- 1Montreal Heart Institute, Montreal, QC, Canada
- 2l'institut du thorax, INSERM, CNRS, UNIV Nantes, Nantes, France
- 3Faculty of Health Sciences, University of Ottawa, Ottawa, ON, Canada
O-GlcNAcylation is a ubiquitous and reversible post-translational protein modification that has recently gained renewed interest due to the rapid development of analytical tools and new molecules designed to specifically increase the level of protein O-GlcNAcylation. The level of O-GlcNAc modification appears to have either deleterious or beneficial effects, depending on the context (exposure time, pathophysiological context). While high O-GlcNAcylation levels are mostly reported in chronic diseases, the increase in O-GlcNAc level in acute stresses such as during ischemia reperfusion or hemorrhagic shock is reported to be beneficial in vitro, ex vivo, or in vivo. In this context, an increase in O-GlcNAc levels could be a potential new cardioprotective therapy, but the ambivalent effects of protein O-GlcNAcylation augmentation remains as a key problem to be solved prior to their transfer to the clinic. The emergence of new analytical tools has opened new avenues to decipher the mechanisms underlying the beneficial effects associated with an O-GlcNAc level increase. A better understanding of the exact roles of O-GlcNAc on protein function, targeting or stability will help to develop more targeted approaches. The aim of this review is to discuss the mechanisms and potential beneficial impact of O-GlcNAc modulation, and its potential as a new clinical target in cardiology.
Introduction
Definition, Pathway, and Regulation
The O-N-acetyl glucosaminylation, commonly known as O-GlcNAcylation, is a reversible post-translational modification (PTM) that involves the addition of the monosaccharide β-D-N-acetylglucosamine to serine and threonine residues of proteins. It was first described by Torres and Hart on the internal surface of plasma membranes of lymphocytes (1). More recently, it has also been identified on cytosolic, nuclear, mitochondrial and membrane proteins (2–4). Over 3,000 proteins have been identified so far to be O-GlcNAcylated (5), and this number will probably increase with the development of new analytical techniques of O-GlcNAcylation detection such as the copper-catalyzed azide-alkyne cycloaddition “click” reaction described recently (6). From 1984 to 2004, <200 publications mentioning the MeSH terms “O-GlcNAc” or “O-GlcNAcylation” are referenced in Pubmed, while in 2017 alone almost 200 references can be found. Initially, little attention was paid to this minor sugar moiety, probably because the tools available to study this PTM were limited. In fact, detecting it is particularly difficult as it cannot be studied with classic techniques such as electrophoresis or high pressure liquid chromatography (HPLC) as the O-GlcNAc moiety has no impact on molecular weight or isoelectric point, and it is very labile (7). More recently, with the development of more specific pharmacological compounds, protein O-GlcNAcylation has regained attention. Whilst it is now evident that protein O-GlcNAcylation is involved in many pathologies (from cancer to neurological disorders and cardiac function), the overall impact of protein O-GlcNAcylation remains unclear as in some situations it is reported to be beneficial (e.g., ischemia/reperfusion) or deleterious (e.g., diabetes). This suggests a potential role of this PTM in adapting to stress response and its importance in pathophysiological situations.
Protein O-GlcNAcylation is regulated by the concerted actions of only three enzymes. When glucose enters a cell it can be metabolized in a number of different metabolic pathways including glycogen synthesis, the pentose phosphate pathway, the glycolysis, or the hexosamine biosynthetic pathway (HBP, Figure 1). The first enzyme, glutamine fructose-6P amidotransferase (GFAT) uses glutamine and 2 to 5% of glycolytic fructose-6-P to perform the first step of the HBP. As the rate-limiting enzyme, GFAT controls HBP flow and consequently the O-GlcNAcylation level (8, 9). Mammals express two GFAT isoforms, GFAT1 and GFAT2, which are coded by separate genes. Both isoforms are expressed in heart. GFAT1 is ubiquitous, and mainly expressed in placenta, pancreas, testis and skeletal muscle. GFAT2 shares 75% homology with GFAT1, and this isoform is mostly expressed in heart and the central nervous system. Once the UDP-GlcNAc group is formed, it can be added or removed from proteins by two enzymes: the O-GlcNAc transferase (OGT) and the β-N-acetylglucosaminidase (OGA), respectively (Figure 1). As for GFAT, different splice variants of OGT and OGA exist. Alternative splicing of ogt results in the generation of three isoforms, a nucleocytoplasmic (116 kDa-ncOGT), a smaller isoform (70 kDa) named short form (sOGT) and a mitochondrial (103 kDa-mOGT) isoform of OGT. It is unclear if this last isoform is active. The first two isoforms are expressed mainly in the cytoplasm and nucleus as heterotrimers consisting of 2 ncOGT and 1 sOGT subunits (8). Two isoforms of OGA have been formerly described, a long one (lOGA) of 102 kDa found in the nucleocytoplasm, and a shorter one (sOGA) of 76 kDa resulting from alternative splicing. The smaller isoform is found in the sarcoplasmic reticulum and lipid droplets and is less active (4). The existence of a functional mitochondrial OGA isoform is debatable and represents an important area of ongoing research.
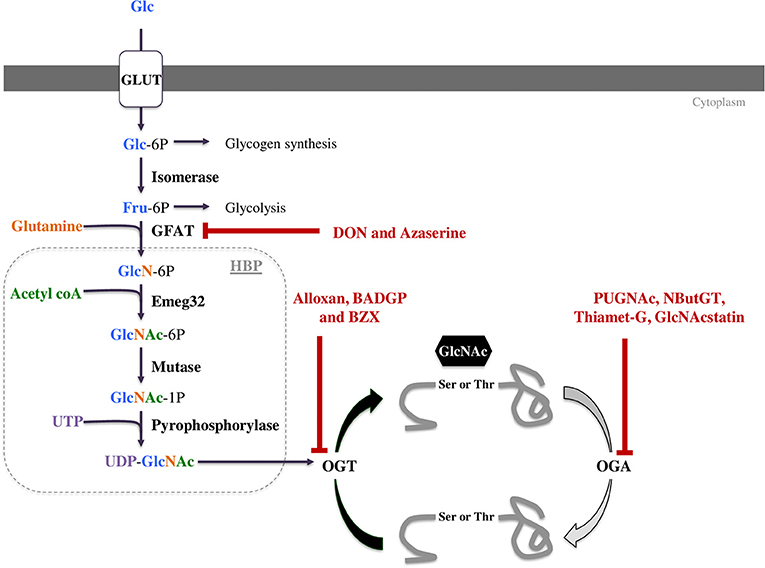
Figure 1. The hexosamine biosynthetic pathway (HBP) leads to UDP-GlcNAc formation and regulates O-GlcNAcylation. This pathway is regulated by only three enzymes: GFAT (glutamine fructose-6P aminotransferase), OGT (O-GlcNAc transferase) and OGA (O-GlcNAcase). These three enzymes can be targeted by pharmacological compounds to modulate O-GlcNAc levels. Some of them decrease O-GlcNAc levels such as DON and azaserine inhibiting GFAT or Alloxan, BZX and BADGP inhibiting OGT. OGA inhibitors like PUGNAc, NButGT, Thiamet-G and GlcNAcstatin increase protein O-GlcNAcylation.
Pharmacological Regulation
The development of pharmacological tools to modulate protein O-GlcNAcylation has been very challenging. As discussed below, potent OGA inhibitors are now available for clinical use, but OGT inhibitors still need further development to be used properly in vivo. Altogether, these new molecules have allowed extensive characterization of this PTM.
Decrease in O-GlcNAc Levels
GFAT inhibitors
In the 1950's, when O-GlcNAc moiety was not yet discovered, O-diazoacetyl-L-serine (azaserine) and 6-diazO-5-oxO-L-norleucine (DON) were developed to reduce tumor growth (10, 11). These molecules are structural analogs of glutamine and act as competing agonists or antagonists, respectively. They react with the catalytic region of most amidO-transferases, among them GFAT. They have a very low selectivity for GFAT. Whilst these compounds were used to efficiently induce antineoplastic effects, they presented a number of side effects including nausea and vomiting or loss of enthusiasm (12), and DON also causes hepatotoxicity in children (13). Moreover, through amidO-transferase inhibition, these compounds had pleiotropic effects in cells (Table 1). Developing new GFAT inhibitors represents a challenge because UDP-GlcNAc is also used for other cellular process, and its inhibition could have many side effects. As a result more attention has been paid to the development of OGT and OGA inhibitors.
