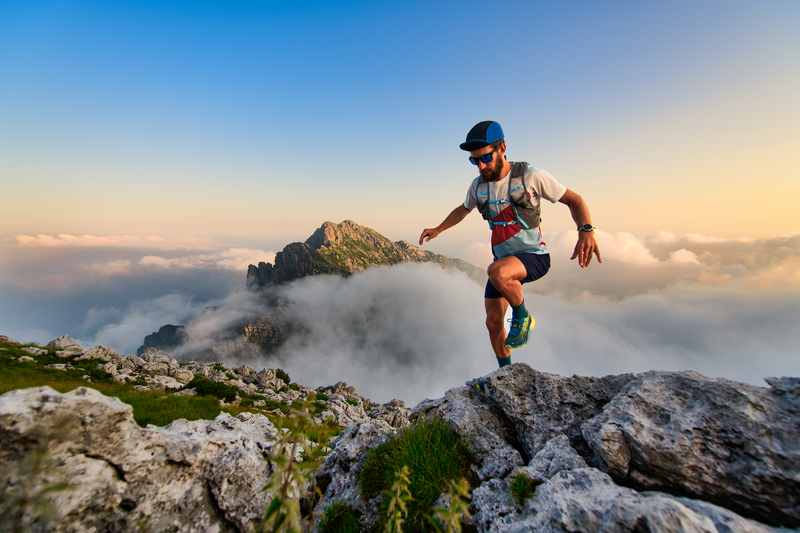
94% of researchers rate our articles as excellent or good
Learn more about the work of our research integrity team to safeguard the quality of each article we publish.
Find out more
REVIEW article
Front. Endocrinol. , 21 February 2020
Sec. Clinical Diabetes
Volume 11 - 2020 | https://doi.org/10.3389/fendo.2020.00057
This article is part of the Research Topic The Role of Hypoxia in the Regulation of Metabolism View all 14 articles
Hypoxia is characterized as insufficient oxygen delivery to tissues and cells in the body and is prevalent in many human physiology processes and diseases. Thus, it is an attractive state to experimentally study to understand its inner mechanisms as well as to develop and test therapies against pathological conditions related to hypoxia. Animal models in vivo fail to recapitulate some of the key hallmarks of human physiology, which leads to human cell cultures; however, they are prone to bias, namely when pericellular oxygen concentration (partial pressure) does not respect oxygen dynamics in vivo. A search of the current literature on the topic revealed this was the case for many original studies pertaining to experimental models of hypoxia in vitro. Therefore, in this review, we present evidence mandating for the close control of oxygen levels in cell culture models of hypoxia. First, we discuss the basic physical laws required for understanding the oxygen dynamics in vitro, most notably the limited diffusion through a liquid medium that hampers the oxygenation of cells in conventional cultures. We then summarize up-to-date knowledge of techniques that help standardize the culture environment in a replicable fashion by increasing oxygen delivery to the cells and measuring pericellular levels. We also discuss how these tools may be applied to model both constant and intermittent hypoxia in a physiologically relevant manner, considering known values of partial pressure of tissue normoxia and hypoxia in vivo, compared to conventional cultures incubated at rigid oxygen pressure. Attention is given to the potential influence of three-dimensional tissue cultures and hypercapnia management on these models. Finally, we discuss the implications of these concepts for cell cultures, which try to emulate tissue normoxia, and conclude that the maintenance of precise oxygen levels is important in any cell culture setting.
Oxygen first began to significantly accumulate in the Earth's atmosphere with the advent of photosynthesis, a process enabling the ancestors of cyanobacteria to obtain hydrogen from water and combine it with atmospheric CO2 to produce hydrocarbon molecules (1, 2). Subsequently, most of contemporary life was presumably exterminated, having no line of defense against reactive oxygen species, in a process that has sometimes been labeled as the “oxygen holocaust.” However, conditions were ideal for the evolution of oxygen-consuming organisms who could take advantage of more energy-efficient aerobic metabolism. Oxygen thus became a necessary molecule that enabled the life of eukaryotic organisms including humans, because they acquire energy by oxidative phosphorylation where oxygen serves as the ultimate acceptor of electrons (3).
The universal oxygen demand in complex organisms created the requirement for an effective system that distributed oxygen into the entire body and satisfied the metabolic requirements of all tissues (3). Diffusion in the lungs and peripheral tissues is the key process in the transport of oxygen to the mitochondria; therefore, concentration gradients have developed across the human body leading to variable tissue O2 levels in different organs (4). Importantly, anaerobic metabolism has not been entirely forgotten by eukaryotic cells. In fact, some cells, such as erythrocytes, rely completely on anaerobic phosphorylation, whereas others resort to such means during diminished oxygen supply (i.e., hypoxia), for example, during intensive exercise (5, 6). Similarly, a systemic response of the entire body to high altitude is triggered by hypoxia (7, 8). Hypoxia is an integral part of the pathophysiology of many diseases, including chronic obstructive pulmonary disease (9), heart failure (10, 11), sleep apnea syndrome (12), anemia (13), and cancer, and its basic research can reveal mechanisms that may 1 day be exploited in therapy development (14).
In vivo models of hypoxia face considerable shortcomings (15); therefore, cell cultures represent a viable option for this line of research. However, the importance of the precise modulation and definition of hypoxia is often not reflected in the design of in vitro experiments, due to historical reasons (16) and technical limitations (17–19). However, with the advancements in various scientific fields, including cell biology and material science, the requirement for adequate control of pericellular oxygen levels in the experimental setup increases in importance, particularly as technological solutions become more readily available.
In this review, we aimed to summarize the current approaches in experimental hypoxia research with special emphasis on cell culture models. The topics covered include the physical limitation of gas diffusion in liquids, methods of inducing sustained and intermittent pericellular hypoxia, and measurements of dissolved oxygen. We also discuss the physiological relevance of mimicking the oxygen dynamics of certain diseases in cell cultures as closely as possible and the implications of the mentioned principles on in vitro models mimicking tissue normoxia.
Humans as well as animals can be exposed to hypobaric (HH) or normobaric hypoxia (NH) in order to study wide variety of diseases, including pulmonary hypertension (20), reoxygenation injury (21), pre-eclampsia (22), hypoxic insult of the brain (23), and diabetic retinopathy (24). While HH, which physically resembles a high-altitude environment, is induced by decreasing atmospheric pressure under 101 325 Pa (1 atm, 760 mm hg) typically in a tightly sealed hypobaric chamber (25), NH exposure is based on the reduction of the partial pressure of oxygen (pO2) at normal atmospheric pressure, which typically occurs through the administration of nitrogen to a face mask (26), hypoxic tent (27), or environmental chamber (22, 23).
It remains debatable as to whether the two hypoxic states are interchangeable under experimental settings (8). Several differences have been observed by multiple studies, such as in minute ventilation, tidal volume, peripheral O2 saturation, arterial CO2 pressure, and exhaled NO levels, which appear to be lower and acute mountain sickness symptoms more pronounced in HH However, these symptoms as well as minute ventilation only differ during the acute phase of hypoxia, possibly due to the initial difference between alveolar and ambient N2 tension in HH, whereas long-term effects of both states are comparable. Other parameters such as arterial pressure of O2 and CO2 have been found to be either similar or variable depending on the study (7, 8, 28). Biochemical markers of hypoxia have been measured and found to be equivalent during exercise in NH and HH, with both conditions being different from exercise in a normoxic environment (29).
Ethical problems as formulated by the “3R's” rule, cost-related issues, and limited reproducibility in humans remain the most apparent hurdles of animal model applications (15). Human cell cultures represent a compelling alternative in important areas of biomedical research, such as drug discovery (30) or disease modeling (31), largely due to important advancements in pluripotent stem cell applications over the past two decades (32, 33).
Recent advancements in tissue engineering have enabled researchers to perform in vitro experiments not only at the cellular and molecular levels, but also to explore inter-cell and inter-organ interactions using three-dimensional (3D) cell models and complex organoids (34, 35). Nevertheless, significant variability in laboratory-to-laboratory protocols and procedures hamper reproducibility and impose challenges for interpretation and generalizability of results. Despite numerous factors, including cell confluency, composition of culture media, and frequency of media exchange typically reported in method descriptions, a fundamental factor for cell life—pericellular oxygen level—remains largely overlooked. An accumulating body of literature suggests that oxygen levels in standard cell culture experiments not only significantly deviate from a physiological range, but also shows that pericellular oxygen levels vary dramatically under different experimental settings, cell types investigated, cell confluency, and volume and timing of media exchange (17, 18, 36). The key determinants of pericellular oxygen levels and possible means of their control in cell cultures are summarized in the following text.
In a standard cell culture experiment, cells are kept in incubators that maintain the following stable conditions: temperature of 37°C, atmospheric air (21% volume fraction of O2) enriched by 5% CO2, and humidity provided by spontaneous water evaporation (37). The volume fraction of oxygen in the incubator atmosphere reaches 18.6% (132.5 mmHg) because of the addition of partial pressures of CO2 and water vapor, as described by Dalton's and Amagat's laws (38). Thus, 18.6% O2 and its corresponding partial pressure in the incubator is what many would consider as a conventional, standard, or “normoxic” setup (36). Two important limitations of such a paradigm must be addressed. First, pericellular oxygen levels are dramatically different from oxygen levels in the incubator atmosphere, as discussed below. Second, a physiological range of oxygen levels observed in tissues in vivo (tissue normoxia or physioxia) is profoundly variable and significantly lower, as discussed in section Hypoxia Mimetics. In fact, physiological alveolar partial pressure falls below that of the incubator oxygen level, following the alveolar gas equation (39).
The first concerns about the possibility of limited pericellular pO2 were voiced over a century ago (40), with the first confirmations of pericellular hypoxia reported during the early years of conventional cell culture experiments (17, 41). Metzen et al. (17) showed that under common normoxic conditions as described above, adherent cells may suffer from pericellular hypoxia or even anoxia. When measuring pericellular O2 levels 24 h after media exchange, it was found that the cell lines with a high oxygen demand (e.g., human hepatoma Hep3B and HepG2, and renal epithelial LLC-PK1 and LLC-MK2 cell lines) eventually reached an anoxic state. These authors developed mathematical model based on Fick's law calculating expected pericellular O2 levels, which were subsequently verified by real-life measurements.
It has since been acknowledged that in conventional cultureware, the only way for oxygen to reach the adherent cells is by diffusion through the water-based medium overlay. Moreover, if the oxygen consumption rate of cells that exhibit higher metabolic activity exceeds the speed of oxygen delivery (determined by the oxygen solubility coefficient, diffusion constant, medium overlay height, surface area, and partial pressure of oxygen above the medium), the pericellular oxygen pressure eventually equilibrates at a hypoxic or anoxic value after 2 h following medium exchange (17). However, oscillations of pericellular O2 tensions around the equilibrated state are also known to occur. These periodical changes likely occur because of a decrease in the respiratory rate following the depletion of oxygen around the cells and, as its availability begins to increase again, oxygen consumption increases as well, exhausting its supply and completing the cycle. It has also been proposed that these oscillations are what ultimately drive the molecular response to hypoxia (36).
