- Department of Neurobiology and Physiology, School of Medicine, Zhejiang University, Hangzhou, China
Insulin-like growth factor-binding protein-2 (IGFBP-2) is a pleiotropic polypeptide that functions as autocrine and/or paracrine growth factors. IGFBP-2 is the most abundant of the IGFBPs in the cerebrospinal fluid (CSF), and developing brain showed the highest expression of IGFBP-2. IGFBP-2 expressed in the hippocampus, cortex, olfactory lobes, cerebellum, and amygdala. IGFBP-2 mRNA expression is seen in meninges, blood vessels, and in small cell-body neurons (interneurons) and astrocytes. The expression pattern of IGFBP-2 is often developmentally regulated and cell-specific. Biological activities of IGFBP-2 which are independent of their abilities to bind to insulin-like growth factors (IGFs) are mediated by the heparin binding domain (HBD). To execute IGF-independent functions, some IGFBPs have shown to bind with their putative receptors or to translocate inside the cells. Thus, IGFBP-2 functions can be mediated both via insulin-like growth factor receptor-1 (IGF-IR) and independent of IGF-Rs. In this review, I suggest that IGFBP-2 is not only involved in the growth, development of the brain but also with the regulation of neuronal plasticity to modulate high-level cognitive operations such as spatial learning and memory and information processing. Hence, IGFBP-2 serves as a neurotrophic factor which acts via metaplastic signaling from embryonic to adult stages.
Introduction
The insulin-like growth factor (IGF) system is a mitogenic protein family that includes IGF-I and IGF-II and six binding proteins (IGFBP-I–IGFBP-6) and is involved in functions from embryonic growth to cell differentiation to homeostasis, mostly mediated by IGF-lR (1–3). In the central nervous system (CNS), this system performs various functions, including neurotropic, neuromodulatory, and neuroendocrine ones during embryonic, and postnatal development. This system is responsible for the regulation of brain mass homeostasis and neural stem cell differentiation and proliferation (4–9). In addition, IGFs regulate the growth and differentiation of fetal neurons in culture, and, in addition to promoting synapse formation; IGFs stimulate myelin synthesis and regulate neuronal cytoskeletal protein synthesis with modulation of the expression of immediate early genes (10–13). IGF-system also regulates metabolic functions, including glucose uptake in glial cells, and demonstrates neuromodulatory activities, including enhanced serotonin biosynthesis, inhibition of norepinephrine reuptake by neurons, and maintenance of the Na+/K+ pump in synaptosomes (14). IGF-ll is involved with memory enhancement and consolidation (7). IGF-l signaling yield through the phosphoinositide 3-kinase (PI3K)-AKT and RAS-extracellular signal-related kinase (ERK) cascades via IGF-IR (15) while the IGF-IIR induces signaling through G proteins that activate protein kinase C (PKC) and phospholipase C (PLC), ultimately regulates Ca2+ homeostasis (16).
In the systemic circulation, the bioavailability, and functions of IGF-l and IGF-ll are mainly regulated by six high-affinity IGFBPs. Though IGFBPs share common sequence homology and functions to regulate IGFs actions, individual proteins (IGFBP-1-6) each have their own unique properties and functions. It has been suggested that overexpression of IGFBPs could be a good model to elucidate the physiological functions of individual IGFBPs (17, 18). IGFBP-2 is most abundant in the CSF (19) and highly expressed in the developing brain. In terms of structure, IGFBP-2 consists of three regions: the N-terminal cysteine-rich region, the middle or linker region, and the C-terminal cysteine-rich region (20, 21). Interestingly, IGF-I and IGF-II bind with the N-terminal and C-terminal regions of IGFBP-2 indicates that the high-affinity interactions are mediated by those terminal regions (22, 23). NMR analysis showed that C-terminal region is more prominent in binding with IGF-I and IGF-II and therefore prevent their binding with IGF-IR (24). IGFBP-2 binds with IGFs to control their bioavailability and localization. Thus, IGFBP-2 may act as an important modulator of IGFs signaling in the CNS. Interestingly, local activity-dependent release of IGFs in the CNS also reported. Thus, most important biological functions of either IGFBP-2 or IGFs would be mediated independently in the CNS but passively by IGFBP-2/IGFs complex. Binding of IGFBP-2 to glycosaminoglycans depends on IGFBP-2/IGFs complex (25), however, opposite effect of IGFBP-2 and IGF-I was observed in follicle-stimulating hormone (FSH)-dependent aromatase expression. Thus, deepening on certain physiological conditions, cell type, and tissue/organ, in order to interact with cell surface or ECM, IGFBP-2 may or may not require binding with IGFs. IGFBP-2 also possesses a nuclear localization signal (NLS) sequence within its linker region to interact with Importin-α (26).
In addition, IGFBP-2 possesses several binding domain and among them the two important binding domains (27, 28) are: the Arginine, Glycine, and Aspartate domain (RGD) and the heparin-binding domain (HBD).The RGD domain consists of the sequence Arg-Gly-Asp at peptide position 265RGD267 (the numbering used excludes the signal peptide sequence) in humans and at 246RGD248 in the rat (20, 29) and other species, at C-terminus region (30), and it is responsible for recognition by integrin receptors (31). The RGD domain of IGFBP-2 mediates association with integrins on the cell membrane (32–35). The HBD domain of IGFBP-2 has a consensus sequence for glycosaminoglycan, and, this recognition via HBD is represented by the sequence 160PKKLRP166 in rat IGFBP-2 and 179PKKLRP184 in human IGFBP-2 protein (36). Moreover, the HBD domain in IGFBP-2, the HBD-1, is unique and is not present in other IGFBPs. HBD-1 mediates the binding of IGFBP-2 to cell surface receptor proteins such as tyrosine phosphatase β (37). An additional pH-sensitive heparin-binding site, the HBD-2, shares sequence similarity with other HBD containing IGFBPs such as IGFBP-3 and IGFBP-5 and has been located within the C-terminal region and thyroglobulin type-1 domain of IGFBP-2 [Figure 1; (38)].
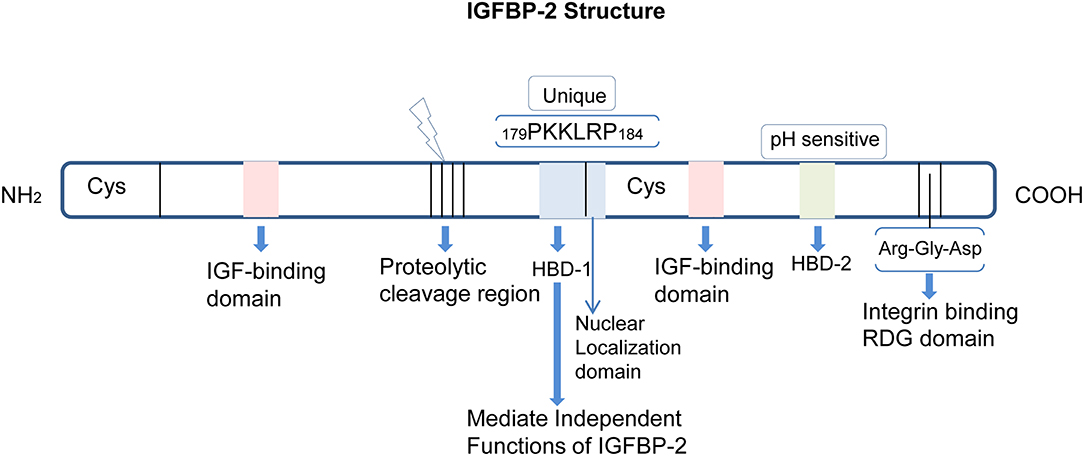
Figure 1. Structure and domains of IGFBP-2. IGFBP-2 consists of N-terminal and C-terminal regions linked by a linker domain. Both the N- and C-terminal have one IGF-binding domain. There is a proteolytic cleavage site between the two terminals. The HBD-1, HBD-2, and RDG domains are located in the cysteine-rich C-terminal region. The nuclear localization domain is adjacent to the HBD1 domain.
Thus, IGFBP-2 is a multifunctional protein that contains IGF-, integrin-, and heparin-binding domains (39), indicating complex regulation and functions of this protein. It has been reported that IGFBP-2 can be cleaved proteolytically, and the smaller fragments that contain HBD still possess the biological activity necessary to bind with extracellular matrix proteins (ECM) and to bind with low affinity to IGF-l (39, 40). Khan et al. (41) showed that the HBD domain of IGFBP-2 independently activates glutamate receptors that translate into enhanced information processing in the hippocampus in a cell type-specific manner, which is crucial for cognitive development at early life in rodents. In addition, IGFBP-2 binding to cell surface proteoglycan in rat olfactory bulb is solely mediated by HBD and not by RGD peptides (39). Mice lacking the RGD domain in IGFBP-2 showed growth impairment compared to wild-type control mice (42) and decreased chondrocyte differentiation, proliferation, and apoptosis in enchondral ossification (43). Moreover, IGFBP-2 enhanced glioma tube formation via RGD domain by interacting with integrin α-5 or β-1 (44). Thus, biological activities of IGFBP-2 can be independent of their abilities to bind to IGFs and these IGF-independent actions can be mediated via the HBD or RGD domains of IGFBP-2.
Transgenic mice overexpressing IGFBP-2 showed reduced body weight without affecting organ mass except in spleen, suggesting that IGFBP-2 is a negative regulator of postnatal growth (45). On the contrary, IGFBP-2 knockout mice revealed no change in body weight, including no effect on brain size or morphology. Targeted inactivation of the IGFBP2 gene in mice resulted in only subtle phenotypic changes, potentially due to functional compensation by other IGFBPs (46). In addition, IGFBP-1, IGFBP-3, and IGFBP-4 levels were increased in IGFBP-2 knockout mice, suggesting the existence of a compensatory mechanism in the absence of IGFBP-2 (46), a further extension to the complexity of IGFBP-2 functions. In rat brain olfactory bulb, IGFBP-2 has been detected with proteoglycans in the interstitial space and on cell membranes of the mitral cell layer (39, 47). Via the HBD domain, IGFBP-2 can bind to different glycosaminoglycans: chondroitin-4 and−6 sulfate, keratan sulfate, aggrecan, heparin, vitronectin, laminin, collagens, and fibronectin, a probable mechanism for IGFBP-2 to bind with ECM proteins prior to complex formation with IGF-I/II (25, 39, 48, 49), suggesting IGFs-independent functions of IGFBP-2 mediated by the HBD domain. Interestingly, proteolytic degradation of IGFBP-2 can occur on the neuronal surface (39), may be the probable mechanism by which the HBD may directly bind with IGF-IR or other receptors (AMPAR, NMDAR, GABAR) on neurons (41, 50). IGFBP-2 also exerts its functions within cells since it has been detected within the nucleus, nuclear surface, and cytoplasm (51). Thus, IGFBP-2 has both extracellular and intracellular functions. Unlike other IGFBPs, IGFBP-2 does not glycosylate. IGFBP-2 is phosphorylated at serine 106 at an analogous position out of the HBD domain, but phosphorylation levels are significantly lower than that of other IGFBPs (e.g., IGFBP-5) (52). Besides the regulation of IGFs functions (53), several IGFBPs have been shown to reveal IGF-independent functions, especially in bone formation (39). However, in the CNS, IGF-independent functions of IGFBP-2 mostly remain unknown.
Expression Pattern Of IGFBP-2 In CNS
In the postnatal period, IGFBP-2 is most abundant in the CNS (53) and the second most abundant IGFBP in circulation (39). During early fetal development, IGFBP-2 is expressed in the neuroepithelium of the telencephalon, whereas in later stages of fetal development, it is concentrated in astroglial cells adjacent to IGF-I expressing projection neurons in the retina, cerebellar cortex, and sensory relay centers such as thalamus (53). In the embryonic CNS, IGFBP-2 is expressed in three types of non-neuronal tissues, such as the epithelium of the choroid plexus which is responsible for the production of CSF; the floor plate which is responsible for neuronal outgrowth from spinal cord commissural neurons; and the infundibulum which is responsible for the production of the posterior pituitary (54).
