- Poultry Production and Product Safety Research Unit, Agricultural Research Service, United States Department of Agriculture, Fayetteville, AR, United States
The study of host-microbe neuroendocrine crosstalk, termed microbial endocrinology, suggests the impact of diet on host health and microbial viability is, in part, reliant upon nutritional modulation of shared host-microbe neuroendocrine axes. In the 1990's it was first recognized that neuroendocrine pathways are major components of the microbiota-gut-brain axis, and that diet-induced changes in the gut microbiota were correlated with changes in host behavior and cognition. A causative link, however, between nutritional-induced shifts in microbiota composition and change in host behavior has yet to be fully elucidated. Substrates found in food which are utilized by bacteria in the production of microbial-derived neurochemicals, which are structurally identical to those made by the host, likely represent a microbial endocrinology-based route by which the microbiota causally influence the host and microbial community dynamics via diet. For example, food safety is strongly impacted by the microbial production of biogenic amines. While microbial-produced tyramine found in cheese can elicit hypertensive crises, microorganisms which are common inhabitants of the human intestinal tract can convert L-histidine found in common foodstuffs to histamine and thereby precipitate allergic reactions. Hence, there is substantial evidence suggesting a microbial endocrinology-based role by which the gastrointestinal microbiota can utilize host dietary components to produce neuroactive molecules that causally impact the host. Conversely, little is known regarding the reverse scenario whereby nutrition-mediated changes in host neuroendocrine production affect microbial viability, composition, and/or function. Mechanisms in the direction of brain-to-gut, such as how host production of catecholamines drives diverse changes in microbial growth and functionality within the gut, require greater examination considering well-known nutritional effects on host stress physiology. As dietary intake mediates changes in host stress, such as the effects of caffeine on the hypothalamic-pituitary-adrenal axis, it is likely that nutrition can impact host neuroendocrine production to affect the microbiota. Likewise, the plasticity of the microbiota to changes in host diet has been hypothesized to drive microbial regulation of host food preference via a host-microbe feedback loop. This review will focus on food as concerns microbial endocrinology with emphasis given to nutrition as a mediator of host-microbe bi-directional neuroendocrine crosstalk and its impact on microbial viability and host health.
Introduction
Food composition underscores diet-induced changes in host health and behavior as well as the viability, composition, and functionality of the microbiome (1). Although the importance of the macro- and micro-nutrient content of food in driving such changes independently in host and microbiome is widely-appreciated, significantly less is understood regarding how food-induced changes in the microbiome mediated via evolutionarily shared neurochemistry can causally impact the host and vice-versa (2). Microbial endocrinology, which is the evolutionary-based study of the neuroendocrine routes of bi-directional communication between host and microbiome, has been proposed as a testable framework within which mechanistic pathways of the microbiota-gut-brain axis can be identified (3). It is important to recognize that several species of the microbiota share many of the same enzymatic pathways used by the host in the production of neurotransmitters and hormones. Food can contain, and is independently metabolized by host and microbe into, neuroendocrine molecules many of which (i.e., acetylcholine, serotonin (5-hydroxytryptamine; 5-HT), gamma-amino butyric acid (GABA), histamine and others) are structurally identical regardless of host or microbial origin. As both host and microbe express many of the same receptors with which to recognize these molecules (4), the neuroendocrine axes constitute an evolutionary-based, bi-directional interkingdom language. Seminal experiments in 1992 were the first to demonstrate that microorganisms directly respond to neuroendocrine hormones (5). Since that time, microbial endocrinological-based mechanisms have been hypothesized to play a critical role by which the microbiome influences host food preference (6) and appetite (7). Indeed, microbes produce key food intake-regulatory hormones, such as somatostatin (8), as well as affect host ghrelin, leptin, insulin, glucagon-like peptide (GLP)-1, and other neuroendocrine molecules (9).
Although it is well-recognized that host diet causes alterations in the microbiome (10), little attention has been given to how food-induced changes in host physiology may affect the microbiome. Specifically, as the microbiota-gut-brain axis is bi-directional, microbial endocrinological-mechanisms involve gut-to-brain as well as brain-to-gut pathways. Food contains numerous non-nutritive substances that influence host physiology and behavior. For example, caffeine, a psychoactive found in coffee, tea, as well as some foods can elicit a response by host neuroendocrine stress pathways (11) known to interact with the microbiota. Multiple other feedback loops exist between food-induced changes in host and microbe. In addition to psychoactive substances, commonly consumed food and beverages have been known for decades to contain neurotransmitters of plant or microbial origin. Plant sources of animal feed, such as silage, contain histamine and food for human consumption, as an example, tea can contain GABA. Histamine in silage (12) is also detectable in cattle feces (13), which suggests microbiome exposure to neurotransmitters of diet origin. Further, this evolutionary-based crosstalk based on shared neurochemistry has also been observed in farm production animals where it has been shown to affect ruminant eating behavior (14). Likewise, in human volunteers, GABA intake (15) has been demonstrated to reduce fatigue (16) and psychological stress (17).
Such neurotransmitter crosstalk between host, food and microbe also represents a means by which food-induced changes in the microbiome can causally impact the host. Indeed, bacteria that inhabit the gastrointestinal tract (18) are capable of utilizing host dietary elements in the biosynthesis of neuroendocrine molecules, such as dopamine (19, 20). Human fecal isolates of several bacterial genera produce biogenic amines (21) which affect host health. For example, Morganella morganii, which produces histamine from amino acids and is found in the human gastrointestinal tract, is one of several bacterial taxa responsible for the production of histamine in spoiled fish, the consumption of which can cause anaphylactic shock and death (22). Hence, diet provides precursors useable by the microbiome in the de novo production of signaling molecules that can affect host neuroendocrine axes.
Many aspects of diet, including food composition, consumption patterns, and cultural habits therefore have the potential to affect host-microbe interaction via diverse neuroendocrine routes involving the microbiota-gut-brain axis (Box 1). Microbial endocrinology stands to provide a strong conceptual framework for the design of testable hypotheses in the pursuit of uncovering mechanisms by which diet and nutrition mediate changes in the host or microbiome along the shared evolutionary bridge of neuroendocrine communication.
BOX 1. Current knowledge and future research directions.
What is known?
• Diet contains a wide range of neuroendocrine molecules and their precursors (2, 23).
• Many of the neuroendocrine constituents in foods survive the digestive process and are absorbed in the upper gastrointestinal tract or reach the gut lumen (24, 25).
• Oral intake of neuroendocrine molecules has been reported to affect cognitive and emotional outcomes in volunteers and animals (16, 26, 27).
• The ability of nutrition to alter the microbiota and influence the microbiota-gut-brain axis and thereby influence memory and learning has been shown (28).
• Several members of the microbiota in a variety of host species have been demonstrated to respond to, uptake, and synthesize neuroendocrine molecules which are structurally-identical to those produced by the host (3).
• Certain products found in foods, such as caffeine, have direct effects on host stress neuroendocrine axes (29).
• Stress is well-recognized to influence eating behavior and food choice (30); and many foods, including chocolate (31), contain neuroactive components which can affect host emotional and cognitive state.
What is unknown?
• Does the neuroendocrine content of food affect compositional, and more importantly, functional changes in the microbiome? Would such changes be meaningful to the host? Could a diet be designed to specifically feed the microbiota with therapeutic implications for the host?
• Stress neuroendocrine axes are hubs of host-microbe crosstalk. How do foods that directly affect host stress physiology, such as caffeine and the hypothalamic-pituitary-adrenal (HPA)-axis, alter host-microbe interaction?
• How do legal requirements and cultural preferences in food processing (e.g., pasteurized vs. unpasteurized dairy products), as well as geographical considerations such as soil composition and crop cultivation, create population-specific exposure to neuroendocrine content in food?
• Does host-microbe neuroendocrine crosstalk influence host stress-induced feeding behavior, such as comfort eating, and disorders such as binge-eating?
• Although the ingestion of neuroendocrine-rich foods such as banana can increase postprandial plasma catecholamine concentrations, what, if any, are the implications of such changes on the host? Moreover, as catecholamines in the bloodstream can reach the gut, what, if any, are the implications on the microbiota and host-microbe interaction?
Overview of Review Structure
In examining the role of diet on the bi-directional neuroendocrine pathways that underscore host-microbe crosstalk via the microbiota-gut-brain axis, it is necessary to draw from diverse literatures such as food and agricultural sciences, microbiology, endocrinology, clinical psychiatry and neuroscience as well as others. This review will first address the presence of neurochemicals and relevant precursors in food and then progress to discussing how these can affect known neuroendocrine axes of host-microbe interaction. From there, food-induced changes in the microbiota-gut-brain axis will be examined from bottom-up (i.e., microbiota-to-gut-to-brain) and top-down (i.e., brain-to-gut-to-microbiota) perspectives (Figure 1).
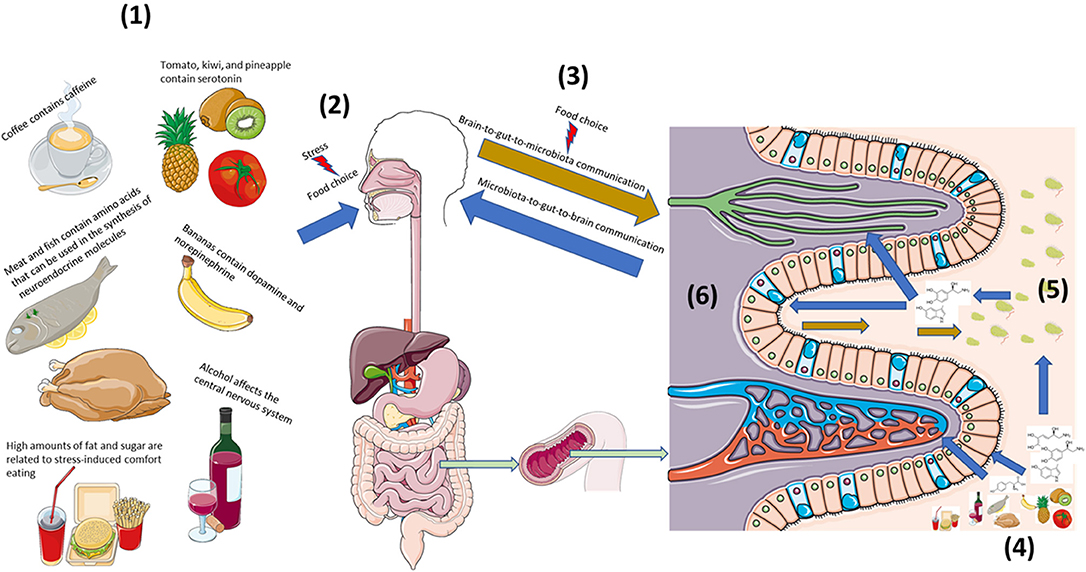
Figure 1. Diet strongly influences bi-directional neuroendocrine crosstalk along the microbiota-gut-brain axis. (1) Diet is both a source of neuroactive and neuroendocrine molecules as well precursors that can affect host neuroendocrine physiology (2). (2) Stress can influence food preference and consumption, (3) which can affect host-microbe bi-directional communication. (4) Neuroendocrine constituents and precursors found in foods act locally on host intestinal cells as well as the (5) microbiota to influence local interkingdom crosstalk. (6) Capillaries, lymphatic channels, as well as afferent and efferent nerves can extend into gut villi to provide multiple routes of bi-directional communication along the microbiota-gut-brain axis. This figure was made using templates adapted from Servier Medical Art by Servier. Original images are licensed under a Creative Commons Attribution 3.0 Unported License; https://smart.servier.com.