Cell surface area and pO2 are characterized by the dimensions of the culture dish and the 5% CO2 incubator atmosphere. Thus, the medium overlay height represents the main variable that limits oxygen diffusion (17). The medium height and cell oxygen consumption rate both determine pericellular oxygen concentration, and thus significantly affect contemporary cell culture research owing to the lack of standardization (reporting) of media amount supplied to cells and the attention given to the differences in oxygen demand of different cell lines under various experimental conditions (17, 36).
An existing discrepancy in current terminology must be discussed here. Namely, there is a lack of consensus in the usage of terms and units when it comes to measurements of pericellular O2 levels. Many researchers describe the pericellular oxygen availability as concentration given in percent (18, 19, 42–48). However, this is not accurate from the physical point of view as these studies are actually referring to the volume fraction of oxygen in the ambient air that corresponds to the actual molar concentration of oxygen in an aqueous solution—the medium. Based on Henry's and Amagat's laws, the molar concentration is determined by the volume fraction of oxygen in the air and the atmospheric pressure, which can be summarized as the pO2 (38, 49, 50). Therefore, should the atmospheric pressure decrease with an increasing altitude, the actual amount of oxygen in the cell culture medium would also change accordingly.
Such disparities affect not only standard cell culture where no attention is given to the pericellular pO2, but they also make it difficult to unify the results of published material on in vitro hypoxia measurements. We could hypothesize that authors using the term oxygen concentration refer to atmospheric pressure at sea level; however, as they usually do not elaborate sufficiently enough on how they derived their concentration values from pericellular pO2 measurements, we cannot be certain of this. Therefore, we are unable to precisely calculate pO2 and/or molar concentrations of these experiments and directly compare them to the units used in other studies.
Therefore, we propose that this terminology should not be used in future to avoid further confusion. Instead, the values of molar concentration of oxygen (51) or oxygen partial pressure, which can easily be converted to one another under constant temperature following the ideal gas equation (36) or Henry's law (49, 50), should be used henceforth to promote reproducibility and intelligibility of the results. In fact, O2 partial pressure is also a non-sensical term to use when describing the concentration of a gas in a liquid, which only corresponds to partial pressure of a gaseous phase (49, 50). However, it has become the most widespread way of characterizing oxygenation in both medical physiology and clinical practice because the majority of oxygen in the blood is not dissolved, but is transported while bound to hemoglobin (50).
Before moving on to removing the issue represented by limited oxygen supply to cells in vitro, one must first be able to characterize the oxygenation of the cells properly. One way of doing this is using a polarographic O2-sensitive electrode, named the Clark electrode after its discoverer (52). Based on the principle of electrolytical reduction of oxygen, this electrode allows for the measurement of oxygen levels at a precise location in a pericellular area. However, manipulating the electrode can also disrupt the cells/samples. Due to its construction, it does not enable for simultaneous detection of O2 levels in all dimensions, which prevents it from measuring the concentration gradients in 3D cultures or inhomogeneities in the microenvironment of the cells (18). Furthermore, the electrode itself has non-negligible O2 consumption, which must be considered during prolonged experiments, as it requires re-calibration or stirring of the medium (Figure 1A) (4, 17, 52). To overcome these limitations, alternative and complementary methods have been developed to monitor pericellular O2 levels.
Figure 1. Schematic drawing of pericellular pO2 measurement. (A) Clark electrode immersed in media, enabling measurement of pO2 at any depth including pericellular area of adherent culture. Adapted with permission according to (18). (B) Optical pO2 sensor based on quenching of fluorescence by oxygen may be positioned as a thin film on the bottom of cultureware surface. To perform measurement, a fluorescent excitation source is placed below the cultureware. Strong emission signal is registered from areas with low pO2, while oxygen-mediated quenching results in limited emission from oxygen-rich regions. Adapted with permission according to (53).
Certain tissue dyes such as Hypoxyprobe (pimonidazole hydrochloride) coupled with monoclonal antibodies are provided only with semi-quantitative assessments of hypoxia (4). Moreover, Hypoxyprobe is generally designed for use in patients and animal models to observe hypoxia of an explanted tissue (54, 55), although sporadic use in vitro has also been reported (56). Nevertheless, there is another staining method that yields exact values of pO2, which is based on oxygen-mediated quenching of the fluorescent signal that is inversely proportionate to pO2 (57), the intensity of which is quantifiable by a microscope (58) or commercially available devices (59). This principle can also be implemented using dishes with pre-calibrated oxygen sensors positioned at the bottom of each well (Figure 1B) (46, 53). Custom-made amperometric electrodes, which utilize ion current quantification to assess analyte concentration, may also be integrated to the cell culture system in a similar fashion (47).
Motivated by the ability to monitor pericellular pO2, as demonstrated above, investigators developed multiple approaches that enabled O2 control at the cell level throughout experiments. The most straightforward option was the empirical adjustment of air composition in the incubator based on the measurement of oxygen tension around the cells, which has been used repeatedly owing to its technical ease (44, 48, 60, 61). However, it has been demonstrated that in metabolically active cells (17), pericellular O2 reaches extremely low levels owing to a mismatch between cellular oxygen consumption and the amount of O2 delivery by diffusion through the culture media. Early attempts to tackle the limitation of diffusion were rather simple and aimed to drastically reduce the height of the medium above the cells. Unfortunately, the amount of medium is crucial for keeping the cells well-provisioned with nutrients and for maintaining a stable environment. Hence, this method is suboptimal for prolonged cell culture. Additionally, the meniscus of the medium forming within the culture well-causes a significant difference in the diffusion distance across the culture surface, which requires maintaining the cells in the middle of the well (62), which is difficult to achieve when using cell lines with significant proliferative capacity. Alternatively, researchers used stirring or shaking of the culture vessel as a simple method to increase oxygen diffusion (36), although this was at the cost of inducing mechanical stress to the cells (63).
To overcome the above-mentioned limitations, placement of a commercially available culture dish with a gas-permeable bottom made of a fluorocarbon membrane in a modular incubator chamber (an air-tight sealed plastic chamber) filled with atmosphere-containing predetermined O2 and CO2 levels has been employed (45, 64). Using this setup, adherent cells receive O2 directly from the modular incubator chamber atmosphere via the permeable membrane without having to rely on diffusion through the medium, which has been shown to be both effective and simple in terms of being able to regulate pericellular pO2 closely with relatively fast equilibration times (45, 65). Additional advantages of modular incubator chambers compared to standard incubators (with or without control of O2 levels) include the elimination of gas leaks (changing oxygen levels) and the minimization of convective forces associated with incubator openings (66).
While reaching and maintaining sustained pericellular O2 levels in vitro is feasible via the methods described in the previous section, a much greater challenge lies in developing a system that provides researchers with a means of modeling IH, where precise cyclic control of pericellular oxygen tension as well as its fast equilibration is mandatory. OSA syndrome represents one of the most blatant examples because the cycles of IH occur as often as 60 times per hour (67). This dictates the need to create a system in which pericellular pO2 would change every minute, while achieving equilibrium with the gas phase during each period.
Mere fast-paced changes (within minutes) in headspace O2 levels inside a modular incubator chamber cannot achieve the desired pericellular pO2 as the equilibration of oxygen levels across media takes a significantly longer time, depending on the thickness of the medium overlay. For instance, a 170 μm-thick media barrier between a human osteosarcoma cell line and the incubator atmosphere allows for a 1.5 min long equilibration, whereas this time increases 10-fold if the overlay height is a mere 1 mm (68). Perforation of a culture plate lid has been shown to speed up the equilibration between the pericellular and headspace pO2, suggesting that this barrier is also important in slowing down the diffusion of O2 toward adherent cells (19).
Multiple models for IH in vitro that meet the requirements of a more dynamic approach have been developed and validated. For instance, cyclic changes of gas mixtures flowing directly into cell culture flasks (Figure 2A) (69) or microfluidic devices capable of directly supplying cells with precise gas mixtures via a system of miniature channels (72–74) have been used. Other perfusion-based systems rely on growing the cells directly on the walls of tube-like channels, through which medium is pushed (Figure 2B) (70). Alternatively, bioreactors containing peristaltic pumps that drive the hypoxic and normoxic media to the cells in a cyclical manner from reservoirs pretreated by bubbling the desired gas mixtures through the liquid may be employed (Figure 2C) (71, 75). A shared downside of perfusion-based approaches is the shear stress being exerted on the cells during perfusion with the equilibrated medium and/or gases. To minimize this problem, a polydimethylsiloxane (PDMS) microfluidic chip consisting of a chamber through which oxygen-rich and oxygen-poor air is pumped in a periodic fashion has been constructed. Unlike in other microfluidic settings, cells are not in the chamber itself but rather are in direct contact with it by growing on a gas-permeable membrane, which allows for close control and fast equilibration of pericellular O2, while diminishing mechanical stress. Furthermore, the modulation of pressure in the chamber mediates a cyclical stretching of the cell culture that simulates the periodic expansion of the heart or lung (Figure 2D) (63). Using a similar principle, the development of a PDMS pillar with an oxygen perfusion channel, coated with Parylene-C to ensure better oxygen isolation and faster equilibration, has been reported. The pillar can be mounted on the top of each well of a common culture dish (Figure 2E) (58, 68). It then streamlines a precisely defined gas mixture in very close vicinity of the cellular monolayer, bypassing most of the culture medium diffusion barrier. Cells are isolated from the apparatus itself; therefore, no shear force is present (58). Unlike the microfluid approach (63), this method is well-suited for culture plates using standard-sized wells as it does not have to cope with the limited space of miniaturized chambers inside a chip.
Figure 2. Schematic drawing of flow-through systems for in vitro intermittent hypoxia. (A) Administration of desired gas mixtures directly into a cell culture flask. Cyclic change of hypoxic and normoxic atmosphere is ensured by a solenoid valve. Adapted with permission according to (69). (B) Perfusion-based system with cells growing on the wall of a capillary tube. Pre-warmed medium is first divided into two circuits, which are treated with both hypoxic and normoxic gas mixtures, respectively. Both circuits then alternately open into the capillary area seeded with cells by passing through a valve. Adapted with permission according to (70). (C) Bioreactor based on cyclic perfusion of cells with hypoxic and normoxic medium prepared by bubbling with gas. Both hypoxic and normoxic circuit has its own pump, pushing the medium in and out of a culture dish through a periodically-opening valve. Adapted with permission according to (71). (D) Microchip for intermittent hypoxia coupled with cyclic stretch to cell cultures. Varying gas mixtures are pushed into a well at the center of the microchip via a valve or a gas blender, flowing out of the well-through a separate gas outlet tube. A venting tube connected to the well-leads to a solenoid valve (not shown), which serves the purpose of periodically changing pressure inside the well, applying indirect mechanical stimuli to cells growing on the outside of a gas-permeable membrane of the well. Adapted according to (63) under the CC BY license. (E) Cell culture insert for intermittent hypoxia. A pillar fixated on the lid of a cell culture plate with integrated channel for gas perfusion is immersed in medium in order to reach close vicinity of pericellular area. The desired gas is then delivered to cells via the channel and a gas-permeable membrane as the thickness of the diffusion barrier represented by the medium may be limited down to 170 μm. Adapted with permission according to (68).