After birth, IGFBP-2 levels significantly decrease in glial cells, although levels have been reported to increase with age (55). Moreover, IGFBP-2 in the CSF is thought to be locally synthesized by the epithelium of the choroid plexus, rather than derived from plasma crossing the blood-brain barrier (BBB) (56), although it may possible that IGFBP-2 may cross the BBB (1). Interestingly, in humans, central and peripheral expressions of IGFBP-2 differ considerably. The highest concentrations of plasma IGFBP-2 were shown at birth. IGFBP-2 levels declined with age during the early years of life in humans but had little change after puberty (57). On the other hand, Ho and Baxter showed a positive correlation between IGFBP-2 levels in serum with age in healthy individual (58). Moreover, IGFBP-2 levels were found no change throughout the puberty aged 7–20 (59), while Blum et al. showed lower levels of IGFBP2 at puberty (60). Similarly, IGFBP-2 is also differentially expressed in the CNS. Neonatal rat CSF contained more IGFBP-2 than adult CSF. IGFBP-2 found in CSF may also be synthesized locally by glia and neurons (19). In addition, IGFBP-2 differed substantially between serum and plasma (57). Males showed slightly higher values of IGFBP-2 in serum than females (57). Moreover, it may also be possible that IGFBP-2 expression also differs in humans and rodents. During mouse adulthood, IGFBP-2 and IGF-II levels in the CNS and CSF remain elevated (61, 62), in contrast to IGF-I levels which generally decline throughout the CNS following development, except in the olfactory bulb, suggesting that IGFBP-2 may regulate the function of IGF-II in the CNS.
Developing rats with malnutrition showed decreased levels of IGF-I in circulation, but IGF-I and IGF-IR in the hypothalamus and cerebellum were increased with the concomitant reduction of IGFBP-2 in the hypothalamus (63). This suggests independent functions of IGFBP-2 in the CNS to maintain brain mass homeostasis. It is also reported that none of the major sites of IGFBP-2 mRNA accumulation in the CNS contain detectable IGF-II mRNA (54), further suggesting IGFBP-2 mediated developmental processes may be independent of IGFs binding. IGFBP-2 is also expressed by Bergmann glia in the cerebellum and Muller cells and astrocytes of the retina (55). Interestingly, at later stages of brain development, IGFBP-2 expression is directly proportional to the expression of IGF-I (55) in the cerebellum, retina, and developing sensory networks (Figure 2). However, in the developing hippocampus and neocortex, IGFBP-2 expression, particularly in astrocytes, is not directly proportional to the expression of IGF-I (53), suggesting that IGFBP-2 may play a crucial role in the hippocampus that is independent of IGF binding.
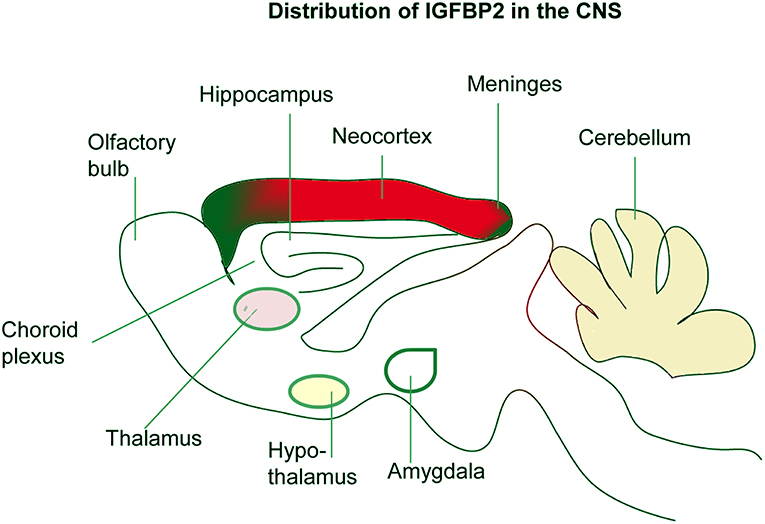
Figure 2. Localization of IGFBP-2 in the brain. IGFBP-2 is abundantly expressed in the hippocampus, neocortex, olfactory bulb, and cerebellum. IGFBP-2 is also found in the meninges, choroid plexus, thalamus, hypothalamus, and amygdala.
Neurotropic and Regenerative Functions OF IGFBP-2 IN CNS
It is been reported that a variety of neurological insults can enhance IGFBP-2 expression. For example, cerebral hypoxic-ischemia in the neonatal rat results in a loss of IGFBP-2 and IGF-IR expression. In addition, prolonged hypoxia decreased the expression of IGFBP-2, whereas shorter hypoxia insult showed an increase above control. In another study, prolonged hypoxia led to enhanced expression of IGFBP-2 with extensive neuronal loss in the ligated hemisphere as compared to the control hemisphere. Therefore, hypoxia leads to alterations in IGFBP-2 expression (41, 64). Different patterns of IGFBP-2 expression associated with different degrees of injury suggest that IGFBP-2 may contribute to neuronal rescue and/or brain repair processes [Figure 3; (65)]. Similarly, cryogenic spinal cord injury in the rat causes an increase in IGFBP-2 and IGF-IR expression in oligodendrocytes (66). IGFBP-2 and IGF-II expression were enhanced but IGF-IIR expression was decreased after cytotoxic lesion of the rat dentate gyrus (DG). Likewise, a marked increase of IGFBP-2 is observed in astrocytes, neurons, and monocytic cells after brain injury (67). Another rat contusion model showed an increase in both IGFBP-2 and IGF-I adjacent to the injured area (68). The same phenomenon is also observed in perineuronal astrocytes following facial nerve transaction (69). In the case of cryogenic spinal cord injury, at 3 days IGF-I mRNA expression was detected. After 14 days of the lesion, both IGF-I and IGFBP-2 expression was detected, suggesting that both of these peptides have independent and cooperative functions at different stage of repair process. At 28 days after lesion, IGF-IR was detected indicating its role at the later stage of repair process. Thus, the nature of interaction between IGFBP-2/GF-IR may be the “fine-tuning” of healing (66). In another study of cerebral wounds, IGF-I, IGFBP-2, and IGF-IR were detected at 1–7 days post-lesion, suggesting a “cooperative nature” of interaction may exist between IGFBP-2 and IGF-IR (67). In hypoxic-ischemic injury model, IGFBP-2 levels significantly enhanced after 2 days but no noticeable change observed in IGF-IR levels, suggesting an independent of IGFBP-2 in repair process (68). Thus, the nature of IGFBP-2 functions and interaction depend on the type of injury. Together, these findings suggest that IGFBP-2 interaction with IGF-IR may participate in neuronal regeneration in the CNS depending on the nature of injury.
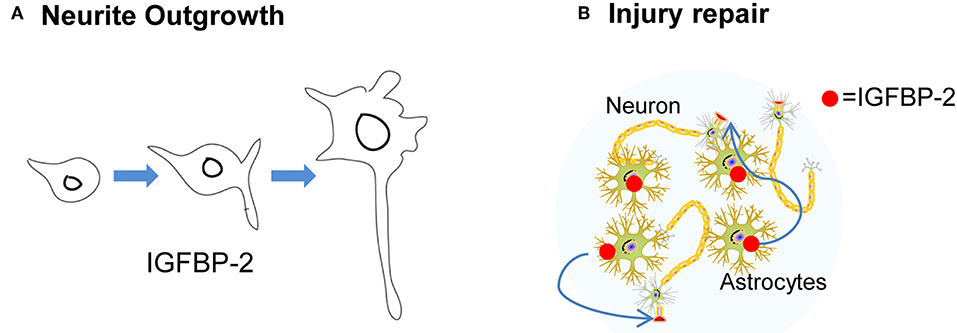
Figure 3. The neurotropic function of IGFBP-2. (A) IGFBP-2 is involved in neurite outgrowth. (B) IGFBP-2 plays a crucial role in CNS injury and repair.
It has been reported that transgenic mice overexpressing IGFBP-2 lacking a specific heparin-binding domain (HBD-1) showed severe deficits in brain growth throughout their lifetime. In addition, these mice when young (12–21 days) had reduced levels of GTPase dynamin-I, and 12 weeks old mice showed weight reduction in the hippocampus, prefrontal cortex, cerebellum, and olfactory bulb, concomitant with reduced myelin basic protein in the cerebellum (17). These data imply that IGFBP-2 is not only involved in brain development but also with information processing and cognition. In addition to supporting neuronal regeneration, IGFBP-2 actions include the ability to promote neurite outgrowth via IGF-IR (70). Shen et al. reported that IGFBP-2 enhances neural stem cells (NSCs) proliferation and maintenance. Moreover, knockdown of IGFBP-2 significantly reduced the expression of cell cycle, differentiation, Notch pathway genes in NSCs (71).
It was found that mice with chronic immobilization stress-induced depressive-like behavior showed reduced expression of IGFBP-2 in the central amygdala (70) and prenatal stress resulted in decreased expression of IGFBP-2 in the hippocampus and frontal cortex in adult male rats (72). When relatively higher concentrations of IGFBP-2 were administered with IGF-II, there was an increased percentage of neurite-bearing cells and an increased average of neurite length (70). Such neurite outgrowth is mediated by IGF-IR associated signaling. During hippocampal neurogenesis at the embryonic stage, there was a dynamic change of gene expression in response to leaf extract of Ginkgo biloba (73). The mRNA level of IGFBP-2 was increased in the fetal hippocampus by prenatal exposure to Ginkgo biloba extract, suggesting that IGFBP-2 may be involved in brain development by acting as both a survival and a differentiation factor for the neural cells (73). High expression of IGFBP-2 in the DG indicates that this protein may be involved in hippocampal neurogenesis. Evidence also suggests that the expression of IGFBP-2 may enhance regenerative sprouting and contribute to neuronal repair in a sensory spinal axonal injury model in the rat (74). On the other hand, mitochondrial dysfunction in Alzheimer's disease (AD) may be associated with enhanced expression of IGFBP-2 (75). AD patients have elevated levels of plasma and CSF IGFBP-2 compare to normal old individuals and plasma IGFBP-2 associated with AD-like brain atrophy (76, 77). Moreover, blood protein analysis showed that IGFBP-2 levels enhanced in serum before the onset of clinical features of AD (78). However, there is a relation between IGFBP-2 and CSF Aβ-42 was observed in the hippocampus. Interestingly, smaller hippocampal volumes associated with higher IGFBP-2 levels only in the amyloid negative individuals (79). Thus, in an age-dependent manner, IGFBP-2 may differentially modulate normal physiological and pathological functions (6, 80).