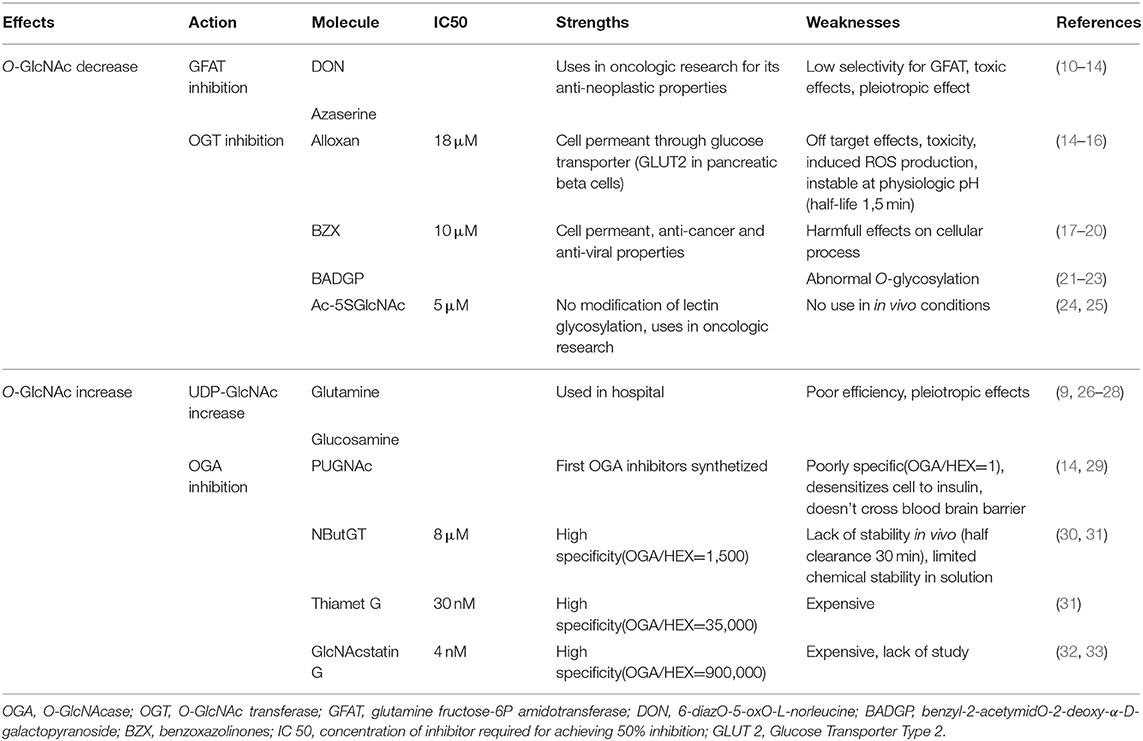
Table 1. Summary of molecules currently available for O-GlcNAc level modulation: their actions, specificity, known limits and strengths.
OGT inhibitors
Different types of OGT inhibitors exist (Figure 1, Table 1). In this review, we will only focus on the four most described and used molecules. (1) Alloxan is a uracil analog developed at the beginning of the Twentieth Century (34). It was shown to be an OGT inhibitor on isolated pancreatic islets in vitro (35), and is absorbed via the glucose transporter GLUT2 in pancreatic β-cells. However, alloxan is highly unstable at physiological pH (half-life 1.5 min), it drives cell toxicity due to ROS production, and is not OGT specific as it also inhibits OGA (14–16). (2) At the end of the 1960's, benzoxazolinones (4-methoxyphenyl 6-acetyl-2-oxO-2,3-dihydrO-1,3-benzoxazole-3-carboxylate, or BZX) and their derived-compounds were proposed as potential new therapeutics due to their anti-cancer and anti-viral properties (17–19). However, as BZX irreversibly inactivates OGT, it cannot be used clinically due to its potential harmful effects on other cellular process (20). (3) BADGP (benzyl-2-acetymidO-2-deoxy-α-D-galactopyranoside), a N-acetylgalactosamine derivative, was also used as an OGT inhibitor (36, 37). Permanent exposure to BADGP induced abnormal O-glycosylation of mucins in HT-29 cells with potential negative effects on host defenses against pathogens (21–23). (4) Finally, a last inhibitor: Ac-5SGlcNAc, was proposed in 2011. This compound produced 5S-UDP-GlcNAc that binds to OGT and inhibits its activity. Authors demonstrated dose and time-dependent effects of this inhibitor (from 0.1 to 1,000 μM) and an EC50 at 5 μM in COS7 cells. These results were confirmed in fibroblasts, hepatocytes and neuronal cells, and by others in different cell types especially in an oncologic context. Contrary to the three first OGT inhibitors, Ac-5SGlcNAc does not perturb lectin glycosylation even at the highest dose. Thus, this compound is more frequently used in basic research, however, there is, today, no evaluation of its in vivo efficiency and safety (24).
In summary, whilst OGT inhibitors currently lack specificity and selectivity, improvements in OGT inhibitors is necessary to be able to reduce protein O-GlcNAc levels and represents a potentially huge opportunity to reduce cancer or diabetes burden.
Increase in O-GlcNAc Levels
The development of strategies to increase O-GlcNAc levels has been more successful and can be used in human. Two different approaches are available to increase protein O-GlcNAc levels: increase in total UDP-GlcNAc through an increase in HBP flux or pharmacological inhibition of OGA.
Increase in UDP-GlcNAc concentration
Glucosamine (GlcN) bypass the rate limiting enzyme of the HBP, GFAT (38) resulting in higher UDP-GlcNAc levels, consequently it increases protein O-GlcNAcylation. Yet, if GlcN increases protein O-GlcNAc levels by 2 or 3-fold, this compound also has side effects such as: decrease in ATP production or increase in proteoglycans production (39, 40).
OGA inhibitors
Another way to increase protein O-GlcNAcylation is to inhibit OGA, and different molecules have been developed over the last 20 years. (1) The first described in the literature is the O-(2-acetamidO-2-deoxy-D-glucopyranosyliden)-aminO-N-phenylcarbamate commonly known as PUGNAc. It has been the most widely used compound to inhibit OGA for about a decade. However, PUGNAc also reacts with other hexosaminidases (HEX) such as lysosomal β-hexosaminidases with an inhibition ratio OGA/HEX of 1 (29). Recent OGA crystallography studies lead to the development of specific OGA inhibitors (31). These molecules interact directly with the active site of OGA, and include NButGT, Thiamet-G, and GlcNAcstatins. (2) NButGT (1,2- dideoxy-2'-propyl-α-D-glucopyranosO-(2,1-d)-Δ2'-thiazoline) is a competitive inhibitor of OGA and has good efficiency and specificity (Table 1). However, according to a study by Macauley et al. NButGT has a half-clearance of only 30 min in vivo, and lacks stability in solution (few days to weeks) (30). (3) Thiamet-G [(3aR,5R,6S,7R,7aR)-2-ethylaminO-3a,6,7,7a-tetrahydrO-5-(hydroxymethyl)-5H-pyrano(3,2-d)thiazole-6,7-diol] was developed several years later, and is more stable (31). (4) More recently, GlcNAcstatin has been described. It presents a molecular architecture noticeably similar to PUGNAc (Table 1) (32, 33), with a high selectivity and efficiency. Unfortunately, synthesis of GlcNAcstatin remains complex and very expensive (41).
Over the last decade, pharmacological modulators of O-GlcNAc levels have been developed for (i) improved knowledge of physiological conditions, as well as (ii) potential utilization as therapeutic strategies in different pathologies.
Potential Impact of Modulating O-GlcNAc Levels in Pathologies
The consequence of an increase in protein O-GlcNAcylation has mainly been evaluated in diabetes or cancers. In these pathologies, patients with high O-GlcNAc levels present with the poorest outcome. In this context, a reduction in O-GlcNAc levels appears to be an interesting therapeutic strategy. Alternatively, in acute pathologies, O-GlcNAc stimulation using different approaches to increase O-GlcNAc levels could be a promising therapeutic approach.
Increasing O-GlcNAc Levels in Acute Pathology, a Potent Therapeutic Approach?
Several studies have demonstrated the importance of O-GlcNAc response to a stress, and especially an increase in O-GlcNAc levels following this stress. This augmentation is reported to improve cell survival through a decrease in pro-apoptotic pathway molecules (p53, FOXO3, caspase 8 or GPAT1) and activation of sirtuin deacetylase (SIRT1) (42–45). Similarly, the beneficial effects of protein O-GlcNAcylation stimulation, with GlcN or siOGA, are associated with a decrease in apoptosis (46–48). These in vitro results were confirmed in vivo for different types of stress (e.g., hypoxia, inflammation, oxidative stress), and in different tissues and/or different pathologies.
In kidney, damage caused by hypoxia or an acute injury using a rabbit model are attenuated by GlcN administration (49). Hu et al. also reported an improvement in renal function and a decrease in apoptosis and oxidative stress markers, and these effects were abolished with alloxan (45). In a brain model, using a middle cerebral artery occlusion (MCAO), it was shown that an increase in O-GlcNAc levels, by GlcN or Thiamet G, resulted in reduced infarct volume and an improvement in cognitive function. These effects could be explained by a reduction in apoptosis and inflammation (suglia and NF-κB activation, reduction of cytokine production and leukocyte infiltration) (50–52). In hemorrhagic shock, hypovolemia is associated with an alteration in glucose utilization. Using in vitro (neonatal rat ventricular cardiomyocytes) and in vivo (rat) models of hemorrhagic shock Chatham's group demonstrated that augmentation of O-GlcNAc levels improved global outcomes. Specifically, GlcN improved organ perfusion, cardiac function, the inflammatory state and finally, increased survival (53, 54). Several years later, these results were confirmed with a specific inhibitor of OGA, PUGNAc, validating the potential therapeutic role of protein O-GlcNAcylation in this pathology (53, 55). In all these studies, cardiovascular function was significantly improved.
Ischemia-Reperfusion From ex vivo to in vivo
Myocardial infarction results from an obstruction of a coronary artery creating an ischemia leading to tissue necrosis. Reperfusion, by thrombolysis or invasive procedures, is the only way to preserve cardiac function and to save patient life and must be performed as early as possible and within the first 12 h. Unfortunately, reperfusion exacerbates cardiac injury through an excessive oxidative stress and inflammatory response. In this context, the introduction of an infarct-limiting therapy in clinical practice might have a clinical and socioeconomic impact (56).