Growing cells on commercially available cultureware dishes, fitted with gas-permeable fluorocarbon membrane, may be used not only for maintenance of sustained hypoxia (as mentioned above), but also for efficient recreation of intermittent hypoxia. Enclosing such a culture dish in a sealed cabinet while controlling O2 levels, e.g., by a programmable digital controller, enables for rapid and reproducible exposure of cells to intermittent hypoxia, without the need for diffusion through a culture medium (Figure 3A) (45, 65). The limitations of such an approach include the large volume of gases (O2, N2, and CO2) required to achieve the rapid exchange of the inner cabinet atmosphere associated with significant culture media evaporation (despite humidification) and gas pressure changes inside the cabinet or inside the sealed culture dishes due to heat expansion of gas (45), all of which adversely affect the performance of IH exposure. Minoves et al. (64) modified the setup by combining the gas permeable culture dishes with a customized plate-holder equipped with its own gas tubing. This was designed to seal the plate off from the surrounding atmosphere, replacing the hypoxic chamber with a significantly smaller space, and thus limiting the volume of air to be pumped in and out during each cycle (Figure 3B).
Figure 3. Schematic drawing of membrane-bottom systems for in vitro intermittent hypoxia. (A) Periodic change of pO2 in a cell culture cabinet is appropriately reflected only in the pericellular area of a gas-permeable membrane cultureware, which allows for unlimited diffusion of oxygen and fast equilibration times, whereas standard plastic dishes only enable diffusion through the medium overlay, posing as a barrier. Adapted with permission according to (45). (B) Custom-made plateholders of a volume no more than 20 ml connected to gas-permeable bottom dishes in an air tight fashion serve the purpose of limiting the volume of gas mixtures that need to be periodically exchanged during intermittent hypoxia regimes. The required gas mixtures are prepared and pre-warmed in a gas blender, brought into the plate holder by gas inlet tubing, allowing for unlimited diffusion of oxygen between the plate holder and adherent cells, and subsequently flushed out through a gas outlet tube to make room for a fresh gas mixture. Adapted with permission according to (64).
Instead of exercising control over oxygen availability, some in vitro as well as in vivo models utilize hypoxia mimetic agents which simulate hypoxic conditions predominantly by increasing availability of intrinsic HIF-1α in standard cell culture settings. This methodology can be used for both sustained and intermittent hypoxia models, the latter of which can be achieved by cyclic exposure to the agent (76, 77).
Precise mechanism of action of hypoxia mimetic agents may vary depending on the particular agent used. Many of the compounds inhibit HIF-prolyl hydroxylases (PHDs), which are crucial for HIF-1α degradation. Cobalt chloride (CoCl2), arguably the most widely used hypoxia mimetic, competes with Fe2+ ions, which are necessary for enzymatic activity of PHDs. Iron chelators, such as deferoxamine mesylate (DFO) work by similar means. Dimethyloxalylglycine (DMOG) is a 2-oxoglutarate analog, which also inhibits PHDs and may be utilized in hypoxic cell culture models (78–81). However, one of the downsides to the most common PHD inhibitors is their cytotoxicity. To overcome this problem, another PHD inhibitor hydralazine has been successfully employed to mimic hypoxic conditions and proved to be significantly less cytotoxic than CoCl2 (82).
Nevertheless, other mechanisms of action, such as mitochondrial uncoupling in case of bafilomycin A1 have been exploited in cell culture models of hypoxia (83). Inhibition of proteasome degradation, miRNA approaches and application of isoflurane or N-acetyl cysteine also have HIF-1α stabilizing effects, but these methods are predominantly utilized to ameliorate ischemia-reperfusion injury rather than to mimic hypoxia (80, 81).
To realize the importance of tissue normoxia for hypoxic cellular models and its distinction from hypoxia, one must first understand that the use and definition of the terms “normoxia,” “hypoxia,” and “hyperoxia” are somewhat arbitrary in cell culture literature as the composition of headspace gas and not the actual pericellular microenvironment is typically considered. Hypoxia is usually defined as the insufficient supply of oxygen to the relevant tissue, although several other definitions have been proposed. These definitions revolve around the state of mitochondrial respiration and temporal dynamics of the molecular apparatus that are centered around HIF-1α (84).
Different types of tissues, however, have various oxygen demands (85) and variable capillary network and blood flow regulation, resulting in largely different tissue pO2 in vivo. Varying pO2 in different body organs in humans have been comprehensively reviewed by others (4). The unique pO2 of each organ, called physioxia or tissue normoxia, warrants more elaborate experimental settings, ideally mimicking such tissue-specific physioxia in vitro. Clearly, considering the usual environment of an incubator as “normoxic” represents a failure to recapitulate basic physiological parameters. In fact, a standard incubator atmosphere (18.6% O2) might induce severely “hyperoxic” conditions in some cultured cells and nearly anoxic pericellular oxygen levels in other cultured cells—all fundamentally deviating from physiological oxygen levels observed in tissues (4, 84). Any particular in vitro model of hypoxia should thus aim to reach oxygen levels lower than tissue normoxia (physioxia). Ideally, oxygen tensions present in the tissue or disease in vivo should be implemented if the exact values are known.
For example, pO2 in human adipose tissue has been found to be approximately 55 mmHg, but lower pO2 levels have been measured in the subcutaneous fat of obese subjects with a possible link to inflammation of the tissue (86), and thus also the pathophysiology of type 2 diabetes mellitus (87). It has also been reported that adipose tissue-derived stromal cells retain their natural phenotype when the O2 levels of their physiological niche are maintained (88). Similarly, pO2 in fetal arterial circulation, as opposed to adults, equals approximately 30 mmHg (89), whereas that of a trophoblast is slightly higher, 40–60 mmHg (48). The ideal pO2 for early stage human embryonic development presented in vivo and utilized by in vitro fertilization laboratories appears to be in the range from 2% (~15 mmHg) to 5% (~38 mmHg) tension of oxygen (90). This knowledge has been exploited in studies of embryonic and induced pluripotent stem cells as the quality of pluripotent stem cell culture characterized by proliferative capacity and expression of pluripotency markers is significantly improved when the cells are grown in incubator atmosphere commonly described in literature as hypoxic, ranging from 1 to 10%, while higher O2 concentrations referred to as normoxic showed to be detrimental (91–98).
Finally, tissue pO2 has been measured and found to be significantly reduced in the majority of tumors in patients. Knowing these values is particularly important because the properties of cancer cells, such as sensitivity to chemotherapeutic agents, change dramatically under hypoxic conditions (4). Modified tissue pO2 has also been observed and recorded in myocardial infarction (99), retinopathy (100), and pre-eclampsia (101), and to a limited extent also in OSA (102, 103).
The need to tailor experimental conditions of in vitro hypoxia, namely O2 levels, to meet those found in living patients is further purported by mechanisms occurring in hypoxia at cellular and molecular levels, many of which are directly involved in disease pathophysiology. These predominantly include the upregulation of HIF-1α (104), nuclear factor-kappa B (NF-κB) (105, 106), and reactive oxygen species (ROS); the decreased availability of nitric oxide (107); or complex changes in ion channel activity (108). It has been shown multiple times in both cell culture and in vivo that these processes are very tightly governed by O2 concentration (109–112). Namely, HIF-1 expression, which is a central molecule in cellular signaling during hypoxia, increases exponentially as oxygen tension decreases (113). Using gas-permeable plates that ensure close control of pericellular pO2 cycles for IH has found that different pO2 levels around the cells and its dynamics significantly vary in their effect on HIF and NF-κB expression (45). This would explain the contradictory results reported by different studies employing varying modes of hypoxia induction when exploring its influence on HIF mechanisms (69, 75).
The influence of pericellular pO2 measurement and control can be shown by the example of tumor hypoxia. The in vivo tumor microenvironment is characterized by unique oxygen tension values, which may have a considerable influence on clinical treatment, influencing the efficacy of anti-cancer drugs and radiotherapy (4). In fact, in vitro hypoxia has already been utilized to simulate the effect of oxygenation dynamics on breast cancer radiosensitivity, which was found to be diminished in hypoxia, taking advantage of the ability to continuously measure pericellular pO2 (114). The cancer cell culture model has also been employed to develop a new hypoxic probe, which accumulated inside tumors in vivo as well as in vitro, which implies that this culture condition might prove to be a useful tool in drug testing (115). To this end, a microfluidic chip, capable of creating pO2 gradients and evoking multiple oxygenation states, has been developed (116). A myriad of other microfluidic devices could also be devised for the purpose of accurately recreating tumor phenotypes in a dish (63, 117).
In vitro hypoxia may also be studied to uncover the molecular mechanisms ameliorating ischemia/reperfusion injury in neurons (118) or cardiomyocytes (119). A perfusion-based model of murine cardiomyocytes subjected to abrupt anoxia and reperfusion was discovered to be an optimal platform for demonstrating the opening of mitochondrial permeability transition pores (mPTP). Because mPTP is a protein complex in mitochondria activated during ischemia-reperfusion injury leading to cell death, this model could be utilized to study its molecular nature, which still has not been fully elucidated, and eventually to develop a pharmacological approach to block it (119). In addition, a steady perfusion-based microfluidic system has been developed to continuously monitor the effects of hypoxic insults on the electrophysiological properties of cardiomyocytes. At the hypoxic level, which is translatable to a 5% oxygen concentration, L-type calcium currents were decreased that corresponded to in vivo observations and the stunned myocardium hypothesis (120).
The significance of precise pO2 maintenance also applies to IH modeling. A recent study explored the effect of IH in OSA on insulin resistance and the results from the in vitro model, which utilized gas-permeable dishes and OSA pathophysiology, were in accord with the animal model and patient cohort observations, including changes in NF-κB modulation (65). Moreover, adipocytes grown on the same type of cultureware and that were subjected to clinically-relevant IH exhibited an accumulation of triglycerides, which correlates with the observed link between obesity and OSA in patients (42). Conversely, culturing adipocytes in suboptimal settings without pericellular pO2 monitoring has led to conflicting results on whether hypoxia increases HIF expression (87, 121, 122).
Similar to adipocytes, when other cell types underwent a protocol of IH with defined pO2 in vitro, the results were consistent with other IH models. For instance, gene expression profiles of neutrophils, monocytes, and airway epithelial cells all matched the results found in OSA patients, further hinting at the role of inflammation in the pathophysiology of the disease (123–126). Constant monitoring of pO2 of the PC12 cell line confirmed the central role of HIF-1α in the molecular response to IH (127). The same molecule was found to be upregulated in skin vasculature taken from the biopsies of OSA patients as well as in the aortas of mice and human cultures of coronary artery endothelial cells, where IH was maintained by gas bubbling in the medium (128). Additionally, a protective mechanism of pancreatic cells exposed to IH and hyperglycemia based on ROS reduction both in vivo and in vitro has been described (129).
