- 1Facultad de Ciencias de la Vida, Universidad Andrés Bello, Santiago, Chile
- 2Interdisciplinary Center for Aquaculture Research (INCAR), Universidad de Concepción, Concepción, Chile
- 3Department of Biology, Faculty of Marine and Environmental Sciences, Instituto Universitario de Investigación Marina (INMAR), Campus de Excelencia Internacional del Mar (CEI-MAR), University of Cádiz, Cádiz, Spain
- 4Department of Marine Biology and Aquaculture, Instituto de Ciencias Marinas de Andalucía (ICMAN-CSIC), Puerto Real, Spain
Teleost fish are exposed to diverse stressors in farming and wildlife conditions during their lifespan. Cortisol is the main glucocorticoid hormone involved in the regulation of their metabolic acclimation under physiological stressful conditions. In this context, increased plasma cortisol is associated with energy substrate mobilization from metabolic tissues, such as liver and skeletal muscle, to rapidly obtain energy and cope with stress. The metabolic actions of cortisol have primarily been attributed to its genomic/classic action mechanism involving the interaction with intracellular receptors, and regulation of stress-responsive genes. However, cortisol can also interact with membrane components to activate rapid signaling pathways. In this work, using the teleost fish gilthead sea bream (Sparus aurata) as a model, we evaluated the effects of membrane-initiated cortisol actions on the early modulation of glucose metabolism. For this purpose, S. aurata juveniles were intraperitoneally administrated with cortisol and with its membrane impermeable analog, cortisol-BSA. After 1 and 6 h of each treatment, plasma cortisol levels were measured, together with glucose, glycogen and lactate in plasma, liver and skeletal muscle. Transcript levels of corticosteroids receptors (gr1, gr2, and mr) and key gluconeogenesis (g6pc and pepck)- and glycolysis (pgam1 and aldo) related genes in the liver were also measured. Cortisol and cortisol-BSA administration increased plasma cortisol levels in S. aurata 1 h after administration. Plasma glucose levels enhanced 6 h after each treatment. Hepatic glycogen content decreased in the liver at 1 h of both cortisol and cortisol-BSA administration, while increased at 6 h due to cortisol but not in response to cortisol-BSA. Expression of gr1, g6pc, pgam1, and aldo were preferentially increased by cortisol-BSA in the liver. Taking all these results in consideration, we suggest that non-canonical cortisol mechanisms contribute to the regulation of the early glucose metabolism responses to stress in S. aurata.
Introduction
Fish are exposed to diverse stressful conditions in farming and wildlife during their lifespan (1, 2). Physiological acclimation to stress is essential to mobilize energy reserves (primarily glucose) and help the animal to overcome the threat (3). The physiological response to stress is initiated by the activation of the hypothalamic–pituitary–adrenal (HPA) and the hypothalamic-pituitary-interrenal (HPI) axis, secreting catecholamines and glucocorticoids, respectively (1). Both hormones are critical to coordinate multiples steps of the physiological and metabolic responses to maintain homeostasis (1, 4, 5).
Cortisol is the main glucocorticoid hormone involved in the regulation of the metabolic/physiological adaptation under stress conditions in teleost fish (4, 6). During the first phases of the stress response, increases of plasma cortisol levels produce the enhancement in glucose mobilization to vital organs mainly through the induction of hepatic gluconeogenesis as well as the inhibition of glycolysis and glucose uptake of peripheral organs (4, 7). In this context, it is well-known that cortisol is directly involved in the expression of key gluconeogenesis-related genes such as phosphoenolpyruvate carboxykinase (pepck) (8). Conversely, cortisol action on the transcriptional regulation of skeletal muscle glucose homeostasis is less understood and is mainly associated to the permissive-role for catecholamines induced glycogenolysis, as well as the modulation of glycolysis related genes such as pyruvate dehydrogenase kinase (pdk) (7). Accordingly, cortisol has an important role in the transcriptional modulation of glucose metabolism in fish (9).
The mechanism of cortisol action is associated with its genomic/classic signaling pathways involving the interaction with intracellular receptors and the modulation of stress responsive genes (9). Owing to the lipophilic nature of cortisol, this hormone is capable of crossing inside the cell and interact with its intracellular mineralocorticoid (MR) and glucocorticoid (GR) receptors (9, 10). The complex cortisol-receptor acts as a transcriptional factor interacting with glucocorticoid response elements (GRE) localized in stress-responsive genes. In this context, the identification of GRE located in gluconeogenesis and glycolysis related genes (e.g., pepck and g6pc) supports the idea of a direct regulation of the glucose metabolism by cortisol through transcriptional mechanisms (11, 12).
In addition to classical intracellular receptor binding, there is evidence that cortisol and other steroid hormones can also interact with membrane components activating rapid signaling pathways (13–15). This novel mechanism for cortisol is known as a non-genomic action and it has mainly been characterized using steroids analogs coupled to hydrophilic molecules such as bovine serum albumin (BSA) (13, 16). The complex glucocorticoid-BSA is an exclusive inductor of membrane-initiated effects and has been successfully used to discriminate the novel glucocorticoid actions in both in vitro and in vivo models (16–19). In fish models, the non-genomic cortisol actions have been associated with the suppression of phagocytosis in the freshwater teleost Channa punctatus (17) and with the increased plasma membrane fluidity and activation of protein kinase cAMP-dependent (PKA), protein kinase B (PKB), and protein kinase C (PKC) signaling pathways in rainbow trout hepatocytes (20, 21). In addition, this cortisol action also induces the extracellular signal-regulated kinase (ERK)—cAMP-responsive element binding protein (CREB) signaling pathways activation and the up regulation of peroxisome proliferator-activated receptor γ co-activator 1 α (pgc1a) expression in isolated rainbow trout myotubes (22). Even though, the mechanisms involved in the non-genomic cortisol pathways are complex and diverses, the impact in the adaptive response in fish are far from clear (23). In addition, until know whether non-canonical cortisol actions contribute to the regulation of glucose-metabolism gene expression is unknown.
Gilthead sea bream (Sparus aurata) is a well-studied marine fish used as a model in diverse studies related to physiological acclimation to stress (24, 25). Exogenous cortisol administration in S. aurata has revealed key aspects of this hormone in the transcriptional regulation of osmoregulatory processes, immune responses, and metabolic acclimation (26–28). In this line, even though it seems clear that cortisol increases circulatory glucose levels through the induction of hepatic gluconeogenesis-related genes, the impact of non-genomic cortisol actions over this process has not been explored yet.
In the present study, the rapid effects of membrane-initiated cortisol actions on the regulation of glucose and transcription of key glucose metabolism-related genes were studies in S. aurata. For this propose, we performed an in vivo assay in which S. aurata juveniles were injected with cortisol or with the membrane impermeable cortisol analog, cortisol-BSA. After 1 and 6 h of each treatment, plasma cortisol levels were measured, together with several metabolites in plasma, liver, and skeletal muscle. Hepatic transcript levels of corticosteroids receptors, as well as key gluconeogenesis and glycolysis related genes were also measured. The results were discussed in relation to the possible contribution of membrane-initiated cortisol actions on the regulation of glucose metabolism in fish liver and skeletal muscle.