The first evidence of the potential beneficial effects of O-GlcNAc was shown in vitro. Champattanachai and collaborators reported that an increase in O-GlcNAcylated proteins through GlcN infusion in rat neonatal cardiomyocytes improved cell viability following IR. Moreover, with the use of different pharmacological compounds (glucosamine, PUGNAc, azaserine, alloxan), they demonstrated a positive correlation between O-GlcNAc levels and cell viability in IR. According to the authors, the beneficial effects of GlcN are associated with a decrease in calcium overload and apoptosis through a reduction in mitochondrial permeability, transitional pores, or mPTP opening (57, 58). A second team confirmed these results by specifically targeting OGA. They modulated O-GlcNAc levels by PUGNAc, adenoviral overexpression of OGA or siRNA's against OGA, and demonstrated that an increase in O-GlcNAc levels increased cell viability and decreased apoptosis and oxidative stress in responses to IR (59, 60).
To confirm these initial in vitro findings, ex vivo studies have also been performed. A first study using a Langendorff model of IR demonstrated that glutamine improved cardiac function through an improvement of left cardiac function (ventricular pressures and heart rate) and a decrease in infarct size (cardiac troponin I release). According to these authors, this could be explained by a restoration in cellular ATP concentration (61). Once again, these beneficial effects were confirmed using an OGA inhibitor, NAG thiazolines, and this compound also reduced infarct size and mechanical arrhythmic activity (62).
Intriguingly, the number of in vivo studies is quite limited and only focuses on murine models. Considering the potential clinical impact of O-GlcNAc stimulation on cardiac function, it remains as an important step to continue toward clinical validation. The only direct in vivo evidence confirming in vitro results is from mice treated with PUGNAc at the reperfusion stage. This treatment efficiently reduced infarct size, apoptosis and mPTP opening (63). However, many in vivo studies indirectly suggest that O-GlcNAc stimulation could improve patient outcomes. For example, hearts submitted to preconditioning (two periods of 5 min ischemia and 5 min reperfusion) presented a higher myocardial glucose uptake and a higher protein O-GlcNAcylation, and a better recovery. In this context, the authors explained that the O-GlcNAc level increase was responsible for the cardioprotective preconditioning effect (64).
TOWARD a Potential Clinical Application
From Bench to Bedside, a Complicated Step
Several studies in cellular and animal models have demonstrated the potential beneficial effects of O-GlcNAc level augmentation in acute pathologies, and especially in cardiac IR. However, several limitations still exist and these need to be studied before a potential clinical application. Whilst acute O-GlcNAc level augmentation is cardioprotective in murine models, the adverse effects of a long-term exposure to high O-GlcNAc levels should also be considered. For instance, hearts isolated 1 month after myocardial infarction induced by coronary ligation presented high levels of protein O-GlcNAcylation, and especially higher O-GlcNAcylation of troponin T, and this observation was linked to higher cardiac dysfunction (65). Similarly, in rats subjected to hypoxic conditions (alternating 2 min 21% O2 and 2 min 6–8% O2 8 h per day) O-GlcNAc levels started to rise 2 weeks after the first stress. This observation was associated with higher apoptosis and inflammatory markers (66). Myocardial infarction and the resulting reperfusion injury is associated with cardiac remodeling, hypertrophy and heart failure, a situation aggravated by high O-GlcNAc levels, even if there is still no consensus regarding the link between O-GlcNAcylation of proteins and cardiac hypertrophy (67, 68). However, several studies have demonstrated an increase in cardiac protein O-GlcNAcylation in in vitro and in vivo models of hypertrophy (69–73). O-GlcNAc levels in the left ventricular myocardium were increased in patients with heart failure (73). Furthermore, increases in O-GlcNAc levels were associated with heart failure development (65, 74). In parallel, augmentation of protein O-GlcNAcylation turns out to be an adverse therapy for diabetic patients. In diabetic IR conditions, hyperglycemia and high O-GlcNAc levels are also associated with an aggravation of cardiac dysfunction and infarct size (75, 76).
In summary, before clinical trials can be conducted, more studies are necessary to characterize the potential long-term impact of O-GlcNAc stimulation and to evaluate the best dose, time-point and duration of treatment to avoid adverse effects.
To Future Potential Clinical Trial in Cardiology
Glutamine and glucosamine are metabolites used in the HBP pathway and could be a way to increase O-GlcNAc levels via an increase in HBP flux. These two molecules are already used clinically to treat inflammatory disease or improve cardiac function, and could provide proof of potential utilization in cardiac acute pathologies like IR. Despite this, their impact on O-GlcNAc level has never been tested in humans.
Glutamine supplementation is now recommended for parenteral or enteral supplementation in neonatal, pediatric and adult intensive care units and seems to be safe (26, 27). Moreover, in pediatric or adult intensive care units, patients with low plasma glutamine (<420 μmol/l) at admission are at higher risk of mortality and increased incidence of multiple organ failure (77, 78). Whilst this observation suggests a potential benefit from increasing glutamine concentration the consequence on protein O-GlcNAcylation has never been studied. Enteral glutamine supplementation has been shown to reduce the incidence of serious neonatal infections in preterm and/or very low birth weight children (79) and enterocolitis (80, 81). In critical conditions, glutamine enteral supplementation decreases the incidence of sepsis, pneumonia, and bacteremia in trauma (82) and burn patients (83). Moreover, glutamine may have a perioperative cardioprotective role. Glutamine use in patients with ischemic heart disease operated under conditions of extracorporeal blood circulation or cardiopulmonary bypass reduces troponin release at day 1, the systemic vascular resistance index and improves cardiac and stroke index (84, 85). As well, perioperative glutamine supplementation during aortic surgery can compensate renal arginine synthesis loss induced by aortic clamping and could also improve post-operative renal function (86). A recent clinical trial showed that patients who receive oral glutamine have less complications, myocardial damage, morbidity and mortality after coronary revascularization under cardiopulmonary bypass (87). A similar protocol in chronic angina patients, delayed the time to onset of more than 1.0 mm of ST segment depression on the electrocardiogram (ECG) by 38 s, but did not improve hemodynamic response to exercise, the time of onset of angina symptoms, maximum workload or total exercise time (88).
GlcN is largely used for osteoarthritis at an average dose of 1,500 mg per day but its usefulness in other pathologies has not been explored in clinical trials. Whereas, long term treatment seems to delay the progression of knee arthritis (28), multiple studies have shown no superiority of glucosamine vs. placebo (89), no improvement of cartilage damage (90) and no role in prevention of osteoarthritis in overweight women (91). Moreover, at the usual doses, GlcN may induce an increase in intraocular pressure (92). This possible deleterious effect of high dose GlcN is supported by a recent review that showed this molecule is not beneficial for all population subgroups (93). Clinical use of GlcN has been associated with potential side effects in vivo, among them vomiting and diarrhea (94) and has also been associated with intracellular ATP depletion (95). Overall, GlcN utilization for joint pain appears to be safe.
Glutamine or GlcN supplementation has demonstrated its benefits for a few pathologies but these molecules are not specific to the HBP, and the link between the benefits and an increase in protein O-GlcNAcylation has not been demonstrated. Recently, new molecules targeting OGA have been studying for the treatment of tauopathy. MK-8719, a selective and potent small molecule inhibitor of OGA has shown promising results in the treatment of tauopathy such as Progressive Supranuclear Palsy (PSP). It has been evaluated in a recent phase I study in healthy volunteers. Interestingly, MK-8719 administration elicited PBMC O-GlcNAcylated protein increases in a dose dependent-manner, consistent with preclinical observations. Moreover, ASN120290, a brain-permeable small-molecule OGA inhibitor, has been also studied in a randomized, double-blind, placebo-controlled phase I study. These molecules seem to be safe and well tolerated (96).
These molecules represent a huge opportunity for progression to future clinical trials. Despite the beneficial impact of an increase in total O-GlcNAc level in IR, the treatment remains non-selective and can have potential side effects that have not been clearly evaluated in most studies. In future studies, more attention should be paid to doses and administration time to avoid any drawback.
What Should be Confirmed Prior to Performing Clinical Trials?
Understanding Metabolism and O-GlcNAc Level Variation Through Aging
O-GlcNAc levels are linked to GFAT activity and cellular metabolism, particularly that of glucose. This observation is particularly important in cardiac tissue as cardiac metabolism constantly adapts to conditions and evolves throughout the first stage of life, especially the substrate selection for energy production. The predominant substrates for energy production in fetal hearts are carbohydrates (mainly glucose, lactate and pyruvate) and cardiac metabolism is mainly anaerobic. After birth, the ability of hearts to oxidize fatty acids increases within the first week (97), and in the adult heart, energy is mainly supplied by fatty acid oxidation (60–80%), carbohydrates (20–30%) and ketone bodies (10%). These proportions are constantly modulated to fit requirements and substrate availability. During fetal life, cardiac glucose uptake is controlled by a low affinity insulin-independent glucose membrane transporter, Glucose transporter type I (GLUT1). Shortly after birth, cardiac glucose transporters switch from the GLUT1 isoform to the GLUT4 isoform. GLUT4 is an insulin-sensitive glucose transporter, which is the predominant transporter in the adult heart (97). This observation is of particular importance as changes in glucose transporter expression, such as GLUT1 and GLUT4, have been shown to influence UDP-GlcNAc levels in mice. Interestingly, GFAT activity and glucose flux via the HBP are increased in muscles of GLUT1-overexpressing mice but not GLUT4-overexpressing mice (98). Consequently, during the first days of life, cardiac metabolism is subjected to dramatic changes with a major increase in fatty acid oxidation and a reduction in carbohydrate metabolism, whilst the impact on protein O-GlcNAcylation remains unknown. Altogether, the metabolic modification associated with the first days of life could impact O-GlcNAc levels and be of great importance in cardiac development and maturation. They could also be responsible for the higher capacity of the heart to withstand stress during the first days of life (99–101). Furthermore, in situations of stress or specific pathological conditions, the proportion of carbohydrates metabolized will increase to sustain cardiac needs. In the long run, this modification will affect HBP flux and modulate O-GlcNAc levels resulting in a potential impact on cardiac function. Interestingly, no study has evaluated if these modifications actually have an impact on cardiac O-GlcNAcylation.