Furthermore, a myocardial ischemia model was adopted using gas-permeable culture dishes to study the effect of different hypoxic modalities. Continuous measurements of pericellular pO2 showed that IH, simulating repeating cycles of ischemia and reperfusion, OSA, or several pulmonary conditions resulted in a considerably more pronounced inflammatory response and cell injury than that of mild hypoxia, comparable to or at earlier stages even greater than that of severe hypoxia. This is in agreeance with the fact that OSA is considered to be an independent risk factor of cardiovascular disorders (130). The addition of cyclic stretching mimicking heart and/or lung movements was demonstrated to act synergistically with IH, upregulating the HIF-1α pathway in mesenchymal stem cells, and showing that this model could be superior to others when simulating IH in these organs (63).
Discrepancies in the results of the role that hypoxia has on cell cultures could be attributed to a number of variables, such as using different cell types (69) or species (87). Nevertheless, if the native pO2 of the tissue type in question as well as its temporal development in the disease being studied were to be respected and incorporated into the in vitro model as suggested (4), variability in results could arguably be reduced.
Despite this, many recent publications pertaining to in vitro hypoxia still implement the simplified model, which does not consider the difference between headspace and pericellular O2 tensions. Such studies encompass various areas of hypoxic research, ranging from IH (131–133) to the tumor microenvironment (134, 135) to reperfusion injury modeling. In regards to the latter, placing cells into anoxic conditions generally reflects the ischemic insult occurring in vivo (132, 136). Notwithstanding, the absence of pericellular O2 measurements makes it virtually impossible to ascertain whether there is a higher concentration of oxygen in the control group and by what margin. This makes any interpretation of the results complicated, especially because the cell cultures used in these studies for their sensitivity to ischemic hypoxic injury, such as cardiomyocytes (132), neurons (137), kidney (138), and endothelial cells (139), tend to have relatively high oxygen consumption rates (85). Moreover, these studies generally do not include information about the height of the medium overlay above the adherent cell culture, which introduces yet another unknown variable that possibly affects the results and reproducibility (17, 18). Furthermore, certain studies employed only chemical insults to mimic hypoxia (134, 140–142). Logically, some studies then report that cell cultures have no merit in this area of hypoxia research (139), while others state that the results gained from these models are in line with studies in vivo (136, 143). This highlights the importance of maintaining strict conditions for in vitro hypoxia characterizing the experimental setup in detail, including pericellular pO2 values and, in case of IH, pO2 equilibration time.
A special consideration must be given to 3D tissue cultures as they differ significantly from the cultured adherent cells discussed in this review. Introducing the element of three-dimensionality to cell cultures, which is arguably an intrinsic feature of all multicellular organisms, can improve the potential of in vitro models to recapitulate the in vivo environment (144). This also applies to conditions in which hypoxia plays an important role, including cancer, as the 3D organization has been found to play an integral part in tumor biology, considering the actual tumor architecture and dynamic interactions with the surrounding environment (145). While some models employ only single cell type spheroids (146), more complex platforms, reflecting physiological interactions found in vivo include multiple cell types in a 3D structure, such as cancer stromal or endothelial cells (147, 148) as reviewed earlier (149). For example, a study investigating the role of OSA in cancer employed a 3D cell culture model comprising both tumor spheroids and patient-derived monocytes subjected to IH and found that the monocyte-induced HIF-1α-dependent production of VEGF promoted tumor growth (150), providing some molecular insights into the link between the two diseases (151).
Similarly, 3D cell culture technology has been used to study the effect of hypoxia in the context of ischemia in various cell types, including cardiomyocytes (152–154), astrocytes (155), endothelial cells (156), and hypoxia related to pulmonary fibrosis in fetal lung fibroblasts (157). Furthermore, the effects of both continuous hypoxia and IH on vascular sprouting has been explored in endothelial cells (158–160). Hypoxic 3D tissue structures comprising retinal astrocytes and endothelial cells represent a useful drug-screening tool, outperforming standard 2D co-cultures (161).
With the proliferation of experiments conducted in 3D cell cultures, critical consideration of pericellular pO2 is warranted, particularly because the element of three-dimensionality and variable thickness of cellular structures introduces additional irregularities that hamper gas diffusion and lead to the formation of oxygen concentration gradients (162, 163). Several novel approaches and techniques have emerged tackling the challenges of pO2 in 3D tissue structures. Analogically to adherent cell cultures, oxygen-sensing microelectrodes have been employed to measure pericellular oxygen gradients in thicker hydrogel-based tissues (164, 165). However, the disadvantages of this approach, such as its invasive nature, time demands and technical challenges requiring repetitive calibrations and measurements in different spots inside the tissue construct, motivated the search for alternative approaches. A number of fluorescence quenching probes has been tested, which penetrate through cells (166, 167) or are incorporated into microbeads dispersed in a 3D hydrogel (Figure 4A) (168), and subsequently visualized using confocal microscope imaging. Such applications enable the establishment of a dense network of pO2 reporter points throughout the 3D cell culture block. Semi-quantitative approaches to the assessment of pericellular pO2 in 3D cultures include mathematical models (162) and probes (e.g., Hypoxyprobe) (163) or the incorporation of paramagnetic particles into cellular spheroids with subsequent electron paramagnetic resonance-based detection (171).
Figure 4. Schematic drawing of systems for 3D in vitro hypoxia. (A) Fluorescent sensor-laden microbeads incorporated into a 3D hydrogel with cells, serving as a pO2 reporter throughout the 3D system. Based on a drawing by (168). (B) 3D culture positioned between two gas perfusion microchannels, which are in contact with the culture via a gas-permeable membrane, allowing for an oxygen gradient to be formed across the culture between an oxygen-rich and oxygen-poor environment. Adapted with permission according to (169). (C) Encapsulation of a 3D cell culture or explanted tissue by an oxygen-consuming hydrogel, creating a hypoxic environment. The oxygen consumption is a result of the cross-linking reaction and hydrogel formation, resulting in an oxygen gradient from the top layer of the culture to deeper, more oxygen-deprived regions. Adapted with permission according to (165). (D) Preformed oxygen-consuming hydrogel immersed in a 3D culture creates a hypoxic environment with an oxygen gradient toward the hydrogel. The gradient and the level of hypoxia can be adjusted by moving the hydrogel across the culture on a mobile pillar. Adapted according to (170) under the CC BY license.
A unique feature of 3D cell culture systems is represented by the possibility of actively inducing a controlled oxygen gradient across the model, based on the experimental needs. Such gradients can be induced by perfusion with an oxygen scavenger in the medium (159); by positioning the culture between two micro-channel circuits perfused with gas, each with a different oxygen level (Figure 4B) (169, 172); or by incorporation of an oxygen-consuming reaction of specific hydrogel materials, either encapsulating (Figure 4C) (164, 165) or being in close vicinity of the cells (Figure 4D) (170), and thus regulating the pericellular oxygen levels.
Cell culture models represent an invaluable research tool for understanding the fundamental mechanisms of the pathogenesis of hypoxia-associated conditions and diseases, as well as for the development of therapies combatting them. However, physical laws pertaining to gas diffusion and oxygen distribution in cell cultures impede pericellular oxygen levels, and thus determine cellular processes. Multiple factors, including media thickness, media mixing, convective forces, cellular oxygen consumption, and headspace pO2 determine the pericellular concentration of O2, which is significantly different from the O2 levels in the gas phase in standard incubators. As even a small change in pericellular O2 levels may elicit variable molecular responses, the precise control of pericellular O2 levels is required for the appropriate interpretation of the physiological relevance of observed results as well as for laboratory-to-laboratory uniformity. Recent advances have produced several accessible, cost-effective, and high-throughput tools that are capable of emulating constant hypoxic or IH exposure closely reminiscent of the in vivo conditions. Moreover, the incorporation of 3D tissues into cellular models of hypoxia might bolster this line of research even further.
JPa and JPo conceived and wrote the manuscript and performed the research of bibliography. JPa conceived and made the figures. Both authors directly and substantially contributed to the work and gave permission for this manuscript to be published.
This work was supported by a grant from the Czech Science Foundation (GACR 18-10144S), by the Charles University projects GAUK No. 818419, SVV 260387/2019, and PROGRES Q36 and by a grant from the Czech Health Research Council (NV19-08-00122).
The authors declare that the research was conducted in the absence of any commercial or financial relationships that could be construed as a potential conflict of interest.
1. Mulkidjanian AY, Koonin EV, Makarova KS, Mekhedov SL, Sorokin A, Wolf YI, et al. The cyanobacterial genome core and the origin of photosynthesis. Proc Natl Acad Sci USA. 103:13126–31. doi: 10.1073/pnas.0605709103
2. Canfield DE. The early history of atmospheric oxygen: homage to Robert M. Garrels. Annu Rev Earth Planet Sci. (2005) 33:1–36. doi: 10.1146/annurev.earth.33.092203.122711
3. Hsia CCW, Schmitz A, Lambertz M, Perry SF, Maina JN. Evolution of air breathing: oxygen homeostasis and the transitions from water to land and sky. Compr. Physiol. (2013) 3:849–915. doi: 10.1002/cphy.c120003
4. Carreau A, Hafny-Rahbi BE, Matejuk A, Grillon C, Kieda C. Why is the partial oxygen pressure of human tissues a crucial parameter? Small molecules and hypoxia. J Cell Mol Med. (2011) 15:1239–53. doi: 10.1111/j.1582-4934.2011.01258.x
5. Jamshidi N, Palsson B. Systems biology of the human red blood cell. Blood Cells Mol Dis. (2006) 36:239–47. doi: 10.1016/j.bcmd.2006.01.006
6. Favier FB, Britto FA, Freyssenet DG, Bigard XA, Benoit H. HIF-1-driven skeletal muscle adaptations to chronic hypoxia: molecular insights into muscle physiology. Cell Mol Life Sci. (2015) 72:4681–96. doi: 10.1007/s00018-015-2025-9
7. Conkin J, Wessel JH. Critique of the equivalent air altitude model. Aviat Space Environ Med. (2008) 79:975–82. doi: 10.3357/ASEM.2331.2008
8. Coppel J, Hennis P, Gilbert-Kawai E, Grocott MP. The physiological effects of hypobaric hypoxia versus normobaric hypoxia: a systematic review of crossover trials. Extrem Physiol Med. (2015) 4:2. doi: 10.1186/s13728-014-0021-6
9. Zhuan B, Yu Y, Yang Z, Zhao X, Li P. Mechanisms of oxidative stress effects of the NADPH oxidase-ROS-NF-κB transduction pathway and VPO1 on patients with chronic obstructive pulmonary disease combined with pulmonary hypertension. Eur Rev Med Pharmacol Sci. (2017) 21:3459–64.
10. Ostadal P, Mlcek M, Strunina S, Hrachovina M, Kruger A, Vondrakova D, et al. Novel porcine model of acute severe cardiogenic shock developed by upper-body hypoxia. Physiol Res. (2016) 65:711–5.