Methodology
Emulation of Acute Stress in S. aurata Through the Exogenous Administration of Cortisol and Cortisol-BSA
Immature gilthead seabream (S. aurata) (16.1 ± 0.2 g body mass, mean ± SEM, n = 48) were obtained from Servicios Centrales de Investigación en Cultivos Marinos (SCI-CM, CASEM, University of Cadiz, Puerto Real, Cádiz, Spain; Spanish Operational Code REGA ES11028000312). Animals were randomly distributed in four 80-L tanks (~2.5 kg m−3 density) in a flow-through system, under natural photoperiod and constant temperature (18–19°C). Fish were fed daily with commercial pellets (1% of total animal weight). After 10 days of acclimation, fish of the first and second tanks were intraperitoneally administered with 0.01028 μM of cortisol (Sigma, St. Luis, MO) and 0.01028 μM of hydrocortisone-3-CMO-BSA (cortisol-BSA) (US biological, USA) dissolved in DMSO 1X, PBS 1X, and NP 40 0.05%, respectively. Preliminary experiments demonstrated that injection and manipulation of fish did not induce endogenous cortisol release and also this dose was appropriate to reach plasma levels of cortisol (~150 ng/mL) similar to those of an acute stress in S. aurata (29, 30) (Supplementary Figure 1). Fish of the third and fourth groups were administered with vehicle solution (DMSO, PBS 1X, NP 40 0.05%) and BSA (0.00892 mg/kg), respectively. The experiment was performed using duplicate tanks for each group. After 1 and 6 h of each treatment, all fish were euthanized by an overdose of 2-phenoxyethanol (1 mL/L). Blood was collected from caudal vessels with ammonium-heparinized syringes (Sigma-Aldrich H6279, 25000 units in 3 mL of saline 0.6 % NaCl).
Plasma was separated from cells by centrifugation of blood (3 min, 10,000 × g, 4°C), snap frozen in liquid nitrogen and stored at −80°C until analysis. The spinal cord of the fish was sectioned, and liver were collected, placed into tubes with 10-volumes (v/w) of RNAlater™ Soln (Invitrogen by Thermo Fisher Scientific), held for 24 h at 4°C and then stored at −20°C until total RNA isolation. In addition, liver and skeletal muscle were also excised, and the biopsies collected in microtubes were snap frozen in liquid nitrogen and stored at −80°C until the assay of metabolites content. The experiment complied with the EU directives for the protection of animals used for scientific purposes (2010/63/EU), the Spanish laws (law 32/2007 and RD 53/2013), and it was authorized by the Ethical Committee of the Universidad de Cádiz (Spain) for the use of laboratory animals and the Ethical Committee from the Andalusian Government (Junta de Andalucía reference number 28-04-15-241).
Plasma and Tissue Parameters
Plasma cortisol levels were measured by EIA kit (Arbor assays) as previously described by Estensoro et al. (31) in this fish species. Plasma glucose and lactate levels were quantified with the following Spinreact kits (Barcelona, Spain): HK Ref. 1001200 and Lactate Ref. 1001330, adapted to 96-well microplates. For the analysis of tissue metabolites, frozen skeletal muscle and liver were homogenized by ultrasonic disruption and analyzed according to the protocol of Vargas-Chacoff et al. (32). Glycogen levels were measured according to the method of Keppler and Decker (33), in which the glucose obtained via glycogen breakdown (after subtracting the free glucose levels) is determined using the previously described commercial glucose kit.
Enzymatic Activities Assays
Frozen liver and skeletal muscle portions were homogenized by ultrasonic disruption in 10 volumes of ice-cold homogenization buffer (50 mM imidazole, 1 mM 2-mercaptoethanol, 50 mM NaF, 4 mM EDTA, 0.5 mM phenylmethylsulfonyl fluoride (PMSF) and 250 mM sucrose; pH 7.5). The homogenate was centrifuged for 30 min at 3,220 × g and 4°C, and the supernatant stored at −80°C for further analysis. Enzyme activities of: Glycogen phosphorylase (GPt), Hexokinase (HK), Fructose 1,6-bisphosphatase (FBP), Lactate dehydrogenase (LDH), and Glucose 6-phosphate dehydrogenase (G6PDH) were determined as previously described for Sparus aurata (34) using a PowerWave™ 340 microplate spectrophotometer (Bio-Tek Instruments, Winooski, VT, USA). Reaction rates of enzymes were determined by changes in absorbance from the reduction of NAD(P)+ to NAD(P)H, measured at 340 nm and 37°C, during pre-established times (10–15 min). Activities were referenced against the protein content of homogenate (U mg prot−1).
RNA Extraction and cDNA Synthesis
Total RNA from S. aurata liver was extracted using the NucleoSpin RNA kit (Macherey–Nagel) according to manufacturer's instructions. An on-column RNase-free DNase digestion was used for gDNA elimination. RNA integrity and quantification were evaluated using 2100 Bioanalyzer and the RNA 6000 Nano Kit (Agilent Technologies, Santa Clara, CA, USA), and Qubit® 2.0 Fluorometer (Life Technology, Carlsbad, CA, USA), respectively. Only RNA with RIN >8 was used for cDNA synthesis. Retro transcription was carried out with the qScript™ cDNA Synthesis Kit (Quanta BioSciences) using 500 ng of liver RNA as input.
Real Time PCR
Real time PCR was performed by semi-quantitative fluorescence with a CFX Connect™ Real-Time PCR System (Bio-Rad Laboratories) in 96 white wells Hard-Shell® PCR plates covered with Microseal® “B” Seals (Bio-Rad). Briefly, each reaction mixture (in a volume of 10 μL) contained 0.5 μL of each specific forward and reverse primers (4 μM), 5 μL of PerfeCTa SYBR Green FastMixTM 2x (Quanta BioSciences) and 4 μL containing 10 ng of cDNA from the S. aurata liver.
Several calibration plots with different template concentrations in serial dilutions (from 10 ng to 100 fg) of input total RNA from liver had amplification efficiencies between 92.9 and 104.7%, for all primer pairs used. The PCR profile was as follows: 95°C, 10 min; [95°C, 15 s; 60°C, 30 s] ×40 cycles; and a melting curve [60–95°C, increasing 0.5°C every 5 s]. The melting curve was used to ensure that a single product was amplified and to verify the absence of primer-dimer artifacts. Results were normalized against beta actin (actb) and elongation factor 1a (ef1a) as a internal reference genes. Relative gene quantification was performed using the ΔΔCT method (35).
Candidate sequences corresponding to fructose-bisphosphate aldolase (aldo), glucose 6 phosphatase (g6pc), pepck, actb, and ef1α were available in NCBI database. Sequence of phosphoglycerate mutase 1 (pgam1) was obtained from available database belong to the S. aurata sequencing project (36). Primers were designed using Primer 3 available tool (http://frodo.wi.mit.edu/primer3/)and validated in NetPrimer (http://www.premierbiosoft.com/netprimer/) Oligo analyzer 3.1 (https://www.idtdna.com/calc/analyzer) available online tools. Finally, primers of glucocorticoid (gr1, gr2) and mineralocorticoid (mr) receptors were obtained from Tsalafouta et al. (37). All primers sequences used in this study are listed in Table 1.