While metabolism is subject to modifications throughout aging, and while protein O-GlcNAcylation has been described as a metabolic sensor, the exact link between metabolism's age-associated variations and O-GlcNAc levels has never been explored. In fact, many authors have only focused on senescence, and demonstrated that O-GlcNAc levels have an impact on the development and the progression of chronic diseases (102, 103).
The variation of O-GlcNAc levels during aging has not reached a consensus yet. Fülöp et al. showed a decrease in O-GlcNAc levels in rat hearts which was associated with a reduction in OGT expression between adolescence (6 weeks) and adulthood (22 weeks) (104). However, in another study, the authors showed an increase in O-GlcNAc levels in rat hearts between 5 and 24 months, which surprisingly, was associated with a decrease in OGT expression (105). In the brain, which is the most studied organ for this question, age-associated variation of protein O-GlcNAcylation is not clear. O-GlcNAc levels rapidly decrease between the 1st and the 24th month (106) or increase between 5 and 24th month (105), whereas Rex-Mathes et al. showed no change in protein O-GlcNAcylation between the 3rd and 13th month (107) (Figure 2).
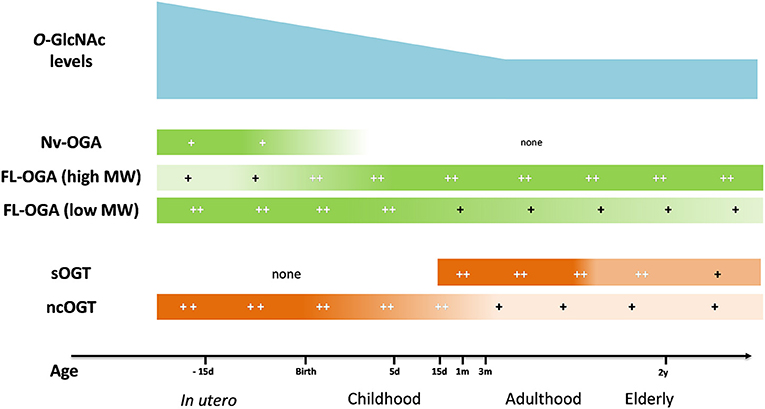
Figure 2. Evolution of O-GlcNAc levels, OGA and OGT expression in brain tissue throughout lifespan. OGA, O-GlcNAcase; OGT, O-GlcNAc transferase; FL-OGA, full-length OGA; high MW, high molecular weight, low MW, low molecular weight; Nv-OGA, Nuclear variant OGA; ncOGT, nucleo-cytoplasmic OGT; sOGT, short OGT;−15 d, 15 days before birth; 5 d, 5 days post-natal; 15 d, 15 days; 1 m, 1 month; 3 m, 3 months; 2 y, 2 years (105–107).
The lack of consensus on O-GlcNAc level variation throughout aging and in organ function underlines the need for new standardized studies to improve the understanding of O-GlcNAc levels on cellular development, survival and potential impacts on pathology or treatment responses.
Doses and Timing of Treatment
The ambiguous effects of O-GlcNAc is not restricted to the differences in basal O-GlcNAc levels, as it is now evident that there are dose-dependent effects of protein O-GlcNAcylation. The beneficial effects associated with increase in O-GlcNAc are lost for high increase in O-GlcNAc level. Gu et al. showed for brain IR that a high increase in O-GlcNAc levels (~7 fold) compared to a moderate increase in O-GlcNAc level (~3 fold) leads to a detrimental effect with an increase in infarct size. Strikingly, most of the beneficial effects described in the literature are associated with a 2–5 fold increase in protein O-GlcNAcylation levels in the heart (45, 57, 61), and in the brain (51, 108–110). Champattanachai et al. showed similar results, and highlighted a close link between O-GlcNAc levels and cell viability in their model (57). Unfortunately, few studies have focused on O-GlcNAc level modulation in physiological or pathophysiological conditions. In addition, the same authors showed that GlcN increases the level of protein O-GlcNAcylation by 1.5 fold in normoxia, and by 3 fold in hypoxia (57). The stimulation of this PTM could induce different responses in pathophysiological conditions. Interestingly, a recent clinical study showed that delayed sepsis treatment using 0.35 g/kg of glutamine per day i.v. and 30 g per day glutamine given via enteral administration to patients with two or more failing organs, had no effect on the outcome of organ failure and infections. It even increased mortality in hospital and at 6 months (111). These results might be explained by the high dose of glutamine (dose-dependent effect) and/or the late initiation of the protocol (time-dependent effect).
The lack of data on dose and time of treatment remains the key safety issue that needs to be satisfied in order to progress toward a clinical trial. Only one study has focused on establishing an optimal glutamine supplementation dose in pediatric cancer patients, however they did not evaluate the impact on O-GlcNAc levels (112).
Improvement of Tools
In order to improve the understanding of the impact of O-GlcNAc on proteins and cell survival considerable progress has been made in developing new tools. Three main themes are of particular importance and have gained momentum over the last couple of years: (i) identifying O-GlcNAcylation sites on protein using mass spectrometry, (ii) improving pharmacological tools to improve specificity and biocompatibility, and (iii) understanding the impact of O-GlcNAcylation on specific proteins.
Emerging Analytical Tools
As discussed above, there is a significant conundrum for protein O-GlcNAcylation, with on one-hand, potential short-term beneficial effects and on the other hand long-term deleterious effects. It is important to decipher which O-GlcNAc sites are of interest and potentially beneficial or potentially detrimental. Hopefully, in the near future, specifically targeting these sites will potentiate the beneficial effects and limit adverse effects associated with untargeted increases in O-GlcNAc level. Global mapping of O-GlcNAcylated proteins and peptides has recently been possible using mass spectrometry approaches. Recent advances in analytical techniques have also allowed to determine O-GlcNAcylation sites. For example, Thompson et al. highlighted “emerging technologies for quantitative, site-specific MS-based O-GlcNAc proteomics (O-GlcNAcomics), which allow proteome-wide tracking of O-GlcNAcylation dynamics at individual sites.” In this article, the authors listed the current technique for O-GlcNAc identification using mass spectrometry (6). Many papers have described the successful use of a combination of fractionation and click chemistry to label and identify O-GlcNAc sites on proteins. For example, Griffin et al. extensively studied the O-GlcNAcylation site on OGT with a chemically cleavable tag and suggested a potential implication of O-GlcNAc on the regulation of protein function (113). More recently, Deracinois et al. used this approach on skeletal muscle proteins and reported that some O-GlcNAcylation sites were located in interaction sites that open new area of research for this PTM (114). Thanks to the development and thorough validation of these new tools, consensus sequences have recently been proposed (115). Recent advances in technologies represent a huge opportunity and will definitely help to improve the understanding of the role of this PTM.
Toward Specific Pharmacological Tools
Pharmacology has long been used to study different pathways and targets, and OGA, GFAT and OGT inhibitors have been known about since the early 90's. At present however, the main drawback of these compounds are their lack of specificity and affinity and their toxicity.
OGA inhibitors have been most beneficial and are now available for clinical trials. In fact, they have been the prime targets to develop a therapeutic strategy (29). The remaining challenge is in the potential directed system administration, in order to limit the potential off target effects. While the use of enzymatically triggered prodrugs is well known in the field of cancer, this strategy is poorly developed for treatment of other pathologies. In parallel, a second strategy could be to design new OGA inhibitors targeting specific organelles (e.g., mitochondria) or organs. Such tools would present two major advantages as they would help to: (i) decipher the role of this PTM on different organelles and (ii) improve treatment specificity. On the other hand, while OGT inhibitors potentially represent an interesting therapeutic strategy for cancer, they still lack specificity, are not cell permeant or are toxic.
Recent advances in crystallography opens potentially new avenues to study the impact of O-GlcNAc levels on cellular function. The next step will be to specifically target cellular compartments and/or specific proteins to be able to maximize potential beneficial impacts on selected pathways or cellular processes (61, 115–118).
Site Specific Evaluation
Recent advances in O-GlcNAc proteomics has produced a very large quantity of potential sites to explore. It is now important to develop screening tools to be able to further advance our understanding of protein O-GlcNAcylation. Because only two enzymes are involved in O-GlcNAcylation, a solution may rely on biochemistry, and more specifically on site-specific mutagenesis through the incorporation of non-natural amino acids (NAA) such as selenocysteine derivatives. NAA are useful tools to add new properties to proteins at specific positions. They can be incorporated into a protein sequence during translation through genetic code expansion by an orthogonal (i.e., not interfering with the natural amino acids system) aminoacyl-tRNA synthetase (aaRS). This TAG codon is positioned in an appropriate position into the recombinant proteins gene (119, 120). Protein function could then be evaluated through enzymatic assays for example.