11. Morita Y, Chin-Yee I, Yu P, Sibbald WJ, Martin CM. Critical oxygen delivery in conscious septic rats under stagnant or anemic hypoxia. Am J Respir Crit Care Med. (2003) 167:868–72. doi: 10.1164/rccm.200205-490OC
12. Lévy P, Kohler M, McNicholas WT, Barbé F, McEvoy RD, Somers VK, et al. Obstructive sleep apnoea syndrome. Nat Rev Dis Prim. (2015) 1:15015. doi: 10.1038/nrdp.2015.24
13. Benjamin JS, Culpepper CB, Brown LD, Wesolowski SR, Jonker SS, Davis MA, et al. Chronic anemic hypoxemia attenuates glucose-stimulated insulin secretion in fetal sheep. Am J Physiol Integr Comp Physiol. (2017) 312:R492–500. doi: 10.1152/ajpregu.00484.2016
14. Kizaka-Kondoh S, Kuchimaru T, Kadonosono T. Pathophysiological response to hypoxia — from the molecular mechanisms of malady to drug discovery: hypoxia-inducible factor-1 (HIF-1)-active cells as a target for cancer therapy. J Pharmacol Sci. (2011) 115:440–5. doi: 10.1254/jphs.10R20FM
15. Jaroch K, Jaroch A, Bojko B. Cell cultures in drug discovery and development: the need of reliable in vitro-in vivo extrapolation for pharmacodynamics and pharmacokinetics assessment. J Pharm Biomed Anal. (2018) 147:297–312. doi: 10.1016/j.jpba.2017.07.023
16. Jedrzejczak-Silicka M. History of cell culture. In: Gowder SJT, editor. New Insights Into Cell Culture Technology. London: InTech (2017). p. 43936.
17. Metzen E, Wolff M, Fandrey J, Jelkmann W. Pericellular PO2 and O2 consumption in monolayer cell cultures. Respir Physiol. (1995) 100:101–6. doi: 10.1016/0034-5687(94)00125-J
18. Pettersen EO, Larsen LH, Ramsing NB, Ebbesen P. Pericellular oxygen depletion during ordinary tissue culturing, measured with oxygen microsensors. Cell Prolif . (2005) 38:257–67. doi: 10.1111/j.1365-2184.2005.00345.x
19. Allen CB, Schneider BK, White CW. Limitations to oxygen diffusion and equilibration in in vitro cell exposure systems in hyperoxia and hypoxia. Am J Physiol Lung Cell Mol Physiol. (2001) 281:L1021–7. doi: 10.1152/ajplung.2001.281.4.L1021
20. Stenmark KR, Meyrick B, Galie N, Mooi WJ, McMurtry IF. Animal models of pulmonary arterial hypertension: the hope for etiological discovery and pharmacological cure. Am J Physiol Cell Mol Physiol. (2009) 297:L1013–32. doi: 10.1152/ajplung.00217.2009
21. Labossiere JR, Pelletier J, Thiesen A, Schulz R, Bigam DL, Cheung P. Doxycycline attenuates renal injury in a swine model of neonatal hypoxia-reoxygenation. Shock. (2015) 43:99–105. doi: 10.1097/SHK.0000000000000257
22. Thompson LP, Pence L, Pinkas G, Song H, Telugu BP. Placental hypoxia during early pregnancy causes maternal hypertension and placental insufficiency in the hypoxic guinea pig model. Biol Reprod. (2016) 95:128. doi: 10.1095/biolreprod.116.142273
23. Thelin EP. Experimental models combining traumatic brain injury and hypoxia. Methods Mol Biol. (2016) 1462:459–79. doi: 10.1007/978-1-4939-3816-2_26
24. Cao Z, Jensen LD, Rouhi P, Hosaka K, Länne T, Steffensen JF, et al. Hypoxia-induced retinopathy model in adult zebrafish. Nat Protoc. (2010) 5:1903–10. doi: 10.1038/nprot.2010.149
25. Bourdillon N, Saugy J, Schmitt L, Rupp T, Yazdani S, Vesin J-M, et al. Acute and chronic changes in baroreflex sensitivity in hypobaric vs. normobaric hypoxia. Eur J Appl Physiol. (2017) 117:2401–7. doi: 10.1007/s00421-017-3726-6
26. Louis M, Punjabi NM. Effects of acute intermittent hypoxia on glucose metabolism in awake healthy volunteers. J Appl Physiol. (2009) 106:1538–44. doi: 10.1152/japplphysiol.91523.2008
27. Weiss JW, Pépin JL, Gilmartin GS, Thomas R, Nespoulet H, Launois SH, et al. A new model of chronic intermittent hypoxia in humans: effect on ventilation, sleep, and blood pressure. J Appl Physiol. (2009) 107:17–24. doi: 10.1152/japplphysiol.91165.2008
28. Self DA, Mandella JG, Prinzo OV, Forster EM, Shaffstall RM. Physiological equivalence of normobaric and hypobaric exposures of humans to 25,000 feet (7620 m). Aviat Space Environ Med. (2011) 82:97–103. doi: 10.3357/ASEM.2908.2011
29. Woods DR, O'Hara JP, Boos CJ, Hodkinson PD, Tsakirides C, Hill NE, et al. Markers of physiological stress during exercise under conditions of normoxia, normobaric hypoxia, hypobaric hypoxia, and genuine high altitude. Eur J Appl Physiol. (2017) 117:893–900. doi: 10.1007/s00421-017-3573-5
30. Ko HC, Gelb BD. Concise review: drug discovery in the age of the induced pluripotent stem cell. Stem Cells Transl Med. (2014) 3:500–509. doi: 10.5966/sctm.2013-0162
31. Sterneckert JL, Reinhardt P, Schöler HR. Investigating human disease using stem cell models. Nat Rev Genet. (2014) 15:625–39. doi: 10.1038/nrg3764
32. Shi Y, Inoue H, Wu JC, Yamanaka S. Induced pluripotent stem cell technology: a decade of progress. Nat Rev Drug Discov. (2017) 16:115–30. doi: 10.1038/nrd.2016.245
33. Ilic D, Ogilvie C. Concise review: human embryonic stem cells-what have we done? What are we doing? Where are we going? Stem Cells. (2017) 35:17–25. doi: 10.1002/stem.2450
34. Dutta D, Heo I, Clevers H. Disease modeling in stem cell-derived 3D organoid systems. Trends Mol Med. (2017) 23:393–410. doi: 10.1016/j.molmed.2017.02.007
35. Zhang YS, Aleman J, Shin SR, Kilic T, Kim D, Mousavi Shaegh SA, et al. Multisensor-integrated organs-on-chips platform for automated and continual in situ monitoring of organoid behaviors. Proc Natl Acad Sci USA. (2017) 114:E2293–302. doi: 10.1073/pnas.1612906114
36. Place TL, Domann FE, Case AJ. Limitations of oxygen delivery to cells in culture: an underappreciated problem in basic and translational research. Free Radic Biol Med. (2017) 113:311–22. doi: 10.1016/j.freeradbiomed.2017.10.003
37. Cree IA. Cancer cell culture. In: Cree IA, editor. Totowa, NJ: Humana Press (2011). doi: 10.1007/978-1-61779-080-5
38. Woo W, Yeo SI. Dalton's law vs, amagat's law for the mixture of real gases. SNU J Educ Res. (1995) 5:127–34.
39. Story DA. Alveolar oxygen partial pressure, alveolar carbon dioxide partial pressure, and the alveolar gas equation. Anesthesiology. (1996) 84:1011. doi: 10.1097/00000542-199604000-00036
40. Krogh A. The rate of diffusion of gases through animal tissues, with some remarks on the coefficient of invasion. J Physiol. (1919) 52:391–408. doi: 10.1113/jphysiol.1919.sp001838
41. Stevens KM. Oxygen requirements for liver cells in vitro. Nature. (1965) 206:199. doi: 10.1038/206199a0
42. Weiszenstein M, Pavlikova N, Elkalaf M, Halada P, Seda O, Trnka J, et al. The effect of pericellular oxygen levels on proteomic profile and lipogenesis in 3T3-L1 differentiated preadipocytes cultured on gas-permeable cultureware. PLoS ONE. (2016) 11:e0152382. doi: 10.1371/journal.pone.0152382
43. Musutova M, Elkalaf M, Klubickova N, Koc M, Povysil S, Rambousek J, et al. The effect of hypoxia and metformin on fatty acid uptake, storage, and oxidation in L6 differentiated myotubes. Front Endocrinol. (2018) 9:1–11. doi: 10.3389/fendo.2018.00616
44. Oze H, Hirao M, Ebina K, Shi K, Kawato Y, Kaneshiro S, et al. Impact of medium volume and oxygen concentration in the incubator on pericellular oxygen concentration and differentiation of murine chondrogenic cell culture. Vitr Cell Dev Biol Anim. (2012) 48:123–30. doi: 10.1007/s11626-011-9479-3
45. Polak J, Studer-Rabler K, McHugh H, Hussain MA, Shimoda LA. System for exposing cultured cells to intermittent hypoxia utilizing gas permeable cultureware. Gen Physiol Biophys. (2015) 34:235–47. doi: 10.4149/gpb_2014043
46. Maddalena LA, Selim SM, Fonseca J, Messner H, McGowan S, Stuart JA. Hydrogen peroxide production is affected by oxygen levels in mammalian cell culture. Biochem Biophys Res Commun. (2017) 493:246–51. doi: 10.1016/j.bbrc.2017.09.037
47. Kieninger J, Aravindalochanan K, Sandvik JA, Pettersen EO, Urban GA. Pericellular oxygen monitoring with integrated sensor chips for reproducible cell culture experiments. Cell Prolif . (2014) 47:180–8. doi: 10.1111/j.1365-2184.2013.12089.x
48. Chen B, Longtine MS, Nelson DM. Pericellular oxygen concentration of cultured primary human trophoblasts. Placenta. (2013) 34:106–9. doi: 10.1016/j.placenta.2012.11.011
49. Christmas KM, Bassingthwaighte JB. Equations for O2 and CO2 solubilities in saline and plasma: combining temperature and density dependences. J Appl Physiol. (2017) 122:1313–20. doi: 10.1152/japplphysiol.01124.2016
51. Buck LD, Inman SW, Rusyn I, Griffith LG. Co-regulation of primary mouse hepatocyte viability and function by oxygen and matrix. Biotechnol Bioeng. (2014) 111:1018–27. doi: 10.1002/bit.25152
52. Clark LC, Kaplan S, Matthews EC, Edwards FK, Helmsworth JA. Monitor and control of blood oxygen tension and pH during total body perfusion. J Thorac Surg. (1958) 36:488–96.