The ability of IGFBP-2 to promote neuronal survival is associated with its ability to prevent apoptosis. The Bcl-2 (B-cell lymphoma 2) family of proteins is important in the regulation of apoptosis, and BCL-2 mRNA is localized in the hippocampus at the same sites of IGF/IGFBPs expression (81). The IGF/IGFBPs system and the pro-survival Bcl-2 proteins protect cells from apoptosis and play a key role in brain development. Moreover, IGFBP-2 expression is markedly increased around the site of injury (82), thus providing evidence that IGFBP-2 may inhibit neuronal apoptosis. Baker et al. (83) showed that IGFBP-2 exerts anti-apoptotic effects in developing mouse brain probably via Bcl-2 overexpression. This resulted in the induction and overexpression of IGFBP-2 in mitral cells, demonstrating a previously unknown mechanism for cell survival by Bcl-2 via IGFBP-2 in developing brain (83). On the other hand, IGFBP-2 may have some link with psychiatric disorders. For example, serum IGFBP-2 level was significantly higher in schizophrenic patients than control (84, 85). Serum IGFBP-2-protein and -mRNA expression was downregulated in patients with bipolar disease (86, 87), and serum IGFBP-2 protein levels were lower in patients with atypical and melancholic depression than in controls (88). Moreover, it has been reported that the brains of individuals with schizophrenia showed faster aging than normal (89). Mitochondrial dysfunction plays a key role in neuropsychiatric disorders including schizophrenia (90). Thus, senescent cells may have mitochondrial dysfunction and elevated IGFBP-2. On the other hand, fibrotic lung disease is characterized by the presence of senescent cells, where IGFBP-2 expression was moderately high (91). Stress-induced cellular senescence often recognized by the expression of p16Ink4a accumulates in various tissues. Senescence positive cells showed higher expression of IGFBP-2 (92). Thus, IGFBP-2 may associate with cellular senescence related to psychiatric disorders.
The number of IGFBP-2-positive cells was decreased in the hippocampus of a mouse model of amyotrophic lateral sclerosis (ALS) (93). IGF-l is a growth facilitator that propels axon regeneration and can restore corticospinal axon function (94). Chromatin remodeling via histone methylation is critical for peripheral nerve myelination by Schwann cells. Knock-out of Schwann cell-specific subunit of the polycomb repressive complex-2 (PRC-2) called Eed, which catalyzes methylation of histone H3 Lys27 causes hypermyelination of axon. In addition, such knock out of Eed was responsible for enhanced Akt phosphorylation accompanied by decreased expression of IGFBP2 gene (95). Thus, these data in addition to down-regulation of IGFBP-2 in the distal stump of sciatic nerve cut model mice (c-Jun deleted in Schwann cells) (96), implying that IGFBP-2 is a potential factor in the epigenetics pathway for mature myelinated axons.
Bidirectional Effect Of IGFBP-2 On CNS Myelination
It has been reported that IGF-I increases myelination, while IGFBP-1 suppresses myelination (97). Immunoreactivity of IGF-II and IGFBP-2 colocalized on oligodendrocyte membrane and myelin sheaths (98). It may possible that IGFBP-2 is transported on myelin tracks from its sites of synthesis. In another study, IGFBP-2 and IGF-II colocalized in sites remote from their expression sites, especially in the myelin sheaths of axons and nerve tracts in the brain [Figure 4; (99)]. This spatial discrepancy between the sites of synthesis and the sites of localization suggests a bidirectional role of IGFBP-2: transport of IGF-II to its target cells and IGFBP-2's own independent functions. In addition, during encephalomyelitis, IGFBP-2 expression in reactive astrocytes targets oligodendrocytes, expressing IGF-I and IGF-IR, which are responsible for remyelination (100). In contrast, in vitro treatment of primary astrocytes with IGF-I/IGFBP-2 complexes revealed no inhibitory effect on IGF-I induced proliferation, but the same complexes significantly inhibit IGF-I induced survival of oligodendrocytes (101). In addition, peripheral nerve myelination is mediated by IGFBP-2 via Akt activation in Schwann cells (95, 102). Thus, IGFBP-2 may follow differential mechanisms for central and peripheral myelination.
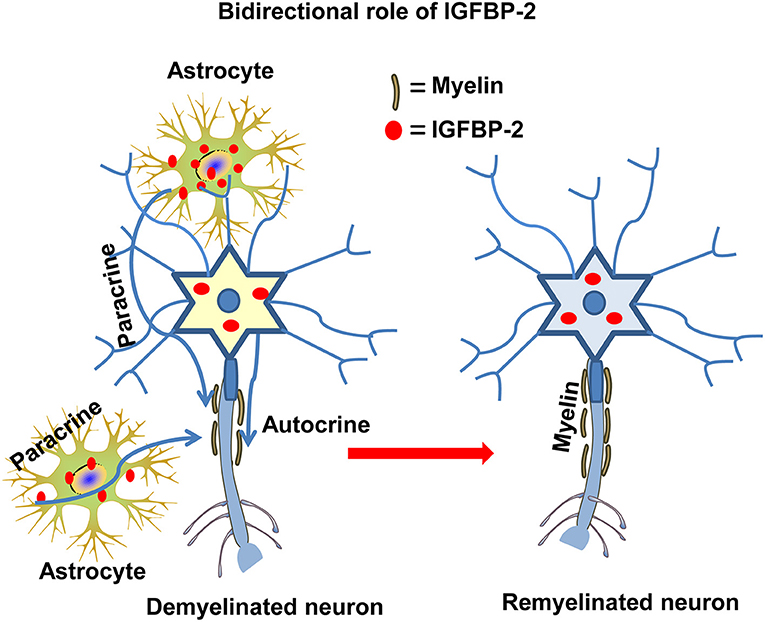
Figure 4. The autocrine and paracrine action of IGFBP-2 in myelination. IGFBP-2 is involved in myelination and myelin repair via autocrine and paracrine mechanisms both in CNS and PNS neurons.
Interestingly, gray matter astrocytes express high levels of IGFBP-2, whereas IGF-I mRNA and IGF-I peptide are not detected. This dissociated astrocytic expression suggests that IGFBP-2 and IGF-I responses may be regulated differentially by neurons. In cryogenic spinal cord injury, it has been reported that IGFBP-2 expression was significantly increased by astrocytes (66), suggesting that IGFBP-2 may have a role in promoting myelin regeneration. However, oligodendrocytes treated with IGFBP-2 revealed decreased expression of myelin protein and myelin-associated glycoprotein (103). In vitro, IGFBP-2 attenuated cell differentiation by reducing IGF-I. In contrast, cryogenic spinal cord injury leading to demyelination and axonal loss resulted in enhanced IGF-I and IGFBP-2 production by astrocytes (104). In another study in the human adult brain, IGFBP-1-6 has been shown in astrocytes but not in microglia (101, 105). Chronic active multiple sclerosis (MS) lesions showed enhanced expression of IGFBP-2 and IGFBP-4 in astrocytes but not in microglia or macrophages. Although the signaling pathway for enhanced expression of IGFBP-2 in MS remains unknown, up-regulation of IGF-I and IGF-II, including proinflammatory cytokines, may be responsible for enhanced IGFBP-2 expression in MS.
Moreover, cytokines and epidermal growth factor (EGF) can induce specific IGFBPs production which plays a role in the cell growth in human salivary cell line (HSG) (106). Thus, Cytokines include IL-1b (interleukin-1b), IL-6 (interleukin-6); TNF-a (Tumor necrosis factor-alpha), and IFN-g (interferon-gamma), all of which are proinflammatory cytokines (hallmark of persistent inflammation), responsible for enhanced expression of IGFBP-2 in astrocytes and microglia and, a feature of active MS (18, 107). Thus, it was hypothesized that IGFBP-2 may protect cells from cytokine release, an effect that is mediated by IGFs and which may enhance astrogliosis (18). In addition, the induction of encephalomyelitis in rats resulted in enhanced expression of IGFBP-2 and IGF-I in astrocytes and coincided with remyelination by targeting promyelinating oligodendrocytes (106). Thus, it is assumed that IGFBP-2 may be a therapeutic target for MS treatment via mechanisms where IGFBP-2 differentially modulates CNS myelination during pathological and normal physiological conditions.
Possible Functions Of IGFBP-2 In The Hippocampus
The components of the IGF system are highly expressed in regions that are undergoing remodeling or enhanced plasticity, such as the hippocampus. It was demonstrated that IGF-IR colocalized to or near sites of IGF/IGFBPs expression in the hippocampus. Moreover, IGFBP-2 binds to cell surface proteoglycans (39). It is suggested, therefore, that there is an autocrine or paracrine mode of IGFBP-2 action in the developing hippocampus (108). Cell type specific expression and distribution of IGFBP-2 suggest complex regulation of IGFBP-2 action in the CNS.
IGF-IR is expressed at high levels during development by most regions of the CNS (1, 109). However, IGF-IR shows significantly different structural characteristics in non-neural tissues from those expressed by the brain. The major difference is that the carbohydrate residues of the peripheral IGF-IR include N-linked high-mannose and contain sialic acid molecules. On the other hand, in the neural IGF-IR, a polymer of sialic acid is present and is resistant to neuraminidase catalysis (110, 111). In some cases, sialic acid residues may be absent in neuronal IGF-lR. Such alterations may be responsible for the different affinities of the ligands with neuronal IGF-IR, which may explain the differential roles of the IGF/IGFBP complex in the brain. Interestingly, glial cells express the non-neuronal type IGF-IR (109, 112), suggesting that neurons are specialized for functions that are different from those of glia.
In developing the hippocampus, IGF-IR mRNA is abundant in pyramidal neurons (PNs), granule cells, and interneurons (111). Moreover, PNs show high levels of IGF-IR gene expression in conjunction with local IGFBP-2 expression (53, 70, 113, 114), demonstrating that IGFBP-2 may act via IGF-IR in the hippocampus. There is relatively stable and uniform level of IGF-IR gene expression in all neuroepithelial lineages, indicating this pattern of receptor distribution may be the target for peripheral IGF/IGFBPs and subserve a very basic metabolic or trophic function, demonstrating that there may be specific local autocrine and/or paracrine IGF/IGFBPs actions mediated by IGF-IR in the brain (108). IGFBP-2 has been reported to prolong and modulate cellular functions independent of IGF-I binding (3), and IGFBP-2 can prevent IGF-I from binding to its receptor (20, 114). Therefore, IGF-IR activation may be required for IGFBP-2 functions. The C-tail region of IGF-IR is the site for binding of different signaling molecules. For IGFBPs binding to IGF-IR, a single detergent molecule contacts residues known to be critical (111). Proteolytic degradation of IGFBP-2 near cell surface receptors has lower affinity for IGFs and thus by competing with IGFs, HBD of IGFBP-2 may bind with that detergent molecule in IGF-IR to initiate downstream signaling via autophosphorylation of the receptor (41). Moreover, the presence or absence of sialic acid residue in the IGF-IR may be another factor for interaction with IGFBP-2.
Moreover, Khan et al. (41) showed that the HBD domain of IGFBP-2 can influence AMPA and GABA receptors reflected by enhanced miniature EPSC and IPSC frequency and amplitudes, while treatment with IGF-IR antagonist (JB-1) significantly reduced those events, suggesting that HBD domain may interact with the IGF-IR. Interestingly, microinjection of IGFBP-2 in wild type (WT) and igfbp2−/− mice showed no change in IGF-I/II levels, indicating no needs IGFs for HBD domain of IGFBP-2 to bind with the receptors (41). It may also be possible that the interaction between IGFBP-2 and IGF-IR may induce a rapid and transient increase in intracellular free calcium concentration (115), which is responsible for enhanced synaptic plasticity. Moreover, the bath application of HBD domain of IGFBP-2 significantly enhanced and rescued long term potentiation (LTP) in WT and igfbp2−/− mice, respectively. In spatial learning and memory test, igfbp2−/− mice showed slower learning profile and spent less time in the target quadrate (41). Thus, IGFBP-2 may differentially modulate both the short-term and long-term synaptic plasticity.
In the HBD domain, IGFBP-2 possesses nuclear localization signal (NLS) motif (116) which performs various non-IGF-IR dependent functions such as IGFBP-2 (HBD domain) involves in bone formation via both IGF-dependent and IGF-independent mechanisms (117–119). Moreover, HDB domain of IGFBP-2 can rescue differentiation and the expression of osteocalcin in igfbp2−/− mice via RPTPβ (120). Moreover, IGFBP-2 inhibits adipogenesis and fat development without interacting with IGFs (121). In addition, IGFBP-2 down-regulates the tumor suppressor gene phosphatase and tensin homolog (PTEN) via an integrin-mediated mechanism to enhance IGF-I induced Akt pathway activation (37). It is worth emphasizing that IGFBP-2 could derive either from neurons or astrocytes (105, 122, 123), because there may be a source independent of local autocrine and/or paracrine action of IGFBP-2 (Figure 4) that is mediated by IGF-IR (102) rather than by the integrin (124).
