- 1Department of Psychosomatic Medicine and Psychotherapy, University Hospital Tübingen, Tübingen, Germany
- 2Charité Center for Internal Medicine and Dermatology, Department for Psychosomatic Medicine, Charité - Universitätsmedizin Berlin, Corporate Member of Freie Universität Berlin, Humboldt-Universität zu Berlin and Berlin Institute of Health, Berlin, Germany
- 3CURE/Digestive Diseases Research Center, Vatche and Tamar Manoukian Digestive Diseases Division, David Geffen School of Medicine, University of California, Los Angeles, Los Angeles, CA, United States
- 4VA Greater Los Angeles Health Care System, Los Angeles, CA, United States
The gut-brain axis represents a bidirectional communication route between the gut and the central nervous system comprised of neuronal as well as humoral signaling. This system plays an important role in the regulation of gastrointestinal as well as homeostatic functions such as hunger and satiety. Recent years also witnessed an increased knowledge on the modulation of this axis under conditions of exogenous or endogenous stressors. The present review will discuss the alterations of neuroendocrine gut-brain signaling under conditions of stress and the respective implications for the regulation of food intake.
Introduction
Peripheral signals reach the brain via neuronal and humoral pathways. The neuronal connection from the gut to the brain through vagal afferents originating from pseudo-unipolar cell bodies located in the nodose ganglia is the most extensively investigated (1, 2). The vagus nerve is composed of over 80% of afferent fibers which convey chemical and mechanosensory signals involved in the regulation of food intake and body weight (3). Peptide hormones predominantly produced in the gut interact with cognate G protein seven transmembrane domain receptors localized on nodose ganglia neurons (4). The expression of these receptors is modulated by feeding and fasting (2, 5) underlining the importance of vagal pathways in the control of energy homeostasis.
Stress influences the expression or circulating levels of several gastrointestinal peptides involved in the regulation of metabolic status under conditions of hunger or satiety (6). The impact of these alterations on the stress response has subsequently been investigated. The present review will highlight the impact of stress on peptidergic gut-brain hormones primarily involved in the regulation of food intake along with the functional implications.
Modulation of Gut-Brain Signaling Under Conditions of Stress
Ghrelin
Ghrelin has been identified in the rat stomach (7) which is by far the major site of synthesis as indicated by the pronounced decrease of circulating ghrelin levels following gastrectomy (8). Ghrelin is produced in gastric endocrine X/A-like cells (human nomenclature: P/D1 cells) (9) and bears a unique fatty acid residue on its third amino acid essential to bind to its receptor, the growth hormone secretagogue receptor 1a (GHSR1a) (7) now also designated as the ghrelin receptor (GRLN) (10). The enzyme catalyzing this acylation was identified later on and named ghrelin-O-acyltransferase (GOAT) (11, 12). Double labeling showed that GOAT immunoreactive cells co-labeled with ghrelin expression in rodents (13) and humans (14). In addition, the finding that GOAT was detected in the pancreas (15) and circulation of rodents (13) and humans (16) supports additional extragastric acylation of the peptide.
Early on, ghrelin has been reported to stimulate food intake after peripheral and central injection in animals (17) and peripheral infusion in humans (18) leading to an increased body weight after repeated injections (19). Expression of the GHSR1a on vagal afferents and the blunting of the peptide's orexigenic action by vagotomy or selective reduction of the GHSR1a in nodose ganglia support a major role of the vagus in mediating ghrelin's action in rats (20, 21). Nonetheless, ghrelin was also shown to cross the blood-brain barrier in both directions (22) indicative of an additional humoral mode of signaling. In the hypothalamus, the GHSR1a is expressed on neuropeptide Y (NPY)/agouti-related peptide (AgRP) neurons of the arcuate nucleus (23, 24). Neuroanatomical and functional studies using optogenetics indicate that ghrelin expressed in axon terminals innervating hypothalamic nuclei increases NPY/AgRP activity (25, 26). In addition, the ghrelin-induced stimulation of food intake is abolished in NPY/AgRP knockout mice (27) demonstrating an essential role of these signaling pathways in mediating ghrelin's orexigenic action in the hypothalamus.