Neuroendocrine Molecules and Precursors Found in Food
As neuroendocrine pathways play significant and diverse roles in plant, mammalian, avian, aquatic life, insect, and reptilian physiologies, it should not come as a surprise that diet is a source of neuroendocrine molecules and their precursors (Table 1). It should be emphasized that this is not exclusive to human diets but includes many types of livestock animal feeds, as well as the diets of wildlife. This section will serve to give a brief overview of select neuroendocrine content of common food sources [for a comprehensive overview, the reader is directed to a recent review (23)] with associated outcomes related to their consumption. Due to the many types of digestive systems ranging from humans to wildlife to insects, it is not possible to generalize which neuroendocrine components and precursors survive digestion.
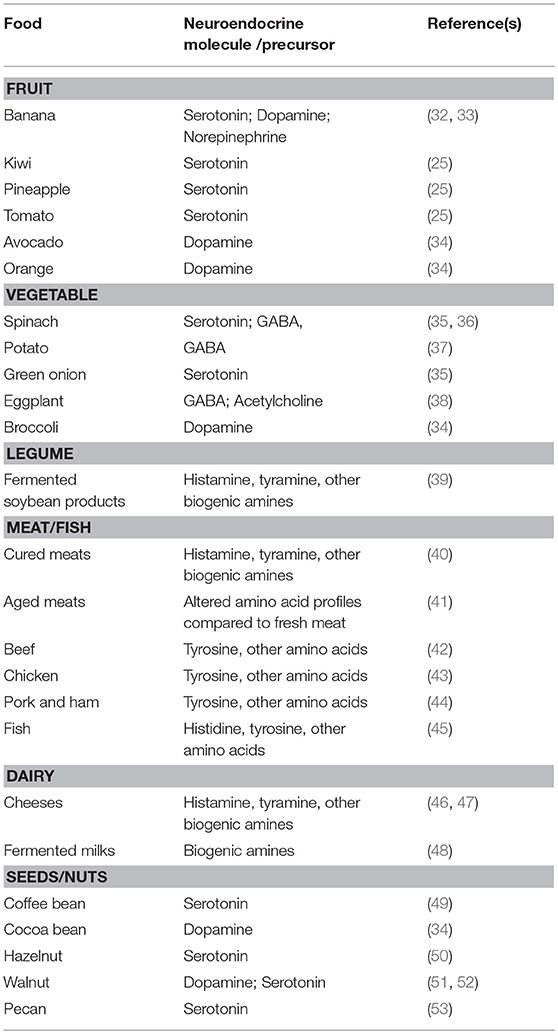
Table 1. A non-exhaustive summary of the neuroendocrine and neuroendocrine-precursor content of common foods.
Dietary amino acids tryptophan, tyrosine, and histidine to name a few, are precursors to the neurotransmitters serotonin, dopamine/norepinephrine/epinephrine, and histamine, res-pectively. A wide range of protein sources serve as rich sources of these amino acids including—but not limited to—fish, meat, legumes, nuts, and cheeses. Tryptophan, an essential amino acid in humans, is commonly employed in psychiatric-based clinical and preclinical studies to assess the relationship between serotonergic function and neuropsychiatric diseases such as depression. Acute tryptophan depletion in animals and humans, achieved through diet, has helped demonstrate the multifactorial relationship between serotonergic function and neuropsychiatric disease (54). Tyrosine, a non-essential amino acid in humans which can be obtained through diet, has been shown to enhance resilience to cognitive impairment during stress (55, 56), reduce environmental stress (57), as well as elevate anger under stressful conditions (58). Histidine, an essential amino acid in humans, is found in significant quantities in fermented products such as dried bonito flakes. Human consumption of dried bonito flakes has been associated with improved mood and reduced fatigue (59). Oral ingestion of histidine was demonstrated to improve working memory and attentiveness in healthy volunteers (60) and animals (61).
Like amino acids, neuroendocrine molecules and other precursors found in foods can survive the digestive process to reach the intestinal tract. Despite abundant evidence of neuroendocrine communication between host and microbe, surprisingly few microbiota-gut-brain axis-focused publications have examined the consequence of consuming neuroendocrine-rich foods. Indeed, the majority of investigations examining the fate and effect of dietary-derived neuroendocrine molecules have been published in the clinical and neuropsychiatric-based literature.
GABA is a constituent of several edible plants including those used in the preparation of tea (62). Investigations examining the effects of oral administration of GABA have shown GABA can be readily absorbed by the gastrointestinal tract, appearing in the plasma 1–1.5 h postprandial (24). Ex vivo and in vitro studies of animal (63) and human (64) intestinal tissues have identified potential GABA transport mechanisms. It is important to note that GABA receptors within the enteric nervous system are widely distributed throughout the gastrointestinal tract (65) and that GABA plays several physiological roles in a region-dependent manner along the gastrointestinal tract. Additionally, afferent ends of the vagal nerve, a pathway of gut-to-brain communication (66), extend into villi of the small intestine (67) and express GABA receptors (68, 69). Although GABA supplementation has been reported as effective in mediating favorable behavioral outcomes in animals and people, the mechanisms by which this occurs remain largely unknown as it is unclear whether, and under what conditions, GABA may cross the blood brain barrier (BBB) (70). Hence, it is unknown whether reports demonstrating that oral administration of GABA results in a reduction of fatigue in animals (27) and human volunteers (16), as well as antidepressant-like effects (26), improved task completion (71), and anti-insomnia effects (72) (73), are due to GABA acting locally on gastrointestinal receptors in a putative gut-to-brain mechanism, are due to circulating GABA, or if some GABA does indeed cross the BBB.
Serotonin is found in significant quantities in bananas, and a potential clinical role for serotonin obtained through banana consumption to treat constipation in human subjects was tested decades ago (33). Although it is controversial whether serotonin affects gastrointestinal motility and fecal transit (74), dietary-derived serotonin does reach the intestinal tract, is absorbed and undergoes hepatic metabolism into 5-hydroxyindoleacetic acid (5-HIAA) which can result in a postprandial elevation in plasma 5-HIAA concentration (75) and excretion in the urine (76). Other dietary sources of serotonin, such as nuts, pineapple, tomato, and kiwi have been shown to cause unique changes in human postprandial serum concentrations of 5-HIAA compared to banana (25).
Bananas also contain abundant amounts of dopamine and norepinephrine (32). Healthy volunteer plasma catecholamine concentrations were elevated following banana consumption (77). Likewise, urinary concentrations of homovanillic acid, a major metabolite of dopamine, increased after having eaten bananas (34). Banana, as well as other dietary sources of catecholamines such as pineapple and walnuts, were also reported to affect plasma concentrations in healthy volunteers of multiple catecholamine metabolites, including normetanephrine and 3-methoxytyramine which are breakdown products of norepinephrine and dopamine, respectively (78).
Although the Cavendish banana, a major commercial cultivar (79), has high concentrations of monoamines, a comparison of monoamine levels of other cultivars of banana consumed throughout world is needed. Cultural (i.e., governmental regulations and food safety laws) as well as geographic (i.e., soil, climate, and other conditions that affect, for example, crop growth) influences likely shape population-specific exposure, and therefore health and economic implications, to unique neuroendocrine profiles of common foods. For example, biogenic amine concentrations are typically lower in pasteurized compared to unpasteurized dairy products as pasteurization reduces microbial load, including bacteria that produce biogenic amines (46). The consumption of dietary-derived biogenic amines can have direct effects on host physiology. For example, consumption of tyramine-rich (tyramine etymology stems from the words tyrosine and amine, “tyros” is Greek for “cheese”) foods is contraindicated for patients prescribed monoamine oxidase inhibitors (MAO-I) in the treatment of psychiatric illness (80). Typically, dietary tyramine, which survives stomach acid, is metabolized within the intestine by monoamine oxidase (MAO). The use of MAO-I inhibits MAO which allows excess tyramine to enter circulation. Once present in the circulatory system it can then be converted to the catecholamine norepinephrine, thereby resulting in the precipitation of a hypertensive crisis.
Likewise, the geographical origin of food sources is well recognized to affect the chemical compositions of livestock feed ingredients (81, 82) which in turn can impact digestibility (83). Biogenic amines in animal feeds appear to have species-specific effects and are not strictly deleterious or beneficial (84). For example, poultry broilers fed diets that included high concentrations of putrescine, cadaverine, histamine, and/or phenylethylamine did not exhibit reduced performance or display histopathologic alterations (85). This literature review did not reveal any study that profiled the neuroendocrine content of different feeds sourced either domestically or internationally.
Interaction of Neuroendocrine Axes and Food Intake—a Microbial Endocrinological Perspective
Meal timing and feeding behavior are intimately linked to neuroendocrine physiology. The sensitivity of circadian cellular and physiological functions to chrono-patterns of feed intake has been recognized for decades (86). However, such findings largely have yet to integrate recent discoveries of bacterial regulation of feeding behavior and influence on metabolically-important host neuroendocrine profiles. For example, ghrelin, an orexigenic hormone (87) that influences central dopamine (88) and serotonin (89), is increased in rodent plasma before scheduled meal times and changes in serum ghrelin concentrations have been correlated with higher or lower counts of certain fecal bacterial taxa (90). Likewise, germ-free mice exhibit reduced hypothalamic ghrelin concentrations which are normalized following colonization with a conventional microbiota (91). Nevertheless, ghrelin knockout rodents exhibit normal appetite and feed intake (92), suggesting compensatory mechanisms in driving food consumption. As ghrelin's orexigenic effects are mediated through dopaminergic signaling (93), and dopamine has been suggested to influence appetite (94), microbial utilization of the dopamine precursor L-dopa (95) may suggest a microbial endocrinological route of host appetite modulation. Hence, in a study that utilized human subjects, reduced appetite reported by Helicobacter pylori-infected patients (96) may be linked not only to lowered ghrelin via H. pylori effects on gastric endocrine cells, but to H. pylori utilization of L-dopa and the resulting impact on central dopaminergic function.
Other chronobiological pathways that regulate feeding behavior also are likely to include bi-directional microbial endocrinological-based mechanisms. Changes in leptin, an anorexigenic hormone, are subject to both circadian rhythm and patterns of feed intake. Although leptin is derived from gastric and adipose origin (97), gastric leptin is secreted into both the gastric juice as well as into the bloodstream (98). In addition to gastric leptin appearing in circulation, gastric leptin also reaches the intestinal lumen (99). As intestinal epithelial cells express leptin receptors on both the basolateral membrane and on the apical microvilli, gastric leptin exerts diverse effects on the intestinal epithelium (100). Little is known, however, regarding how leptin affects the gastrointestinal microbiome. Interestingly, it has been reported that, compared to conventional mice, germ-free mice exhibit greater leptin sensitivity within the central nervous system (CNS) (101). Although the authors did not speculate on a microbial-based mechanism being responsible for the relative leptin resistance observed in conventional mice, clues may still be gleaned from other studies. For example, leptin knockout mice display both hyperphagia and alterations in gastrointestinal microbial diversity (102). Conversely, knockout of murine intestinal epithelial leptin receptor, which was identified in the submucosa of each region of the small intestine, did not cause compositional changes in the fecal microbiome (103).