Electroconvulsive seizure therapy (ECS) is a clinically proven treatment for depression. ECS increases the expression of specific neurotrophic factors that could block or reverse the atrophy and cell loss resulting from stress and depression. Research into the mechanism of action of ECS has reported that expression of neurotrophic-growth factors and related signaling pathways in the hippocampus and in the choroid plexus were enhanced, both areas where IGFBP-2 is highly expressed (125). In adult male rats, IGFBP-2 (1 μg/kg, i.v.) increased the density of mature dendritic spines and total spine density in the DG and medial prefrontal cortex, which is the underlying mechanisms explaining the therapeutic-like effects of IGFBP-2 in post-traumatic stress disorder (PTSD) (126). In most cases, depressive symptoms associated with PTSD (127). Intracerebroventricular (icv) injection of NBI-31772 (10–30 mg; a non-specific IGFBP inhibitor) enhanced anxiolytic-like behavior in four-plate test and elevated zero maze tests. On the contrary, treatment with the same NBI-31772 (3–30 mg) showed decreased immobility time in the tail suspension test, an indicator of antidepressant-like effects (128). In another study, exposure to stressful stimuli showed depression-like behavior in rats accompanied by reduced IGF-l protein levels in the frontal cortex and in the hippocampus with differential expression of IGFBPs. IGFBP-2 and IGFBP-3 levels were decreased while IGFBP-4 was increased with no change in IGFBP-1 and IGFBP-6 in those stressed rats (72). Taken together, these data suggest that IGFBP-2 acts as an antidepressant and anxiolytic agent by reducing stress-induced cell loss or atrophy, which is consistent with the finding that persons with atypical depression showed lower levels of IGFBP-2 (93).
In addition, IGFBP-2 is also involved in neurodegeneration. It has been reported that the accumulation of high levels of Abeta can be toxic, although the alpha-secretase cleaved amyloid precursor protein (APP) is neuroprotective because it increases the expression levels of several neuroprotective genes, including IGFBP-2. Thus, IGFBP-2 may protect hippocampal neurons from Abeta-induced tau phosphorylation and neuronal death in AD (129). This finding is consistent with the notion that knocking out the IGFBP2 gene may reduce hippocampal cell number. In addition, in age impaired rats, G-protein coupled receptors and other signaling processes were down-regulated in the hippocampus, whereas IGFBP-2 expression was unaltered, suggesting that IGFBP-2 may not be involved in G-protein-coupled receptor signaling (130).
Dynamic synaptic plasticity is critical for learning and memory throughout life. It has already been reported that insulin-like peptide plays an important role in neurite outgrowth (1). Moreover, IGFBP-2 increased total spines number in dentate granule neurons of the hippocampus and mature spine density in medial prefrontal cortex (MPFC) layer-V pyramidal neurons (126). Thus, IGFBP-2 may involve in increasing the number of functional synapses which can be the underlying mechanism of enhanced LTP by IGFBP-2. Interestingly, intermittent hypoxia (IH, 16% O2) enhanced spatial learning and memory (41, 131). It was reported that IH enhanced expression of IGFBP-2, IGF-IR, CRF, and CRF-lR in neonatal hippocampus (41, 132). Additionally, CRF enhanced the propagation of neuronal activity from DG to the CA1 region (133). Thus, there may be interplay between IGFBP-2 and CRF leading to enhanced synaptic plasticity (41). Diabetes differentially modulates IGFBP-2 levels in organs (134). In addition, diabetes impairs hippocampal function through glucocorticoid (135), whereas stress (e.g., hypoxia) enhances IGFBP-2 levels in the hippocampus (132, 136). Taken together, this could signal the interplay between IGFBP-2 and stress hormones in the hippocampus in physiological and pathological conditions. In this case, IGFBP-2 may directly modulate neural functions independent of IGF-IR.
Concluding Remarks And Prospects
A variety of signaling pathways and extracellular factors such as IGF-I/II, corticotrophin releasing factor (CRF), glucocorticoid, TGF-beta, interleukin 1, and estradiol may influence IGFBP-2 expression (137). In addition, several physiological conditions such as fasting, stroke, and hypoxia enhanced IGFBP-2 levels and IGFBP-2 locally expressed in the CNS. Thus, there is a complex regulation and mechanism of action of IGFBP-2 in the CNS. IGFBP-2 may act by itself or via with IGF-IR or indirectly via IGF-I and/or IGF-II. As a consequence, IGFBP-2 seems to have four or more mechanisms (Figure 5) of action in the CNS by which it mediates its own independent functions: (1) The binding of the IGFBP-2 via the HBD domain to putative receptors on the cell membrane may stimulate the signaling pathway independent of IGF-R and mediate the effect of IGFBP-2 in a cell-type-specific manner. (2) Since IGFBP-2 co-localized with IGF-IR in the hypothalamus (138), thus IGFBP2 may initiate downstream signaling (1). (3) IGFBP-2 may transport into the nucleus via its nuclear localization signal and cause transcriptional activation of genes (116). (4) IGFBP-2 can bind with the integrin receptor via the RGD domain, which happens in cases of CNS malignancy but not in the normal physiological function of neurons. Different mechanisms are crucial for the different physiological functions of IGFBP-2. For example, binding to a neural membrane receptor (e.g., IGF-IR, AMPAR, GABAR, and NMDAR) via the HBD domain may be responsible for neurotransmission and synaptic plasticity, whereas direct transport to the nucleus may be responsible for neurite outgrowth. In addition, it may also be possible that these mechanisms of IGFBP-2 function may overlap with each other and their interplay may be responsible for the conversion of short-term plasticity to long-term memory. IGFBP-2, therefore, exhibits spatial and temporal patterns of action.
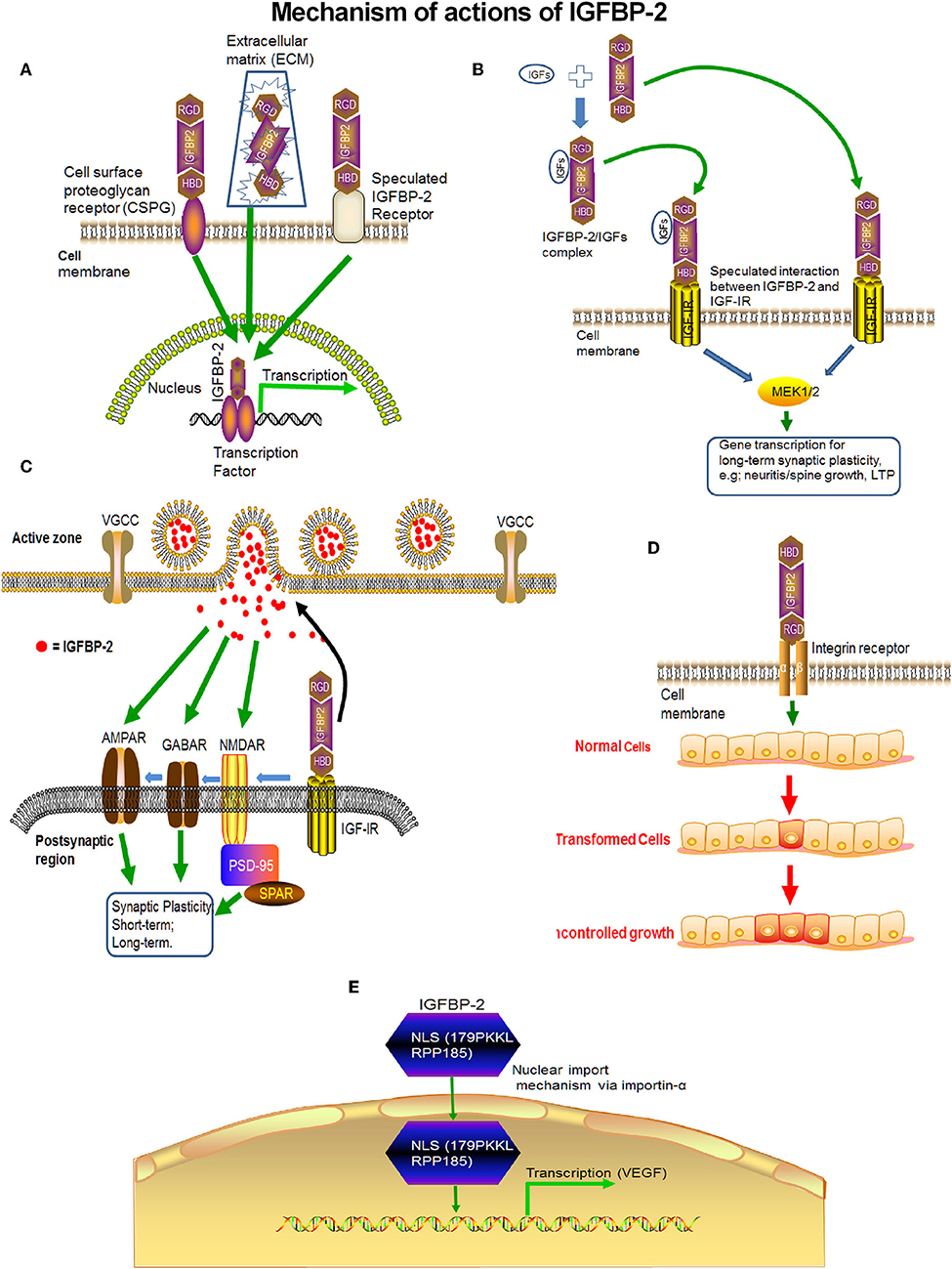
Figure 5. The probable mechanisms of action of IGFBP-2 in the CNS. (A) IGFBP-2 may directly bind with putative IGFBP-2 receptors on the neural membrane and may initiate transcription. (B) IGFBP-2 may bind with IGF-IR via the HBD domain and may initiate downstream signaling via the ERK1/2-MAPK pathway. (C) After binding with IGF-IR via the HBD domain, IGFBP-2 may transfer the signal to AMPAR, GABAR, or NMDAR to modulate neurotransmission for short term synaptic plasticity. (D) IGFBP-2 binds with integrin receptors via the RDG-domain and initiates malignancy. (E) IGFBP-2 may directly cross the cell membrane via nuclear localization signaling and initiate transcription.
IGFBP-2 expression in hippocampal neurons and astrocytes peaks during brain development, coincidently when neuron progenitor proliferation and/or neuritic outgrowth occur, while expression of IGF-IR appears ubiquitous. This expression pattern of IGFBP-2 during brain growth suggests highly regulated and developmentally timed IGFBP-2 actions on specific neural cell populations. Several studies clearly implicate IGFBP-2 involvement in specific higher-order brain functions via spatial and temporal regulations. Thus, IGFBP-2 is not only a binding protein but a crucial CNS growth factor that is responsible for cognition and information processing in the brain (Figure 6). Although targeting of IGFBP-2 signaling to particular cell types remains to be established, IGFBP-2 not only regulates brain development at early embryonic stages but is also crucial in neuronal and adult stages for higher-order brain functions, such as learning, memory, and information processing. IGFBP-2 is revealing its many secrets not only in the embryonic stage but also in other multi-faceted areas from neuroprotection to cognitive function, thus it spans from bench to bedside.