The predominant form of circulating ghrelin is, however, the non-acylated form, des-acyl ghrelin (28, 29). Des-acyl ghrelin initially received little attention due to its lack of affinity to the GHSR1a (7). Nonetheless, des-acyl ghrelin exhibits several biological actions such as decreasing anxiety after intraperitoneral injection in receptor knockout mice (30). Peripheral or intracerebroventricular pretreatment with des-acyl ghrelin blunts the orexigenic action of ghrelin in rats (31) and mice (32). Des-acyl ghrelin's action takes place in a subset of arcuate nucleus neurons distinct from those activated by ghrelin (32). Studies using fluorescein des-acyl ghrelin injected intracerebroventricularly in mice demonstrate that the peptide binds selectively and mainly on arcuate neurons in a GHSR1a independent manner (32). However, to date the receptor mediating des-acyl ghrelin's effects remains to be identified.
Exposure to various acute or chronic stressors influences ghrelin expression and circulating levels although the response varies with the modality of stressors and experimental conditions as detailed in a previous review (6). Tail pinch or starvation increases gastric ghrelin mRNA expression in mice (33). Several other acute stressors including psychological (water avoidance stress, trier social stress test), physical (cold ambient temperature, restraint at 18° C, cholecystectomy, colectomy, cold pressure test) or metabolic (fasting) increase circulating ghrelin levels (34–43). It is to note that in the clinical setting the acute social stress test-induced rise in circulating ghrelin and cortisol levels was not associated with binge eating (35). Likewise, chronic stressors such as repeated restraint in rats, social defeat in mice or trauma in humans also induce a sustained elevation of circulating ghrelin levels lasting for months after the cessation of the stress making ghrelin a persistent biomarker of chronic stress (44–50). The rise in ghrelin may represent a compensatory action to counteract chronic stress-induced anxiety and depression-like behavior (49, 51, 52). Indeed, ghrelin increased the rewarding aspect of food (46) and body weight observed under these conditions, effects no longer observed in GHSR1a knockout mice (47).
By contrast, conditions of stress associated with inflammation decrease ghrelin expression or circulating levels (6). In detail, immune stress triggered by intraperitoneal injection of lipopolysaccharide results in a rapid decline in ghrelin levels associated with a decrease in circulating GOAT protein concentration likely contributing to the reduced acylation in rats (53). Abdominal surgery associated with gastric inflammation (54) decreases acyl and des-acyl ghrelin levels (55, 56) and food intake (57) in rats, an effect blunted by rikkunshito, a herbal medicine stimulating the release of ghrelin (58). Central vagal stimulation, which normalizes the gastric inflammatory response (54), prevents the reduction of plasma ghrelin (55). Chronic inflammatory stress elicited by adjuvant-induced arthritis in rats or rheumatoid arthritis in humans reduces circulating ghrelin levels (59). There are also reports that a psychological stressor such as novelty stress in mice decreases plasma levels of ghrelin and food intake, alterations prevented by rikkunshito (60, 61). Chronic restraint stress or exposure to foot-shock downregulates ghrelin mRNA expression in the mouse hypothalamus (62) and reduces plasma levels of ghrelin in rats (63). These alterations were associated with decreased food intake and body weight gain in mice (62).
Whether the differential alterations of ghrelin by stressors reflect differences in species, metabolic status and/or stressor-related specific recruitment of central and/or peripheral signaling pathways regulating ghrelin release (56, 64) warrant further investigations. Moreover, it cannot be ruled out that difference in modalities to determine ghrelin (total vs. acyl, radioimmunoassay vs. enzyme-linked immunosorbent assay, commercial vs. custom-made kits) might also affect the levels reported.
However, while stressors modulate circulating ghrelin levels, there is also evidence that ghrelin stimulates the hypothalamic-pituitary adrenal axis (33). The peptide injected peripherally upregulates hypothalamic corticotropin-releasing factor (CRF) (33), a key peptide involved in the stress response (65). Recent studies indicate that ghrelin acts via the inhibition of hypothalamic GABAergic signaling on CRF neurons in the paraventricular nucleus of the hypothalamus (PVN) (66). In hypothalamic 4B cells in vitro, ghrelin stimulates CRF promoter activity through activation of protein kinase A and phospholipase C pathways resulting in increased CRF mRNA levels (67). These data suggest a bidirectional interaction between CRF and the ghrelin signaling system.