Thus, a key question not addressed in the literature, is whether leptin itself has any direct effects on the microbiota (i.e., do bacteria sense and uptake extracellular leptin?), especially given that gastric leptin reaches the gut lumen and interacts with the intestinal epithelium. Relatedly, ob/ob mice, which are obese due to hyperphagia from being leptin-deficient, exhibit increased intestinal bacterial translocation possibly related to dysregulated glucose metabolism (104) compared to conventional mice (105). When these ob/ob mice were administered leptin, intestinal mucosal bacterial adherence and translocation were reduced. Hence, leptin signaling may be a source of host-microbe neuroendocrine bidirectional communication. As the evidence indicates that leptin affects bacterial adherence and translocation in the intestine, it can therefore be suggested that leptin serves to eliminate unwanted microbial colonization of the gastrointestinal tract. If a direct inhibitory effect of leptin on certain members of the microbiota is found, perhaps bacterial tolerance to leptin may represent an evolutionary niche by which certain species are able to colonize different regions of the upper gastrointestinal tract, such as the jejunum (106). Indeed, it would be interesting to investigate whether leptin signaling, via a microbial endocrinological-based mechanism, unites the separate observations that obesity is associated with leptin resistance (107) as well as the risk of small intestinal bacterial overgrowth (108).
Whether host-microbe dialogue via neuroendocrine stress pathways mediates the effects of stress on dietary consumption patterns is a particularly relevant, yet little understood, intersection of feeding behavior and the microbiota-gut-brain axis. For example, stress is recognized to affect eating behavior (109), and while significant attention has been given to associations of the microbiota and changes in feeding behavior (i.e., “correlation”), very little is understood in the way of causation (110). The first evidence that neurohormones constitute an interkingdom language between prokaryote and eukaryote was reported in the early 1990s (5, 111, 112). Microbial endocrinological-based investigations have since revealed the mechanisms by which several stress-related neurohormones, including serotonin and norepinephrine allow for bi-directional communication between host and microbe. While the causation underlying stress-induced eating disorders is multifactorial, the involvement of central catecholaminergic pathways in feeding behavior has been recognized for decades (113). Likewise, vagotomy [i.e., severing of the vagus nerve, a major bi-directional route of microbiota-gut-brain communication (114)] has been reported to associate with decreased hunger, gustatory intensity, and related sensations (115). Rodent studies have demonstrated the microbiota to significantly alter host catecholaminergic systems (116) and, for example, via the vagus nerve, affect brain function (117) as well as anxiety- (118) and depressive-like behaviors (119). It is important to note that several types of vagal afferents are distributed throughout the mucosa of different regions of the gastrointestinal tract (120), and that the receptors found on vagal afferents are not homogenously distributed; hence, functionality of vagal afferents cannot be generalized across different gastrointestinal regions (121). Nevertheless, some vagal afferents lie in close proximity to enteroendocrine cells (EEC) (122). As the apical surface of EEC can reach the gut lumen, EEC luminal sensing of microbial metabolites and dietary components allows for paracrine activation of receptors found on vagal afferents (123). Indeed, diet-induced changes in the microbiota were recently associated with alterations in the vagal afferent innervation of the rodent cecum (124). EECs express several types of receptors, including adreno- and serotonin-receptors. Application of norepinephrine to murine colonic intestinal epithelium ex-vivo was shown to elicit sensory neuronal response via an EEC-based mechanism (125). Likewise, in rodents, in response to luminal stimuli, serotonin released from intestinal EEC was demonstrated to activate the vagal afferent 5-HT3 receptor (126). Interestingly, the proximal colon contains vagal afferent endings which are mechanosensitive (127), and proximal colon distension activated catecholaminergic neurons in the brainstem (128). Since the brainstem plays a critical role in receiving gut-derived signals in order to regulate food intake (129), it is therefore reasonable to suggest that host-microbe neuroendocrine signaling within the gastrointestinal tract may signal via the vagus nerve to affect feeding-regulatory regions of the CNS.
In addition to vagal afferent innervation, the intestinal tract is innervated by vagal efferent nerves (130, 131). This is an important consideration as it allows the host to rapidly respond to signals, including those that are food-related, originating from the gut via vagal afferent stimulation. One such vagal afferent/efferent circuit is the cholinergic anti-inflammatory pathway (CAP) (132). Indeed, the effect of a high fat diet in reducing pro-inflammatory cytokine response in rats that underwent hemorrhagic shock was shown to be mediated via the CAP (133). Hence, enteric nutrition may hold therapeutic potential in mediating inflammatory response to trauma via a gut-to-brain vagal mechanism. It is important to note that it is unknown how CAP activation may differ depending on intake of different types of dietary fat, or whether other dietary-acquired constituents such as 5-HT may act on vagal afferent receptors in gut villi to initiate CAP.
Microbial Influences on Host Feeding Behaviors: From Microbiota-to-Gut-to-Brain
“I'm cuckoo for Cocoa Puffs!®”—Sonny the Cuckoo Bird®
Although it may never be known whether microbes influence Sonny's® predilection for Cocoa Puffs®, several lines of evidence suggest the microbiome can interact with host feeding-related physiology, food preference, as well as affect food-seeking behavior and cognition. Indeed, recent studies have described a role for the microbiome in influencing host intake and chemo-sensing systems of carbohydrate (134), fat (135), and protein (136). The first evidence linking diet-induced changes in the microbiome to alterations in host behavior and cognition was reported in 2009 (28). Compared to mice maintained on a standard chow diet, mice fed a meat-based diet exhibited both significantly greater fecal microbial diversity which was positively correlated with improvements in working and reference memories, as well as comparatively less anxiety-like behavior and a slower speed in seeking food. Little is understood, however, of how such diet-induced shifts in the microbiota mechanistically affect the host. From within the gastrointestinal tract, microbes can utilize host dietary components in the de novo synthesis of neuroendocrine molecules and precursors that may affect host health and hedonistic behaviors via microbial endocrinological-pathways of the gut-brain axis (2).
EEC-mediated mechanisms represent one such pathway by which the microbiota can potentially affect the host through diet via microbial endocrinological-based signaling. EEC express a repertoire of luminal metabolite- and nutrient-sampling receptors. Such receptors include sweet-taste receptors which bind caloric sugars and non-nutritive sweeteners (137), bitter-taste receptors, free-fatty acid receptors (FFARs) which bind dietary fatty acids as well as microbial-generated short-chain fatty acids (138), and amino acid receptors (139). Compared to conventional rodents, germ-free rodents displayed greater preference for drinking solutions that contained higher concentrations of sucrose (134). Dietary sugars, such as sucrose in drinking water, are absorbed in the upper small intestine, including the duodenum and jejunum (140). Duodenal EEC-induced release of serotonin in response to maltose, a disaccharide of glucose, or glucose has also been shown to elicit duodenal vagal afferent firing (126). Relatedly, the microbiome composition was observed to diverge between mouse lines selectively bred for low or high saccharin taste phenotype (6). Low or high saccharin preference in rodents has been reported to be associated with social behavior as well as reactivity to stress (141). As the vagus nerve is important in mediating some probiotic anxiolytic effects on the host (3), it may be hypothesized that any microbial influence on host preference for the type and quantity of sugar intake could drive behavioral effects via an EEC-vagus-mediated mechanism of the microbiota-gut-brain axis.
Also found in the distal ileum and the large intestine, EEC can be exposed to products of bacterial metabolism of metabolic host dietary constituents that escaped absorption in the small intestine. Non-digestible carbohydrates, such as resistant starch, can be fermented by the microbiota into short chain fatty acids (SCFAs). Butyrate, one such microbial SCFA, can act on human EEC FFARs to stimulate the release of peptide YY (PYY) (142). In rodents, PYY, a satiety signal, has been shown to bind to the Y2 receptor on neighboring gut vagal afferents to activate hypothalamic neurons that help regulate food intake (143). Likewise, butyrate can influence host catecholamine synthesis (144) and, at certain concentrations, elicits activation of the hypothalamic-pituitary-adrenal (HPA)-axis, a classical neuroendocrine pathway of the stress response (145). As nerve terminals that ramify into the intestinal mucosa synthesize dopamine and norepinephrine (146), butyrate may act locally in the gut to alter luminal catecholamine production. As HPA-axis activation is associated with anxiety, it is important to note that mice fed ad-libitum high amylose type-2 resistant starch exhibited changes in the microbiota that associated with host anxiety-like behavior (147). Colonic epithelial cells (148) as well as endothelial cells of the BBB (149) both express the SCFA transporter monocarboxylate transporter (MCT)-1. Hence, butyrate and acetate may be transported from the intestinal lumen into the circulation to cross the BBB and thereby affect brain and behavior. For example, radio-labeled acetate absorbed from the colon can cross the BBB and suppress appetite in rodents (150). Multiple routes may therefore enable microbial SCFAs, derived from host dietary components, to potentially influence the host along neuroendocrine pathways of the microbiota-gut-brain axis.
The microbiota also affects the amino acid profiles of the gastrointestinal tract (151) and circulation. For example, intestinal microbial-produced free lysine and threonine has been detected in human plasma (152). Lysine was recently shown to play an important function in avian neuroendocrine regulation of food intake, however, a role for the microbiota was not investigated (153). Amino acids are precursors to several microbial-derived neuroendocrine molecules, including the biogenic amines agmatine, derived from lysine; cadaverine, derived from arginine; and putrescine, derived from ornithine (154). Each of these biogenic amines have been demonstrated to be related to diet. For example, the oral administration of agmatine in rats was reported to affect host metabolism accompanied by reductions in diet-associated weight gain (155). Likewise, mice fed a high protein diet had elevated cadaverine in colonic content compared to mice on a moderate protein intake (156). Finally, hypertensive obese patients fed a hypocaloric diet with cheese containing Lactobacillus plantarum, a probiotic which synthesizes putrescine, exhibited reduced body mass index (BMI) and arterial blood pressure compared to patients fed cheese without the probiotic (157).
The microbiota can also utilize host dietary amino acids in the synthesis of xenobiotic compounds, such as indoles, which affect host health and behavior (158). Microbial-produced indoles, which are derived from tryptophan, can affect host anxiety-like behavior (159). Nevertheless, what may be gleaned from these studies is that much remains to be understood regarding not only the extent of microbial production of the amino acid-derived neuroendocrine molecules in the gastrointestinal tract in vivo, but whether the in vivo production, and effect on the host, of these products mirrors what has been reported from their oral ingestion.
As mentioned earlier in this review, bananas contain significant concentrations of free catecholamines, which following banana consumption, appear as sulfate-conjugated catecholamines in the plasma of healthy volunteers (77). In this study, the authors suggested that “sulfate conjugation of free catecholamines in banana largely takes place in the gut.” Specifically, conjugation of free catecholamines takes place predominantly in the mesentery (160). The site of conjugation is important as microbial β-glucuronidase in the gut lumen was recently discovered as necessary for the deconjugation of sulfate- and glucuronide-conjugated catecholamines (116) as well as serotonin (161). Hence, within the gut lumen the microbiota plays a critical role in the conversion of biologically-inactive, conjugated monoamines to biologically-free, active monoamines. As the microbiota responds to free catecholamines with significant implications for host health (2) and, for example, uptake extracellular serotonin (4), it will be critically important to understand how the ingestion of neurochemical-containing food affects the microbiota to causally-impact the host. Moreover, as the microbiota can convert dietary amino acids into biologically-active monoamines in simulated in vivo conditions (20), it will be essential to determine how host food intake and dietary habits fuel microbial production of monoamines that can then affect neuroendocrine host-microbe crosstalk along the microbiota-gut-brain axis.