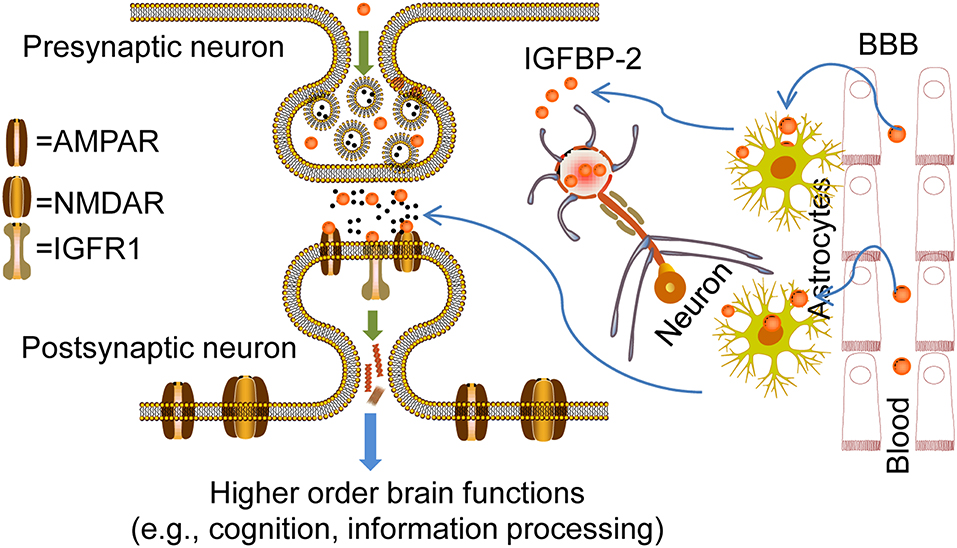
Figure 6. Overview of IGFBP-2 action in CNS for higher order brain functions. Probable mechanisms for the entry of IGFBP-2 across the blood-brain barrier (BBB) to cerebrospinal fluid (CSF): Transport could happen via transcytosis through the choroid plexus where low-density lipoprotein receptor-related proteins-1/2 (LRP-1/2) and IGF-IR may play a crucial role in IGFBP-2 entry from blood to CSF. In this type of entry, concentrations of IGFBP-2 in the blood determine entry. IGFBP-2 in the CSF interacts with IGF-IR in the hippocampus because of its location adjacent to the ventricular system. IGFBP-2 released from astrocytes in response to any kind of CNS insult may directly interact with IGF-IR and be involved in the CNS repair process, neural development, and growth. On the other hand, IGFBP-2 in the neurons is released upon neuronal excitability. In this case, IGFBP-2 may directly bind with the receptors such as AMPAR, GABAR, and NMDAR in the dendrites and axon terminals to mediate synaptic plasticity mechanisms for higher-order brain function, from information processing to cognition. In addition, IGFBP-2 may phosphorylate different ion channels by phosphoinositide 3-kinase (PI3K) or extracellular signal-related kinases (ERKs). IGFBP-2 synthesized inside the neurons may regulate the synthesis of synaptic proteins by direct localization into the nucleus.
Author Contributions
The author confirms being the sole contributor of this work and has approved it for publication.
Conflict of Interest
The author declares that the research was conducted in the absence of any commercial or financial relationships that could be construed as a potential conflict of interest.
Acknowledgments
I dedicate this paper to the memory of Ji-Zeng Du, my dear supervisor, who passed away on June 30, 2017 while this manuscript is in progress.
I acknowledge the manuscript editing by Renee Cockerham (cmNvY2tlcmhhbSYjeDAwMDQwO3NvbS51bWFyeWxhbmQuZWR1), Program Manager, Office of Postdoctoral Scholars, University of Maryland, Baltimore.
Abbreviations
IGFBPs, insulin-like growth factor binding proteins; IGFBP-2, insulin-like growth factor binding protein-2; CSF, cerebrospinal fluid; IGFs, insulin-like growth factors; IGF-I, insulin-like growth factor-1; IGF-II, insulin-like growth factor-2; HBD, heparin binding domain; IGF-IR, insulin-like growth factor receptor-1; IGF-IR, insulin-like growth factor receptor-2; CNS, central nervous system; RGD domain, Arginine-Glycine-Aspartate tripeptide; DG, dentate gyrus; MS, multiple sclerosis; ECM, extracellular matrix; GTPase, guanosine triphosphatase; BBB, blood brain barrier; mRNA, messenger ribonucleic acid; AD, Alzheimer's disease; ALS, amyotrophic lateral sclerosis; Bcl-2, B-cell lymphoma 2; Akt, serine/threonine-specific protein kinase; IL, interleukin; TNF-a, tumor necrosis factor alpha; IFN-g, interferon gamma; AMPAR, α-amino-3-hydroxy-5-methyl-4-isoxazolepropionic acid receptor; GABAR, gamma-aminobutyric acid receptor; NMDAR, N-methyl-D-aspartate receptor; ECS, electroconvulsive seizure therapy; APP, amyloid precursor protein; LTP, long term potentiation; CRF, corticotrophin releasing factor; CRF-lR, corticotrophin releasing factor receptor 1; IH, intermittent hypoxia; CA1, Cornu Ammonis-1; PNs, pyramidal neurons.
References
1. Fernandez AM, Torres AI. The many faces of insulin-like peptide signalling in the brain. Nat Rev Neurosci. (2012) 13:225–39. doi: 10.1038/nrn3209
2. Yu H, Rohan T. Role of the insulin-like growth factor family in cancer development and progression. J Natl Cancer Inst. (2000) 92:1472–89. doi: 10.1093/jnci/92.18.1472
3. Hoeflich A, Reisinger R, Lahm H, Kiess W, Blum WF, Kolb HJ, et al. Insulin-like growth factor-binding protein 2 in tumorigenesis: protector or promoter? Cancer Res. (2001) 61:8601–10.
4. Bianchi VE, Locatelli V, Rizzi L. Neurotrophic and neuroregenerative effects of GH/IGF1. Int J Mol Sci. (2017) 18:E2441. doi: 10.3390/ijms18112441
5. Dyer AH, Vahdatpour C, Sanfeliu A, Tropea D. The role of insulin-like growth factor 1 (IGF-1) in brain development, maturation and neuroplasticity. Neuroscience. (2016) 325:89–99. doi: 10.1016/j.neuroscience.2016.03.056
6. Ziegler AN, Levison SW, Wood TL. Insulin and IGF receptor signalling in neural-stem-cell homeostasis. Nat Rev Endocrinol. (2015) 11:161–70. doi: 10.1038/nrendo.2014.208
7. Chen DY, Stern SA, Garcia OA, Saunier RB, Pollonini G, Bambah MD, et al. A critical role for IGF-II in memory consolidation and enhancement. Nature. (2011) 469:491–7. doi: 10.1038/nature09667
8. Mardinly AR, Spiegel I, Patrizi A, Centofante E, Bazinet JE, Tzeng CP, et al. Sensory experience regulates cortical inhibition by inducing IGF1 in VIP neurons. Nature. (2016) 531:371–5. doi: 10.1038/nature17187
9. Shcheglovitov A, Shcheglovitova O, Yazawa M, Portmann T, Shu R, Sebastiano V, et al. SHANK3 and IGF1 restore synaptic deficits in neurons from 22q13 deletion syndrome patients. Nature. (2013) 503:267–71. doi: 10.1038/nature12618
10. Mozell RL, McMorris FA. Insulin-like growth factor I stimulates oligodendrocyte development and myelination in rat brain aggregate cultures. J Neurosci Res. (1991) 30:382–90. doi: 10.1002/jnr.490300214
11. Recio PE, Lang FF, Ishii DN. Insulin and insulin-like growth factor II permit nerve growth factor binding and the neurite formation response in cultured human neuroblastoma cells. Proc Natl Acad Sci USA. (1984) 81:2562–6. doi: 10.1073/pnas.81.8.2562
12. Kimpinski K, Mearow K. Neurite growth promotion by nerve growth factor and insulin-like growth factor-1 in cultured adult sensory neurons: role of phosphoinositide 3-kinase and mitogen activated protein kinase. J Neurosci Res. (2001) 63:486–99. doi: 10.1002/jnr.1043
13. Mason JL, Xuan S, Dragatsis I, Efstratiadis A, Goldman JE. Insulin-like growth factor (IGF) signaling through type 1 IGF receptor plays an important role in remyelination. J Neurosci. (2003) 23:7710–8. doi: 10.1523/JNEUROSCI.23-20-07710.2003
14. Werner H, Adamo M, Roberts CT, LeRoith D. Molecular and cellular aspects of insulin-like growth factor action. Vitam Horm. (1994) 48;1–58. doi: 10.1016/S0083-6729(08)60495-1
15. Sehat B, Tofigh A, Lin Y, Trocmé E, Liljedahl U, Lagergren J, et al. SUMOylation mediates the nuclear translocation and signaling of the IGF-1 receptor. Sci Signal. (2010) 3:ra10. doi: 10.1126/scisignal.2000628
16. Hawkes C, Jhamandas JH, Harris KH, Fu W, MacDonald RG, Kar S. Single transmembrane domain insulin-like growth factor-II/mannose-6-phosphate receptor regulates central cholinergic function by activating a G-protein-sensitive, protein kinase C-dependent pathway. J Neurosci. (2006) 26:585–96. doi: 10.1523/JNEUROSCI.2730-05.2006
17. Schindler N, Mayer J, Saenger S, Gimsa U, Walz C, Brenmoehl J, et al. Phenotype analysis of male transgenic mice overexpressing mutant IGFBP-2 lacking the Cardin-Weintraub sequence motif: reduced expression of synaptic markers and myelin basic protein in the brain and a lower degree of anxiety-like behaviour. Growth Horm IGF Res. (2017) 33:1–8. doi: 10.1016/j.ghir.2016.11.003
18. Chesik D, De KJ, Wilczak N. Insulin-like growth factor binding protein-2 as a regulator of IGF actions in CNS: implications in multiple sclerosis. Cytokine Growth Factor Rev. (2007) 18:267–78. doi: 10.1016/j.cytogfr.2007.04.001
19. Ocrant I, Fay CT, Parmelee JT. Characterization of insulin-like growth factor binding proteins produced in the rat central nervous system. Endocrinology. (1990) 127:1260–7. doi: 10.1210/endo-127-3-1260
20. Firth SM, Baxter RC. Cellular actions of the insulin-like growth factor binding proteins. Endocr Rev. (2002) 23:824–54. doi: 10.1210/er.2001-0033
21. Galea CA, Mobli M, McNeil KA, Mulhern TD, Wallace JC, King GF, et al. Insulin-like growth factor binding protein-2: NMR analysis and structural characterization of the N-terminal domain. Biochimie. (2012) 94:608–16. doi: 10.1016/j.biochi.2011.09.012
22. Russo VC, Rekaris G, Baker NL, Bach LA, Werther GA. Basic fibroblast growth factor induces proteolysis of secreted and cell membrane-associated insulin-like growth factor binding protein-2 in human neuroblastoma cells. Endocrinology. (1999) 140:3082–90. doi: 10.1210/endo.140.7.6771
23. Hobba GD, Forbes BE, Parkinson EJ, Francis GL, Wallace JC. The insulin-like growth factor (IGF) binding site of bovine insulin-like growth factor binding protein-2 (bIGFBP-2) probed by iodination. J Biol Chem. (1996) 271:30529–36. doi: 10.1074/jbc.271.48.30529
24. Carrick FE, Hinds MG, McNeil KA, Wallace JC, Forbes BE, Norton RS. Interaction of insulin-like growth factor (IGF)-I and -II with IGF binding protein-2: mapping the binding surfaces by nuclear magnetic resonance. J Mol Endocrinol. (2005) 34:685–98. doi: 10.1677/jme.1.01756