Statistical Analysis
Normality and homogeneity of variances were analyzed using the Kolmogorov-Smirnov's and the Levene's test, respectively. All data were analyzed using a one-way ANOVA with treatment as the factors of variance, followed by a Tukey's honestly significant difference (HSD) as a post-hoc test, using the Graph Prism 7.0 software (GraphPad Software, Inc., San Diego, CA). A probability level of p < 0.05 was used as a threshold to indicate statistical significance.
Results
S. aurata individuals intraperitoneally injected with cortisol and cortisol-BSA reached plasma cortisol levels of 103.0 ± 0.9 and 107.2 ± 38.1 ng/mL, respectively. However, these values returned to basal levels after 6 h of treatment (Figure 1A). In addition, plasma glucose and lactate levels enhanced after 6 h of cortisol and cortisol-BSA treatments (Figures 1B,C).
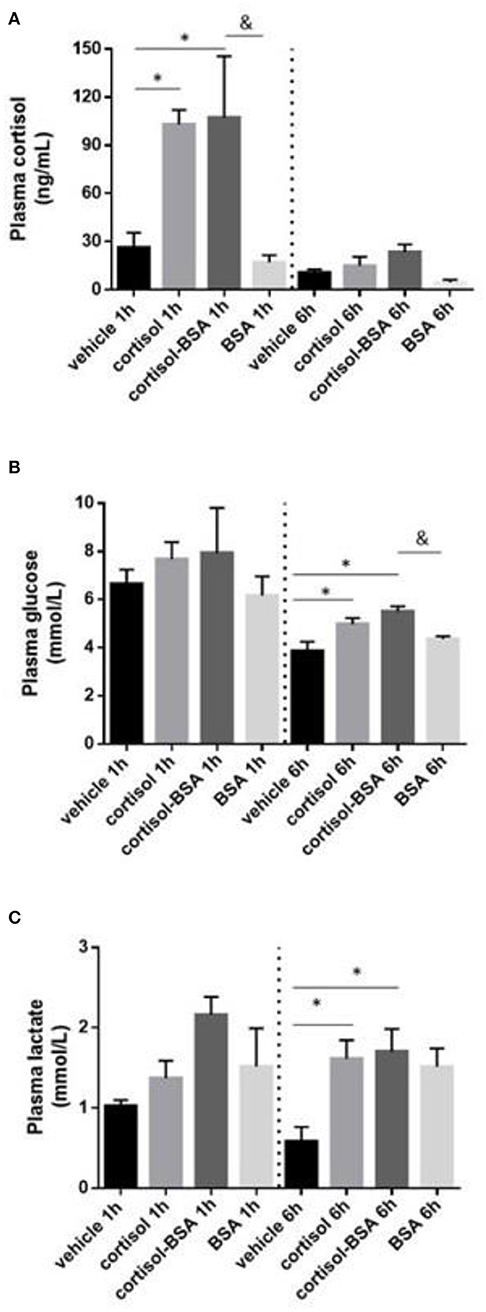
Figure 1. Plasma cortisol (A), glucose (B), and lactate (C) levels in vehicle (sham-control), cortisol, cortisol-BSA and BSA administrated fish at 1 and 6 h post-treatment. Results are expressed as mean ± SEM (n = 7). Asterisks and & represent significant differences (p < 0.05) against vehicle and BSA group at each sampling time, respectively.
Liver glycogen content decreased after 1 h of cortisol and cortisol-BSA treatment (Figure 2A). However, these values were recovered in cortisol but not in cortisol-BSA group after 6 h of treatment (Figure 2A). Hepatic glucose levels decreased in both cortisol-BSA and BSA treated fish after 1 h (Figure 2B). Finally, there were no differences in liver lactate levels at 1 and 6 h of cortisol and cortisol-BSA treatment (Figure 2C). Regarding mobilization of energetic substrates in the skeletal muscle, there was no variation in glycogen or glucose levels in this tissue after cortisol treatment (Figures 3A,B). However, skeletal muscle lactate was increased at 6 h in both cortisol and cortisol-BSA treated S. aurata (Figure 3C).
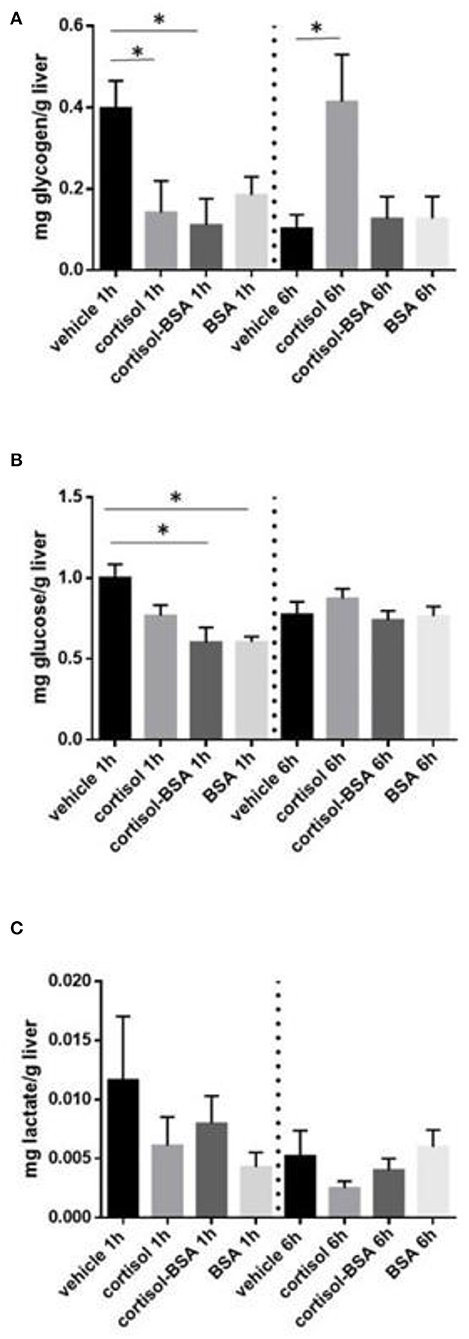
Figure 2. Hepatic glycogen (A), glucose (B), and lactate (C) levels in vehicle, cortisol, cortisol-BSA and BSA administrated fish at 1 and 6 h post-treatment. Results are expressed as means ± SEM (n = 7). Asterisks represent significant differences (p < 0.05) against vehicle group at each sampling time.