Host Feeding Behavior Influences the Microbiota: From Brain-to-Gut-to-Microbiota
“They're Gr-r-r-reat!”—Tony the Tiger®
Tony the Tiger's® subjective opinion of Frosted Flakes® might or might not be shared with his microbiota. Indeed, food selection is well-recognized to be influenced by conditions of psychological and physical stress. Comfort eating, food choice which lessens the feelings of stress (30), is engaged in by both sexes as well as animals and has been shown to affect physiological response (162–164) and emotional perception (165) of stress. In light of recent findings in rodents that stress and sex uniquely interact to influence the microbiota-gut-brain axis (166), and that the microbiota can affect host food choice, it is important to note that the effect of comfort eating in reducing stress in female rodents is estrous-cycle dependent (167). Likewise, chemical constituents of foods, such as caffeine, can elicit HPA-axis activation. As food therefore can affect host neuroendocrine systems, this gives rise to altered brain-to-gut signaling which could affect the microbiota.
How foods influence host neuroendocrine physiology is multifactorial. Chocolate is a principal example (168) as it contains the psychoactive substances threonine and caffeine, a combination of fat and sugar, a subjectively-appealing aroma, in addition to other factors. Recent studies, however, have highlighted a role for the fat and sugar, perhaps the latter more so than the former, content of chocolate in being the main drivers of chocolate's psychoactive effects (31). Foods that contain high sugar and fat were shown to alter rodent stress-induced eating behavior (169). The effects of comfort eating on the HPA-axis are well-described, with glucocorticoids playing a major role in increasing the intake of palatable food (170). Glucocorticoids, including corticosterone, are synthesized by intestinal epithelial cells and released into the gut lumen (171). For example, fecal corticosterone can be measured in rodents (172), canines (173), and wildlife (174) as a non-invasive indicator of environmental stress. As glucocorticoids are released into the gut lumen, it is reasonable to assume the microbiota are exposed to host-produced glucocorticoids. An effect on microbial viability, growth, or function following exposure to corticosterone or other major host-produced glucocorticoids is unknown. Gnotobiotic animals, such as the defined 8-species microbiota of the Altered Schaedler Flora (ASF) rodent, may allow for interrogation of how host glucocorticoids or neuroendocrine molecules secreted into the gut affect the microbiota in a brain-to-gut-to-microbiota mechanism (175). Hence, deliberate host food choice under times of stress to reduce the host's own stress response may causally impact the microbiota.
Caffeine, a psychoactive substance found in coffee, tea, and other beverages and foods, is consumed in virtually all societies (176). Although health regulatory bodies, such as the European Food Safety Authority have set recommended daily caffeine intake limits for children and adults as 3 mg/kg and 400 mg (total), respectively, animal (11) and human studies (177, 178) have demonstrated caffeine as anxiogenic. Indeed, a single caffeine dose of 250 mg in healthy adult volunteers caused an elevation in anxiety and diastolic blood pressure (29). That low doses of caffeine alter the host HPA-axis is notable for several reasons. First, HPA-axis responsivity is part of the bi-directional microbiota-gut-brain axis (179). HPA-axis activation stimulates the production of the stress catecholamines norepinephrine and epinephrine that effect unique and direct responses in the microbiome (180, 181). As norepinephrine and epinephrine are found at several host-microbiome interfaces, including in the gut lumen (116, 125) and lung alveolar fluid (182), it is reasonable to suggest that changes in the host HPA-axis and its responsiveness following food or beverage consumption likely affect the microbiome.
Relatedly, in healthy subjects, consumption of a triple espresso or 250 mg of caffeine has been shown to stimulate sympathetic nervous system (SNS) activity (183). SNS activation can cause the release of catecholamines from the adrenal medulla, which feed back onto the HPA-axis, sympathetic nerve fibers also ramify throughout the intestinal tract. As sympathetic nerve terminals express tyrosine hydroxylase and synthesize norepinephrine, that they can be found in the intestinal mucosa (184) provides yet another route of neuroendocrine host-microbe crosstalk. Indeed, norepinephrine from sympathetic efferent nerve terminals throughout the body can escape breakdown to appear in the gut (185) as well as plasma (186). It is currently unknown whether the intake of caffeine or other substances in foods that activate the SNS may influence the microbiome.
Like caffeine, alcohol consumption is a virtually global occurrence (187). Alcohol affects the CNS as well as causes physical changes in several areas along the gastrointestinal tract (188). Protracted alcohol abuse can cause dramatic changes in CNS neurochemistry and brain structure (189). It is largely unknown how alcohol's direct degenerative effects on the CNS may bi-directionally impact the microbiota-gut-brain axis. Nevertheless, alterations in neuronal architecture, one may assume, would contribute to changes in the microbiota-gut-brain axis in the direction of the brain-to-gut-to-microbiota. This direction of the microbiota-gut-brain axis should be considered in the context of alcohol use, as alcohol can be absorbed in the stomach and upper small intestine (190), therefore not all alcohol ingested may reach the intestinal tract and microbiota. It should be noted that substantial attention has been given to alterations in the gut microbiota following mild as well as chronic alcohol consumption, and hypotheses have been proposed describing alcohol's influence on the microbiota-gut-brain axis in the direction of the microbiota-to-gut-to-brain (191, 192).
Conclusions and Future Directions
The relationships between diet, nutrition, and the microbiota-gut-brain axis are incredibly complex, yet mechanistic pathways which causally unite these categories remain to be fully elucidated. We are truly at the beginning of our understanding of these complex inter-relationships. The neuroendocrine system is a major bi-directional set of pathways along the microbiota-gut-brain axis that is both affected by food choice and at the same time is a locus of host-microbe crosstalk. Microbial endocrinology, the study of neuroendocrine host-microbe interkingdom communication, represents an important lens, and certainly not the only one, through which diet, nutrition, and the microbiota-gut-brain axis may be mechanistically linked. Indeed, foods and beverages contain diverse neuroendocrine molecules and precursors that separately affect host physiology as well as microbial viability and function. Moreover, microbial utilization of host dietary components can result in the production of neuroendocrine signaling molecules that can affect host health, especially considering that several microbe-derived molecules are structurally identical to those produced by the host. Likewise, diet-induced changes in host neuroendocrine physiology likely have direct consequences for the microbiota. Microbial endocrinological-based mechanisms are a promising avenue by which future nutrition-oriented microbiota-gut-brain axis research may identify causal, mechanistic-driven pathways of how diet affects the bi-directional nature of host-microbiota interaction that ultimately may define host health and behavior.
Author Contributions
The author confirms being the sole contributor of this work and has approved it for publication.
Conflict of Interest Statement
The author declares that the research was conducted in the absence of any commercial or financial relationships that could be construed as a potential conflict of interest.
Acknowledgments
The author gratefully acknowledges the assistance of Professor Mark Lyte, Iowa State University (Ames, Iowa, United States), in the editing of the manuscript.
References
1. Flint HJ, Duncan SH, Louis P. The impact of nutrition on intestinal bacterial communities. Curr Opin Microbiol. (2017) 38:59–65. doi: 10.1016/j.mib.2017.04.005
2. Lyte M. Microbial endocrinology and nutrition: a perspective on new mechanisms by which diet can influence gut-to-brain communication. PharmaNutrition (2013) 1:35–9. doi: 10.1016/j.phanu.2012.11.002
3. Lyte M. Probiotics function mechanistically as delivery vehicles for neuroactive compounds: microbial endocrinology in the design and use of probiotics. Bioessays (2011) 33:574–81. doi: 10.1002/bies.201100024
4. Lyte M, Brown DR. Evidence for PMAT- and OCT-like biogenic amine transporters in a probiotic strain of Lactobacillus: implications for interkingdom communication within the microbiota-gut-brain axis. PLoS ONE (2018) 13:e0191037. doi: 10.1371/journal.pone.0191037
5. Lyte M, Ernst S. Catecholamine induced growth of gram negative bacteria. Life Sci. (1992) 50:203–12. doi: 10.1016/0024-3205(92)90273-R
6. Lyte M, Fodor AA, Chapman CD, Martin GG, Perez-Chanona E, Jobin C, et al. Gut microbiota and a selectively bred taste phenotype: a novel model of microbiome-behavior relationships. Psychosom Med. (2016) 78:610–9. doi: 10.1097/PSY.0000000000000318
7. Norris V, Molina F, Gewirtz AT. Hypothesis: bacteria control host appetites. J Bacteriol. (2013) 195:411–6. doi: 10.1128/JB.01384-12
8. Leroith D, Pickens W, Vinik AI, Shiloach J. Bacillus subtilis contains multiple forms of somatostatin-like material. Biochem Biophys Res Commun. (1985) 127:713–9. doi: 10.1016/S0006-291X(85)80001-2
9. Neuman H, Debelius JW, Knight R, Koren O. Microbial endocrinology: the interplay between the microbiota and the endocrine system. FEMS Microbiol Rev. (2015) 39:509–21. doi: 10.1093/femsre/fuu010