25. Arai T, Busby W Jr, Clemmons DR. Binding of insulin-like growth factor (IGF) I or II to IGF-binding protein-2 enables it to bind to heparin and extracellular matrix. Endocrinology. (1996) 137:4571–5. doi: 10.1210/endo.137.11.8895319
26. Chelsky D, Ralph R, Jonak G. Sequence requirements for synthetic peptide-mediated translocation to the nucleus. Mol Cell Biol. (1989) 9:2487–92. doi: 10.1128/MCB.9.6.2487
27. Fowlkes JL, Serra DM. Characterization of glycosaminoglycan-binding domains present in insulin-like growth factor-binding protein-3. J Biol Chem. (1996) 271:14676–9. doi: 10.1074/jbc.271.25.14676
28. Jones JI, Gockerman A, Busby WH Jr, Wright G, Clemmons DR. Insulin-like growth factor binding protein 1 stimulates cell migration and binds to the alpha 5 beta 1 integrin by means of its Arg-Gly-Asp sequence. Proc Natl Acad Sci USA. (1993) 90:10553–7. doi: 10.1073/pnas.90.22.10553
29. Hoeflich A, Reisinger R, Vargas GA, Elmlinger MW, Schuett B, Jehle PM, et al. Mutation of the RGD sequence does not affect plasma membrane association and growth inhibitory effects of elevated IGFBP-2 in vivo. FEBS Lett. (2002) 523:63–7. doi: 10.1016/S0014-5793(02)02935-6
30. Daza DO, Sundstrom G, Bergqvist CA, Duan C, Larhammar D. Evolution of the insulin-like growth factor binding protein (IGFBP) family. Endocrinology. (2011) 152:2278–89. doi: 10.1210/en.2011-0047
31. Wang GK, Hu L, Fuller GN, Zhang W. An interaction between insulin-like growth factor-binding protein 2 (IGFBP2) and integrin alpha5 is essential for IGFBP2-induced cell mobility. J Biol Chem. (2006) 281:14085–91. doi: 10.1074/jbc.M513686200
32. Song SW, Fuller GN, Khan A, Kong S, Shen W, Taylor E, et al. IIp45, an insulin-like growth factor binding protein 2 (IGFBP-2) binding protein, antagonizes IGFBP-2 stimulation of glioma cell invasion. Proc Natl Acad Sci USA. (2003) 100:13970–5. doi: 10.1073/pnas.2332186100
33. Frommer KW, Reichenmiller K, Schutt BS, Hoeflich A, Ranke MB, Dodt G, et al. IGF-independent effects of IGFBP-2 on the human breast cancer cell line Hs578T. J Mol Endocrino. (2006) 37:13–23. doi: 10.1677/jme.1.01955
34. Pereira JJ, Meyer T, Docherty SE, Reid HH, Marshall J., Thompson EW, et al. Bimolecular interaction of insulin-like growth factor (IGF) binding protein-2 with alphavbeta3 negatively modulates IGF-I-mediated migration and tumor growth. Cancer Res. (2004) 64:977–84. doi: 10.1158/0008-5472.CAN-03-3056
35. Perks CM, Vernon EG, Rosendahl AH, Tonge D, Holly JM. IGF-II and IGFBP-2 differentially regulate PTEN in human breast cancer cells. Oncogene. (2007) 26:5966–72. doi: 10.1038/sj.onc.1210397
36. Russo VC, Schutt BS, Andaloro E, Ymer SI, Hoeflich A, Ranke MB, et al. Insulin-like growth factor binding protein-2 binding to extracellular matrix plays a critical role in neuroblastoma cell proliferation, migration, and invasion. Endocrinology. (2005) 146:4445–55. doi: 10.1210/en.2005-0467
37. Shen X, Xi G, Maile LA, Wai C, Rosen CJ, Clemmons DR. Insulin-like growth factor (IGF) binding protein 2 functions coordinately with receptor protein tyrosine phosphatase and the IGF-I receptor to regulate IGF-I-stimulated signaling. Mol Cell Biol. (2012) 32:4116–30. doi: 10.1128/MCB.01011-12
38. Kuang Z, Yao S, Keizer DW, Wang CC, Bach LA, Forbes BE, et al. Structure, dynamics and heparin binding of the C-terminal domain of insulin-like growth factor-binding protein-2 (IGFBP-2). J Mol Biol. (2006) 364:690–704. doi: 10.1016/j.jmb.2006.09.006
39. Russo VC, Azar WJ, Yau SW, Sabin MA, Werther GA. IGFBP-2: the dark horse in metabolism and cancer. Cytokine Growth Factor Rev. (2015) 26:329–46. doi: 10.1016/j.cytogfr.2014.12.001
40. Collett SPF, Cohen P. The role of the insulin-like growth factor binding proteins and the IGFBP proteases in modulating IGF action. Endocrinol Metab Clin North Am. (1996) 25:591–614. doi: 10.1016/S0889-8529(05)70342-X
41. Khan S, Lu X, Huang Q, Tang J, Weng J, Yang Z, et al. IGFBP2 plays an essential role in cognitive development during early life. Adv Sci. (2019). doi: 10.1002/advs.201901152. [Epub ahead of print].
42. Hoeflich A, Reyer A, Ohde D, Schindler N, Brenmoehl J, Spitschak M, et al. Dissociation of somatic growth, time of sexual maturity, and life expectancy by overexpression of an RGD-deficient IGFBP-2 variant in female transgenic mice. Aging Cell. (2016) 15:111–7. doi: 10.1111/acel.12413
43. Inoue T, Hashimoto R, Matsumoto A, Jahan E, Rafiq AM, Udagawa J, et al. In vivo analysis of Arg-Gly-Asp sequence/integrin α5β1-mediated signal involvement in embryonic enchondral ossification by exo utero development system. J Bone Miner Res. (2014) 29:1554–63. doi: 10.1002/jbmr.2166
44. Liu Y, Li F, Yang YT, Xu XD, Chen JS, Chen TL, et al. IGFBP2 promotes vasculogenic mimicry formation via regulating CD144 and MMP2 expression in glioma.Oncogene. (2018) 38:1815–31. doi: 10.1038/s41388-018-0525-4
45. Hoeflich A, Wu M, Mohan S, Foll J, Wanke R, Froehlich T, el al. Overexpression of insulin-like growth factor-binding protein-2 in transgenic mice reduces postnatal body weight gain. Endocrinology. (1999) 140:5488–96. doi: 10.1210/endo.140.12.7169
46. Wood TL, Rogler LE, Czick ME, Schuller AG, Pintar JE. Selective alterations in organ sizes in mice with a targeted disruption of the insulin-like growth factor binding protein-2 gene. Mol Endocrinol. (2000) 14:1472–82. doi: 10.1210/mend.14.9.0517
47. Russo VC, Bach LA, Werther GA. Cell membrane association of insulin-like growth factor binding protein-2 (IGFBP-2) in the rat brain olfactory bulb. Prog Growth Factor Res. (1995) 6:329–36. doi: 10.1016/0955-2235(95)00018-6
48. Conover CA, Khosla S. Role of extracellular matrix in insulin-like growth factor (IGF) binding protein-2 regulation of IGF-II action in normal human osteoblasts. Growth Horm IGF Res. (2003) 13:328–35. doi: 10.1016/S1096-6374(03)00092-3
49. Russo VC, Gluckman PD, Feldman EL, Werther GA. The insulin-like growth factor system and its pleiotropic functions in brain. Endocr Rev. (2005) 26:916–43. doi: 10.1210/er.2004-0024
50. Allard JB, Duan C. IGF-binding proteins: why do they exist and why are there so many? Front Endocrinol. (2018) 9:117. doi: 10.3389/fendo.2018.00117
51. Oeflich A, Reisinger R, Schuett BS, Elmlinger MW, Russo VC, Vargas GA, et al. Peri/nuclear localization of intact insulin-like growth factor binding protein-2 and a distinct carboxyl-terminal IGFBP-2 fragment in vivo. Biochem Biophys Res Commun. (2004) 324:705–10. doi: 10.1016/j.bbrc.2004.09.111
52. Graham ME, Kilby DM, Firth SM, Robinson PJ, Baxter RC. The In vivo phosphorylation and glycosylation of human insulin-like growth factor-binding protein-5. Mol Cell Proteomics. (2007) 6:1392–405. doi: 10.1074/mcp.M700027-MCP200
53. Lee WH, Michels KM, Bondy CA. Localization of insulin-like growth factor binding protein-2 messenger RNA during postnatal brain development: correlation with insulin-like growth factors I and II. Neuroscience. (1993) 53:251–65. doi: 10.1016/0306-4522(93)90303-W
54. Wood TL, Brown AL, Rechler MM, Pintar JE. The expression pattern of an insulin-like growth factor (IGF)-binding protein gene is distinct from IGF-II in the midgestational rat embryo. Mol Endocrinol. (1990) 4:1257–63. doi: 10.1210/mend-4-8-1257
55. Lee WH, Javedan S, Bondy CA. Coordinate expression of insulin-like growth factor system components by neurons and neuroglia during retinal and cerebellar development. J Neurosci. (1992) 12:4737–44. doi: 10.1523/JNEUROSCI.12-12-04737.1992
56. Tseng LY, Brown AL, Yang YW, Romanus JA, Orlowski CC, Taylor T, et al. The fetal rat binding protein for insulin-like growth factors is expressed in the choroid plexus and cerebrospinal fluid of adult rats. Mol Endocrinol. (1989) 3:1559–68. doi: 10.1210/mend-3-10-1559
57. Yu H, Mistry J, Nicar MJ, Khosravi MJ, Diamandis A, van Doorn J, et al. Insulin-like growth factors (IGF-I, free IGF-I and IGF-II) and insulin-like growth factor binding proteins (IGFBP-2, IGFBP-3, IGFBP-6, and ALS) in blood circulation. J Clin Lab Anal. (1999) 13:166–72. doi: 10.1002/(SICI)1098-2825(1999)13:4<166::AID-JCLA5>3.0.CO;2-X
58. Ho PJ, Baxter RC. Insulin-like growth factor-binding protein-2 in patients with prostate carcinoma and benign prostatic hyperplasia. Clin Endocrinol. (1997) 46:333–42. doi: 10.1046/j.1365-2265.1997.1100922.x
59. Juul A, Dalgaard P, Blum WF, Bang P, Hall K, Michaelsen KF. Serum levels of insulin-like growth factor (IGF)-binding protein-3 (IGFBP-3) in healthy infants, children, and adolescents: the relation to IGF-I, IGF-II, IGFBP-1, IGFBP-2, age, sex, body mass index, and pubertal maturation. J Clin Endocrinol Metab. (1995) 80:2534–42. doi: 10.1210/jcem.80.8.7543116
60. Blum WF, Horn N, Kratzsch J, Jørgensen JO, Juul A, Teale D. Clinical studies of IGFBP-2 by radioimmunoassay. Growth Regul. (1993) 3:100–4.