53. Detz RJ, Abiri Z, Kluwer AM, Reek JNH. A fluorescence-based screening protocol for the identification of water oxidation catalysts. ChemSusChem. (2015) 8:3057–61. doi: 10.1002/cssc.201500558
54. Hong S, Tilan JU, Galli S, Acree R, Connors K, Mahajan A, et al. In vivo model for testing effect of hypoxia on tumor metastasis. J Vis Exp. (2016) 118:1–12. doi: 10.3791/54532
55. Kizaka-Kondoh S, Konse-Nagasawa H. Significance of nitroimidazole compounds and hypoxia-inducible factor-1 for imaging tumor hypoxia. Cancer Sci. (2009) 100:1366–73. doi: 10.1111/j.1349-7006.2009.01195.x
56. Shahrzad S, Bertrand K, Minhas K, Coomber B. Induction of DNA hypomethylation by tumor hypoxia. Epigenetics. (2007) 2:119–25. doi: 10.4161/epi.2.2.4613
57. Zhdanov AV, Ogurtsov VI, Taylor CT, Papkovsky DB. Monitoring of cell oxygenation and responses to metabolic stimulation by intracellular oxygen sensing technique. Integr Biol. (2010) 2:443–51. doi: 10.1039/c0ib00021c
58. Oppegard SC, Blake AJ, Williams JC, Eddington DT. Precise control over the oxygen conditions within the Boyden chamber using a microfabricated insert. Lab Chip. (2010) 10:2366. doi: 10.1039/c004856a
59. Taylor CT, Kent BD, Crinion SJ, McNicholas WT, Ryan S. Human adipocytes are highly sensitive to intermittent hypoxia induced NF-kappaB activity and subsequent inflammatory gene expression. Biochem Biophys Res Commun. (2014) 447:660–5. doi: 10.1016/j.bbrc.2014.04.062
60. Toffoli S, Roegiers A, Feron O, Van Steenbrugge M, Ninane N, Raes M, et al. Intermittent hypoxia is an angiogenic inducer for endothelial cells: role of HIF-1. Angiogenesis. (2009) 12:47–67. doi: 10.1007/s10456-009-9131-y
61. Toffoli S, Delaive E, Dieu M, Feron O, Raes M, Michiels C. NDRG1 and CRK-I/II are regulators of endothelial cell migration under intermittent hypoxia. Angiogenesis. (2009) 12:339–54. doi: 10.1007/s10456-009-9156-2
62. Koch CJ. A thin-film culturing technique allowing rapid gas-liquid equilibration (6 sec) with no toxicity to mammalian cells. Radiat Res. (1984) 97:434–42. doi: 10.2307/3576294
63. Campillo N, Jorba I, Schaedel L, Casals B, Gozal D, Farré R, et al. A novel chip for cyclic stretch and intermittent hypoxia cell exposures mimicking obstructive sleep apnea. Front Physiol. (2016) 7:1–12. doi: 10.3389/fphys.2016.00319
64. Minoves M, Morand J, Perriot F, Chatard M, Gonthier B, Lemarié E, et al. An innovative intermittent hypoxia model for cell cultures allowing fast P <scp>o</scp> 2 oscillations with minimal gas consumption. Am J Physiol Physiol. (2017) 313:C460–8. doi: 10.1152/ajpcell.00098.2017
65. Murphy AM, Thomas A, Crinion SJ, Kent BD, Tambuwala MM, Fabre A, et al. Intermittent hypoxia in obstructive sleep apnoea mediates insulin resistance through adipose tissue inflammation. Eur Respir J. (2017) 49:1601731. doi: 10.1183/13993003.01731-2016
66. Otto CM, Baumgardner JE. Effect of culture PO2 on macrophage (RAW 264.7) nitric oxide production. Am J Physiol Cell Physiol. (2001) 280:C280–7. doi: 10.1152/ajpcell.2001.280.2.C280
67. Redline S, Gottlieb DJ, Ho V, Crainiceanu CM, Punjabi NM. Calibration model for apnea-hypopnea indices: impact of alternative criteria for hypopneas. Sleep. (2015) 38:1887–92. doi: 10.5665/sleep.5234
68. Oppegard SC, Eddington DT. Device for the control of oxygen concentration in multiwell cell culture plates. Conf Proc. Annu Int Conf IEEE Eng Med Biol Soc IEEE Eng Med Biol Soc Annu Conf . (2009) 2009:2097–100. doi: 10.1109/IEMBS.2009.5332491
69. Polotsky VY, Savransky V, Bevans-Fonti S, Reinke C, Li J, Grigoryev DN, Shimoda LA. Intermittent and sustained hypoxia induce a similar gene expression profile in human aortic endothelial cells. Physiol Genomics. (2010) 41:306–314. doi: 10.1152/physiolgenomics.00091.2009
70. Baumgardner JE, Otto CM. In vitro intermittent hypoxia: challenges for creating hypoxia in cell culture. Respir Physiol Neurobiol. (2003) 136:131–9. doi: 10.1016/S1569-9048(03)00077-6
71. Tsapikouni T, Garreta E, Melo E, Navajas D, Farré R. A bioreactor for subjecting cultured cells to fast-rate intermittent hypoxia. Respir Physiol Neurobiol. (2012) 182:47–52. doi: 10.1016/j.resp.2012.01.001
72. Polinkovsky M, Gutierrez E, Levchenko A, Groisman A. Fine temporal control of the medium gas content and acidity and on-chip generation of series of oxygen concentrations for cell cultures. Lab Chip. (2009) 9:1073. doi: 10.1039/b816191g
73. Lam RHW, Kim M, Thorsen T. Culturing aerobic and anaerobic bacteria and mammalian cells with a microfluidic differential oxygenator. Anal Chem. (2009) 81:5918–24. doi: 10.1021/ac9006864
74. Lo JF, Wang Y, Blake A, Yu G, Harvat TA, Jeon H, et al. Islet preconditioning via multimodal microfluidic modulation of intermittent hypoxia. Anal Chem. (2012) 84:1987–93. doi: 10.1021/ac2030909
75. Ryan S, Taylor CT, McNicholas WT. Selective activation of inflammatory pathways by intermittent hypoxia in obstructive sleep apnea syndrome. Circulation. (2005) 112:2660–7. doi: 10.1161/CIRCULATIONAHA.105.556746
76. Wree A, Mayer A, Westphal S, Beilfuss A, Canbay A, Schick RR, et al. Adipokine expression in brown and white adipocytes in response to hypoxia. J Endocrinol Invest. (2012) 35:522–7. doi: 10.3275/7964
77. Haslip M, Dostanic I, Huang Y, Zhang Y, Russell KS, Jurczak MJ, et al. Endothelial uncoupling protein 2 regulates mitophagy and pulmonary hypertension during intermittent hypoxia. Arterioscler Thromb Vasc Biol. (2015) 35:1166–78. doi: 10.1161/ATVBAHA.114.304865
78. Mees G, Dierckx R, Vangestel C, Laukens D, Van Damme N, Van De Wiele C. Pharmacologic activation of tumor hypoxia: a means to increase tumor 2-deoxy-2-[18F]fluoro-D-glucose uptake? Mol Imaging. (2013) 12:49–58. doi: 10.2310/7290.2012.00020
79. Liu S, Yin Y, Yu R, Li Y, Zhang W. R-spondin3-LGR4 signaling protects hepatocytes against DMOG-induced hypoxia/reoxygenation injury through activating β-catenin. Biochem Biophys Res Commun. (2018) 499:59–65. doi: 10.1016/j.bbrc.2018.03.126
80. Sethi K, Rao K, Bolton D, Patel O, Ischia J. Targeting HIF-1 α to prevent renal ischemia-reperfusion injury: does it work? Int J Cell Biol. (2018) 2018:1–7. doi: 10.1155/2018/9852791
81. Davis CK, Jain SA, Bae O-N, Majid A, Rajanikant GK. Hypoxia mimetic agents for ischemic stroke. Front Cell Dev Biol. (2019) 6:1–12. doi: 10.3389/fcell.2018.00175
82. Chatard M, Puech C, Perek N, Roche F. Hydralazine is a suitable mimetic agent of hypoxia to study the impact of hypoxic stress on in vitro blood-brain barrier model. Cell Physiol Biochem. (2017) 42:1592–602. doi: 10.1159/000479399
83. Zhdanov AV, Dmitriev RI, Papkovsky DB. Bafilomycin A1 activates HIF-dependent signalling in human colon cancer cells via mitochondrial uncoupling. Biosci Rep. (2012) 32:587–95. doi: 10.1042/BSR20120085
84. Wenger RH, Kurtcuoglu V, Scholz CC, Marti HH, Hoogewijs D. Frequently asked questions in hypoxia research. Hypoxia. (2015) 3:35–43. doi: 10.2147/HP.S92198
85. Wagner BA, Venkataraman S, Buettner GR. The rate of oxygen utilization by cells. Free Radic Biol Med. (2011) 51:700–12. doi: 10.1016/j.freeradbiomed.2011.05.024
86. Pasarica M, Sereda OR, Redman LM, Albarado DC, Hymel DT, Roan LE, et al. Reduced adipose tissue oxygenation in human obesity: evidence for rarefaction, macrophage chemotaxis, and inflammation without an angiogenic response. Diabetes. (2009) 58:718–25. doi: 10.2337/db08-1098
87. Ye J. Emerging role of adipose tissue hypoxia in obesity and insulin resistance. Int J Obes. (2009) 33:54–66. doi: 10.1038/ijo.2008.229
88. Choi JR, Pingguan-Murphy B, Wan Abas WAB, Yong KW, Poon CT, Noor Azmi MA, et al. In situ normoxia enhances survival and proliferation rate of human adipose tissue-derived stromal cells without increasing the risk of tumourigenesis. PLoS ONE. (2015) 10:e0115034. doi: 10.1371/journal.pone.0115034
89. Maltepe E, Saugstad OD. Oxygen in health and disease: regulation of oxygen homeostasis–clinical implications. Pediatr Res. (2009) 65:261–8. doi: 10.1203/PDR.0b013e31818fc83f
90. Morin SJ. Oxygen tension in embryo culture: does a shift to 2% O2 in extended culture represent the most physiologic system? J Assist Reprod Genet. (2017) 34:309–14. doi: 10.1007/s10815-017-0880-z
91. Ezashi T, Das P, Roberts RM. Low O2 tensions and the prevention of differentiation of HES cells. Nat Methods. (2005) 2:325. doi: 10.1073/pnas.0501283102
92. Forsyth NR, Kay A, Hampson K, Downing A, Talbot R, McWhir J. Transcriptome alterations due to physiological normoxic (2% O2) culture of human embryonic stem cells. Regen Med. (2008) 3:817–33. doi: 10.2217/17460751.3.6.817
93. Chen H-F, Kuo H-C, Lin S-P, Chien C-L, Chiang M-S, Ho H-N. Hypoxic culture maintains self-renewal and enhances embryoid body formation of human embryonic stem cells. Tissue Eng Part A. (2010) 16:2901–13. doi: 10.1089/ten.tea.2009.0722
94. Lim HJ, Han J, Woo DH, Kim SE, Kim SK, Kang HG, et al. Biochemical and morphological effects of hypoxic environment on human embryonic stem cells in long-term culture and differentiating embryoid bodies. Mol Cells. (2011) 31:123–32. doi: 10.1007/s10059-011-0016-8
95. Fynes K, Tostoes R, Ruban L, Weil B, Mason C, Veraitch FS. The differential effects of 2% oxygen preconditioning on the subsequent differentiation of mouse and human pluripotent stem cells. Stem Cells Dev. (2014) 23:1910–22. doi: 10.1089/scd.2013.0504
96. Chen HF, Kuo HC, Chen W, Wu FC, Yang YS, Ho HN. A reduced oxygen tension (5%) is not beneficial for maintaining human embryonic stem cells in the undifferentiated state with short splitting intervals. Hum Reprod. (2009) 24:71–80. doi: 10.1093/humrep/den345
97. Mohyeldin A, Garzón-Muvdi T, Quiñones-Hinojosa A. Oxygen in stem cell biology: a critical component of the stem cell niche. Cell Stem Cell. (2010) 7:150–61. doi: 10.1016/j.stem.2010.07.007
98. Chen KG, Mallon BS, McKay RDG, Robey PG. Human pluripotent stem cell culture: considerations for maintenance, expansion, and therapeutics. Cell Stem Cell. (2014) 14:13–26. doi: 10.1016/j.stem.2013.12.005
99. Rumsey WL, Pawlowski M, Lejavardi N, Wilson DF. Oxygen pressure distribution in the heart in vivo and evaluation of the ischemic “border zone.” Am J Physiol. (1994) 266:H1676–80. doi: 10.1152/ajpheart.1994.266.4.H1676
100. Linsenmeier RA, Zhang HF. Retinal oxygen: from animals to humans. Prog Retin Eye Res. (2017) 58:115–51. doi: 10.1016/j.preteyeres.2017.01.003
101. Zamudio S, Borges M, Echalar L, Kovalenko O, Vargas E, Torricos T, et al. Maternal and fetoplacental hypoxia do not alter circulating angiogenic growth effectors during human pregnancy1. Biol Reprod. (2014) 90:1–9. doi: 10.1095/biolreprod.113.115592
102. Farré R, Navajas D, Planas AM, Almendros I, Bonsignore MR, Torres M, et al. Tissue oxygenation in brain, muscle, and fat in a rat model of sleep apnea: differential effect of obstructive apneas and intermittent hypoxia. Sleep. (2011) 34:1127–33. doi: 10.5665/SLEEP.1176
103. Thorn CE, Knight B, Pastel E, McCulloch LJ, Patel B, Shore AC, et al. Adipose tissue is influenced by hypoxia of obstructive sleep apnea syndrome independent of obesity. Diabetes Metab. (2017) 43:240–7. doi: 10.1016/j.diabet.2016.12.002
104. Abe H, Semba H, Takeda N. The roles of hypoxia signaling in the pathogenesis of cardiovascular diseases. J Atheroscler Thromb. (2017) 24:884–94. doi: 10.5551/jat.RV17009
105. Fan J, Fan X, Li Y, Ding L, Zheng Q, Guo J, et al. Chronic normobaric hypoxia induces pulmonary hypertension in rats: role of NF-κB. High Alt Med Biol. (2016) 17:43–9. doi: 10.1089/ham.2015.0086
106. Liu M, Ning X, Li R, Yang Z, Yang X, Sun S, et al. Signalling pathways involved in hypoxia-induced renal fibrosis. J Cell Mol Med. (2017) 21:1248–59. doi: 10.1111/jcmm.13060
107. Jaitovich A, Jourd'heuil D. In: ed Wang Y-X. Pulmonary Vasculature Redox Signaling in Health and Disease. Cham: Springer International Publishing (2017).