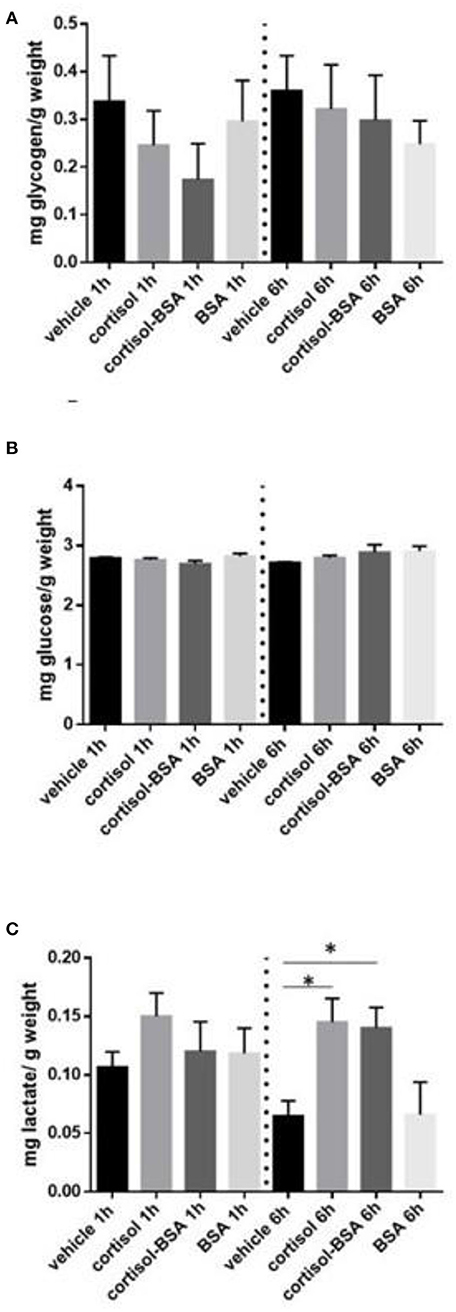
Figure 3. Skeletal muscle glycogen (A), glucose (B) and lactate (C) levels in vehicle, cortisol, cortisol-BSA and BSA administrated fish at 1 and 6 h post-treatment. Results are expressed as means ± SEM (n = 7). Asterisks represent significant differences (p < 0.05) against vehicle group at each sampling time.
To determine potentially effects of the membrane-initiated cortisol action on the regulation of glucose-metabolism related genes, we evaluated the activity of Glycogen phosphorylase (GPt), Hexokinase (HK), Fructose 1,6-bisphosphatase (FBP), Lactate dehydrogenase (LDH), and Glucose-6-phosphate dehydrogenase (G6PDH) in the liver and skeletal muscle. Overall, we observed that the activity of GPt, HK, and FBP does not change neither in the liver nor in skeletal muscle of injected S.aurata (Supplementary Figures 2A–C, 3A–C). Interestingly, we observed and increase in the activity of G6PDH in the liver of S.aurata treated with both cortisol or cortisol-BSA (Supplementary Figure 2E) and an increase of LDH activity in the skeletal muscle of S.aurata treated with cortisol-BSA (Supplementary Figure 3D).
To determine whether non-canonical cortisol effects contribute to modulate the expression of corticosteroids receptors and metabolism-related genes involved in the energetic substrate mobilization, we also evaluated hepatic mRNA levels of gr1, gr2, and mr, as well as key participants in gluconeogenesis (g6pc and pepck) and glycolysis (aldo and pgam1).
We observed a significant up regulation of gr1 under 1 hour of cortisol-BSA treatment (Figure 4A). On the other hand, there was not change in gr2 and mr expression in our experiment (Figures 4B,C).
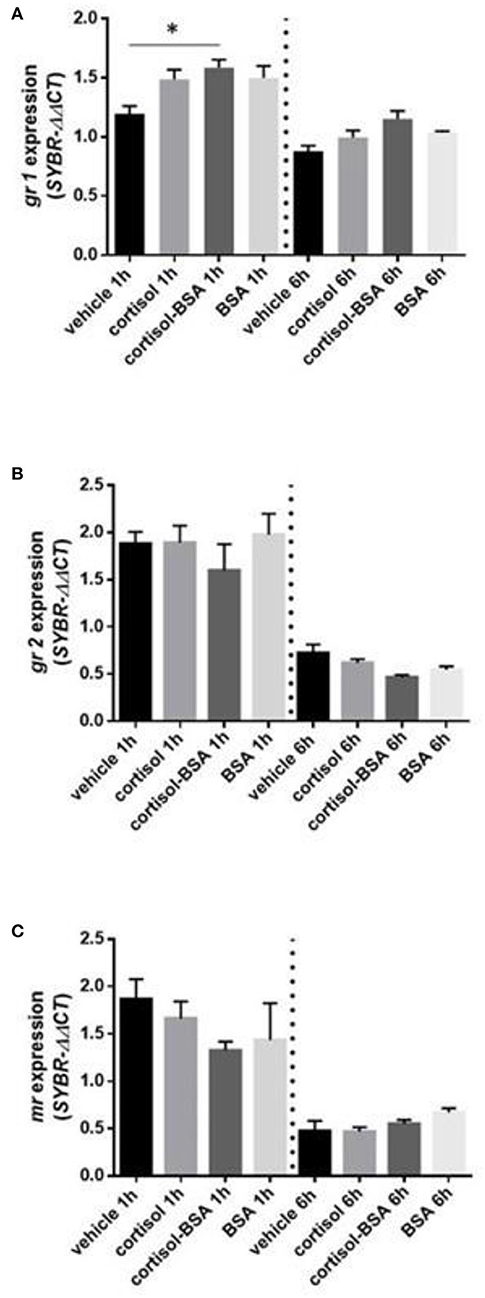
Figure 4. mRNA levels of corticosteroids receptor: glucocorticoid receptor 1 (gr1) (A), glucocorticoid receptor 2 (gr2) (B) and mineralocorticoid receptor (mr) (C). Expression values were normalized against elongation factor 1 alpha (ef1α) and beta actin (actb). Results are expressed as mean ± SEM (n = 6). Asterisks and & represent significant differences (p < 0.05) against vehicle and BSA group at each sampling time, respectively.
Both cortisol and cortisol-BSA increased mRNA levels of g6pc at 1 h after treatment. However, cortisol-BSA, but not cortisol, enhanced this expression after 6 h (Figure 5A). Conversely, pepck mRNA levels remained without variations throughout the entire trial (Figure 5B). Both glycolysis-related genes assessed (aldo and pgam1) were up regulated at 1 h in the liver under cortisol-BSA but not cortisol treatment (Figures 6A,B). This enhancement was observed also at 6 h but only for pgam1 expression (Figure 6B).
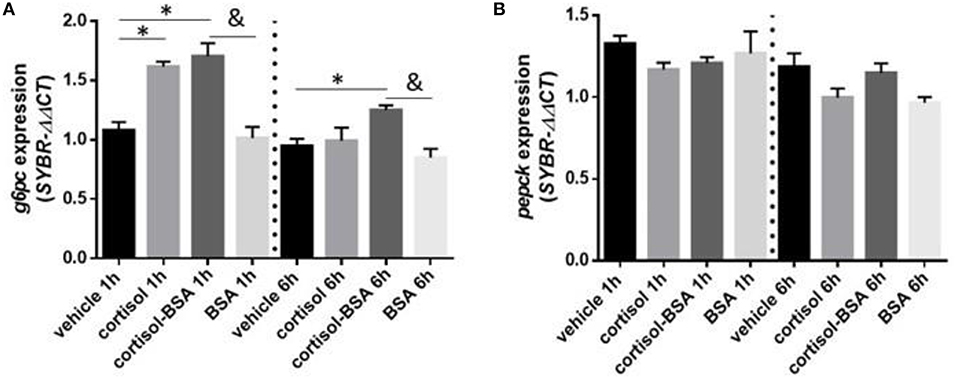
Figure 5. mRNA levels of hepatic gluconeogenesis enzymes glucose 6 phosphatase (g6pc) (A) and phosphoenolpyruvate carboxykinase (pepck) (B). Expression values were normalized against elongation factor 1 alpha (ef1α) and beta actin (actb). Results are expressed as mean ± SEM (n = 6). Asterisks and & represent significant differences (p < 0.05) against vehicle and BSA group at each sampling time, respectively.