61. Wolf E, Kramer R, Blum WF, Foll J, Brem G. Consequences of postnatally elevated insulin-like growth factor-II in transgenic mice: endocrine changes and effects on body and organ growth. Endocrinology. (1994) 135:1877–86. doi: 10.1210/endo.135.5.7525257
62. Reijnders CM, Koster JG, van Buul-Offers SC. Overexpression of human IGF-II mRNA in the brain of transgenic mice modulates IGFBP-2 gene expression in the medulla oblongata. J Endocrinol. (2004) 182:445–55. doi: 10.1677/joe.0.1820445
63. Chowen JA, Goya L, Ramos S, Busiguina S, Garcia SLM, Argente J, et al. Effects of early undernutrition on the brain insulin-like growth factor-I system. J Neuroendocrinol. (2004) 14:163–9. doi: 10.1046/j.0007-1331.2001.00758.x
64. Beilharz EJ, Russo VC, Butler G, Baker NL, Connor B, Sirimanne ES, et al. Co-ordinated and cellular specific induction of the components of the IGF/IGFBP axis in the rat brain following hypoxic-ischemic injury. Brain Res Mol Brain Res. (1998) 59:119–34. doi: 10.1016/S0169-328X(98)00122-3
65. Klempt M, Klempt ND, Gluckman PD. Hypoxia and hypoxia/ischemia affect the expression of insulin-like growth factor binding protein 2 in the developing rat brain. Brain Res Mol Brain Res. (1993) 17:347–50. doi: 10.1016/0169-328X(93)90021-G
66. Yao DL, West NR, Bondy CA, Brenner M, Hudson LD, Zhou J, et al. Cryogenic spinal cord injury induces astrocytic gene expression of insulin-like growth factor I and insulin-like growth factor binding protein 2 during myelin regeneration. J Neurosci Res. (1995) 40:647–59. doi: 10.1002/jnr.490400510
67. Walter HJ, Berry M, Hill DJ, Logan A. Spatial and temporal changes in the insulin-like growth factor (IGF) axis indicate autocrine/paracrine actions of IGF-I within wounds of the rat brain. Endocrinology. (1997) 138:3024–34. doi: 10.1210/endo.138.7.5284
68. Sandberg NAC, von HH, Holmin S, Sara VR, Bellander BM, Schalling M. Increase of insulin-like growth factor (IGF)-1, IGF binding protein-2 and−4 mRNAs following cerebral contusion. Brain Res Mol Brain Res. (1996) 38:285–93. doi: 10.1016/0169-328X(95)00346-T
69. Gehrmann J, Yao DL, Bonetti B, Bondy CA, Brenner M, Zhou J, et al. Expression of insulin-like growth factor-I and related peptides during motoneuron regeneration. Exp Neurol. (1994) 128:202–10. doi: 10.1006/exnr.1994.1128
70. Jeong EY, Kim S, Jung S, Kim G, Son H, Lee DH, et al. Enhancement of IGF-2-induced neurite outgrowth by IGF-binding protein-2 and osteoglycin in SH-SY5Y human neuroblastoma cells. Neurosci Lett. (2013) 548:249–54. doi: 10.1016/j.neulet.2013.05.038
71. Shen F, Song C, Liu Y, Zhang J, Wei Song S. IGFBP2 promotes neural stem cell maintenance and proliferation differentially associated with glioblastoma subtypes. Brain Res. (2019) 1704:174–86. doi: 10.1016/j.brainres.2018.10.018
72. Basta KA, Szczesny E, Glombik K, Slusarczyk J, Trojan E, Tomaszewski KA, et al. Prenatal stress leads to changes in IGF-1 binding proteins network in the hippocampus and frontal cortex of adult male rat. Neuroscience. (2014) 274:59–68. doi: 10.1016/j.neuroscience.2014.05.010
73. Li W, Trovero F, Cordier J, Wang Y, Drieu K, Papadopoulos V. Prenatal exposure of rats to Ginkgo biloba extract (EGb 761) increases neuronal survival/growth and alters gene expression in the developing fetal hippocampus. Brain Res Dev Brain Res. (2003) 144:169–80. doi: 10.1016/S0165-3806(03)00168-8
74. Roet KC, Franssen EH, de Bree FM, Essing AH, Zijlstra SJ, Fagoe ND, et al. A multilevel screening strategy defines a molecular fingerprint of proregenerative olfactory ensheathing cells and identifies SCARB2, a protein that improves regenerative sprouting of injured sensory spinal axons. J. Neurosci. (2013) 33:11116–35. doi: 10.1523/JNEUROSCI.1002-13.2013
75. Oka S, Leon J, Sakumi K, Ide T, Kang D, LaFerla FM, et al. Human mitochondrial transcriptional factor A breaks the mitochondria-mediated vicious cycle in Alzheimer's disease. Sci Rep. (2016) 6:37889. doi: 10.1038/srep37889
76. Toledo JB, Da X, Bhatt P, Wolk DA, Arnold SE, Shaw LM, et al. Relationship between plasma analytes and SPARE-AD defined brain atrophy patterns in ADNI. PLoS ONE. (2013) 8:e55531. doi: 10.1371/journal.pone.0055531
77. Hu WT, Holtzman DM, Fagan AM, Shaw LM, Perrin R, Arnold SE. Plasma multianalyte profiling in mild cognitive impairment and Alzheimer disease. Neurology. (2012) 79:897–905. doi: 10.1212/WNL.0b013e318266fa70
78. Lista S, Faltraco F, Prvulovic D, Hampel H. Blood and plasma-based proteomic biomarker research in Alzheimer's disease. Prog Neurobiol. (2013) 101–2:1–17. doi: 10.1016/j.pneurobio.2012.06.007
79. Lane EM, Hohman TJ, Jefferson AL. Insulin-like growth factor binding protein-2 interactions with Alzheimer's disease biomarkers. Brain Imaging Behav. (2017) 11:1779–86. doi: 10.1007/s11682-016-9636-0
80. Chen RL, Kassem NA, Sadeghi M, Preston JE. Insulin-like growth factor-II uptake into choroid plexus and brain of young and old sheep. J Gerontol A Biol Sci Med Sci. (2008) 63:141–8. doi: 10.1093/gerona/63.2.141
81. Castren E, Ohga Y, Berzaghi MP, Tzimagiorgis G, Thoenen H, Lindholm D. bcl-2 messenger RNA is localized in neurons of the developing and adult rat brain. Neuroscience. (1994) 61:165–77. doi: 10.1016/0306-4522(94)90069-8
82. Klempt ND, Klempt M, Gunn AJ, Singh K, Gluckman PD. Expression of insulin-like growth factor-binding protein 2 (IGF-BP 2) following transient hypoxia-ischemia in the infant rat brain. Brain Res Mol Brain Res. (1992) 15:55–61. doi: 10.1016/0169-328X(92)90151-Z
83. Baker NL, Carlo RV, Bernard O, D'Ercole AJ, Werther GA. Interactions between bcl-2 and the IGF system control apoptosis in the developing mouse brain. Brain Res Dev Brain Res. (1999) 118:109–18. doi: 10.1016/S0165-3806(99)00136-4
84. Schwarz E, Guest PC, Rahmoune H, Harris LW, Wang L, Leweke FM, et al. Identification of a biological signature for schizophrenia in serum. Mol Psychiatry. (2012) 17:494–502. doi: 10.1038/mp.2011.42
85. Chan MK, Krebs MO, Cox D, Guest PC, Yolken RH, Rahmoune H, et al. Development of a blood-based molecular biomarker test for identification of schizophrenia before disease onset. Transl Psychiatry. (2015) 5:e601. doi: 10.1038/tp.2015.91
86. Milanesi E, Zanardini R, Rosso G, Maina G, Barbon A, Mora C, et al. Insulin-like growth factor binding protein 2 in bipolar disorder: an expression study in peripheral tissues. World J Biol Psychiatry. (2018) 19:610–8. doi: 10.1080/15622975.2017.1282172
87. Benedetti F, Poletti S, Hoogenboezem TA, Mazza E, Ambree O, de Wit H, et al. Inflammatory cytokines influence measures of white matter integrity in Bipolar disorder. J Affect Disord. (2016) 202:1–9. doi: 10.1016/j.jad.2016.05.047
88. Lamers F, Bot M, Jansen R, Chan MK, Cooper JD, Bahn S, et al. Serum proteomic profiles of depressive subtypes. Transl Psychiatry. (2016) 6:e851. doi: 10.1038/tp.2016.115
89. Kaufmann T, van-der Meer D, Doan NT, Schwarz E, Lund MJ, Agartz I, et al. Common brain disorders are associated with heritable patterns of apparent aging of the brain. Nat Neurosci. (2019) 22:1617–23. doi: 10.1038/s41593-019-0471-7
90. Filiou MD, Sandi C. Anxiety and brain mitochondria: a bidirectional crosstalk. Trends Neurosci. (2019) 42:573–88. doi: 10.1016/j.tins.2019.07.002
91. Schafer MJ, White TA, Iijima K, Haak AJ, Ligresti G, Atkinson EJ, et al. Cellular senescence mediates fibrotic pulmonary disease. Nat Commun. (2017) 8:14532. doi: 10.1038/ncomms14532
92. Baker DJ, Childs BG, Durik M, Wijers ME, Sieben CJ, Zhong J, et al. Naturally occurring p16(Ink4a)-positive cells shorten healthy lifespan. Nature. (2016) 530:184–9. doi: 10.1038/nature16932
93. Sim SE, Chung YH, Jeong JH, Yun SW, Lim HS, Kim D, et al. Immunohistochemical localization of insulin-like growth factor binding protein 2 in the central nervous system of SOD1(G93A) transgenic mice. J Mol Histol. (2009) 40:157–63. doi: 10.1007/s10735-009-9219-0
94. Liu Y, Wang X, Li W, Zhang Q, Li Y, Zhang Z, et al. A sensitized IGF1 treatment restores corticospinal axon-dependent functions. Neuron. (2017) 95:817–3.e4. doi: 10.1016/j.neuron.2017.07.037
95. Ma KH, Hung HA, Srinivasan R, Xie H, Orkin SH, Svaren J. Regulation of peripheral nerve myelin maintenance by gene repression through polycomb repressive complex 2. J Neurosci. (2015) 35:8640–52. doi: 10.1523/JNEUROSCI.2257-14.2015
96. Arthur FPJ, Latouche M, Wilton DK, Quintes S, Chabrol E, Banerjee A, et al. c-Jun reprograms Schwann cells of injured nerves to generate a repair cell essential for regeneration. Neuron. (2012) 75:633–47. doi: 10.1016/j.neuron.2012.06.021
97. Ye P, Carson J, D'Ercole AJ. In vivo actions of insulin-like growth factor-I (IGF-I) on brain myelination: studies of IGF-I and IGF binding protein-1 (IGFBP-1) transgenic mice. J Neurosci. (1995) 15:7344–56. doi: 10.1523/JNEUROSCI.15-11-07344.1995
98. Ye P, Li L, Richards RG, DiAugustine RP, D'Ercole AJ. Myelination is altered in insulin-like growth factor-I null mutant mice. J Neurosci. (2002) 22:6041–51. doi: 10.1523/JNEUROSCI.22-14-06041.2002
99. Logan A, Gonzalez AM, Hill DJ, Berry M, Gregson NA, Baird A. Coordinated pattern of expression and localization of insulin-like growth factor-II (IGF-II) and IGF-binding protein-2 in the adult rat brain. Endocrinology. (1994) 135:2255–64. doi: 10.1210/endo.135.5.7525264
100. Liu X, Yao DL, Bondy CA, Brenner M, Hudson LD, Zhou J, et al. Astrocytes express insulin-like growth factor-I (IGF-I) and its binding protein, IGFBP-2, during demyelination induced by experimental autoimmune encephalomyelitis. Mol Cell Neurosci. (1994) 5:418–30. doi: 10.1006/mcne.1994.1052
101. Chesik D, De KJ, Glazenburg L, Wilczak N. Insulin-like growth factor binding proteins: regulation in chronic active plaques in multiple sclerosis and functional analysis of glial cells. Eur J Neurosci. (2006) 24:1645–52. doi: 10.1111/j.1460-9568.2006.05034.x
102. Ma KH, Hung HA, Svaren J. Epigenomic regulation of schwann cell reprogramming in peripheral nerve injury. J Neurosci. (2016) 36:9135–47. doi: 10.1523/JNEUROSCI.1370-16.2016
103. Kuhl NM, De KJ, De VH, Hoekstra D. Insulin-like growth factor binding proteins-1 and−2 differentially inhibit rat oligodendrocyte precursor cell survival and differentiation in vitro. J Neurosci Res. (2002) 69:207–16. doi: 10.1002/jnr.10293
104. Yao DL, Liu X, Hudson LD, Webster HD. Insulin-like growth factor I treatment reduces demyelination and up-regulates gene expression of myelin-related proteins in experimental autoimmune encephalomyelitis. Proc Natl Acad Sci USA. (1995) 92:6190–4. doi: 10.1073/pnas.92.13.6190
105. Chesik D, De KJ, Wilczak N. Involvement of insulin-like growth factor binding protein-2 in activated microglia as assessed in post mortem human brain. Neurosci Lett. (2004) 362:14–6. doi: 10.1016/j.neulet.2004.01.039
106. Katz J, Weiss H, Goldman B, Kanety H, Stannard B, LeRoith D, et al. Cytokines and growth factors modulate cell growth and insulin-like growth factor binding protein secretion by the human salivary cell line (HSG). J Cell Physiol. (1995) 165:223–7. doi: 10.1002/jcp.1041650202
107. Streit WJ, Walter SA, Pennell NA. Reactive microgliosis. Prog Neurobiol. (1999) 57:563–81. doi: 10.1016/S0301-0082(98)00069-0
108. Bondy C, Werner H, Roberts CT Jr, LeRoith D. Cellular pattern of type-I insulin-like growth factor receptor gene expression during maturation of the rat brain: comparison with insulin-like growth factors I and II. Neuroscience. (1992) 46:909–23. doi: 10.1016/0306-4522(92)90193-6
109. Chernausek SD, Murray MA, Cheung PT. Expression of insulin-like growth factor binding protein-4 (IGFBP-4) by rat neural cells–comparison to other IGFBPs. Regul Pept. (1993) 48:123–32. doi: 10.1016/0167-0115(93)90341-5
110. Burgess SK, Jacobs S, Cuatrecasas P, Sahyoun N. Characterization of a neuronal subtype of insulin-like growth factor I receptor. J Biol Chem. (1987) 262:1618–22.