Conclusion
Over the last 30 years, knowledge on protein O-GlcNAcylation has increased considerably in many areas, yet, the cardiovascular field remains largely underexplored. A pubmed search using the “cardiovascular” and “O-GlcNAc” MeSH term retrieved only 187 papers in October 2018. More effort has been expended on chronic pathologies such as diabetes, cancer, and Alzheimers disease, leading to potential new approaches for the patient. On the acute side, augmentation of O-GlcNAc levels may represent a new therapeutic solution for cardiovascular dysfunction or ischemia/reperfusion, yet its potential harmful effects at higher doses, or the impact of long term stimulation remain to be determined. Furthermore, deciphering which protein or pathway is involved in O-GlcNAc effects represents the key element to be able to specifically target them. The future may be hidden in organelle specific O-GlcNAc modulation or in new proteomic approaches with powerful tools to study O-GlcNAcylation. Increasing the understanding of this very specific PTM will open complete new area of research for protein-targeted mutation.
Author Contributions
MF, MD, AP, and RR wrote the review. BL corrected the final manuscript.
Conflict of Interest Statement
The authors declare that the research was conducted in the absence of any commercial or financial relationships that could be construed as a potential conflict of interest.
Acknowledgments
We would like to thank Dr. Bryce Van Denderen for English revision. We would also thank Fédération Française de Cardiologie, Fondation d'entreprises Génavie for their financial support and the IBISA core facility Therassay for its assistance and their technical support.
References
1. Torres CR, Hart GW. Topography and polypeptide distribution of terminal N-acetylglucosamine residues on the surfaces of intact lymphocytes. Evidence for O-linked GlcNAc. J Biol Chem. (1984) 259:3308–17.
2. Holt GD, Hart GW. The subcellular distribution of terminal N-acetylglucosamine moieties. Localization of a novel protein-saccharide linkage, O-linked GlcNAc. J Biol Chem. (1986) 261:8049–57.
3. Schindler M, Hogan M, Miller R, DeGaetano D. A nuclear specific glycoprotein representative of a unique pattern of glycosylation. J Biol Chem. (1987) 262:1254–60.
4. Yang X, Qian K. Protein O-GlcNAcylation: emerging mechanisms and functions. Nat Rev Mol Cell Biol. (2017) 18:452–65. doi: 10.1038/nrm.2017.22
5. Ma J, Hart GW. O-GlcNAc profiling: from proteins to proteomes. Clin Proteomics (2014) 11:8. doi: 10.1186/1559-0275-11-8
6. Thompson JW, Griffin ME, Hsieh-Wilson LC. Methods for the detection, study, and dynamic profiling of O-GlcNAc glycosylation. Methods Enzymol. (2018) 598:101–35. doi: 10.1016/bs.mie.2017.06.009
7. Hart GW, Slawson C, Ramirez-Correa G, Lagerlof O. Cross talk between O-GlcNAcylation and phosphorylation: roles in signaling, transcription, and chronic disease. Annu Rev Biochem. (2011) 80:825–58. doi: 10.1146/annurev-biochem-060608-102511
8. Love DC, Hanover JA. The hexosamine signaling pathway: deciphering the “O-GlcNAc code.” Sci. STKE (2005) 2005:re13. doi: 10.1126/stke.3122005re13
9. Marshall S, Bacote V, Traxinger RR. Discovery of a metabolic pathway mediating glucose-induced desensitization of the glucose transport system. Role of hexosamine biosynthesis in the induction of insulin resistance. J Biol Chem. (1991) 266:4706–12.
10. Coffey GL, Ehrlich J, Fisher MW, Hillegas AB, Kohberger DL, Machamer HE, et al. 6-Diazo-5-oxo-L-norleucine, a new tumor-inhibitory substance. I Biologic studies. Antibiot Chemother. (1956) 6:487–97.
11. Stock CC, Reilly HC, Buckley SM, Clarke DA, Rhoads CP. Azaserine, a new tumour-inhibitory substance; studies with Crocker mouse sarcoma 180. Nature (1954) 173:71–2. doi: 10.1038/173071a0
12. Milewski S. Glucosamine-6-phosphate synthase–the multi-facets enzyme. Biochim Biophys Acta (2002) 1597:173–92. doi: 10.1016/S0167-4838(02)00318-7
13. Cervantes-Madrid D, Romero Y, Dueñas-González A. Reviving lonidamine and 6-diazo-5-oxo-L-norleucine to be used in combination for metabolic cancer therapy. BioMed Res Int. (2015) 2015:690492. doi: 10.1155/2015/690492
14. Ostrowski A, van Aalten DMF. Chemical tools to probe cellular O-GlcNAc signalling. Biochem J. (2013) 456:1–12. doi: 10.1042/BJ20131081
15. Lee TN, Alborn WE, Knierman MD, Konrad RJ. Alloxan is an inhibitor of O-GlcNAc-selective N-acetyl-beta-D-glucosaminidase. Biochem Biophys Res Commun. (2006) 350:1038–43. doi: 10.1016/j.bbrc.2006.09.155
16. Trapannone R, Rafie K, van Aalten DMF. O-GlcNAc transferase inhibitors: current tools and future challenges. Biochem Soc Trans. (2016) 44:88–93. doi: 10.1042/BST20150189
17. Advani Shyam B, Sam J. Potential anticancer and antiviral agents. Substituted 3-[1′(2′,3′,4′-tri-O-benzoyl-β-d-ribopyranosyl)]-2-benzoxazolinones. J Heterocycl Chem. (1968) 5:119–22. doi: 10.1002/jhet.5570050123
18. Caldwell SA, Jackson SR, Shahriari KS, Lynch TP, Sethi G, Walker S, et al. Nutrient sensor O-GlcNAc transferase regulates breast cancer tumorigenesis through targeting of the oncogenic transcription factor FoxM1. Oncogene (2010) 29:2831–42. doi: 10.1038/onc.2010.41
19. Itkonen HM, Minner S, Guldvik IJ, Sandmann MJ, Tsourlakis MC, Berge V, et al. O-GlcNAc transferase integrates metabolic pathways to regulate the stability of c-MYC in human prostate cancer cells. Cancer Res. (2013) 73:5277–87. doi: 10.1158/0008-5472.CAN-13-0549
20. Jiang J, Lazarus MB, Pasquina L, Sliz P, Walker S. A neutral diphosphate mimic crosslinks the active site of human O-GlcNAc transferase. Nat Chem Biol. (2011) 8:72–7. doi: 10.1038/nchembio.711
21. Celiberto LS, Chan JY, Law HT, Bhullar K, Xia L, Cavallini DC, et al. A10 core-1 derived o-glycosylation of the mucin muc2 plays a key role in host defense against enteric citrobacter rodentium infection. J Can Assoc Gastroenterol. (2018) 1:15–6. doi: 10.1093/jcag/gwy009.010
22. Hennebicq-Reig S, Lesuffleur T, Capon C, De Bolos C, Kim I, Moreau O, et al. Permanent exposure of mucin-secreting HT-29 cells to benzyl-N-acetyl-alpha-D-galactosaminide induces abnormal O-glycosylation of mucins and inhibits constitutive and stimulated MUC5AC secretion. Biochem J. (1998) 334:283–95.