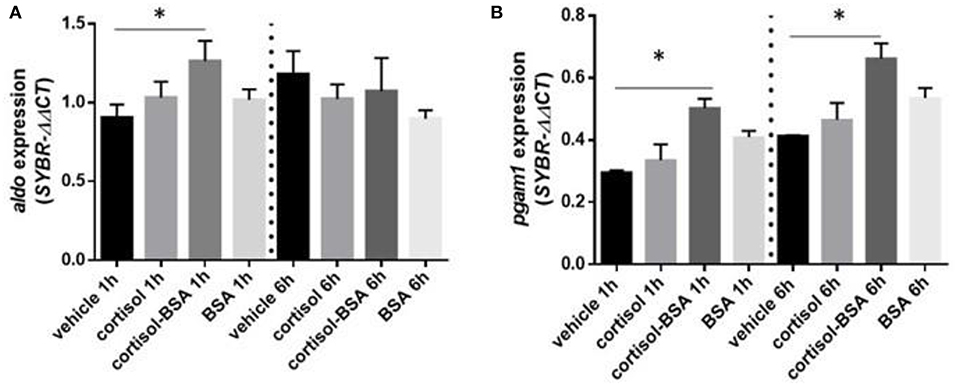
Figure 6. mRNA levels of hepatic glycolysis enzymes fructose-bisphosphate aldolase (aldo) (A) and phosphoglycerate mutase 1 (pgam1) (B). Expression values were normalized against elongation factor 1 alpha (ef1α) and beta actin (actb). Results are expressed as mean ± SEM (n = 6). Asterisks and & represent significant differences (p < 0.05) against vehicle and BSA group at each sampling time, respectively.
Discussion
The stress response in fish is attributed to the genomic/classic mechanisms of action of cortisol, involving the interaction with intracellular GR and the subsequent modulation of stress-responsive genes (9). In this work, using the membrane-impermeable cortisol analog cortisol-BSA, we were able to provide evidence for those membrane-initiated cortisol actions contributing to the mobilization of energy substrates and the transcriptional regulation of glucose metabolism in S. aurata. These results are in line with our recently study that revealed that exogenous cortisol and cortisol-BSA administration is capable to emulate an acute stress condition in rainbow trout promoting the up-regulation of pyruvate dehydrogenase kinase 2 (pdk2) expression in skeletal muscle (38).
Membrane-Initiated Cortisol Actions Modulate the Early Energy Substrates Mobilization in Liver and Skeletal Muscle of S. aurata
It is well-known that cortisol-mediated stress induces plasma glucose enhancement to provide energy for coping with stress (1, 4). In this work, we observed for the first time that stress-related doses of both cortisol and cortisol-BSA are able to increase plasma glucose levels in S. aurata 1 h after treatment. Because cortisol-BSA does not have the capacity to cross plasmatic membrane (38), our results suggest a potential role of membrane-initiated cortisol action in the glucose release to the bloodstream. In line with this result, recent studies using mammalian in vitro models have revealed that non-genomic cortisol pathways are involved in the rapid glucose mobilization, suggesting a possible role of this novel cortisol action in the cellular metabolic acclimation (39). In addition, this rapid non-genomic cortisol signaling has been associated with activity decrease of glucose related enzymes in fish, such glucose-6-phosphate dehydrogenase (G6PDH) and isocitrate dehydrogenase (ICDH) (40). Particularly, we observed and increase of G6PDH in the liver of Sparus aurata injected with cortisol or cortisol-BSA which support the idea that membrane-initiated cortisol action could be contributing in the glucose metabolism at this enzymatic level. However, the molecular mechanism underlying the glucose metabolism regulation owing to membrane-initiated cortisol action still is unclear. Interestingly, plasma glucose levels show a tendency to decrease in the vehicle group comparing 1 h with 6 h of treatment, this result could be related to a possible stress triggered by the handling of the animals and/or with the daily rhythmicity in the secretion of cortisol, which has previously been identified in S.aurata (41).
In addition to glucose, plasma lactate levels enhancement is another classic stress indicator that is also modulated by exogenous cortisol administration in fish (1, 28). We determined that plasma lactate levels also increased in S. aurata treated with cortisol and cortisol-BSA, suggesting a potential contribution to this non-canonical cortisol action in fish. However, until now, the non-canonical mechanisms of cortisol mediating increases of lactate are unknown.
A key metabolic response to stress is the hepatic metabolic capacity stimulation, promoting free glucose to coping with stress through hepatic gluconeogenesis as well as inhibition of glycolysis and glucose uptake in peripheral tissues (7, 42). Surprisingly, we observed that membrane-cortisol action is potentially involved in the mobilization of energy substrates in liver and skeletal muscle in S. aurata. Regarding the glucose regulation in the liver, we found that non-canonical cortisol effects induced glycogen breakdown (glycogenolysis) which is an essential process to provide free glucose to blood during the first phase of the stress response (26, 43). Even though glycogenolysis is mainly associated with the rapid secretion of catecholamines in teleost, including S. aurata (30, 44), in vitro mammalian models revealed that cortisol-related hormones (corticosterone) rapidly stimulates glycogenolysis by inducing the rapid phosphorylation of glycogen phosphorylase through a non-genomic mechanism (45). Therefore, we suggest that both classical intracellular membrane actions and rapid non-canonical glucocorticoid actions could be involved in the regulation of fish liver glycogenolysis. Moreover, it is well-known that cortisol is mainly associated to the replenishment of depleted glycogen stores after exposure to a stressor (4, 46). In this line, S. aurata individuals treated with cortisol, but not cortisol-BSA, increased hepatic glycogen content after 6 h, suggesting that glycogen content recovery is mainly mediated trough genomic cortisol actions. Once again, the variation observed in the hepatic glycogen levels between vehicle groups at 1 and 6 h support the idea of a possible handling stress in S.aurata caused during the in vivo protocol. Finally, we also observed a hepatic glucose content depletion in fish treated only with BSA, which could be attributed to the direct regulation of this compound (independent of the cortisol action) on the glucose metabolism in liver, similarly to the mammalian models (47). In this context, it has been previously reported that BSA alone could generates effects in different tissues potentially mediated by the osteonectin (SPARC) protein (48).
Regarding the role of non-genomic cortisol actions on energetic substrates mobilization in the skeletal muscle, in vitro studies revealed a direct role of glucocorticoids in the resynthesis of glycogen content in this tissue in rainbow trout (49). However, in the present study we did not observe changes in glycogen content either with cortisol or cortisol-BSA. It is not clear if this difference is due to species-specific differences or the outcome of in vivo versus in vitro approaches.
Membrane-Initiated Cortisol Action Modulates the mRNA Levels of Glucose Metabolism-Related Genes in the Liver of S. aurata
There are several studies revealing the impact of cortisol on the regulation of genes involved in metabolic adaptation to stress (9, 28, 50). However, whether non-canonical cortisol actions present a role in the transcriptional response to stress are unclear (23).
The increase of gr1 expression under 1 h of cortisol-BSA administration suggest that the rapid adjustments associated with short-term cortisol administration are modulated potentially by this receptor localizated in the surface. In line with this result, in other fish models has already propose the existence of a putative GR in the surface that mediated the rapid cortisol effects (38, 51). However, until now the presence a putative GR membrane receptor in Sparus aurata has not been identify yet. On the other hand, the variation of gr2 and mr mRNA levels observed only between both experimental time (independent of exogenous administration of cortisol) could be associated with the daily rhythmicity on the cortisol secretion in S.aurata (41).