Nesfatin-1
Nesfatin-1 has been first detected in the rat hypothalamus as an 82-amino acid peptide derived from nucleobindin2 (NUCB2) (68). Subsequent research showed a more widespread brain distribution (69) as well as a 10-fold higher expression of NUCB2 mRNA in the stomach indicating that the upper gut is a major site of production (70). Interestingly, immunohistochemical double labeling showed that NUCB2/nesfatin-1 (the antibody recognizes both nesfatin-1 and the full length NUCB2) co-localizes with ghrelin indicating the production in the same gastric endocrine cell type, namely X/A-like cells in rats (70). This finding was later confirmed in humans where these cells are named P/D1 cells (14). Nesfatin-1 is able to reach the brain humorally but likely also acts via the vagus nerve as intraperitoneal injection induces Fos expression in neurons of the nucleus of the solitary tract that receives input from vagal afferents (71). However, the putative receptor mediating nesfatin-1's effects is still unknown. Converging evidence points toward a G protein-coupled receptor (72, 73). A recent autoradiographic study indicates widespread binding of 125I-labeled nesfatin-1 in the brain with signals in the cortex, PVN, area postrema, dorsal motor nucleus of the vagus nerve and cerebellum (74) supporting its centrally mediated pleiotropic effects (75).
The anorexigenic effect of nesfatin-1 has been early on described in several species including rats (68, 76), mice (77), chicks (78), and goldfish (79) following intracerebroventricular injection. In contrast to the convergent findings on the robust food intake-reducing effects of centrally injected nesfatin-1, only one study in mice reported an anorexigenic effect after acute intraperitoneal injection of nesfatin-1 at high doses (71), while other studies in rats (76) and mice (77) showed no effect. Similarly, data following chronic peripheral administration did not produce consistent results: while a reduction of food intake was observed in rats (80), no effect was detected in mice (81). Taken together, the effect of peripheral nesfatin-1 on food intake seems less robust and may not be the primary function of peripherally produced nesfatin-1. By contrast, consistent reports showed that the peptide may play an important role in glucose-stimulated pancreatic insulin release in rats (82) and humans (83).
Convergent findings support an involvement of nesfatin-1 in the stress response. First, several stressors activate nesfatin-1 immunoreactive neurons in the brain, namely psychological (restraint, water avoidance stress) (84–87), physical (abdominal surgery) (88), immunological (injection of lipopolysaccharide) (89) as well as a combination of stressors (chronic variable mild stress) (90). Second, water avoidance stress (91) and injection of lipopolysaccharide (92) elevate circulating levels of nesfatin-1 likely due to the release of the peptide associated with the upregulation of NUCB2 mRNA expression assessed by RT-qPCR and NUCB2/nesfatin-1 protein concentration measured by Western blot in the stomach (92). Third, intracerebroventricular injection of nesfatin-1 increases plasma adrenocorticotropic hormone (ACTH) and corticosterone in rats, an effect likely occurring in the hypothalamus as in vitro nesfatin-1 stimulates cytosolic Ca2+ in CRF-containing cells of the PVN (86). Therefore, nesfatin-1 exerts its stress-mediating effect likely via downstream CRF signaling. Lastly, circulating NUCB2/nesfatin-1 levels are positively correlated with perceived stress in a human female obese population (93) suggesting a potential role in the mediation of stress in humans as well. Interestingly, suicide victims showed altered NUCB2 mRNA expression in a midbrain nucleus implicated in stress-related mood alterations, the Edinger-Westphal nucleus, with an 1.8-fold increase in males and a 2.7-fold decrease in females compared to control subjects who died without any diagnosed neurodegenerative or psychiatric disorder (94).
Urocortins
Belonging to the CRF family, urocortins (Ucns) have been identified, namely Ucn1, a 40-amino acid (aa) peptide sharing 45% sequence identity with rat/human (r/h) CRF (95), Ucn2, a 39-aa peptide sharing 34% identity with r/h CRF and 42% with r/h Ucn1 (96, 97) and Ucn3, a 38-aa peptide sharing 26% homology with r/h CRF and 21% with r/h Ucn1 (98). Ucn1 binds to both CRF receptors, CRF1 and CRF2, with equal affinity, whereas Ucn2 and Ucn3 bind to the CRF2 receptor with high selectivity (99).
Besides their widespread brain distribution extensively reviewed elsewhere (100), Ucns are also expressed in the periphery, namely the heart, skeletal muscle, spleen, kidney, adipose tissue, ovary, skin (101) as well as the gastrointestinal tract including liver, pancreas, stomach, small, and large intestine (101–108).