10. Singh RK, Chang HW, Yan D, Lee KM, Ucmak D, Wong K, et al. Influence of diet on the gut microbiome and implications for human health. J Transl Med. (2017) 15:73. doi: 10.1186/s12967-017-1175-y
11. Patz MD, Day HE, Burow A, Campeau S. Modulation of the hypothalamo-pituitary-adrenocortical axis by caffeine. Psychoneuroendocrinology (2006) 31:493–500. doi: 10.1016/j.psyneuen.2005.11.008
12. Scherer R, Gerlach K, Südekum KH. Biogenic amines and gamma-amino butyric acid in silages: formation, occurrence and influence on dry matter intake and ruminant production. Anim Feed Sci Technol. (2015) 210:1–16. doi: 10.1016/j.anifeedsci.2015.10.001
13. Wrenn TR, Bitman J, Cecil HC, Mench ML. Histamine excretion of cattle fed various rations. J Dairy Sci. (1964) 47:882–4. doi: 10.3168/jds.S0022-0302(64)88795-6
14. Neumark H, Bondi A, Volcani R. Amines, aldehydes and keto-acids in silages and their effect on food intake by ruminants. J Sci Food Agr. (1964) 15:487–92. doi: 10.1002/jsfa.2740150709
15. Liao J, Wu X, Xing Z, Li Q, Duan Y, Fang W, et al. Gamma-Aminobutyric Acid (GABA) accumulation in tea (Camellia sinensis L.) through the GABA shunt and polyamine degradation pathways under Anoxia. J Agric Food Chem. (2017) 65:3013–8. doi: 10.1021/acs.jafc.7b00304
16. Kanehira T, Nakamura Y, Nakamura K, Horie K, Horie N, Furugori K, et al. Relieving occupational fatigue by consumption of a beverage containing gamma-amino butyric acid. J Nutr Sci Vitaminol. (2011) 57:9–15. doi: 10.3177/jnsv.57.9
17. Nakamura H, Takishima T, Kometani T, Yokogoshi H. Psychological stress-reducing effect of chocolate enriched with gamma-aminobutyric acid (GABA) in humans: assessment of stress using heart rate variability and salivary chromogranin A. Int J Food Sci Nutr. (2009) 60(Suppl. 5):106–13. doi: 10.1080/09637480802558508
18. Sender R, Fuchs S, Milo R. Revised estimates for the number of human and bacteria cells in the body. PLoS Biol. (2016) 14:e1002533. doi: 10.1371/journal.pbio.1002533
19. Villageliu D, Lyte M. Dopamine production in Enterococcus faecium: a microbial endocrinology-based mechanism by which probiotics may influence host physiology. PLoS ONE (2018) 13:e0207038. doi: 10.1371/journal.pone.0207038
20. Villageliu DN, Rasmussen S, Lyte M. A microbial endocrinology-based simulated small intestinal medium for the evaluation of neurochemical production by gut microbiota. FEMS Microbiol Ecol. (2018) 94:7. doi: 10.1093/femsec/fiy096
21. Pugin B, Barcik W, Westermann P, Heider A, Wawrzyniak M, Hellings P, et al. A wide diversity of bacteria from the human gut produces and degrades biogenic amines. Microb Ecol Health Dis. (2017) 28:1353881. doi: 10.1080/16512235.2017.1353881
22. Tortorella V, Masciari P, Pezzi M, Mola A, Tiburzi SP, Zinzi MC, et al. Histamine poisoning from ingestion of fish or scombroid syndrome. Case Rep Emerg Med. (2014) 2014:482531. doi: 10.1155/2014/482531
23. Briguglio M, Dell'osso B, Panzica G, Malgaroli A, Banfi G, Zanaboni Dina C, et al. Dietary neurotransmitters: a narrative review on current knowledge. Nutrients (2018) 10:E591. doi: 10.3390/nu10050591
24. Li J, Zhang Z, Liu X, Wang Y, Mao F, Mao J, et al. Study of GABA in healthy volunteers: pharmacokinetics and pharmacodynamics. Front Pharmacol. (2015) 6:260. doi: 10.3389/fphar.2015.00260
25. Tohmola N, Johansson A, Sane T, Renkonen R, Hamalainen E, Itkonen O. Transient elevation of serum 5-HIAA by dietary serotonin and distribution of 5-HIAA in serum protein fractions. Ann Clin Biochem. (2015) 52:428–33. doi: 10.1177/0004563214554842
26. Chuang CY, Shi YC, You HP, Lo YH, Pan TM. Antidepressant effect of GABA-rich monascus-fermented product on forced swimming rat model. J Agric Food Chem. (2011) 59:3027–34. doi: 10.1021/jf104239m
27. Chen H, He X, Liu Y, Li J, He Q, Zhang C, et al. Extraction, purification and anti-fatigue activity of gamma-aminobutyric acid from mulberry (Morus alba L.) leaves. Sci Rep. (2016) 6:18933. doi: 10.1038/srep18933
28. Li W, Dowd SE, Scurlock B, Acosta-Martinez V, Lyte M. Memory and learning behavior in mice is temporally associated with diet-induced alterations in gut bacteria. Physiol Behav. (2009) 96:557–67. doi: 10.1016/j.physbeh.2008.12.004
29. Smith JE, Lawrence AD, Diukova A, Wise RG, Rogers PJ. Storm in a coffee cup: caffeine modifies brain activation to social signals of threat. Soc Cogn Affect Neurosci. (2012) 7:831–40. doi: 10.1093/scan/nsr058
30. Gibson EL. Emotional influences on food choice: sensory, physiological and psychological pathways. Physiol Behav. (2006) 89:53–61. doi: 10.1016/j.physbeh.2006.01.024
31. Nasser JA, Bradley LE, Leitzsch JB, Chohan O, Fasulo K, Haller J, et al. Psychoactive effects of tasting chocolate and desire for more chocolate. Physiol Behav. (2011) 104:117–21. doi: 10.1016/j.physbeh.2011.04.040
32. Waalkes TP, Sjoerdsma A, Creveling CR, Weissbach H, Udenfriend S. Serotonin, norepinephrine, and related compounds in bananas. Science (1958) 127:648–50. doi: 10.1126/science.127.3299.648
33. Connell AM, Rowlands EN, Wilcox PB. Serotonin, bananas, and diarrhoea. Gut (1960) 1:44–7. doi: 10.1136/gut.1.1.44
34. Feldman JM, Lee EM, Castleberry CA. Catecholamine and serotonin content of foods: effect on urinary excretion of homovanillic and 5-hydroxyindoleacetic acid. J Am Diet Assoc. (1987) 87:1031–5.
35. Ly D, Kang K, Choi JY, Ishihara A, Back K, Lee SG. HPLC analysis of serotonin, tryptamine, tyramine, and the hydroxycinnamic acid amides of serotonin and tyramine in food vegetables. J Med Food (2008) 11:385–9. doi: 10.1089/jmf.2007.514
36. Yoon YE, Kuppusamy S, Cho KM, Kim PJ, Kwack YB, Lee YB. Influence of cold stress on contents of soluble sugars, vitamin C and free amino acids including gamma-aminobutyric acid (GABA) in spinach (Spinacia oleracea). Food Chem. (2017) 215:185–92. doi: 10.1016/j.foodchem.2016.07.167
37. Steward FC, Thompson JF, Dent CE. γ-Aminobutyric acid: a constituent of potato tubers? Science (1949) 110:439–40.
38. Yamaguchi S, Matsumoto K, Koyama M, Tian S, Watanabe M, Takahashi A, et al. Antihypertensive effects of orally administered eggplant (Solanum melongena) rich in acetylcholine on spontaneously hypertensive rats. Food Chem. (2019) 276:376–82. doi: 10.1016/j.foodchem.2018.10.017
39. Yang J, Ding X, Qin Y, Zeng Y. Safety assessment of the biogenic amines in fermented soya beans and fermented bean curd. J Agric Food Chem. (2014) 62:7947–54. doi: 10.1021/jf501772s
40. Latorre-Moratalla ML, Bover-Cid S, Veciana-Nogues MT, Vidal-Carou MC. Control of biogenic amines in fermented sausages: role of starter cultures. Front Microbiol. (2012) 3:169. doi: 10.3389/fmicb.2012.00169
41. Moya VJ, Flores M, Aristoy MC, Toldra F. Pork meat quality affects peptide and amino acid profiles during the ageing process. Meat Sci. (2001) 58:197–206. doi: 10.1016/S0309-1740(00)00152-2
42. Wu G, Cross HR, Gehring KB, Savell JW, Arnold AN, Mcneill SH. Composition of free and peptide-bound amino acids in beef chuck, loin, and round cuts. J Anim Sci. (2016) 94:2603–13. doi: 10.2527/jas.2016-0478
43. Chen Y, Qiao Y, Xiao Y, Chen H, Zhao L, Huang M, et al. Differences in physicochemical and nutritional properties of breast and thigh meat from crossbred chickens, commercial broilers, and spent hens. Asian Austr J Anim Sci. (2016) 29:855–64. doi: 10.5713/ajas.15.0840
44. Perez-Palacios T, Barroso MA, Ruiz J, Antequera T. A rapid and accurate extraction procedure for analysing free amino acids in meat samples by GC-MS. Int J Anal Chem. (2015) 2015:209214. doi: 10.1155/2015/209214
45. Mohanty B, Mahanty A, Ganguly S, Sankar TV, Chakraborty K, Rangasamy A, et al. Amino acid compositions of 27 food fishes and their importance in clinical nutrition. J Amino Acids (2014) 2014:269797. doi: 10.1155/2014/269797
46. Linares DM, Del Rio B, Ladero V, Martinez N, Fernandez M, Martin MC, et al. Factors influencing biogenic amines accumulation in dairy products. Front Microbiol. (2012) 3:180. doi: 10.3389/fmicb.2012.00180
47. Schirone M, Tofalo R, Fasoli G, Perpetuini G, Corsetti A, Manetta AC, et al. High content of biogenic amines in Pecorino cheeses. Food Microbiol. (2013) 34:137–44. doi: 10.1016/j.fm.2012.11.022
48. Benkerroum N. Biogenic amines in dairy products: origin, incidence, and control means. Comp Rev Food Sci Food Saf. (2016) 15:801–26. doi: 10.1111/1541-4337.12212
49. Ramakrishna A, Giridhar P, Sankar KU, Ravishankar GA. Melatonin and serotonin profiles in beans of Coffea species. J Pineal Res. (2012) 52:470–6. doi: 10.1111/j.1600-079X.2011.00964.x
50. Taş NG, Yilmaz C, Gökmen V. Investigation of serotonin, free and protein-bound tryptophan in Turkish hazelnut varieties and effect of roasting on serotonin content. Food Res Int. (in press). doi: 10.1016/j.foodres.2018.11.051
51. Kema IP, Schellings AM, Meiborg G, Hoppenbrouwers CJ, Muskiet FA. Influence of a serotonin- and dopamine-rich diet on platelet serotonin content and urinary excretion of biogenic amines and their metabolites. Clin Chem. (1992) 38:1730–6.
52. Tapia MI, Sánchez-Morgado JR, García-Parra J, Ramírez R, Hernández T, González-Gómez D. Comparative study of the nutritional and bioactive compounds content of four walnut (Juglans regia L.) cultivars. J Food Comp Anal. (2013) 31:232–7. doi: 10.1016/j.jfca.2013.06.004
53. Yilmaz C, Tas NG, Kocadagli T, Gokmen V. Determination of serotonin in nuts and nut containing products by liquid chromatography tandem mass spectrometry. Food Chem. (2019) 272:347–53. doi: 10.1016/j.foodchem.2018.08.064
54. Jenkins TA, Nguyen JC, Polglaze KE, Bertrand PP. Influence of tryptophan and serotonin on mood and cognition with a possible role of the gut-brain axis. Nutrients (2016) 8:E56. doi: 10.3390/nu8010056
55. Owasoyo JO, Neri DF, Lamberth JG. Tyrosine and its potential use as a countermeasure to performance decrement in military sustained operations. Aviat Space Environ Med. (1992) 63:364–9.