111. Vajdos FF, Ultsch M, Schaffer ML, Deshayes KD, Liu J, Skelton NJ, et al. Crystal structure of human insulin-like growth factor-1: detergent binding inhibits binding protein interactions. Biochemistry. (2001) 40:11022–9. doi: 10.1021/bi0109111
112. Shemer J, Raizada MK, Masters BA, Ota A, LeRoith D. Insulin-like growth factor I receptors in neuronal and glial cells. Characterization and biological effects in primary culture. J Biol Chem. (1987) 262:7693–9.
113. Heidenreich KA, Freidenberg GR, Figlewicz DP, Gilmore PR. Evidence for a subtype of insulin-like growth factor I receptor in brain. Regul Pept. (1986) 15:301–10. doi: 10.1016/0167-0115(86)90160-6
114. Kiepe D, Ulinski T, Powell DR, Durham SK, Mehls O, Tonshoff B. Differential effects of insulin-like growth factor binding proteins-1,−2,−3, and−6 on cultured growth plate chondrocytes. Kidney Int. (2002) 62:1591–600. doi: 10.1046/j.1523-1755.2002.00603.x
115. Gazit N, Vertkin I, Shapira I, Helm M, Slomowitz E, Sheiba M, et al. IGF-1 receptor differentially regulates spontaneous and evoked transmission via mitochondria at hippocampal synapses. Neuron. (2016) 89:583–97. doi: 10.1016/j.neuron.2015.12.034
116. Azar WJ, Zivkovic S, Werther GA, Russo VC. IGFBP-2 nuclear translocation is mediated by a functional NLS sequence and is essential for its pro-tumorigenic actions in cancer cells. Oncogene. (2014) 33:578–88. doi: 10.1038/onc.2012.630
117. Conover CA, Johnstone EW, Turner RT, Evans GL, John BFJ, Doran PM, et al. Subcutaneous administration of insulin-like growth factor (IGF)-II/IGF binding protein-2 complex stimulates bone formation and prevents loss of bone mineral density in a rat model of disuse osteoporosis. Growth Horm IGF Res. (2002) 12:178–83. doi: 10.1016/S1096-6374(02)00044-8
118. Fisher MC, Meyer C, Garber G, Dealy CN. Role of IGFBP2, IGF-I and IGF-II in regulating long bone growth. Bone. (2005) 37:741–50. doi: 10.1016/j.bone.2005.07.024
119. DeMambro VE, Maile L, Wai C, Kawai M, Cascella T, Rosen CJ, et al. Insulin-like growth factor-binding protein-2 is required for osteoclast differentiation. J Bone Miner Res. (2012) 27:390–400. doi: 10.1002/jbmr.545
120. Xi G, Wai C, DeMambro V, Rosen CJ, Clemmons DR. IGFBP-2 directly stimulates osteoblast differentiation. J Bone Miner Res. (2014) 29:2427–38. doi: 10.1002/jbmr.2282
121. Xi G, Solum MA, Wai C, Maile LA, Rosen CJ, Clemmons DR. The heparin-binding domains of IGFBP-2 mediate its inhibitory effect on preadipocyte differentiation and fat development in male mice. Endocrinology. (2013) 154:4146–57. doi: 10.1210/en.2013-1236
122. Allen NJ, Bennett ML, Foo LC, Wang GX, Chakraborty C, Smith SJ, et al. Astrocyte glypicans 4 and 6 promote formation of excitatory synapses via GluA1 AMPA receptors. Nature. (2012) 486:410–4. doi: 10.1038/nature11059
123. Fletcher L, Isgor E, Sprague S, Williams LH, Alajajian BB, Jimenez DF, et al. Spatial distribution of insulin-like growth factor binding protein-2 following hypoxic-ischemic injury. BMC Neurosci. (2013) 14:158. doi: 10.1186/1471-2202-14-158
124. Warren MS, Bradley WD, Gourley SL, Lin YC, Simpson MA, Reichardt LF, et al. Integrin beta1 signals through Arg to regulate postnatal dendritic arborization, synapse density, and behavior. J Neurosci. (2012) 32:2824–34. doi: 10.1523/JNEUROSCI.3942-11.2012
125. Newton SS, Collier EF, Hunsberger J, Adams D, Terwilliger R, Selvanayagam E, et al. Gene profile of electroconvulsive seizures: induction of neurotrophic and angiogenic factors. J Neurosci. (2003) 23:10841–51. doi: 10.1523/JNEUROSCI.23-34-10841.2003
126. Burgdorf J, Colechio EM, Ghoreishi HN, Gross AL, Rex CS, Zhang XL, et al. IGFBP2 produces rapid-acting and long-lasting effects in rat models of posttraumatic stress disorder via a novel mechanism associated with structural plasticity. Int J Neuropsychopharmacol. (2017) 20:476–84. doi: 10.1093/ijnp/pyx007
127. Suliman S, Mkabile SG, Fincham DS, Ahmed R, Stein DJ, Seedat S. Cumulative effect of multiple trauma on symptoms of posttraumatic stress disorder, anxiety, and depression in adolescents. Compr Psychiatry. (2009) 50:121–7. doi: 10.1016/j.comppsych.2008.06.006
128. Malberg JE, Platt B, Rizzo SJ, Ring RH, Lucki I, Schechter LE, et al. Increasing the levels of insulin-like growth factor-I by an IGF binding protein inhibitor produces anxiolytic and antidepressant-like effects. Neuropsychopharmacology. (2007) 32:2360–8. doi: 10.1038/sj.npp.1301358
129. Stein TD, Anders NJ, DeCarli C, Chan SL, Mattson MP, Johnson JA. Neutralization of transthyretin reverses the neuroprotective effects of secreted amyloid precursor protein (APP) in APPSW mice resulting in tau phosphorylation and loss of hippocampal neurons: support for the amyloid hyothesis. J Neurosci. (2004) 24:7707–17. doi: 10.1523/JNEUROSCI.2211-04.2004
130. Rowe WB, Blalock EM, Chen KC, Kadish I, Wang D, Barrett JE, et al. Hippocampal expression analyses reveal selective association of immediate-early, neuroenergetic, and myelinogenic pathways with cognitive impairment in aged rats. J Neurosci. (2007) 27:3098–110. doi: 10.1523/JNEUROSCI.4163-06.2007
131. Zhang JX, Chen XQ, Du JZ, Chen QM, Zhu CY. Neonatal exposure to intermittent hypoxia enhances mice performance in water maze and 8-arm radial maze tasks. J Neurobiol. (2005) 65:72–84. doi: 10.1002/neu.20174
132. Lu XJ, Chen XQ, Weng J, Zhang HY, Pak DT, Luo JH, et al. Hippocampal spine-associated Rap-specific GTPase-activating protein induces enhancement of learning and memory in postnatally hypoxia-exposed mice. Neuroscience. (2009) 162:404–14. doi: 10.1016/j.neuroscience.2009.05.011
133. Refojo D, Schweizer M, Kuehne C, Ehrenberg S, Thoeringer C, Vogl AM, et al. Glutamatergic and dopaminergic neurons mediate anxiogenic and anxiolytic effects of CRHR1. Science. (2011) 333:1903–7. doi: 10.1126/science.1202107
134. White V, Jawerbaum A, Mazzucco MB, Gauster M, Desoye G, Hiden U. Diabetes-associated changes in the fetal insulin/insulin-like growth factor system are organ specific in rats. Pediatr Res. (2015) 77:48–55. doi: 10.1038/pr.2014.139
135. Stranahan AM, Arumugam TV, Cutler RG, Lee K, Egan JM, Mattson MP. Diabetes impairs hippocampal function through glucocorticoid-mediated effects on new and mature neurons. Nat Neurosci. (2008) 11:309–17. doi: 10.1038/nn2055
136. Agrawal V, Tee MK, Qiao J, Muench MO, Miller WL. Potential role of increased oxygenation in altering perinatal adrenal steroidogenesis. Pediatr Res. (2015) 77:298–309. doi: 10.1038/pr.2014.194
137. Yakar S, Domene H, Meidan R, Cassorla F, Gilad I, Koch I, et al. Growth hormone (GH) stimulates insulin-like growth factor-I (IGF-I) and IGF-binding protein (IGFBP)-2 gene expression in spleens of juvenile rats. Horm Metab Res. (1994) 26:363–6. doi: 10.1055/s-2007-1001707
138. Cardona-Gomez GP, Chowen JA, Garcia-Segura LM. Estradiol and progesterone regulate the expression of insulin-like growth factor-I receptor and insulin-like growth factor binding protein-2 in the hypothalamus of adult female rats. J Neurobiol. (2000) 43:269–81. doi: 10.1002/(sici)1097-4695(20000605)43:3<269::aid-neu5>3.0.co;2-d
Keywords: IGFBP-2, IGF-IR, CNS, growth and repair, learning and memory, information processing
Citation: Khan S (2019) IGFBP-2 Signaling in the Brain: From Brain Development to Higher Order Brain Functions. Front. Endocrinol. 10:822. doi: 10.3389/fendo.2019.00822
Received: 10 July 2019; Accepted: 08 November 2019;
Published: 22 November 2019.
Edited by:
Yoshimi Nakagawa, University of Tsukuba, JapanReviewed by:
Claire Perks, University of Bristol, United KingdomRobert C. Baxter, University of Sydney, Australia
Copyright © 2019 Khan. This is an open-access article distributed under the terms of the Creative Commons Attribution License (CC BY). The use, distribution or reproduction in other forums is permitted, provided the original author(s) and the copyright owner(s) are credited and that the original publication in this journal is cited, in accordance with accepted academic practice. No use, distribution or reproduction is permitted which does not comply with these terms.
*Correspondence: Shumsuzzaman Khan, cDUzcDM1ZG5hJiN4MDAwNDA7eWFob28uY29t
†Present address: Shumsuzzaman Khan, Department of Physiology and Biophysics, School of Medicine, Case Western Reserve University, Cleveland, OH, United States