One of the surprising results of this work was to identify that g6pc is a potential target gene for membrane-initiated cortisol actions in the liver of S. aurata. G6Pase is a rate-limiting gluconeogenesis enzyme that has been defined as a direct cortisol target in vertebrates due to the presence of GRE sites in their promotor region (52). In addition, in vitro studies have revealed that cortisol directly up regulates g6pc expression in hepatocytes of rainbow trout after short-term stimulus (12). Therefore, we suggest that gluconeogenesis is possibly mediated trough membrane-initiated cortisol action in fish, specifically by increasing hepatic g6pc mRNA levels. Moreover, we did not find an increase in pepck mRNA levels, which represents another key rate-limiting enzyme of gluconeogenesis (53). Although pepck has been previously characterized as a target for the GR signaling in other teleosts such as rainbow trout (54), stress-induced cortisol does not necessarily increase hepatic pepck mRNA levels in fish (43).
Finally, part of the glucose production in the liver during the stress response is used in this tissue through glycolysis (43). In this matter, several glycolysis-related genes are up regulated under early stress events in the liver of fish (9, 55). In our study, mRNA levels of glycolysis-related genes (pgam1 and aldo) of fish treated with cortisol-BSA enhanced at 1 and 6 h post-treatment, indicating a potential role of non-genomic cortisol actions in this process. These results suggest a major contribution of rapid non-genomic cortisol actions compared with the classical-initiated cortisol action, al least in the early regulation of glucose metabolism-related enzimes. However, this hypothesis needs to be tested and represents an interesting focus for futures studies.
Conclusion
Taking all these results in consideration, we suggest that non-canonical cortisol actions contribute to the regulation of the early glucose metabolism response to stress in S. aurata. The precise mechanisms of this regulation are still unclear, but they would involve transcriptional regulation of key gluconeogenesis- and glycolysis-related genes such as g6pc, pgam1, and aldo.
Data Availability Statement
The datasets generated for this study can be found in the Candidate sequences corresponding to fructosebisphosphate aldolase (aldo), glucose 6 phosphatase (g6pc), pepck, actb, and ef1α were available in NCBI database (https://www.ncbi.nlm.nih.gov/). Sequence of phosphoglycerate mutase 1 (pgam1) was obtained from available database belong to the S. aurata sequencing project (https://digital.csic.es/handle/10261/55459). Sequences obtained from NCBI: beta actin (actb), accession number: AY362763.1 elongation factor 1α (ef1α), accession number: AF184170.1 phosphoenolpyruvate carboxykinase (pepck), accession number: AF427868.1 fructose-bisphosphate aldolase (aldo), accession number: X82278.1 glucose 6 phosphatase (g6pc), accession number: AF427866.1 Sequence of phosphoglycerate mutase 1 (pgam1) obtained from S. aurata sequencing project: NCBI Sequence Read Archive SRA038178.1—http://hdl.handle.net/10261/55459.
Ethics Statement
The animal study was reviewed and approved by the EU directives for the protection of animals used for scientific purposes (2010/63/EU), the Spanish laws (law 32/2007 and RD 53/2013), and it was authorized by the Ethical Committee of the Universidad de Cádiz (Spain) for the use of laboratory animals and the Ethical Committee from the Andalusian Government (Junta de Andalucía reference number 28-04-15-241).
Author Contributions
JM, JV, and JA conceived and designed the study. JA and IR-J performed the experiments. JA, IR-J, and JM analyzed the data and performed statistical analyses. SB, AM, and GM-R contributed analysis, text, and comments to the paper. JA wrote the manuscript. All authors read and approved the final manuscript.
Funding
This work was funded by Spanish Ministry of Science, Innovation and Universities—MICINN (AGL2013-48835-C2-1-R and AGL2016-76069-C2-1-R) awarded to JM and by CONICYT/FONDAP/15110027 and FONDECYT 1171318 to JV. JA also acknowledges support received by CONICYT (Doctoral Scholarship No. 21150434). The authors (GM-R and JM) belong to the Fish Welfare and Stress Network (AGL2016-81808-REDT) and supported by the Agencia Estatal de Investigación (MICINN, Spanish Government).
Conflict of Interest
The authors declare that the research was conducted in the absence of any commercial or financial relationships that could be construed as a potential conflict of interest.
Acknowledgments
Authors would like to thank Servicios Centrales de Investigación en Cultivos Marinos (SCI-CM, CASEM, University of Cádiz, Puerto Real, Cádiz, Spain) for providing experimental fish. The experiments and analysis were carried out at the Campus de Excelencia Internacional del Mar (CEI·MAR) at two separate institutions (University of Cádiz and ICMAN-CSIC).
Supplementary Material
The Supplementary Material for this article can be found online at: https://www.frontiersin.org/articles/10.3389/fendo.2019.00779/full#supplementary-material
References
1. Wendelaar Bonga SE. The stress response in fish. Physiol Rev. (1997) 77:591–625. doi: 10.1152/physrev.1997.77.3.591
2. Sneddon LU, Wolfenden DC, Thomson JS. Stress management and welfare. In: Schreck CB, Tort L, Farrell AP, Brauner CJ, editors. Fish Physiology. Academic Press (2016). p. 463–539. doi: 10.1016/B978-0-12-802728-8.00012-6
3. Nardocci G, Navarro C, Cortés PP, Imarai M, Montoya M, Valenzuela B, et al. Neuroendocrine mechanisms for immune system regulation during stress in fish. Fish Shellfish Immunol. (2014) 40:531–8. doi: 10.1016/j.fsi.2014.08.001
4. Mommsen TP, Vijayan MM, Moon TW. Cortisol in teleosts: dynamics, mechanisms of action, and metabolic regulation. Rev Fish Biol Fisher. (1999) 9:211–68. doi: 10.1023/A:1008924418720
5. Schreck CB, Tort L. The concept of stress in fish. In: Schreck CB, Tort L, Farrell AP, Brauner CJ, editors. Fish Physiology. Academic Press (2016). p. 1–34. doi: 10.1016/B978-0-12-802728-8.00001-1
6. Ellis T, Yildiz HY, López-Olmeda J, Spedicato MT, Tort L, Øverli Ø, et al. Cortisol and finfish welfare. Fish Physiol Biochem. (2012) 38:163–88. doi: 10.1007/s10695-011-9568-y