Peripheral injection of Ucn1 inhibits food intake in different species including mice (109–111) and sheep (112). In rodents, Ucn1 reduces meal frequency and size and can induce conditioned taste aversion (113) and reduces body weight upon repeated peripheral administration (109). Reports showed that the food intake-reducing effect of Ucn1 is more potent compared to that of CRF, Ucn2, Ucn3, cholecystokinin (CCK) and leptin (109, 110, 113); moreover, a synergistic interaction between Ucn1 and CCK on satiety has been demonstrated (114). The anorexia induced by peripheral Ucn1 is mediated via the CRF2 receptor based on the observation that the selective CRF2 antagonists, antisauvagine-30 and astressin2-B, unlike selective CRF1 antagonists, suppress the Ucn1-induced reduction of food intake (110, 112, 115). The finding that CRF2 knockout mice do not display a reduction of food intake after intraperitoneal injection of Ucn1 further corroborates the implication of this receptor subtype (115). The mechanism through which peripherally injected Ucn1 influences food intake is still to be elucidated. It is unlikely to be mediated by vagal afferent signaling as capsaicin treatment did not alter the anorexigenic response of the peptide in mice (110). Moreover, Ucn1 barely enters the brain through the blood-brain barrier (116). However, CRF2 receptors are densely expressed in brain areas outside of the blood-brain barrier, namely the area postrema (117, 118), and neurons at this site are activated by peripheral Ucn1 (119) suggesting a possible pathway.
Ucn2 and Ucn3 injected peripherally also reduce ad libitum food intake during the dark phase as well as the refeeding response to a fast with Ucn2 being more potent compared to Ucn3 in mice (110, 111, 114, 115, 120), rats (113), and fish (121). The automated dark phase food intake monitoring showed that Ucn2 reduces meal size (increased satiation), while meal frequency (indicative of satiety) is not altered in mice (115). Interestingly, under re-feeding conditions after a fast, meal size is also reduced, however, meal frequency is increased (decreased satiety) (115). It is important to note that Ucn2, unlike Unc1, does not induce signs of taste aversion (113) pointing toward a specific food intake-reducing effect. Moreover, Ucn2 acts synergistically with CCK to reduce food intake, an effect also observed in vitro when recording gastric vagal afferent activity (114). This supports a vagal mode of transmission corroborated by the expression of the CRF2 receptor in the nodose ganglia (122, 123).
Various stressors upregulate the peripheral expression of Ucns. Injection of lipopolysaccharide, an immunological stressor, increases the expression of Ucn1, Ucn2 and Ucn3 mRNA in gastric mucosa and submucosa plus muscle layers (107) which is associated with the reduction of food intake under these conditions (53). Ucn1 and Ucn3 immunoreactivity in blood vessels and submucous neurons of the ileum is also increased following Schistosoma mansoni-induced inflammation (124). Likewise, blood monocyte-derived dendritic cells display largely increased Ucn1 mRNA and protein expression following stimulation with Bacteroides vulgatus or Fusobacterium varium (125). There is also evidence that psychological stressors (chronic social stress) upregulates Ucn2 mRNA expression in the pig colon (126), and maternal deprivation increases duodenal Ucn2 and CRF2 receptor mRNA, whereas CRF1 mRNA is decreased in rats (127).
Cholecystokinin
CCK is mainly produced in I cells scattered within the upper small intestine with more prominent distribution in the duodenum (128). These cells harbor the feature to be in direct contact with other cells via pseudopods (129). Several forms of CCK have been detected including CCK-5,−7,−8,−18,−22,−25,−33,−39, and−58 (representing the number of amino acids) with CCK-8 being the most commonly studied form (128). The demonstration that CCK-58 is the only form detected in the circulation when using a new method for blood processing suggests that the shorter forms are products of degradation (29).
The first described biological action of CCK was the stimulation of gallbladder contraction along with the stimulation of the production and release of pancreatic enzymes and secretion [for review see Sayegh (128)]. The food intake-suppressing effect of CCK was initially reported in rats, and later extended to rabbits, monkeys, pigs, sheep and humans [for review see Sayegh (128)]. Both forms of CCK, CCK-8 and CCK-58 were shown to decrease dark phase food intake following intraperitoneal injection in ad libitum fed rats by reducing meal size (130). However, CCK-58 does not shorten the subsequent inter-meal interval as observed following injection of CCK-8 providing evidence for a more sustained effect of CCK-58 (130). Moreover, CCK also suppresses gastric emptying in rats (131) and humans (132) contributing to its anorexigenic effect.