56. Mahoney CR, Castellani J, Kramer FM, Young A, Lieberman HR. Tyrosine supplementation mitigates working memory decrements during cold exposure. Physiol Behav. (2007) 92:575–82. doi: 10.1016/j.physbeh.2007.05.003
57. Banderet LE, Lieberman HR. Treatment with tyrosine, a neurotransmitter precursor, reduces environmental stress in humans. Brain Res Bull. (1989) 22:759–62. doi: 10.1016/0361-9230(89)90096-8
58. Lieberman HR, Thompson LA, Caruso CM, Niro PJ, Mahoney CR, Mcclung JP, et al. The catecholamine neurotransmitter precursor tyrosine increases anger during exposure to severe psychological stress. Psychopharmacology (2015) 232:943–51. doi: 10.1007/s00213-014-3727-7
59. Kuroda M, Nozawa Y. Effect of dried-bonito broth on mood states: a pooled analysis of four randomized controlled human trials. Biomed Res. (2008) 29:175–9. doi: 10.2220/biomedres.29.175
60. Sasahara I, Fujimura N, Nozawa Y, Furuhata Y, Sato H. The effect of histidine on mental fatigue and cognitive performance in subjects with high fatigue and sleep disruption scores. Physiol Behav. (2015) 147:238–44. doi: 10.1016/j.physbeh.2015.04.042
61. Nozawa Y, Mimura M, Yamada K, Sugita M, Shibakusa T, Koyama N. Dried bonito broth improves cognitive function via the histaminergic system in mice. Biomed Res. (2014) 35:311–9. doi: 10.2220/biomedres.35.311
62. Sawai Y, Yamaguchi Y, Miyama D, Yoshitomi H. Cycling treatment of anaerobic and aerobic incubation increases the content of gamma-aminobutyric acid in tea shoots. Amino Acids (2001) 20:331–4. doi: 10.1007/s007260170049
63. Nacher A, Polache A, Moll-Navarro MJ, Pla-Delfina JM, Merino M. Intestinal absorption pathway of gamma-aminobutyric acid in rat small intestine. Biopharm Drug Dispos. (1994) 15:359–71. doi: 10.1002/bdd.2510150503
64. Thwaites DT, Basterfield L, Mccleave PM, Carter SM, Simmons NL. Gamma-Aminobutyric acid (GABA) transport across human intestinal epithelial (Caco-2) cell monolayers. Br J Pharmacol. (2000) 129:457–64. doi: 10.1038/sj.bjp.0703069
65. Auteri M, Zizzo MG, Serio R. GABA and GABA receptors in the gastrointestinal tract: from motility to inflammation. Pharmacol Res. (2015) 93:11–21. doi: 10.1016/j.phrs.2014.12.001
66. Klarer M, Arnold M, Gunther L, Winter C, Langhans W, Meyer U. Gut vagal afferents differentially modulate innate anxiety and learned fear. J Neurosci. (2014) 34:7067–76. doi: 10.1523/JNEUROSCI.0252-14.2014
67. Powley TL, Spaulding RA, Haglof SA. Vagal afferent innervation of the proximal gastrointestinal tract mucosa: chemoreceptor and mechanoreceptor architecture. J Comp Neurol. (2011) 519:644–60. doi: 10.1002/cne.22541
68. Ashworth-Preece M, Krstew E, Jarrott B, Lawrence AJ. Functional GABAA receptors on rat vagal afferent neurones. Br J Pharmacol. (1997) 120:469–75. doi: 10.1038/sj.bjp.0700909
69. Partosoedarso ER, Young RL, Blackshaw LA. GABA(B) receptors on vagal afferent pathways: peripheral and central inhibition. Am J Physiol Gastrointest Liver Physiol. (2001) 280:G658–68. doi: 10.1152/ajpgi.2001.280.4.G658
70. Shyamaladevi N, Jayakumar AR, Sujatha R, Paul V, Subramanian EH. Evidence that nitric oxide production increases gamma-amino butyric acid permeability of blood-brain barrier. Brain Res Bull. (2002) 57:231–6. doi: 10.1016/S0361-9230(01)00755-9
71. Steenbergen L, Sellaro R, Stock AK, Beste C, Colzato LS. gamma-Aminobutyric acid (GABA) administration improves action selection processes: a randomised controlled trial. Sci Rep. (2015) 5:12770. doi: 10.1038/srep12770
72. Byun JI, Shin YY, Chung SE, Shin WC. Safety and efficacy of gamma-aminobutyric acid from fermented rice germ in patients with insomnia symptoms: a randomized, double-blind trial. J Clin Neurol. (2018) 14:291–5. doi: 10.3988/jcn.2018.14.3.291
73. Yamatsu A, Yamashita Y, Pandharipande T, Maru I, Kim M. Effect of oral γ-aminobutyric acid (GABA) administration on sleep and its absorption in humans. Food Sci Biotechnol. (2016) 25:547–51. doi: 10.1007/s10068-016-0076-9
74. Spencer NJ, Keating DJ. Is there a role for endogenous 5-HT in gastrointestinal motility? how recent studies have changed our understanding. Adv Exp Med Biol. (2016) 891:113–22. doi: 10.1007/978-3-319-27592-5_11
75. Degg TJ, Allen KR, Barth JH. Measurement of plasma 5-hydroxyindoleacetic acid in carcinoid disease: an alternative to 24-h urine collections? Ann Clin Biochem. (2000) 37 (Pt 5):724–6. doi: 10.1258/0004563001899780
76. Feldman JM, Lee EM. Serotonin content of foods: effect on urinary excretion of 5-hydroxyindoleacetic acid. Am J Clin Nutr. (1985) 42:639–43. doi: 10.1093/ajcn/42.4.639
77. Davidson L, Vandongen R, Beilin LJ. Effect of eating bananas on plasma free and sulfate-conjugated catecholamines. Life Sci. (1981) 29:1773–8. doi: 10.1016/0024-3205(81)90187-9
78. De Jong WH, Eisenhofer G, Post WJ, Muskiet FA, De Vries EG, Kema IP. Dietary influences on plasma and urinary metanephrines: implications for diagnosis of catecholamine-producing tumors. J Clin Endocrinol Metab. (2009) 94:2841–9. doi: 10.1210/jc.2009-0303
79. Kanazawa K, Sakakibara H. High content of dopamine, a strong antioxidant, in Cavendish banana. J Agric Food Chem. (2000) 48:844–8. doi: 10.1021/jf9909860
80. Flockhart DA. Dietary restrictions and drug interactions with monoamine oxidase inhibitors: an update. J Clin Psychiatry (2012) 73(Suppl. 1):17–24. doi: 10.4088/JCP.11096su1c.03
81. Grieshop CM, Fahey GC Jr. Comparison of quality characteristics of soybeans from Brazil, China, and the United States. J Agric Food Chem. (2001) 49:2669–73. doi: 10.1021/jf0014009
82. Lee J, Nam DS, Kong C. Variability in nutrient composition of cereal grains from different origins. Springerplus (2016) 5:419. doi: 10.1186/s40064-016-2046-3
83. De Coca-Sinova A, Valencia DG, Jimenez-Moreno E, Lazaro R, Mateos GG. Apparent ileal digestibility of energy, nitrogen, and amino acids of soybean meals of different origin in broilers. Poult Sci. (2008) 87:2613–23. doi: 10.3382/ps.2008-00182
84. Smith TK, Tapia-Salazar M, Cruz-Suarez LE, Ricque-Marie D. Feed-borne biogenic amines: natural toxicants or growth promotors? In: Cruz -Suárez LE, Ricque-Marie D, Tapia-Salazar M, Olvera-Novoa MA, Civera-Cerecedo YR, editors. Avances en Nutrición Acuícola V. Memorias del V Simposium Internacional de Nutrición Acuícola (Mérida) (2000).
85. Friday ML, Firman JD, Bermudez AJ. Effects of biogenic amines on broiler performance. J Appl Poult Res. (1999) 8:408–13. doi: 10.1093/japr/8.4.408
86. Krieger DT, Hauser H, Krey LC. Suprachiasmatic nuclear lesions do not abolish food-shifted circadian adrenal and temperature rhythmicity. Science (1977) 197:398–9. doi: 10.1126/science.877566
87. Uchida A, Zigman JM, Perello M. Ghrelin and eating behavior: evidence and insights from genetically-modified mouse models. Front Neurosci. (2013) 7:121. doi: 10.3389/fnins.2013.00121
88. Abizaid A, Liu ZW, Andrews ZB, Shanabrough M, Borok E, Elsworth JD, et al. Ghrelin modulates the activity and synaptic input organization of midbrain dopamine neurons while promoting appetite. J Clin Invest. (2006) 116:3229–39. doi: 10.1172/JCI29867
89. Hansson C, Alvarez-Crespo M, Taube M, Skibicka KP, Schmidt L, Karlsson-Lindahl L, et al. Influence of ghrelin on the central serotonergic signaling system in mice. Neuropharmacology (2014) 79:498–505. doi: 10.1016/j.neuropharm.2013.12.012
90. Queipo-Ortuno MI, Seoane LM, Murri M, Pardo M, Gomez-Zumaquero JM, Cardona F, et al. Gut microbiota composition in male rat models under different nutritional status and physical activity and its association with serum leptin and ghrelin levels. PLoS ONE (2013) 8:e65465. doi: 10.1371/journal.pone.0065465
91. Covasa M, Duca FA, Swartz TD. Gut microbiota restores central neuropeptide deficits present in germ free animals. FASEB J. (2016) 30:1166.9–1166.9.
92. Sun Y, Ahmed S, Smith RG. Deletion of ghrelin impairs neither growth nor appetite. Mol Cell Biol. (2003) 23:7973–81. doi: 10.1128/MCB.23.22.7973-7981.2003
93. Abizaid A. Ghrelin and dopamine: new insights on the peripheral regulation of appetite. J Neuroendocrinol. (2009) 21:787–93. doi: 10.1111/j.1365-2826.2009.01896.x
94. Hardman CA, Herbert VM, Brunstrom JM, Munafo MR, Rogers PJ. Dopamine and food reward: effects of acute tyrosine/phenylalanine depletion on appetite. Physiol Behav. (2012) 105:1202–7. doi: 10.1016/j.physbeh.2011.12.022
95. Lyte M. Microbial endocrinology as a basis for improved L-DOPA bioavailability in Parkinson's patients treated for Helicobacter pylori. Med Hypotheses (2010) 74:895–7. doi: 10.1016/j.mehy.2009.11.001
96. Jang EJ, Park SW, Park JS, Park SJ, Hahm KB, Paik SY, et al. The influence of the eradication of Helicobacter pylori on gastric ghrelin, appetite, and body mass index in patients with peptic ulcer disease. J Gastroenterol Hepatol. (2008) 23(Suppl. 2):S278–85. doi: 10.1111/j.1440-1746.2008.05415.x
97. Cammisotto PG, Levy E, Bukowiecki LJ, Bendayan M. Cross-talk between adipose and gastric leptins for the control of food intake and energy metabolism. Prog Histochem Cytochem. (2010) 45:143–200. doi: 10.1016/j.proghi.2010.06.001
98. Cammisotto PG, Renaud C, Gingras D, Delvin E, Levy E, Bendayan M. Endocrine and exocrine secretion of leptin by the gastric mucosa. J Histochem Cytochem. (2005) 53:851–60. doi: 10.1369/jhc.5A6620.2005
99. Cammisotto PG, Gingras D, Renaud C, Levy E, Bendayan M. Secretion of soluble leptin receptors by exocrine and endocrine cells of the gastric mucosa. Am J Physiol Gastrointest Liver Physiol. (2006) 290:G242–9. doi: 10.1152/ajpgi.00334.2005
100. Cammisotto P, Bendayan M. A review on gastric leptin: the exocrine secretion of a gastric hormone. Anat Cell Biol. (2012) 45:1–16. doi: 10.5115/acb.2012.45.1.1
101. Schele E, Grahnemo L, Anesten F, Hallen A, Backhed F, Jansson JO. The gut microbiota reduces leptin sensitivity and the expression of the obesity-suppressing neuropeptides proglucagon (Gcg) and brain-derived neurotrophic factor (Bdnf) in the central nervous system. Endocrinology (2013) 154:3643–51. doi: 10.1210/en.2012-2151