23. Olvera A, Martinez JP, Casadell,à M, Llano A, Rosás M, Mothe B, et al. Benzyl-2-acetamido-2-deoxy-α-d-galactopyranoside increases human immunodeficiency virus replication and viral outgrowth efficacy in vitro. Front Immunol. (2018) 8:2010. doi: 10.3389/fimmu.2017.02010
24. Gloster TM, Zandberg WF, Heinonen JE, Shen DL, Deng L, Vocadlo DJ. Hijacking a biosynthetic pathway yields a glycosyltransferase inhibitor within cells. Nat Chem Biol. (2011) 7:174–81. doi: 10.1038/nchembio.520
25. Ma Z, Vosseller K. Cancer metabolism and elevated O-GlcNAc in oncogenic signaling. J. Biol. Chem. (2014) 289:34457–65. doi: 10.1074/jbc.R114.577718
26. Thompson SW, McClure BG, Tubman TRJ. A randomized, controlled trial of parenteral glutamine in ill, very low birth-weight neonates. J Pediatr Gastroenterol Nutr. (2003) 37:550–3. doi: 10.1097/00005176-200311000-00008
27. van den Berg A, van Elburg RM, Teerlink T, Lafeber HN, Twisk JWR, Fetter WPF. A randomized controlled trial of enteral glutamine supplementation in very low birth weight infants: plasma amino acid concentrations. J Pediatr Gastroenterol Nutr. (2005) 41:66–71. doi: 10.1097/01.mpg.0000167497.55321.65
28. Pavelká K, Gatterová J, Olejarová M, Machacek S, Giacovelli G, Rovati LC. Glucosamine sulfate use and delay of progression of knee osteoarthritis: a 3-year, randomized, placebo-controlled, double-blind study. Arch Intern Med. (2002) 162:2113–23. doi: 10.1001/archinte.162.18.2113
29. Macauley MS, Vocadlo DJ. Enzymatic characterization and inhibition of the nuclear variant of human O-GlcNAcase. Carbohydr Res. (2009) 344:1079–84. doi: 10.1016/j.carres.2009.04.017
30. Macauley MS, Whitworth GE, Debowski AW, Chin D, Vocadlo DJ. O-GlcNAcase uses substrate-assisted catalysis: kinetic analysis and development of highly selective mechanism-inspired inhibitors. J Biol Chem. (2005) 280:25313–22. doi: 10.1074/jbc.M413819200
31. Yuzwa SA, Macauley MS, Heinonen JE, Shan X, Dennis RJ, He Y, et al. A potent mechanism-inspired O-GlcNAcase inhibitor that blocks phosphorylation of tau in vivo. Nat Chem Biol. (2008) 4:483–90. doi: 10.1038/nchembio.96
32. Dorfmueller HC, Borodkin VS, Schimpl M, van Aalten DMF. GlcNAcstatins are nanomolar inhibitors of human O-GlcNAcase inducing cellular hyper-O-GlcNAcylation. Biochem J. (2009) 420:221–7. doi: 10.1042/BJ20090110
33. Dorfmueller HC, Borodkin VS, Schimpl M, Shepherd SM, Shpiro NA, van Aalten DMF. GlcNAcstatin: a picomolar, selective O-GlcNAcase inhibitor that modulates intracellular O-glcNAcylation levels. J Am Chem Soc. (2006) 128:16484–5. doi: 10.1021/ja066743n
34. Lenzen S, Panten U. Alloxan: history and mechanism of action. Diabetologia (1988) 31:337–42. doi: 10.1007/BF02341500
35. Konrad RJ, Zhang F, Hale JE, Knierman MD, Becker GW, Kudlow JE. Alloxan is an inhibitor of the enzyme O-linked N-acetylglucosamine transferase. Biochem Biophys Res Commun. (2002) 293:207–12. doi: 10.1016/S0006-291X(02)00200-0
36. Kang E-S, Han D, Park J, Kwak TK, Oh M-A, Lee S-A, et al. O-GlcNAc modulation at Akt1 Ser473 correlates with apoptosis of murine pancreatic β cells. Exp Cell Res. (2008) 314:2238–48. doi: 10.1016/j.yexcr.2008.04.014
37. Pantaleon M, Tan HY, Kafer GR, Kaye PL. Toxic effects of hyperglycemia are mediated by the hexosamine signaling pathway and O-linked glycosylation in early mouse embryos. Biol Reprod. (2010) 82:751–8. doi: 10.1095/biolreprod.109.076661
38. Marshall S, Nadeau O, Yamasaki K. Dynamic actions of glucose and glucosamine on hexosamine biosynthesis in isolated adipocytes: differential effects on glucosamine 6-phosphate, UDP-N-acetylglucosamine, and ATP levels. J Biol Chem. (2004) 279:35313–9. doi: 10.1074/jbc.M404133200
39. Duan W, Paka L, Pillarisetti S. Distinct effects of glucose and glucosamine on vascular endothelial and smooth muscle cells: evidence for a protective role for glucosamine in atherosclerosis. Cardiovasc Diabetol. (2005) 4:16. doi: 10.1186/1475-2840-4-16
40. Little PJ, Drennon KD, Tannock LR. Glucosamine inhibits the synthesis of glycosaminoglycan chains on vascular smooth muscle cell proteoglycans by depletion of ATP. Arch Physiol Biochem. (2008) 114:120–6. doi: 10.1080/13813450802033909
41. Borodkin VS, van Aalten DMF. An efficient and versatile synthesis of GlcNAcstatins-potent and selective O-GlcNAcase inhibitors built on the tetrahydroimidazo[1,2-a]pyridine scaffold. Tetrahedron (2010) 66:7838–49. doi: 10.1016/j.tet.2010.07.037
42. Chuh KN, Batt AR, Zaro BW, Darabedian N, Marotta NP, Brennan CK, et al. The new chemical reporter 6-alkynyl-6-deoxy-GlcNAc reveals O-GlcNAc modification of the apoptotic caspases that can block the cleavage/activation of caspase-8. J Am Chem Soc. (2017) 139:7872–85. doi: 10.1021/jacs.7b02213
43. Groves JA, Maduka AO, O'Meally RN, Cole RN, Zachara NE. Fatty acid synthase inhibits theO-GlcNAcase during oxidative stress. J Biol Chem. (2017) 292:6493–511. doi: 10.1074/jbc.M116.760785
44. Han C, Gu Y, Shan H, Mi W, Sun J, Shi M, et al. O-GlcNAcylation of SIRT1 enhances its deacetylase activity and promotes cytoprotection under stress. Nat Commun. (2017) 8:1491. doi: 10.1038/s41467-017-01654-6
45. Hu J, Chen R, Jia P, Fang Y, Liu T, Song N, et al. Augmented O-GlcNAc signaling via glucosamine attenuates oxidative stress and apoptosis following contrast-induced acute kidney injury in rats. Free Radic Biol Med. (2017) 103:121–32. doi: 10.1016/j.freeradbiomed.2016.12.032
46. Chen Y-J, Huang Y-S, Chen J-T, Chen Y-H, Tai M-C, Chen C-L, et al. Protective effects of glucosamine on oxidative-stress and ischemia/reperfusion-induced retinal injury. Invest Ophthalmol Vis Sci. (2015) 56:1506–16. doi: 10.1167/iovs.14-15726
47. Lee HJ, Ryu JM, Jung YH, Lee KH, Kim DI, Han HJ. Glycerol-3-phosphate acyltransferase-1 upregulation by O-GlcNAcylation of Sp1 protects against hypoxia-induced mouse embryonic stem cell apoptosis via mTOR activation. Cell Death Dis. (2016) 7:e2158. doi: 10.1038/cddis.2015.410
48. Luanpitpong S, Chanthra N, Janan M, Poohadsuan J, Samart P, U-Pratya Y, et al. Inhibition ofO-GlcNAcase sensitizes apoptosis and reverses bortezomib resistance in mantle cell lymphoma through modification of truncated bid. Mol. Cancer Ther. (2018) 17:484–96. doi: 10.1158/1535-7163.MCT-17-0390
49. Suh HN, Lee YJ, Kim MO, Ryu JM, Han HJ. Glucosamine-induced Sp1 O-GlcNAcylation ameliorates hypoxia-induced SGLT dysfunction in primary cultured renal proximal tubule cells. J Cell Physiol. (2014) 229:1557–68. doi: 10.1002/jcp.24599
50. He Y, Ma X, Li D, Hao J. Thiamet G mediates neuroprotection in experimental stroke by modulating microglia/macrophage polarization and inhibiting NF-κB p65 signaling. J Cereb Blood Flow Metab Off J Int Soc Cereb Blood Flow Metab. (2017) 37:2938–51. doi: 10.1177/0271678X16679671
51. Hwang S-Y, Shin J-H, Hwang J-S, Kim S-Y, Shin J-A, Oh E-S, et al. Glucosamine exerts a neuroprotective effect via suppression of inflammation in rat brain ischemia/reperfusion injury. Glia (2010) 58:1881–92. doi: 10.1002/glia.21058
52. Shi J, Gu J, Dai C, Gu J, Jin X, Sun J, et al. O-GlcNAcylation regulates ischemia-induced neuronal apoptosis through AKT signaling. Sci Rep. (2015) 5:14500. doi: 10.1038/srep14500
53. Nöt LG, Marchase RB, Fülöp N, Brocks CA, Chatham JC. Glucosamine administration improves survival rate after severe hemorrhagic shock combined with trauma in rats. Shock Augusta Ga (2007) 28:345–52. doi: 10.1097/shk.0b013e3180487ebb