7. Kuo T, Harris CA, Wang JC. Metabolic functions of glucocorticoid receptor in skeletal muscle. Mol Cell Endocrinol. (2013) 380:79–88. doi: 10.1016/j.mce.2013.03.003
8. Vijayan MM, Raptis S, Sathiyaa R. Cortisol treatment affects glucocorticoid receptor and glucocorticoid-responsive genes in the liver of rainbow trout. Gen Comp Endocrinol. (2003) 132:256–63. doi: 10.1016/S0016-6480(03)00092-3
9. Aluru N, Vijayan MM. Stress transcriptomics in fish: a role for genomic cortisol signaling. Gen Comp Endocrinol. (2009) 164:142–50. doi: 10.1016/j.ygcen.2009.03.020
10. Prunet P, Sturm A, Milla S. Multiple corticosteroid receptors in fish: from old ideas to new concepts. Gen Comp Endocrinol. (2006) 147:17–23. doi: 10.1016/j.ygcen.2006.01.015
11. Vander Kooi BT, Onuma H, Oeser JK, Svitek CA, Allen SR, Vander Kooi CW. The glucose-6-phosphatase catalytic subunit gene promoter contains both positive and negative glucocorticoid response elements. Mol Endocrinol. (2005) 19:3001–22. doi: 10.1210/me.2004-0497
12. Aluru N, Vijayan MM. Hepatic transcriptome response to glucocorticoid receptor activation in rainbow trout. Physiol Genom. (2007) 31:483–91. doi: 10.1152/physiolgenomics.00118.2007
13. Borski RJ. Nongenomic membrane actions of glucocorticoids in vertebrates. Trends Endocrinol Metab. (2000) 11:427–36. doi: 10.1016/S1043-2760(00)00325-8
14. Lösel R, Wehling M. Non-genomic actions of steroid hormones. Nat Rev Mol Cell Biol. (2003) 4:46. doi: 10.1038/nrm1009
15. Levin ER, Hammes SR. Nuclear receptors outside the nucleus: extranuclear signalling by steroid receptors. Nat Rev Mol Cell Biol. (2016) 17:783. doi: 10.1038/nrm.2016.122
16. Jozic I, Vukelic S, Stojadinovic O, Liang L, Ramirez HA, Pastar I, et al. Stress signals, mediated by membranous glucocorticoid receptor, activate PLC/PKC/GSK-3β/β-catenin pathway to inhibit wound closure. J Invest Dermatol. (2017) 137:1144–54. doi: 10.1016/j.jid.2016.11.036
17. Roy B, Rai U. Genomic and non-genomic effect of cortisol on phagocytosis in freshwater teleost Channa punctatus: an in vitro study. Steroids. (2009) 74:449–55. doi: 10.1016/j.steroids.2008.12.013
18. Nahar J, Haam J, Chen C, Jiang Z, Glatzer NR, Muglia LJ, et al. Rapid non-genomic glucocorticoid actions in male mouse hypothalamic neuroendocrine cells are dependent on the nuclear glucocorticoid receptor. Endocrinology. (2015) 156:2831–42. doi: 10.1210/en.2015-1273
19. Rainville JR, Weiss GL, Evanson N, Herman JP, Vasudevan N, Tasker JG. Membrane-initiated nuclear trafficking of the glucocorticoid receptor in hypothalamic neurons. Steroids. (2017) 142:55–64. doi: 10.1016/j.steroids.2017.12.005
20. Dindia L, Murray J, Faught E, Davis TL, Leonenko Z, Vijayan MM. Novel non-genomic signaling by glucocorticoid may involve changes to liver membrane order in rainbow trout. PLoS ONE. (2012) 7:e46859. doi: 10.1371/journal.pone.0046859
21. Dindia L, Faught E, Leonenko Z, Thomas R, Vijayan MM. Rapid cortisol signaling in response to acute stress involves changes in plasma membrane order in rainbow trout liver. Am J Physiol Endocrinol Metab. (2013) 304:E1157–66. doi: 10.1152/ajpendo.00500.2012
22. Espinoza MB, Aedo JE, Zuloaga R, Valenzuela C, Molina A, Valdés JA. Cortisol induces reactive oxygen species through a membrane glucocorticoid receptor in rainbow trout myotubes. J Cell Biochem. (2017) 118:718–25. doi: 10.1002/jcb.25676
23. Das C, Thraya M, Vijayan MM. Non-genomic cortisol signaling in fish. Gen Comp Endocrinol. (2018) 265:121–7. doi: 10.1016/j.ygcen.2018.04.019
24. Martos-Sitcha JA, Wunderink YS, Straatjes J, Skrzynska AK, Mancera JM, Martínez-Rodríguez G. Different stressors induce differential responses of the CRH-stress system in the gilthead sea bream (Sparus aurata). Comp Biochem Physiol A Mol Integr Physiol. (2014) 177:49–61. doi: 10.1016/j.cbpa.2014.07.021
25. Ruiz-Jarabo I, Klaren PH, Louro B, Martos-Sitcha JA, Pinto PIS, Vargas-Chacoff L, et al. Characterization of the peripheral thyroid system of gilthead seabream acclimated to different ambient salinities. Comp Biochem Physiol A Mol Integr Physiol. (2017) 203:24–31. doi: 10.1016/j.cbpa.2016.08.013
26. Laiz-carrión R, Martín Del Río MP, Miguez JM, Mancera JM, Soengas JL. Influence of cortisol on osmoregulation and energy metabolism in gilthead seabream Sparus aurata. J Exp Zool A Comp Exp Biol. (2003) 298:105–18. doi: 10.1002/jez.a.10256
27. Castillo J, Teles M, Mackenzie S, Tort L. Stress-related hormones modulate cytokine expression in the head kidney of gilthead seabream (Sparus aurata). Fish Shellfish Immunol. (2009) 27:493–9. doi: 10.1016/j.fsi.2009.06.021
28. Teles M, Boltaña S, Reyes-López F, Santos MA, Mackenzie S, Tort L. Effects of chronic cortisol administration on global expression of GR and the liver transcriptome in Sparus aurata. Mar Biotechnol. (2013) 15:104–14. doi: 10.1007/s10126-012-9467-y
29. Arends RJ, Mancera JM, Munoz JL, Bonga SW, Flik G. The stress response of the gilthead sea bream (Sparus aurata L.) to air exposure and confinement. J Endocrinol. (1999) 163:149–57. doi: 10.1677/joe.0.1630149
30. Skrzynska AK, Maiorano E, Bastaroli M, Naderi F, Míguez JM, Martínez-Rodríguez G, et al. Impact of air exposure on vasotocinergic and isotocinergic systems in gilthead sea bream (Sparus aurata): new insights on fish stress response. Front Physiol. (2018) 9:96. doi: 10.3389/fphys.2018.00096
31. Estensoro I, Ballester-Lozano G, Benedito-Palos L, Grammes F, Martos-Sitcha JA, Mydland LT, et al. Dietary butyrate helps to restore the intestinal status of a marine teleost (Sparus aurata) fed extreme diets low in fish meal and fish oil. PLoS ONE. (2016) 11:e0166564. doi: 10.1371/journal.pone.0166564
32. Vargas-Chacoff L, Ruiz-Jarabo I, Arjona FJ, Laiz-Carrión R, Flik G, Klaren PH, et al. Energy metabolism of hyperthyroid gilthead sea bream Sparus aurata L. Comp Biochem Physiol A Mol Integr Physiol. (2016) 191:25–34. doi: 10.1016/j.cbpa.2015.09.014
33. Keppler D, Decker K. “Glycogen determination with amyloglucosidase”. In: Bergmeyer HU, editor. Methods of Enzymatic Analysis. New York, NY, Academic Press (1974). p. 127–1131.