108. Shimoda LA, Polak J. Hypoxia. 4. Hypoxia and ion channel function. Am J Physiol Physiol. (2011) 300:C951–67. doi: 10.1152/ajpcell.00512.2010
109. Wang GL, Jiang BH, Rue EA, Semenza GL. Hypoxia-inducible factor 1 is a basic-helix-loop-helix-PAS heterodimer regulated by cellular O2 tension. Proc Natl Acad Sci USA. (1995) 92:5510–4. doi: 10.1073/pnas.92.12.5510
110. Michiels C, Minet E, Mottet D, Raes M. Regulation of gene expression by oxygen: NF-kappaB and HIF-1, two extremes. Free Radic Biol Med. (2002) 33:1231–42. doi: 10.1016/S0891-5849(02)01045-6
111. Zepeda AB, Pessoa A, Castillo RL, Figueroa CA, Pulgar VM, Farías JG. Cellular and molecular mechanisms in the hypoxic tissue: role of HIF-1 and ROS. Cell Biochem Funct. (2013) 31:451–9. doi: 10.1002/cbf.2985
112. Zhang L, Hu Y, Xi N, Song J, Huang W, Song S, et al. Partial oxygen pressure affects the expression of prognostic biomarkers HIF-1 alpha, Ki67, and CK20 in the microenvironment of colorectal cancer tissue. Oxid Med Cell Longev. (2016) 2016:1–12. doi: 10.1155/2016/1204715
113. Jiang BH, Semenza GL, Bauer C, Marti HH. Hypoxia-inducible factor 1 levels vary exponentially over a physiologically relevant range of O2 tension. Am J Physiol. (1996) 271:C1172-80. doi: 10.1152/ajpcell.1996.271.4.C1172
114. Edin NJ, Olsen DR, Sandvik JA, Malinen E, Pettersen EO. Low dose hyper-radiosensitivity is eliminated during exposure to cycling hypoxia but returns after reoxygenation. Int J Radiat Biol. (2012) 88:311–9. doi: 10.3109/09553002.2012.646046
115. Lee B, Chiu N, Hsia C, Shen L. Accumulation of Tc-99m HL91 in tumor hypoxia: in vitro cell culture and in vivo tumor model. Kaohsiung J Med Sci. (2008) 24:461–72. doi: 10.1016/S1607-551X(09)70003-8
116. Wang L, Liu W, Wang Y, Wang J, Tu Q, Liu R, et al. Construction of oxygen and chemical concentration gradients in a single microfluidic device for studying tumor cell-drug interactions in a dynamic hypoxia microenvironment. Lab Chip. (2013) 13:695–705. doi: 10.1039/C2LC40661F
117. Byrne MB, Leslie MT, Gaskins HR, Kenis PJA. Methods to study the tumor microenvironment under controlled oxygen conditions. Trends Biotechnol. (2014) 32:556–63. doi: 10.1016/j.tibtech.2014.09.006
118. Xi C, Liang X, Chen C, Babazada H, Li T, Liu R. Hypoxia Induces Internalization of κ-Opioid Receptor. Anesthesiology. (2017) 126:842–54. doi: 10.1097/ALN.0000000000001571
119. Panel M, Ghaleh B, Morin D. Ca2+ ionophores are not suitable for inducing mPTP opening in murine isolated adult cardiac myocytes. Sci Rep. (2017) 7:4283. doi: 10.1038/s41598-017-04618-4
120. Martewicz S, Michielin F, Serena E, Zambon A, Mongillo M, Elvassore N. Reversible alteration of calcium dynamics in cardiomyocytes during acute hypoxia transient in a microfluidic platform. Integr Biol. (2012) 4:153–64. doi: 10.1039/C1IB00087J
121. Wang B, Wood IS, Trayhurn P. Dysregulation of the expression and secretion of inflammation-related adipokines by hypoxia in human adipocytes. Pflügers Arch Eur J Physiol. (2007) 455:479–92. doi: 10.1007/s00424-007-0301-8
122. Floyd ZE, Kilroy G, Wu X, Gimble JM. Effects of prolyl hydroxylase inhibitors on adipogenesis and hypoxia inducible factor 1 alpha levels under normoxic conditions. J Cell Biochem. (2007) 101:1545–57. doi: 10.1002/jcb.21266
123. Chuang L, Chen N, Lin S-W, Chang Y, Liao H, Lin Y-S, et al. Increased C-C chemokine receptor 2 gene expression in monocytes of severe obstructive sleep apnea patients and under intermittent hypoxia. PLoS ONE. (2014) 9:e113304. doi: 10.1371/journal.pone.0113304
124. Chuang L, Chen N, Lin Y, Ko W, Pang JS. Increased MCP-1 gene expression in monocytes of severe OSA patients and under intermittent hypoxia. Sleep Breath. (2016) 20:425–33. doi: 10.1007/s11325-015-1252-5
125. Philippe C, Boussadia Y, Prulière-Escabasse V, Papon JF, Clérici C, Isabey D, et al. Airway cell involvement in intermittent hypoxia-induced airway inflammation. Sleep Breath. (2015) 19:297–306. doi: 10.1007/s11325-014-1019-4
126. Dyugovskaya L, Polyakov A, Lavie P, Lavie L. Delayed neutrophil apoptosis in patients with sleep apnea. Am J Respir Crit Care Med. (2008) 177:544–54. doi: 10.1164/rccm.200705-675OC
127. Yuan G, Nanduri J, Bhasker CR, Semenza GL, Prabhakar NR. Ca 2+ /calmodulin kinase-dependent activation of hypoxia inducible factor 1 transcriptional activity in cells subjected to intermittent hypoxia. J Biol Chem. (2005) 280:4321–28. doi: 10.1074/jbc.M407706200
128. Kaczmarek E, Bakker JP, Clarke DN, Csizmadia E, Kocher O, Veves A, et al. Molecular biomarkers of vascular dysfunction in obstructive sleep apnea. PLoS ONE. (2013) 8:e70559. doi: 10.1371/journal.pone.0070559
129. Li C, Ni C, Yang M, Tang Y, Li Z, Zhu Y, et al. Honokiol protects pancreatic β cell against high glucose and intermittent hypoxia-induced injury by activating Nrf2/ARE pathway in vitro and in vivo. Biomed Pharmacother. (2018) 97:1229–37. doi: 10.1016/j.biopha.2017.11.063
130. Wu J, Stefaniak J, Hafner C, Schramel JP, Kaun C, Wojta J, et al. Intermittent hypoxia causes inflammation and injury to human adult cardiac myocytes. Anesth Analg. (2016) 122:373–80. doi: 10.1213/ANE.0000000000001048
131. Liu K, Chen G, Lin P-L, Huang J, Lin X, Qi J, et al. Detection and analysis of apoptosis- and autophagy-related miRNAs of mouse vascular endothelial cells in chronic intermittent hypoxia model. Life Sci. (2018) 193:194–9. doi: 10.1016/j.lfs.2017.11.001
132. Zhou Y, Richards AM, Wang P. Characterization and standardization of cultured cardiac fibroblasts for ex vivo models of heart fibrosis and heart ischemia. Tissue Eng Part C Methods. (2017) 23:422–33. doi: 10.1089/ten.tec.2017.0169
133. Ren J, Liu W, Li G, Jin M, You Z, Liu H, et al. Atorvastatin attenuates myocardial hypertrophy induced by chronic intermittent hypoxia in vitro partly through miR-31/PKCε pathway. Curr Med Sci. (2018) 38:405–12. doi: 10.1007/s11596-018-1893-2
134. Gagner J, Lechpammer M, Zagzag D. Induction and assessment of hypoxia in glioblastoma cells in vitro. In: Placantonakis D, editor. Methods in Molecular Biology. New York, NY: Springer Science+Business Media (2018). p. 111–23.