102. Ellekilde M, Krych L, Hansen CH, Hufeldt MR, Dahl K, Hansen LH, et al. Characterization of the gut microbiota in leptin deficient obese mice - Correlation to inflammatory and diabetic parameters. Res Vet Sci. (2014) 96:241–50. doi: 10.1016/j.rvsc.2014.01.007
103. Rajala MW, Patterson CM, Opp JS, Foltin SK, Young VB, Myers MG Jr. Leptin acts independently of food intake to modulate gut microbial composition in male mice. Endocrinology (2014) 155:748–57. doi: 10.1210/en.2013-1085
104. Thaiss CA, Levy M, Grosheva I, Zheng D, Soffer E, Blacher E, et al. Hyperglycemia drives intestinal barrier dysfunction and risk for enteric infection. Science (2018) 359:1376–83. doi: 10.1126/science.aar3318
105. Amar J, Chabo C, Waget A, Klopp P, Vachoux C, Bermudez-Humaran LG, et al. Intestinal mucosal adherence and translocation of commensal bacteria at the early onset of type 2 diabetes: molecular mechanisms and probiotic treatment. EMBO Mol Med. (2011) 3:559–72. doi: 10.1002/emmm.201100159
106. Sundin OH, Mendoza-Ladd A, Zeng M, Diaz-Arevalo D, Morales E, Fagan BM, et al. The human jejunum has an endogenous microbiota that differs from those in the oral cavity and colon. BMC Microbiol. (2017) 17:160. doi: 10.1186/s12866-017-1059-6
107. Farr OM, Gavrieli A, Mantzoros CS. Leptin applications in 2015: what have we learned about leptin and obesity? Curr Opin Endocrinol Diabetes Obes. (2015) 22:353–9. doi: 10.1097/MED.0000000000000184
108. Roland BC, Lee D, Miller LS, Vegesna A, Yolken R, Severance E, et al. Obesity increases the risk of small intestinal bacterial overgrowth (SIBO). Neurogastroenterol Motil. (2018) 30:3. doi: 10.1111/nmo.13199
110. Kim JS, De La Serre CB. Diet, gut microbiota composition and feeding behavior. Physiol Behav. (2018) 192:177–81. doi: 10.1016/j.physbeh.2018.03.026
111. Lyte M. The role of catecholamines in gram-negative sepsis. Med Hypotheses (1992) 37:255–8. doi: 10.1016/0306-9877(92)90197-K
112. Lyte M. The role of microbial endocrinology in infectious disease. J Endocrinol. (1993) 137:343–5. doi: 10.1677/joe.0.1370343
113. Van Der Gugten J, Slangen JL. Activity of central catecholamine systems and feeding behavior. In: Usdin E, Kopin IJ, Barchas Pergamon J, editors. Catecholamines: Basic and Clinical Frontiers Pacific Grove: Pergamon Press (1979), p. 1729–31. doi: 10.1016/B978-1-4832-8363-0.50532-2
114. Bonaz B, Bazin T, Pellissier S. The vagus nerve at the interface of the microbiota-gut-brain axis. Front Neurosci. (2018) 12:49. doi: 10.3389/fnins.2018.00049
115. Kral JG. Behavioral effects of vagotomy in humans. J Auton Nerv Syst. (1983) 9:273–81. doi: 10.1016/0165-1838(83)90147-9
116. Asano Y, Hiramoto T, Nishino R, Aiba Y, Kimura T, Yoshihara K, et al. Critical role of gut microbiota in the production of biologically active, free catecholamines in the gut lumen of mice. Am J Physiol Gastrointest Liver Physiol. (2012) 303:G1288–95. doi: 10.1152/ajpgi.00341.2012
117. Gaykema RP, Goehler LE, Lyte M. Brain response to cecal infection with Campylobacter jejuni: analysis with Fos immunohistochemistry. Brain Behav Immun. (2004) 18:238–45. doi: 10.1016/j.bbi.2003.08.002
118. Bercik P, Park AJ, Sinclair D, Khoshdel A, Lu J, Huang X, et al. The anxiolytic effect of Bifidobacterium longum NCC3001 involves vagal pathways for gut-brain communication. Neurogastroenterol Motil. (2011) 23:1132–9. doi: 10.1111/j.1365-2982.2011.01796.x
119. Bravo JA, Forsythe P, Chew MV, Escaravage E, Savignac HM, Dinan TG, et al. Ingestion of Lactobacillus strain regulates emotional behavior and central GABA receptor expression in a mouse via the vagus nerve. Proc Natl Acad Sci USA. (2011) 108:16050–5. doi: 10.1073/pnas.1102999108
120. Berthoud HR, Neuhuber WL. Functional and chemical anatomy of the afferent vagal system. Auton Neurosci. (2000) 85:1–17. doi: 10.1016/S1566-0702(00)00215-0
121. Costa M, Brookes SHJ, Zagorodnyuk V. How many kinds of visceral afferents? Gut (2004) 53:ii1. doi: 10.1136/gut.2003.033407
122. Dockray GJ. Enteroendocrine cell signalling via the vagus nerve. Curr Opin Pharmacol. (2013) 13:954–8. doi: 10.1016/j.coph.2013.09.007
123. Steinert RE, Beglinger C. Nutrient sensing in the gut: interactions between chemosensory cells, visceral afferents and the secretion of satiation peptides. Physiol Behav. (2011) 105:62–70. doi: 10.1016/j.physbeh.2011.02.039
124. Sen T, Cawthon CR, Ihde BT, Hajnal A, Dilorenzo PM, De La Serre CB, et al. Diet-driven microbiota dysbiosis is associated with vagal remodeling and obesity. Physiol Behav. (2017) 173:305–17. doi: 10.1016/j.physbeh.2017.02.027
125. Bellono NW, Bayrer JR, Leitch DB, Castro J, Zhang C, O'donnell TA, et al. Enterochromaffin cells are gut chemosensors that couple to sensory neural pathways. Cell (2017) 170:185–198 e116. doi: 10.1016/j.cell.2017.05.034
126. Zhu JX, Zhu XY, Owyang C, Li Y. Intestinal serotonin acts as a paracrine substance to mediate vagal signal transmission evoked by luminal factors in the rat. J Physiol. (2001) 530:431–42. doi: 10.1111/j.1469-7793.2001.0431k.x
127. Berthoud HR, Patterson LM, Neumann F, Neuhuber WL. Distribution and structure of vagal afferent intraganglionic laminar endings (IGLEs) in the rat gastrointestinal tract. Anat Embryol (1997) 195:183–91. doi: 10.1007/s004290050037
128. Wang L, Martinez V, Larauche M, Tache Y. Proximal colon distension induces Fos expression in oxytocin-, vasopressin-, CRF- and catecholamines-containing neurons in rat brain. Brain Res. (2009) 1247:79–91. doi: 10.1016/j.brainres.2008.09.094
129. Blouet C, Schwartz GJ. Brainstem nutrient sensing in the nucleus of the solitary tract inhibits feeding. Cell Metab. (2012) 16:579–87. doi: 10.1016/j.cmet.2012.10.003
130. Cailotto C, Gomez-Pinilla PJ, Costes LM, Van Der Vliet J, Di Giovangiulio M, Nemethova A, et al. Neuro-anatomical evidence indicating indirect modulation of macrophages by vagal efferents in the intestine but not in the spleen. PLoS ONE (2014) 9:e87785. doi: 10.1371/journal.pone.0087785
131. Matteoli G, Gomez-Pinilla PJ, Nemethova A, Di Giovangiulio M, Cailotto C, Van Bree SH, et al. A distinct vagal anti-inflammatory pathway modulates intestinal muscularis resident macrophages independent of the spleen. Gut (2014) 63:938–48. doi: 10.1136/gutjnl-2013-304676
132. Bonaz B, Sinniger V, Pellissier S. Anti-inflammatory properties of the vagus nerve: potential therapeutic implications of vagus nerve stimulation. J Physiol. (2016) 594:5781–90. doi: 10.1113/JP271539
133. Luyer MD, Greve JW, Hadfoune M, Jacobs JA, Dejong CH, Buurman WA. Nutritional stimulation of cholecystokinin receptors inhibits inflammation via the vagus nerve. J Exp Med. (2005) 202:1023–9. doi: 10.1084/jem.20042397
134. Swartz TD, Duca FA, De Wouters T, Sakar Y, Covasa M. Up-regulation of intestinal type 1 taste receptor 3 and sodium glucose luminal transporter-1 expression and increased sucrose intake in mice lacking gut microbiota. Br J Nutr. (2012) 107:621–30. doi: 10.1017/S0007114511003412
135. Duca FA, Swartz TD, Sakar Y, Covasa M. Increased oral detection, but decreased intestinal signaling for fats in mice lacking gut microbiota. PLoS ONE (2012) 7:e39748. doi: 10.1371/journal.pone.0039748
136. Leitao-Goncalves R, Carvalho-Santos Z, Francisco AP, Fioreze GT, Anjos M, Baltazar C, et al. Commensal bacteria and essential amino acids control food choice behavior and reproduction. PLoS Biol. (2017) 15:e2000862. doi: 10.1371/journal.pbio.2000862
137. Brown RJ, Rother KI. Non-nutritive sweeteners and their role in the gastrointestinal tract. J Clin Endocrinol Metab. (2012) 97:2597–605. doi: 10.1210/jc.2012-1475
138. Lu VB, Gribble FM, Reimann F. Free fatty acid receptors in enteroendocrine cells. Endocrinology (2018) 159:2826–35. doi: 10.1210/en.2018-00261
139. Symonds EL, Peiris M, Page AJ, Chia B, Dogra H, Masding A, et al. Mechanisms of activation of mouse and human enteroendocrine cells by nutrients. Gut (2015) 64:618. doi: 10.1136/gutjnl-2014-306834
140. Goodman BE. Insights into digestion and absorption of major nutrients in humans. Adv Physiol Educ. (2010) 34:44–53. doi: 10.1152/advan.00094.2009
141. Eaton JM, Dess NK, Chapman CD. Sweet success, bitter defeat: a taste phenotype predicts social status in selectively bred rats. PLoS ONE (2012) 7:e46606. doi: 10.1371/journal.pone.0046606
142. Larraufie P, Martin-Gallausiaux C, Lapaque N, Dore J, Gribble FM, Reimann F, et al. SCFAs strongly stimulate PYY production in human enteroendocrine cells. Sci Rep. (2018) 8:74. doi: 10.1038/s41598-017-18259-0
143. Abbott CR, Monteiro M, Small CJ, Sajedi A, Smith KL, Parkinson JR, et al. The inhibitory effects of peripheral administration of peptide YY(3-36) and glucagon-like peptide-1 on food intake are attenuated by ablation of the vagal-brainstem-hypothalamic pathway. Brain Res. (2005) 1044:127–31. doi: 10.1016/j.brainres.2005.03.011
144. Decastro M, Nankova BB, Shah P, Patel P, Mally PV, Mishra R, et al. Short chain fatty acids regulate tyrosine hydroxylase gene expression through a cAMP-dependent signaling pathway. Brain Res Mol Brain Res. (2005) 142:28–38. doi: 10.1016/j.molbrainres.2005.09.002
145. Gagliano H, Delgado-Morales R, Sanz-Garcia A, Armario A. High doses of the histone deacetylase inhibitor sodium butyrate trigger a stress-like response. Neuropharmacology (2014) 79:75–82. doi: 10.1016/j.neuropharm.2013.10.031
146. Brown DR. Catecholamine-directed epithelial cell interactions with bacteria in the intestinal mucosa. Adv Exp Med Biol. (2016) 874:79–99. doi: 10.1007/978-3-319-20215-0_3
147. Lyte M, Chapel A, Lyte JM, Ai Y, Proctor A, Jane JL, et al. Resistant starch alters the microbiota-gut brain axis: implications for dietary modulation of behavior. PLoS ONE (2016) 11:e0146406. doi: 10.1371/journal.pone.0146406
148. Ritzhaupt A, Wood IS, Ellis A, Hosie KB, Shirazi-Beechey SP. Identification and characterization of a monocarboxylate transporter (MCT1) in pig and human colon: its potential to transport L-lactate as well as butyrate. J Physiol. (1998) 513 (Pt 3):719–32. doi: 10.1111/j.1469-7793.1998.719ba.x