CCK interacts with two receptor subtypes, CCKA (alimentary), expressed in the gastrointestinal tract and on vagal afferents and CCKB (brain), predominantly expressed in the brain (133). CCK is postprandially released from duodenal I cells with lipids and proteins being the most potent stimulators (134–136). Released CCK binds to vagal CCKA expressing afferents and activates neurons in the nucleus of the solitary tract to inhibit food intake, with vagotomy abolishing both the CCK-induced neuronal activation in the brain (137) as well as the anorexigenic effect (138).
A combination of immunological stress using infection with Giardia lamblia and psychological stress using the water avoidance model increases CCK levels in the colonic mucosa of mice (139). The stress-induced visceral hypersensitivity could be blocked using the CCKA antagonist, L-364718, and the CCKB antagonist, L-365260 following psychological but not immunological stress (139) giving rise to a role of CCK in visceral sensitivity under selective stress conditions. By contrast, acute or chronic intraperitoneal injection of CCK exerts a protective effect on the impairment of memory functions under conditions of chronic restraint stress (140, 141). Moreover, OLETF rat pups lacking the CCKA display a higher separation-induced ultrasonic vocalization (142) as a surrogate for increased experience of stress. The link between the stress response and CCK signaling was further corroborated by the observation that a well-established immunological stressor, lipopolysaccharide, increases CCK mRNA expression in PVN CRF-containing neurons (143). Intraperitoneal injection of CCK stimulates neuronal activation in noradrenergic A2 neurons (144) as well as increases corticosterone levels to comparable magnitudes observed after injection of CRF (145). Also repetitive intracerebroventricular injections of cortagine, a CRF1 agonist, increases CCK mRNA as well as CCKB protein expression in the mouse amygdala and hippocampus resulting in heightened anxiety behavior as assessed using the elevated plus maze and open field test, an effect reversed by intracerebroventricular injection of the CCKB antagonist, LY225910 (146). This anxiety-inducing effect of CCK has also been observed in pharmacological provocation studies following intracerebroventricular injection of CCK-8 in rats that reduced exploratory behavior in the light/dark paradigm (147). In humans, intravenous injection of CCK-4 was shown to induce anxiety and panic symptoms (148, 149). Lastly, tail pinch stress-induced eating in rats (150) is reduced by intracerebroventricular injection of CCK-8 (151). Collectively these observations are indicative of a modulation of the stress response by CCK signaling.
Glucagon-Like Peptide 1
Glucagon-like peptide (GLP-1) is produced by endocrine L cells of the small intestine and processed into two biologically active forms, GLP-17−36 amide and GLP-17−37 (152) with GLP-17−36 amide being the predominant form in the human circulation (153). GLP-1 is released postprandially with a biphasic pattern: an early peak of GLP-1 secretion occurs ~15 min after meal intake that involves humoral (154, 155) and vagal (156) stimulation, while a later and larger peak is related to the direct contact of L cells with food components (157).
Peripheral but also central administration of GLP-1, in addition to the well-described incretin effect, results in a decrease of food intake in animals (157–159) and humans (160). In addition, the slowing of gastric and intestinal transit (161, 162) is likely to contribute to the food intake-reducing effect.
GLP-1 signals to the brain via the vagus nerve expressing the GLP-1 receptor (163) as shown by the suppression of the anorexigenic effect of peripherally injected GLP-1 by vagotomy (164, 165). It is to note that GLP-1 is also expressed in the brainstem nucleus of the solitary tract that projects to the PVN (166), and local knockdown of the pro-glucagon gene in the nucleus of the solitary tract increases food intake and also body weight gain (167). Since lesioning of these connections blunts the anorexigenic effect of peripherally injected GLP-1 (164) the gut-vagal-brainstem-hypothalamus connection is essential for the mediation of GLP-1's food intake-suppressing effect. Nonetheless, GLP-1 is able to cross the blood-brain barrier by simple diffusion (168). However, the rapid degradation of the peptide by circulating DPPIV (169) points toward the importance of neural and/or paracrine signaling.
GLP-1 can modulate a number of stress responses. Under basal conditions, GLP-17−36 amide injected peripherally stimulates circulating corticosterone levels in mice and rats as well as cortisol levels in healthy human subjects (170). Other studies showed that targeted knockdown of the GLP-1 receptor in single-minded 1-expressing neurons of the PVN reduces hypothalamic-pituitary-adrenal axis responses to acute and chronic stress and this was associated with reduced anxiety-like behavior and a prevention of body weight reduction under conditions of chronic stress (171). Similarly, injection of the GLP-1 receptor inverse agonist, exendin-(9−39) into the dorsal subregion of the lateral septum blocks the acute restraint stress-induced anorexigenic effect in rats (172). While these studies support GLP-1's permissive role in the activation of stress signaling pathways, other reports showed that mice lacking the GLP-1 receptor display an increased corticosterone response to novel environment stress (173).