135. Zagzag D, Esencay M, Mendez O, Yee H, Smirnova I, Huang Y, et al. Hypoxia- and vascular endothelial growth factor-induced stromal cell-derived factor-1α/CXCR4 expression in glioblastomas. Am J Pathol. (2008) 173:545–60. doi: 10.2353/ajpath.2008.071197
136. Zagzag D, Lukyanov Y, Lan L, Ali MA, Esencay M, Mendez O, et al. Hypoxia-inducible factor 1 and VEGF upregulate CXCR4 in glioblastoma: implications for angiogenesis and glioma cell invasion. Lab Investig. (2006) 86:1221–32. doi: 10.1038/labinvest.3700482
137. Chanana V, Tumturk A, Kintner D, Udho E, Ferrazzano P, Cengiz P. Sex differences in mouse hippocampal astrocytes after in-vitro ischemia. J Vis Exp. (2016) 116:1-9. doi: 10.3791/53695
138. Wang X, Wang W, Wang J, Yang C, Liang C. Effect of apigenin on apoptosis induced by renal ischemia/reperfusion injury in vivo and in vitro. Ren Fail. (2018) 40:498–505. doi: 10.1080/0886022X.2018.1497517
139. Radovits T, Zotkina J, Lin L, Karck M, Szabó G. Endothelial dysfunction after hypoxia–reoxygenation: do in vitro models work? Vascul Pharmacol. (2009) 51:37–43. doi: 10.1016/j.vph.2009.01.009
140. Wu D, Yotnda P. Induction and testing of hypoxia in cell culture. J Vis Exp. (2011) 54:2–5. doi: 10.3791/2899
141. Maugeri G, D'Amico AG, Rasà DM, La Cognata V, Saccone S, Federico C, et al. Caffeine prevents blood retinal barrier damage in a model, in vitro, of diabetic macular edema. J Cell Biochem. (2017) 118:2371–9. doi: 10.1002/jcb.25899
142. Zagzag D, Zhong H, Scalzitti JM, Laughner E, Simons JW, Semenza GL. Expression of hypoxia-inducible factor 1alpha in brain tumors: association with angiogenesis, invasion, and progression. Cancer. (2000) 88:2606–18. doi: 10.1002/1097-0142(20000601)88:11<2606::AID-CNCR25>3.0.CO;2-W
143. Ord JJ, Streeter EH, Roberts ISD, Cranston D, Harris AL. Comparison of hypoxia transcriptome in vitro with in vivo gene expression in human bladder cancer. Br J Cancer. (2005) 93:346–54. doi: 10.1038/sj.bjc.6602666
144. Duval K, Grover H, Han L, Mou Y, Pegoraro AF, Fredberg J, et al. Modeling physiological events in 2D vs. 3D cell culture. Physiology. (2017) 32:266–77. doi: 10.1152/physiol.00036.2016
145. Tanner K, Mori H, Mroue R, Bruni-Cardoso A, Bissell MJ. Coherent angular motion in the establishment of multicellular architecture of glandular tissues. Proc Natl Acad Sci USA. (2012) 109:1973–8. doi: 10.1073/pnas.1119578109
146. Wartenberg M, Ling FC, Müschen M, Klein F, Acker H, Gassmann M, et al. Regulation of the multidrug resistance transporter P-glycoprotein in multicellular tumor spheroids by hypoxia-inducible factor (HIF-1) and reactive oxygen species. FASEB J. (2003) 17:503–5. doi: 10.1096/fj.02-0358fje
147. Magdeldin T, López-Dávila V, Pape J, Cameron GWW, Emberton M, Loizidou M, et al. Engineering a vascularised 3D in vitro model of cancer progression. Sci Rep. (2017) 7:44045. doi: 10.1038/srep44045
148. Benton G, DeGray G, Kleinman HK, George J, Arnaoutova I. In vitro microtumors provide a physiologically predictive tool for breast cancer therapeutic screening. PLoS ONE. (2015) 10:e0123312. doi: 10.1371/journal.pone.0123312
149. Leek R, Grimes DR, Harris AL, Mcintyre A. In: Koumenis C, Coussens LM, Giaccia A, Hammond E, editors. Tumor Microenvironment. Cham: Springer International Publishing (2016).
150. Cubillos-Zapata C, Hernández-Jiménez E, Avendaño-Ortiz J, Toledano V, Varela-Serrano A, Fernández-Navarro I, et al. Obstructive sleep apnea monocytes exhibit high levels of vascular endothelial growth factor secretion, augmenting tumor progression. Med Inflamm. (2018) 2018:1–9. doi: 10.1155/2018/7373921
151. Hunyor I, Cook KM. Models of intermittent hypoxia and obstructive sleep apnea: molecular pathways and their contribution to cancer. Am J Physiol Regul Integr Comp Physiol. (2018) 315:R669–87. doi: 10.1152/ajpregu.00036.2018
152. Bauer M, Kang L, Qiu Y, Wu J, Peng M, Chen HH, et al. Adult cardiac progenitor cell aggregates exhibit survival benefit both in vitro and in vivo. PLoS ONE. (2012) 7:e50491. doi: 10.1371/journal.pone.0050491
153. Sepuri NBV, Angireddy R, Srinivasan S, Guha M, Spear J, Lu B, et al. Mitochondrial LON protease-dependent degradation of cytochrome c oxidase subunits under hypoxia and myocardial ischemia. Biochim Biophys Acta Bioenerg. (2017) 1858:519–28. doi: 10.1016/j.bbabio.2017.04.003
154. Acun A, Zorlutuna P. Engineered myocardium model to study the roles of HIF-1α and HIF1A-AS1 in paracrine-only signaling under pathological level oxidative stress. Acta Biomater. (2017) 58:323–36. doi: 10.1016/j.actbio.2017.06.023
155. Chaitanya G, Minagar A, Alexander JS. Neuronal and astrocytic interactions modulate brain endothelial properties during metabolic stresses of in vitro cerebral ischemia. Cell Commun Signal. (2014) 12:7. doi: 10.1186/1478-811X-12-7
156. Gammella E, Leuenberger C, Gassmann M, Ostergaard L. Evidence of synergistic/additive effects of sildenafil and erythropoietin in enhancing survival and migration of hypoxic endothelial cells. Am J Physiol Cell Mol Physiol. (2013) 304:L230–9. doi: 10.1152/ajplung.00112.2012
157. Sucre JMS, Vijayaraj P, Aros CJ, Wilkinson D, Paul M, Dunn B, et al. Posttranslational modification of β-catenin is associated with pathogenic fibroblastic changes in bronchopulmonary dysplasia. Am J Physiol Cell Mol Physiol. (2017) 312:L186–95. doi: 10.1152/ajplung.00477.2016
158. Ehsan SM, George SC. Vessel network formation in response to intermittent hypoxia is frequency dependent. J Biosci Bioeng. (2015) 120:347–50. doi: 10.1016/j.jbiosc.2015.01.017
159. Lam SF, Shirure VS, Chu YE, Soetikno AG, George SC. Microfluidic device to attain high spatial and temporal control of oxygen. PLoS ONE. (2018) 13:1–16. doi: 10.1371/journal.pone.0209574
160. Alhawarat FM, Hammad HM, Hijjawi MS, Sharab AS, Abuarqoub DA, Al Shhab MA, et al. The effect of cycling hypoxia on MCF-7 cancer stem cells and the impact of their microenvironment on angiogenesis using human umbilical vein endothelial cells (HUVECs) as a model. PeerJ. (2019) 7:e5990. doi: 10.7717/peerj.5990
161. Beharry KD, Cai CL, Valencia GB, Lazzaro D, Valencia AM, Salomone F, et al. Human retinal endothelial cells and astrocytes cultured on 3-D scaffolds for ocular drug discovery and development. Prostaglandins Other Lipid Mediat. (2018) 134:93–107. doi: 10.1016/j.prostaglandins.2017.09.005
162. McMurtrey RJ. Analytic models of oxygen and nutrient diffusion, metabolism dynamics, and architecture optimization in three-dimensional tissue constructs with applications and insights in cerebral organoids. Tissue Eng Part C Methods. (2016) 22:221–49. doi: 10.1089/ten.tec.2015.0375
163. Gomes A, Guillaume L, Grimes DR, Fehrenbach J, Lobjois V, Ducommun B. Oxygen partial pressure is a rate-limiting parameter for cell proliferation in 3D spheroids grown in physioxic culture condition. PLoS ONE. (2016) 11:e0161239. doi: 10.1371/journal.pone.0161239
164. Park KM, Gerecht S. Hypoxia-inducible hydrogels. Nat Commun. (2014) 5:4075. doi: 10.1038/ncomms5075
165. Lewis DM, Blatchley MR, Park KM, Gerecht S. O2-controllable hydrogels for studying cellular responses to hypoxic gradients in three dimensions in vitro and in vivo. Nat Protoc. (2017) 12:1620–38. doi: 10.1038/nprot.2017.059
166. Dmitriev RI, Kondrashina AV, Koren K, Klimant I, Zhdanov AV, Pakan JMP, et al. Small molecule phosphorescent probes for O2 imaging in 3D tissue models. Biomater Sci. (2014) 2:853–66. doi: 10.1039/C3BM60272A
167. Dmitriev RI, Papkovsky DB. Multi-parametric O2 imaging in three-dimensional neural cell models with the phosphorescent probes. Methods Mol Biol. (2015) 1254:55–71. doi: 10.1007/978-1-4939-2152-2_5
168. Lesher-Pérez SC, Kim G-A, Kuo C, Leung BM, Mong S, Kojima T, et al. Dispersible oxygen microsensors map oxygen gradients in three-dimensional cell cultures. Biomater Sci. (2017) 5:2106–13. doi: 10.1039/C7BM00119C
169. Oppegard SC, Eddington DT. A microfabricated platform for establishing oxygen gradients in 3-D constructs. Biomed Microdevices. (2013) 15:407–14. doi: 10.1007/s10544-013-9737-0
170. Li C, Chaung W, Mozayan C, Chabra R, Wang P, Narayan RK. A new approach for on-demand generation of various oxygen tensions for in vitro hypoxia models. PLoS ONE. (2016) 11:1–13. doi: 10.1371/journal.pone.0155921
171. Langan LM, Dodd NJF, Owen SF, Purcell WM, Jackson SK, Jha AN. Direct measurements of oxygen gradients in spheroid culture system using electron parametric resonance oximetry. PLoS ONE. (2016) 11:e0149492. doi: 10.1371/journal.pone.0149492
Keywords: hypoxia, cell culture, animal model, in vitro model, pericellular oxygen, oxygen concentration, partial pressure, normoxia
Citation: Pavlacky J and Polak J (2020) Technical Feasibility and Physiological Relevance of Hypoxic Cell Culture Models. Front. Endocrinol. 11:57. doi: 10.3389/fendo.2020.00057
Received: 27 April 2019; Accepted: 29 January 2020;
Published: 21 February 2020.
Edited by:
Anca Dana Dobrian, Eastern Virginia Medical School, United StatesReviewed by:
Bilikere S. Dwarakanath, Shanghai Proton and Heavy Ion Center (SPHIC), ChinaCopyright © 2020 Pavlacky and Polak. This is an open-access article distributed under the terms of the Creative Commons Attribution License (CC BY). The use, distribution or reproduction in other forums is permitted, provided the original author(s) and the copyright owner(s) are credited and that the original publication in this journal is cited, in accordance with accepted academic practice. No use, distribution or reproduction is permitted which does not comply with these terms.
*Correspondence: Jan Polak, amFuLnBvbGFrQGxmMy5jdW5pLmN6
Disclaimer: All claims expressed in this article are solely those of the authors and do not necessarily represent those of their affiliated organizations, or those of the publisher, the editors and the reviewers. Any product that may be evaluated in this article or claim that may be made by its manufacturer is not guaranteed or endorsed by the publisher.
Research integrity at Frontiers
Learn more about the work of our research integrity team to safeguard the quality of each article we publish.