149. Gerhart DZ, Enerson BE, Zhdankina OY, Leino RL, Drewes LR. Expression of monocarboxylate transporter MCT1 by brain endothelium and glia in adult and suckling rats. Am J Physiol. (1997) 273:E207–13. doi: 10.1152/ajpendo.1997.273.1.E207
150. Frost G, Sleeth ML, Sahuri-Arisoylu M, Lizarbe B, Cerdan S, Brody L, et al. The short-chain fatty acid acetate reduces appetite via a central homeostatic mechanism. Nat Commun. (2014) 5:3611. doi: 10.1038/ncomms4611
151. Mardinoglu A, Shoaie S, Bergentall M, Ghaffari P, Zhang C, Larsson E, et al. The gut microbiota modulates host amino acid and glutathione metabolism in mice. Mol Syst Biol. (2015) 11:834. doi: 10.15252/msb.20156487
152. Metges CC, El-Khoury AE, Henneman L, Petzke KJ, Grant I, Bedri S, et al. Availability of intestinal microbial lysine for whole body lysine homeostasis in human subjects. Am J Physiol. (1999) 277:E597–607. doi: 10.1152/ajpendo.1999.277.4.E597
153. Payne A, Wang X, Ivy MT, Stewart A, Nelson K, Darris C, et al. Lysine mediation of neuroendocrine food regulation in guinea fowl. Poult Sci. (2016) 95:276–86. doi: 10.3382/ps/pev326
154. Matsumoto M, Kibe R, Ooga T, Aiba Y, Kurihara S, Sawaki E, et al. Impact of intestinal microbiota on intestinal luminal metabolome. Sci Rep. (2012) 2:233. doi: 10.1038/srep00233
155. Nissim I, Horyn O, Daikhin Y, Chen P, Li C, Wehrli SL, et al. The molecular and metabolic influence of long term agmatine consumption. J Biol Chem. (2014) 289:9710–29. doi: 10.1074/jbc.M113.544726
156. Mu C, Yang Y, Luo Z, Guan L, Zhu W. The colonic microbiome and epithelial transcriptome are altered in rats fed a high-protein diet compared with a normal-protein diet. J Nutr. (2016) 146:474–83. doi: 10.3945/jn.115.223990
157. Sharafedtinov KK, Plotnikova OA, Alexeeva RI, Sentsova TB, Songisepp E, Stsepetova J, et al. Hypocaloric diet supplemented with probiotic cheese improves body mass index and blood pressure indices of obese hypertensive patients–a randomized double-blind placebo-controlled pilot study. Nutr J. (2013) 12:138. doi: 10.1186/1475-2891-12-138
158. Hubbard TD, Murray IA, Perdew GH. Indole and tryptophan metabolism: endogenous and dietary routes to ah receptor activation. Drug Metab Dispos. (2015) 43:1522–35. doi: 10.1124/dmd.115.064246
159. Medvedev A, Buneeva O, Gnedenko O, Ershov P, Ivanov A. Isatin, an endogenous nonpeptide biofactor: a review of its molecular targets, mechanisms of actions, and their biomedical implications. Biofactors (2018) 44:95–108. doi: 10.1002/biof.1408
160. Goldstein DS. Catecholamines 101. Clin Autonom Res. (2010) 20:331–52. doi: 10.1007/s10286-010-0065-7
161. Hata T, Asano Y, Yoshihara K, Kimura-Todani T, Miyata N, Zhang XT, et al. Regulation of gut luminal serotonin by commensal microbiota in mice. PLoS ONE (2017) 12:e0180745. doi: 10.1371/journal.pone.0180745
162. Markus R, Panhuysen G, Tuiten A, Koppeschaar H. Effects of food on cortisol and mood in vulnerable subjects under controllable and uncontrollable stress. Physiol Behav. (2000) 70:333–42. doi: 10.1016/S0031-9384(00)00265-1
163. Pecoraro N, Reyes F, Gomez F, Bhargava A, Dallman MF. Chronic stress promotes palatable feeding, which reduces signs of stress: feedforward and feedback effects of chronic stress. Endocrinology (2004) 145:3754–62. doi: 10.1210/en.2004-0305
164. Tryon MS, Decant R, Laugero KD. Having your cake and eating it too: a habit of comfort food may link chronic social stress exposure and acute stress-induced cortisol hyporesponsiveness. Physiol Behav. (2013) 114–115:32–7. doi: 10.1016/j.physbeh.2013.02.018
165. Macht M, Mueller J. Immediate effects of chocolate on experimentally induced mood states. Appetite (2007) 49:667–74. doi: 10.1016/j.appet.2007.05.004
166. Tsilimigras MCB, Gharaibeh RZ, Sioda M, Gray L, Fodor AA, Lyte M. Interactions between stress and sex in microbial responses within the microbiota-gut-brain axis in a mouse model. Psychosom Med. (2018) 80:361–9. doi: 10.1097/PSY.0000000000000572
167. Egan AE, Thompson AMK, Buesing D, Fourman SM, Packard AEB, Terefe T, et al. Palatable food affects HPA axis responsivity and forebrain neurocircuitry in an estrous cycle-specific manner in female rats. Neuroscience (2018) 384:224–40. doi: 10.1016/j.neuroscience.2018.05.030
168. Bruinsma K, Taren DL. Chocolate: food or drug? J Am Diet Assoc. (1999) 99:1249–56. doi: 10.1016/S0002-8223(99)00307-7
169. Kinzig KP, Hargrave SL, Honors MA. Binge-type eating attenuates corticosterone and hypophagic responses to restraint stress. Physiol Behav. (2008) 95:108–13. doi: 10.1016/j.physbeh.2008.04.026
170. Warne JP. Shaping the stress response: interplay of palatable food choices, glucocorticoids, insulin and abdominal obesity. Mol Cell Endocrinol. (2009) 300:137–46. doi: 10.1016/j.mce.2008.09.036
171. Cima I, Corazza N, Dick B, Fuhrer A, Herren S, Jakob S, et al. Intestinal epithelial cells synthesize glucocorticoids and regulate T cell activation. J Exp Med. (2004) 200:1635–46. doi: 10.1084/jem.20031958
172. Hunt C, Hambly C. Faecal corticosterone concentrations indicate that separately housed male mice are not more stressed than group housed males. Physiol Behav. (2006) 87:519–26. doi: 10.1016/j.physbeh.2005.11.013
173. Uetake K, Yang CH, Endo A, Tanaka T. Effects of sheltering on behavior and fecal corticosterone level of elderly dogs. Front Vet Sci. (2016) 3:103. doi: 10.3389/fvets.2016.00103
174. Sheriff MJ, Dantzer B, Delehanty B, Palme R, Boonstra R. Measuring stress in wildlife: techniques for quantifying glucocorticoids. Oecologia (2011) 166:869–87. doi: 10.1007/s00442-011-1943-y
175. Lyte JM, Proctor A, Phillips GJ, Lyte M, Wannemuehler M. Altered Schaedler flora mice: a defined microbiota animal model to study the microbiota-gut-brain axis. Behav Brain Res. (2018) 356:221–6. doi: 10.1016/j.bbr.2018.08.022
176. Verster JC, Koenig J. Caffeine intake and its sources: a review of national representative studies. Crit Rev Food Sci Nutr. (2018) 58:1250–9. doi: 10.1080/10408398.2016.1247252
177. O'neill CE, Newsom RJ, Stafford J, Scott T, Archuleta S, Levis SC, et al. Adolescent caffeine consumption increases adulthood anxiety-related behavior and modifies neuroendocrine signaling. Psychoneuroendocrinology (2016) 67:40–50. doi: 10.1016/j.psyneuen.2016.01.030
178. Giles GE, Spring AM, Urry HL, Moran JM, Mahoney CR, Kanarek RB. Caffeine alters emotion and emotional responses in low habitual caffeine consumers. Can J Physiol Pharmacol. (2018) 96:191–9. doi: 10.1139/cjpp-2017-0224
179. Sudo N, Chida Y, Aiba Y, Sonoda J, Oyama N, Yu XN, et al. Postnatal microbial colonization programs the hypothalamic-pituitary-adrenal system for stress response in mice. J Physiol. (2004) 558:263–75. doi: 10.1113/jphysiol.2004.063388
180. Li L, Xu Z, Zhou Y, Sun L, Liu Z, Chen H, et al. Global effects of catecholamines on Actinobacillus pleuropneumoniae gene expression. PLoS ONE (2012) 7:e31121. doi: 10.1371/journal.pone.0031121
181. Lyte M. Microbial endocrinology in the pathogenesis of infectious disease. Microbiol Spectr (2016) 4:2. doi: 10.1128/microbiolspec.VMBF-0021-2015
182. Dickson RP, Erb-Downward JR, Prescott HC, Martinez FJ, Curtis JL, Lama VN, et al. Intraalveolar catecholamines and the human lung microbiome. Am J Respir Crit Care Med. (2015) 192:257–9. doi: 10.1164/rccm.201502-0326LE
183. Corti R, Binggeli C, Sudano I, Spieker L, Hanseler E, Ruschitzka F, et al. Coffee acutely increases sympathetic nerve activity and blood pressure independently of caffeine content: role of habitual versus nonhabitual drinking. Circulation (2002) 106:2935–40. doi: 10.1161/01.CIR.0000046228.97025.3A
184. Lomax AE, Sharkey KA, Furness JB. The participation of the sympathetic innervation of the gastrointestinal tract in disease states. Neurogastroenterol Motil. (2010) 22:7–18. doi: 10.1111/j.1365-2982.2009.01381.x
185. Aneman A, Eisenhofer G, Olbe L, Dalenback J, Nitescu P, Fandriks L, et al. Sympathetic discharge to mesenteric organs and the liver. Evidence for substantial mesenteric organ norepinephrine spillover. J Clin Invest. (1996) 97:1640–6. doi: 10.1172/JCI118590
186. Goldstein DS, Eisenhofer G, Kopin IJ. Sources and significance of plasma levels of catechols and their metabolites in humans. J Pharmacol Exp Ther. (2003) 305:800–11. doi: 10.1124/jpet.103.049270
187. Poznyak V, Fleischmann A, Rekve D, Rylett M, Rehm J, Gmel G. The world health organization's global monitoring system on alcohol and health. Alcohol Res. (2013) 35:244–9.
188. Bode C, Bode JC. Alcohol's role in gastrointestinal tract disorders. Alcohol Health Res World (1997) 21:76–83.
189. Sullivan EV, Harris RA, Pfefferbaum A. Alcohol's effects on brain and behavior. Alcohol Res Health (2010) 33:127–43.
191. Gorky J, Schwaber J. The role of the gut-brain axis in alcohol use disorders. Prog Neuropsychopharmacol Biol Psychiatry (2016) 65:234–41. doi: 10.1016/j.pnpbp.2015.06.013
Keywords: microbial endocrinology, diet, nutrition, microbiota-gut-brain axis, food, neuroendocrine, bacteria, probiotic
Citation: Lyte JM (2019) Eating for 3.8 × 1013: Examining the Impact of Diet and Nutrition on the Microbiota-Gut-Brain Axis Through the Lens of Microbial Endocrinology. Front. Endocrinol. 9:796. doi: 10.3389/fendo.2018.00796
Received: 12 November 2018; Accepted: 19 December 2018;
Published: 29 January 2019.
Edited by:
John Bienenstock, McMaster University, CanadaReviewed by:
Bruno Bonaz, Centre Hospitalier Universitaire de Grenoble, FrancePaolo Magni, University of Milan, Italy
Copyright © 2019 Lyte. This is an open-access article distributed under the terms of the Creative Commons Attribution License (CC BY). The use, distribution or reproduction in other forums is permitted, provided the original author(s) and the copyright owner(s) are credited and that the original publication in this journal is cited, in accordance with accepted academic practice. No use, distribution or reproduction is permitted which does not comply with these terms.
*Correspondence: Joshua M. Lyte, joshua.lyte@usda.gov