54. Yang S, Zou L-Y, Bounelis P, Chaudry I, Chatham JC, Marchase RB. Glucosamine administration during resuscitation improves organ function after trauma hemorrhage. Shock Augusta Ga (2006) 25:600–7. doi: 10.1097/01.shk.0000209563.07693.db
55. Zou L, Yang S, Hu S, Chaudry IH, Marchase RB, Chatham JC. The protective effects of PUGNAc on cardiac function after trauma-hemorrhage are mediated via increased protein O-GlcNAc levels. Shock Augusta Ga (2007) 27:402–8. doi: 10.1097/01.shk.0000245031.31859.29
56. Ibanez B, James S, Agewall S, Antunes MJ, Bucciarelli-Ducci C, Bueno H, et al. 2017 ESC Guidelines for the management of acute myocardial infarction in patients presenting with ST-segment elevation: the task force for the management of acute myocardial infarction in patients presenting with ST-segment elevation of the European Society of Cardiology (ESC). Eur Heart J. (2018) 39:119–77. doi: 10.1093/eurheartj/ehx393
57. Champattanachai V, Marchase RB, Chatham JC. Glucosamine protects neonatal cardiomyocytes from ischemia-reperfusion injury via increased protein-associated O -GlcNAc. Am J Physiol-Cell Physiol. (2007) 292:C178–87. doi: 10.1152/ajpcell.00162.2006
58. Champattanachai V, Marchase RB, Chatham JC. Glucosamine protects neonatal cardiomyocytes from ischemia-reperfusion injury via increased protein O-GlcNAc and increased mitochondrial Bcl-2. Am J Physiol Cell Physiol. (2008) 294:C1509–20. doi: 10.1152/ajpcell.00456.2007
59. Ngoh GA, Hamid T, Prabhu SD, Jones SP. O-GlcNAc signaling attenuates ER stress-induced cardiomyocyte death. Am J Physiol Heart Circ Physiol. (2009) 297:H1711–9. doi: 10.1152/ajpheart.00553.2009
60. Ngoh GA, Watson LJ, Facundo HT, Jones SP. Augmented O-GlcNAc signaling attenuates oxidative stress and calcium overload in cardiomyocytes. Amino Acids (2011) 40:895–911. doi: 10.1007/s00726-010-0728-7
61. Liu J, Marchase RB, Chatham JC. Glutamine-induced protection of isolated rat heart from ischemia/reperfusion injury is mediated via the hexosamine biosynthesis pathway and increased protein O-GlcNAc levels. J Mol Cell Cardiol. (2007) 42:177–85. doi: 10.1016/j.yjmcc.2006.09.015
62. Laczy B, Marsh SA, Brocks CA, Wittmann I, Chatham JC. Inhibition of O-GlcNAcase in perfused rat hearts by NAG-thiazolines at the time of reperfusion is cardioprotective in an O-GlcNAc-dependent manner. Am J Physiol Heart Circ Physiol. (2010) 299:H1715–27. doi: 10.1152/ajpheart.00337.2010
63. Jones SP, Zachara NE, Ngoh GA, Hill BG, Teshima Y, Bhatnagar A, et al. Cardioprotection by N-Acetylglucosamine Linkage to Cellular Proteins. Circulation (2008) 117:1172–82. doi: 10.1161/CIRCULATIONAHA.107.730515
64. Pælestik KB, Jespersen NR, Jensen RV, Johnsen J, Bøtker HE, Kristiansen SB. Effects of hypoglycemia on myocardial susceptibility to ischemia–reperfusion injury and preconditioning in hearts from rats with and without type 2 diabetes. Cardiovasc Diabetol. (2017) 16:148. doi: 10.1186/s12933-017-0628-1
65. Dubois-Deruy E, Belliard A, Mulder P, Bouvet M, Smet-Nocca C, Janel S, et al. Interplay between troponin T phosphorylation and O-N-acetylglucosaminylation in ischaemic heart failure. Cardiovasc Res. (2015) 107:56–65. doi: 10.1093/cvr/cvv136
66. Guo X, Shang J, Deng Y, Yuan X, Zhu D, Liu H. Alterations in left ventricular function during intermittent hypoxia: possible involvement of O-GlcNAc protein and MAPK signaling. Int J Mol Med. (2015) 36:150–8. doi: 10.3892/ijmm.2015.2198
67. Dassanayaka S, Brainard RE, Watson LJ, Long BW, Brittian KR, DeMartino AM, et al. Cardiomyocyte Ogt limits ventricular dysfunction in mice following pressure overload without affecting hypertrophy. Basic Res Cardiol. (2017) 112:23. doi: 10.1007/s00395-017-0612-7
68. Mailleux F, Gélinas R, Beauloye C, Horman S, Bertrand L. O-GlcNAcylation, enemy or ally during cardiac hypertrophy development? Biochim Biophys Acta (2016) 1862:2232–43. doi: 10.1016/j.bbadis.2016.08.012
69. Cannon MV, Silljé HHW, Sijbesma JWA, Vreeswijk-Baudoin I, Ciapaite J, van der Sluis B, et al. Cardiac LXRα protects against pathological cardiac hypertrophy and dysfunction by enhancing glucose uptake and utilization. EMBO Mol. Med. (2015) 7:1229–43. doi: 10.15252/emmm.201404669
70. Facundo HT, Brainard RE, Watson LJ, Ngoh GA, Hamid T, Prabhu SD, et al. O-GlcNAc signaling is essential for NFAT-mediated transcriptional reprogramming during cardiomyocyte hypertrophy. Am J Physiol Heart Circ Physiol. (2012) 302:H2122–30. doi: 10.1152/ajpheart.00775.2011
71. Gélinas R, Mailleux F, Dontaine J, Bultot L, Demeulder B, Ginion A, et al. AMPK activation counteracts cardiac hypertrophy by reducing O-GlcNAcylation. Nat Commun. (2018) 9:374. doi: 10.1038/s41467-017-02795-4
72. Ledee D, Smith L, Bruce M, Kajimoto M, Isern N, Portman MA, et al. c-Myc alters substrate utilization and O-GlcNAc protein posttranslational modifications without altering cardiac function during early aortic constriction. PLoS ONE (2015) 10:e0135262. doi: 10.1371/journal.pone.0135262
73. Lunde IG, Aronsen JM, Kvaløy H, Qvigstad E, Sjaastad I, Tønnessen T, et al. Cardiac O-GlcNAc signaling is increased in hypertrophy and heart failure. Physiol Genomics (2012) 44:162–72. doi: 10.1152/physiolgenomics.00016.2011
74. Muthusamy S, DeMartino AM, Watson LJ, Brittian KR, Zafir A, Dassanayaka S, et al. MicroRNA-539 is up-regulated in failing heart, and suppresses O-GlcNAcase expression. J Biol Chem. (2014) 289:29665–76. doi: 10.1074/jbc.M114.578682
75. Liu B, Wang J, Li M, Yuan Q, Xue M, Xu F, et al. Inhibition of ALDH2 by O-GlcNAcylation contributes to the hyperglycemic exacerbation of myocardial ischemia/reperfusion injury. Oncotarget (2017) 8:19413–26. doi: 10.18632/oncotarget.14297
76. Wang D, Hu X, Lee SH, Chen F, Jiang K, Tu Z, et al. Diabetes exacerbates myocardial ischemia/reperfusion injury by down-regulation of microRNA and up-regulation of O-GlcNAcylation. JACC Basic Transl Sci. (2018) 3:350–62. doi: 10.1016/j.jacbts.2018.01.005
77. Ekmark L, Rooyackers O, Wernerman J, Fläring U. Plasma glutamine deficiency is associated with multiple organ failure in critically ill children. Amino Acids (2015) 47:535–42. doi: 10.1007/s00726-014-1885-x
78. Oudemans-van Straaten HM, Bosman RJ, Treskes M, van der Spoel HJ, Zandstra DF. Plasma glutamine depletion and patient outcome in acute ICU admissions. Intensive Care Med. (2001) 27:84–90. doi: 10.1007/s001340000703
79. Neu J, Roig JC, Meetze WH, Veerman M, Carter C, Millsaps M, et al. Enteral glutamine supplementation for very low birth weight infants decreases morbidity. J Pediatr. (1997) 131:691–9. doi: 10.1016/S0022-3476(97)70095-7
80. Bober-Olesinska K, Kornacka MK. [Effects of glutamine supplemented parenteral nutrition on the incidence of necrotizing enterocolitis, nosocomial sepsis and length of hospital stay in very low birth weight infants]. Med Wieku Rozwoj. (2005) 9:325–33.
81. Sevastiadou S, Malamitsi-Puchner A, Costalos C, Skouroliakou M, Briana DD, Antsaklis A, et al. The impact of oral glutamine supplementation on the intestinal permeability and incidence of necrotizing enterocolitis/septicemia in premature neonates. J Matern-Fetal Neonatal Med. (2011) 24:1294–300. doi: 10.3109/14767058.2011.564240
82. Houdijk AP, Rijnsburger ER, Jansen J, Wesdorp RI, Weiss JK, McCamish MA, et al. Randomised trial of glutamine-enriched enteral nutrition on infectious morbidity in patients with multiple trauma. Lancet (1998) 352:772–6.