34. Jerez-Cepa I, Fernández-Castro M, Del Santo O'Neill TJ, Martos-Sitcha JA, Martínez-Rodríguez G, Mancera JM, et al. Transport and recovery of gilthead seabream (Sparus aurata L.) sedated with clove oil and MS-222: effects on stress axis regulation and intermediary metabolism. Front Physiol. (2019) 10:526. doi: 10.3389/fphys.2019.00612
35. Livak KJ, Schmittgen TD. Analysis of relative gene expression data using real-time quantitative PCR and the 2– ΔΔCT method. Methods. (2001) 25:402–8. doi: 10.1006/meth.2001.1262
36. Yúfera M, Halm S, Beltran S, Fusté B, Planas JV, Martínez-Rodríguez G. Transcriptomic characterization of the larval stage in gilthead seabream (Sparus aurata) by 454 pyrosequencing. Mar Biotechnol. (2012) 14:423–35. doi: 10.1007/s10126-011-9422-3
37. Tsalafouta A, Sarropoulou E, Papandroulakis N, Pavlidis M. Characterization and expression dynamics of key genes involved in the gilthead sea bream (Sparus aurata) cortisol stress response during early ontogeny. Mar Biotechnol. (2018) 20:611–22. doi: 10.1007/s10126-018-9833-5
38. Aedo JE, Zuloaga R, Boltaña S, Molina A, Valdés JA. Membrane-initiated cortisol action modulates early pyruvate dehydrogenase kinase 2 (pdk2) expression in fish skeletal muscle. Comp Biochem Physiol A Mol Integr Physiol. (2019) 233:24–9. doi: 10.1016/j.cbpa.2019.03.022
39. Gong H, Liu L, Ni CX, Zhang Y, Su WJ, Lian YJ, et al. Dexamethasone rapidly inhibits glucose uptake via non-genomic mechanisms in contracting myotubes. Arch Biochem Biophys. (2016) 603:102–9. doi: 10.1016/j.abb.2016.05.020
40. Sunny F, Lakshmy PS, Oommen OV. Rapid action of cortisol and testosterone on lipogenic enzymes in a fresh water fish Oreochromis mossambicus: short-term in vivo and in vitro study. Comp Biochem Physiol B Biochem Mol Biol. (2002) 131:297–304. doi: 10.1016/S1096-4959(02)00023-4
41. Vera LM, Montoya A, Pujante IM, Pérez-Sánchez J, Calduch-Giner JA, Mancera JM, et al. Acute stress response in gilthead sea bream (Sparus aurata L.) is time-of-day dependent: physiological and oxidative stress indicators. Chronobiol Int. (2014) 31:1051–61. doi: 10.3109/07420528.2014.945646
42. Wiseman S, Vijayan MM. Aroclor 1254 disrupts liver glycogen metabolism and enhances acute stressor-mediated glycogenolysis in rainbow trout. Comp Biochem Physiol C Toxicol Pharmacol. (2011) 154:254–60. doi: 10.1016/j.cbpc.2011.06.013
43. López-Patiño MA, Hernández-Pérez J, Gesto M, Librán-Pérez M, Míguez JM, Soengas JL. Short-term time course of liver metabolic response to acute handling stress in rainbow trout, Oncorhynchus mykiss. Comp Biochem Physiol A Mol Integr Physiol. (2014) 168:40–9. doi: 10.1016/j.cbpa.2013.10.027
44. Fabbri E, Moon TW. Adrenergic signaling in teleost fish liver, a challenging path. Comp Biochem Physiol B Biochem Mol Biol. (2016) 199:74–86. doi: 10.1016/j.cbpb.2015.10.002
45. Gomez-Munoz A, Hales P, Brindley DN, Sancho MJ. Rapid activation of glycogen phosphorylase by steroid hormones in cultured rat hepatocytes. Biochem J. (1989) 262:417–23. doi: 10.1042/bj2620417
46. Leung LY, Woo NY. Effects of growth hormone, insulin-like growth factor I, triiodothyronine, thyroxine, and cortisol on gene expression of carbohydrate metabolic enzymes in sea bream hepatocytes. Comp Biochem Physiol A Mol Integr Physiol. (2010) 157:272–82. doi: 10.1016/j.cbpa.2010.07.010
47. Buxton DB, Halvorsen O, Zhou W, Olson MS. Glycogenolytic and haemodynamic responses to bovine serum albumin in isolated perfused livers from sensitized rats. Biochem J. (1993) 292:253–9. doi: 10.1042/bj2920253
48. Merlot AM, Kalinowski DS, Richardson DR. Unraveling the mysteries of serum albumin—more than just a serum protein. Front Physiol. (2014) 5:299. doi: 10.3389/fphys.2014.00299
49. Milligan CL. A regulatory role for cortisol in muscle glycogen metabolism in rainbow trout Oncorhynchus mykiss Walbaum. J Exp Biol. (2003) 206:3167–73. doi: 10.1242/jeb.00538
50. Madison BN, Tavakoli S, Kramer S, Bernier NJ. Chronic cortisol and the regulation of food intake and the endocrine growth axis in rainbow trout. J Endocrinol. (2015) 226:103–19. doi: 10.1530/JOE-15-0186
51. Johnstone WM III, Mills KA, Alyea RA, Thomas P, Borski RJ. Characterization of membrane receptor binding activity for cortisol in the liver and kidney of the euryhaline teleost, Mozambique tilapia (Oreochromis mossambicus). Gen Comp Endocrinol. (2013) 192:107–14. doi: 10.1016/j.ygcen.2013.06.025
52. Yabaluri N, Bashyam MD. Hormonal regulation of gluconeogenic gene transcription in the liver. J Biosci. (2010) 35:473–84. doi: 10.1007/s12038-010-0052-0
53. Radziuk J, Pye S. Hepatic glucose uptake, gluconeogenesis and the regulation of glycogen synthesis. Diabetes/Metab Res Rev. (2001) 17:250–72. doi: 10.1002/dmrr.217
54. Faught E, Vijayan MM. Mechanisms of cortisol action in fish hepatocytes. Comp Biochem Physiol B Biochem Mol Biol. (2016) 199:136–45. doi: 10.1016/j.cbpb.2016.06.012
Keywords: cortisol, gene expression, glucose metabolism, membrane-initiated cortisol action, Sparus aurata, stress response
Citation: Aedo JE, Ruiz-Jarabo I, Martínez-Rodríguez G, Boltaña S, Molina A, Valdés JA and Mancera JM (2019) Contribution of Non-canonical Cortisol Actions in the Early Modulation of Glucose Metabolism of Gilthead Sea Bream (Sparus aurata). Front. Endocrinol. 10:779. doi: 10.3389/fendo.2019.00779
Received: 29 July 2019; Accepted: 24 October 2019;
Published: 12 November 2019.
Edited by:
Russell J. Borski, North Carolina State University, United StatesReviewed by:
Peggy Biga, University of Alabama at Birmingham, United StatesTakashi Yazawa, Asahikawa Medical University, Japan
Copyright © 2019 Aedo, Ruiz-Jarabo, Martínez-Rodríguez, Boltaña, Molina, Valdés and Mancera. This is an open-access article distributed under the terms of the Creative Commons Attribution License (CC BY). The use, distribution or reproduction in other forums is permitted, provided the original author(s) and the copyright owner(s) are credited and that the original publication in this journal is cited, in accordance with accepted academic practice. No use, distribution or reproduction is permitted which does not comply with these terms.
*Correspondence: Juan A. Valdés, anZhbGRlcyYjeDAwMDQwO3VuYWIuY2w=