83. Wischmeyer PE, Lynch J, Liedel J, Wolfson R, Riehm J, Gottlieb L, et al. Glutamine administration reduces Gram-negative bacteremia in severely burned patients: a prospective, randomized, double-blind trial versus isonitrogenous control. Crit Care Med. (2001) 29:2075–80. doi: 10.1097/00003246-200111000-00006
84. Lomivorotov VV, Efremov SM, Shmirev VA, Ponomarev DN, Lomivorotov VN, Karaskov AM. Glutamine is cardioprotective in patients with ischemic heart disease following cardiopulmonary bypass. Heart Surg Forum (2011) 14:E384–8. doi: 10.1532/HSF98.20111074
85. Lomivorotov VV, Efremov SM, Shmirev VA, Ponomarev DN, Svyatchenko AV, Deryagin MN, et al. Does glutamine promote benefits for patients with diabetes mellitus scheduled for cardiac surgery? Heart Lung Circ. (2013) 22:360–5. doi: 10.1016/j.hlc.2012.11.011
86. Brinkmann SJH, Buijs N, Vermeulen MAR, Oosterink E, Schierbeek H, Beishuizen A, et al. Perioperative glutamine supplementation restores disturbed renal arginine synthesis after open aortic surgery: a randomized controlled clinical trial. Am J Physiol Renal Physiol. (2016) 311:F567–75. doi: 10.1152/ajprenal.00340.2015
87. Chávez-Tostado M, Carrillo-Llamas F, Martínez-Gutiérrez PE, Alvarado-Ramírez A, López-Taylor JG, Vásquez-Jiménez JC, et al. Oral glutamine reduces myocardial damage after coronary revascularization under cardiopulmonary bypass. A randomized clinical trial Nutr Hosp. (2017) 34:277–83. doi: 10.20960/nh.519
88. Khogali SEO, Pringle SD, Weryk BV, Rennie MJ. Is glutamine beneficial in ischemic heart disease? Nutr Burbank Los Angel Cty Calif (2002) 18:123–6. doi: 10.1016/S0899-9007(01)00768-7
89. Roman-Blas JA, Castañeda S, Sánchez-Pernaute O, Largo R, Herrero-Beaumont G, CS/GS Combined Therapy Study Group. Combined treatment with chondroitin sulfate and glucosamine sulfate shows no superiority over placebo for reduction of joint pain and functional impairment in patients with knee osteoarthritis: a six-month multicenter, randomized, double-blind, placebo-controlled clinical trial. Arthritis Rheumatol. (2017) 69:77–85. doi: 10.1002/art.39819
90. Kwoh CK, Roemer FW, Hannon MJ, Moore CE, Jakicic JM, Guermazi A, et al. Effect of oral glucosamine on joint structure in individuals with chronic knee pain: a randomized, placebo-controlled clinical trial. Arthritis Rheumatol. (2014) 66:930–9. doi: 10.1002/art.38314
91. de Vos BC, Landsmeer MLA, van Middelkoop M, Oei EHG, Krul M, Bierma-Zeinstra SMA, et al. Long-term effects of a lifestyle intervention and oral glucosamine sulphate in primary care on incident knee OA in overweight women. Rheumatol Oxf Engl. (2017) 56:1326–34. doi: 10.1093/rheumatology/kex145
92. Esfandiari H, Pakravan M, Zakeri Z, Ziaie S, Pakravan P, Ownagh V. Effect of glucosamine on intraocular pressure: a randomized clinical trial. Eye Lond Engl. (2017) 31:389–94. doi: 10.1038/eye.2016.221
93. Runhaar J, Rozendaal RM, van Middelkoop M, Bijlsma HJW, Doherty M, Dziedzic KS, et al. Subgroup analyses of the effectiveness of oral glucosamine for knee and hip osteoarthritis: a systematic review and individual patient data meta-analysis from the OA trial bank. Ann Rheum Dis. (2017) 76:1862–9. doi: 10.1136/annrheumdis-2017-211149
94. Kongtharvonskul J, Anothaisintawee T, McEvoy M, Attia J, Woratanarat P, Thakkinstian A. Efficacy and safety of glucosamine, diacerein, and NSAIDs in osteoarthritis knee: a systematic review and network meta-analysis. Eur J Med Res. (2015) 20:24. doi: 10.1186/s40001-015-0115-7
95. Hresko RC, Heimberg H, Chi MM, Mueckler M. Glucosamine-induced insulin resistance in 3T3-L1 adipocytes is caused by depletion of intracellular ATP. J Biol Chem. (1998) 273:20658–68. doi: 10.1074/jbc.273.32.20658
96. Medina M. An overview on the clinical development of Tau-based therapeutics. Int J Mol Sci. (2018) 19:1160. doi: 10.3390/ijms19041160
97. Onay-Besikci A. Regulation of cardiac energy metabolism in newborn. Mol Cell Biochem. (2006) 287:1–11. doi: 10.1007/s11010-006-9123-9
98. Buse MG, Robinson KA, Marshall BA, Mueckler M. Differential effects of GLUT1 or GLUT4 overexpression on hexosamine biosynthesis by muscles of transgenic mice. J Biol Chem. (1996) 271:23197–202. doi: 10.1074/jbc.271.38.23197
99. Haubner BJ, Adamowicz-Brice M, Khadayate S, Tiefenthaler V, Metzler B, Aitman T, et al. Complete cardiac regeneration in a mouse model of myocardial infarction. Aging (2012) 4:966–77. doi: 10.18632/aging.100526
100. Porrello ER, Mahmoud AI, Simpson E, Hill JA, Richardson JA, Olson EN, et al. Transient regenerative potential of the neonatal mouse heart. Science (2011) 331:1078–80. doi: 10.1126/science.1200708
101. Uygur A, Lee RT. Mechanisms of cardiac regeneration. Dev Cell (2016) 36:362–74. doi: 10.1016/j.devcel.2016.01.018
102. Banerjee PS, Lagerlöf O, Hart GW. Roles of O-GlcNAc in chronic diseases of aging. Mol Aspects Med. (2016) 51:1–15. doi: 10.1016/j.mam.2016.05.005
103. Lefebvre T, Guinez C, Dehennaut V, Beseme-Dekeyser O, Morelle W, Michalski J-C. Does O-GlcNAc play a role in neurodegenerative diseases? Expert Rev Proteomics (2005) 2:265–75. doi: 10.1586/14789450.2.2.265
104. Fülöp N, Mason MM, Dutta K, Wang P, Davidoff AJ, Marchase RB, et al. Impact of Type 2 diabetes and aging on cardiomyocyte function and O-linked N-acetylglucosamine levels in the heart. Am J Physiol Cell Physiol. (2007) 292:C1370–8. doi: 10.1152/ajpcell.00422.2006
105. Fülöp N, Feng W, Xing D, He K, Not LG, Brocks CA, et al. Aging leads to increased levels of protein O-linked N-acetylglucosamine in heart, aorta, brain and skeletal muscle in Brown-Norway rats. Biogerontology (2008) 9:139. doi: 10.1007/s10522-007-9123-5
106. Liu Y, Li X, Yu Y, Shi J, Liang Z, Run X, et al. Developmental regulation of protein O-GlcNAcylation, O-GlcNAc transferase, and O-GlcNAcase in mammalian brain. PLoS ONE (2012) 7:e43724. doi: 10.1371/journal.pone.0043724
107. Rex-Mathes M, Werner S, Strutas D, Griffith LS, Viebahn C, Thelen K, et al. O-GlcNAc expression in developing and ageing mouse brain. Biochimie (2001) 83:583–90. doi: 10.1016/S0300-9084(01)01305-0
108. Gu J, Shi J, Dai C, Ge J, Zhao Y, Chen Y, et al. O-GlcNAcylation Reduces Ischemia-Reperfusion–Induced Brain Injury. Sci Rep. (2017) 7. doi: 10.1038/s41598-017-10635-0
109. Liu S, Sheng H, Yu Z, Paschen W, Yang W. O-linked β-N-acetylglucosamine modification of proteins is activated in post-ischemic brains of young but not aged mice: implications for impaired functional recovery from ischemic stress. J Cereb Blood Flow Metab. (2016) 36:393–8. doi: 10.1177/0271678X15608393
110. Wang AC, Jensen EH, Rexach JE, Vinters HV, Hsieh-Wilson LC. Loss of O-GlcNAc glycosylation in forebrain excitatory neurons induces neurodegeneration. Proc Natl Acad Sci USA. (2016) 113:15120–5. doi: 10.1073/pnas.1606899113
111. Heyland D, Muscedere J, Wischmeyer PE, Cook D, Jones G, Albert M, et al. A randomized trial of glutamine and antioxidants in critically ill patients. N Engl J Med. (2013) 368:1489–97. doi: 10.1056/NEJMoa1212722
112. Ward E, Picton S, Reid U, Thomas D, Gardener C, Smith M, et al. Oral glutamine in paediatric oncology patients: a dose finding study. Eur J Clin Nutr. (2003) 57:31–6. doi: 10.1038/sj.ejcn.1601517
113. Griffin ME, Jensen EH, Mason DE, Jenkins CL, Stone SE, Peters EC, et al. Comprehensive mapping of O-GlcNAc modification sites using a chemically cleavable tag. Mol Biosyst. (2016) 12:1756–9. doi: 10.1039/C6MB00138F
114. Deracinois B, Camoin L, Lambert M, Boyer J-B, Dupont E, Bastide B, et al. O-GlcNAcylation site mapping by (azide-alkyne) click chemistry and mass spectrometry following intensive fractionation of skeletal muscle cells proteins. J Proteomics (2018) 186:83–97. doi: 10.1016/j.jprot.2018.07.005
115. Wang Y, Zhu J, Zhang L. Discovery of cell-permeable O-GlcNAc transferase inhibitors via tethering in situ click chemistry. J Med Chem. (2017) 60:263–72. doi: 10.1021/acs.jmedchem.6b01237
116. Gross BJ, Kraybill BC, Walker S. Discovery of O-GlcNAc transferase inhibitors. J Am Chem Soc. (2005) 127:14588–9. doi: 10.1021/ja0555217
117. Lazarus MB, Nam Y, Jiang J, Sliz P, Walker S. Structure of human O-GlcNAc transferase and its complex with a peptide substrate. Nature (2011) 469:564–7. doi: 10.1038/nature09638
118. Ortiz-Meoz RF, Jiang J, Lazarus MB, Orman M, Janetzko J, Fan C, et al. A small molecule that inhibits OGT activity in cells. ACS Chem Biol. (2015) 10:1392–7. doi: 10.1021/acschembio.5b00004
119. Wang Z, Pandey A, Hart GW. Dynamic interplay between O-linked N-acetylglucosaminylation and glycogen synthase kinase-3-dependent phosphorylation. Mol Cell Proteomics (2007) 6:1365–79. doi: 10.1074/mcp.M600453-MCP200
Keywords: O-GlcNAc, cardiovascular, ischemia-reperfusion, pharmacology, therapy
Citation: Ferron M, Denis M, Persello A, Rathagirishnan R and Lauzier B (2019) Protein O-GlcNAcylation in Cardiac Pathologies: Past, Present, Future. Front. Endocrinol. 9:819. doi: 10.3389/fendo.2018.00819
Received: 16 July 2018; Accepted: 31 December 2018;
Published: 15 January 2019.
Edited by:
Tarik Issad, Institut National de la Santé et de la Recherche Médicale (INSERM), FranceReviewed by:
Miles J. De Blasio, Baker Heart and Diabetes Institute, AustraliaAdam R. Wende, University of Alabama at Birmingham, United States
Copyright © 2019 Ferron, Denis, Persello, Rathagirishnan and Lauzier. This is an open-access article distributed under the terms of the Creative Commons Attribution License (CC BY). The use, distribution or reproduction in other forums is permitted, provided the original author(s) and the copyright owner(s) are credited and that the original publication in this journal is cited, in accordance with accepted academic practice. No use, distribution or reproduction is permitted which does not comply with these terms.
*Correspondence: Marine Ferron, ZmVycm9ubWFyaW5lQGdtYWlsLmNvbQ==