Several protective effects of GLP-1 have been reported under conditions of stress. GLP-1 injected intracerebroventricularly prevents gastric mucosal lesions induced by a combination of cold and restraint stress, an effect blocked by exendin-(9−39) (174). Subcutaneous injection of liraglutide, a GLP-1 analog, inhibits visceral allodynia induced by injection of lipopolysaccharide or repeated water avoidance stress (175). In humans with alcohol dependence, treatment with the GABA-B receptor agonist, baclofen at a dose of 30 mg/day increases circulating levels of GLP-1 (176), possibly associated with a reduced craving for alcohol. Moreover, GLP-1 receptor activation reverses the restraint stress-induced activation of bone marrow sca-1highc-KithighCD48lowCD150high proliferation of hematopoietic stem cells in mice, thereby reducing the inflammatory response (177). In a study using geniposide as GLP-1 agonist these anti-inflammatory effects were associated with an amelioration of depression-like behaviors following repeated restraint stress (178). Also in vitro GLP-17−36 prevents various stressors (e.g., H2O2 and amyloid β1−42)-induced death of murine hippocampal HT22 cells, an effect likely mediated via increased phosphorylation of Akt and ERK1/2 (179).
Peptide YY
Peptide YY (PYY) is derived from L cells located in the distal small intestine and colon (180). The peptide circulates in two forms, PYY1−36 and PYY3−36, which is the predominant form in the blood (181) resulting from the processing by dipeptidyl peptidase IV (182). PYY is well established to reduce food intake in animals and humans following peripheral injection via binding to the Y2 receptor (183). This was demonstrated by the blunting of the peptide's anorexigenic effect by the Y2 antagonist, BIIE0246 (184) and knockout of the Y2 receptor (183) in rodents. The anorexigenic gut-brain mode of action may involve the vagus nerve and humoral pathways. The Y2 receptor is expressed on vagal afferents (185) and vagotomy blocks the anorexigenic effect of PYY (186) In addition, PYY can also cross the blood-brain barrier in a non-saturable manner (187). There is evidence that peripherally injected PYY or PYY3−36 activates brain nuclei such as the nucleus of the solitary tract (188) and hypothalamic nuclei (189) which are known to regulate food intake. In the brain, PYY microinjected directly into the arcuate nucleus, a nucleus involved in the regulation of food intake and expressing the Y2 receptor (190), reduces food intake. This is achieved by decreasing the activity of neuropeptide Y-containing neurons and activating proopiomelanocortin-containing cells (183).
Repetitive water avoidance stress decreases circulating PYY levels compared to non-stressed rats (191). Likewise, in humans a well-established psychological stressor, the Trier social stress test, reduces circulating PYY levels in normal weight and obese women (192). However, in mice, water immersion stress results in increased plasma PYY levels (193). Other studies showed that mice lacking PYY have an enhanced restraint stress-induced fecal pellet output and upper gastrointestinal transit (194); therefore, the peptide might play a modulatory role in the stress response. Whether the contrasting effects of stress on PYY release are related to species or stress modality differences remains to be further investigated.
Summary
Various stressors alter the expression or circulating levels of several gut-brain peptidergic hormones involved in the regulation of hunger and satiety. While most anorexigenic peptides are upregulated under conditions of stress (nesfatin-1, Ucns, and CCK), others were shown to be differentially regulated dependent on the type of stressors (ghrelin and PYY), and for GLP-1 conclusive data are lacking so far. In addition, there is a further activation of the hypothalamus-pituitary-adrenal axis induced by specific gut peptides (ghrelin, nesfatin-1, CCK, and GLP-1) acting via neuronal and/or humoral gut-brain signaling highlighting the PVN as key responsive area orchestrating the stress response. This results in an increased perception of stress (nesfatin-1) and an alteration of anxiety and depressiveness (ghrelin, CCK, and GLP-1) with the PVN and Edinger-Westphal nucleus playing an important role in the behavioral responses. While most peptides contribute to stress-induced anorexia (Ucns, CCK, and GLP-1), ghrelin can stimulate food intake under these conditions (Figure 1). Despite the fact that our knowledge on these regulatory pathways greatly increased during the past years, the interactions between these peptides (114, 195) under stress conditions should be further investigated along with the possible translation of these findings—derived mainly from animal models—to humans.
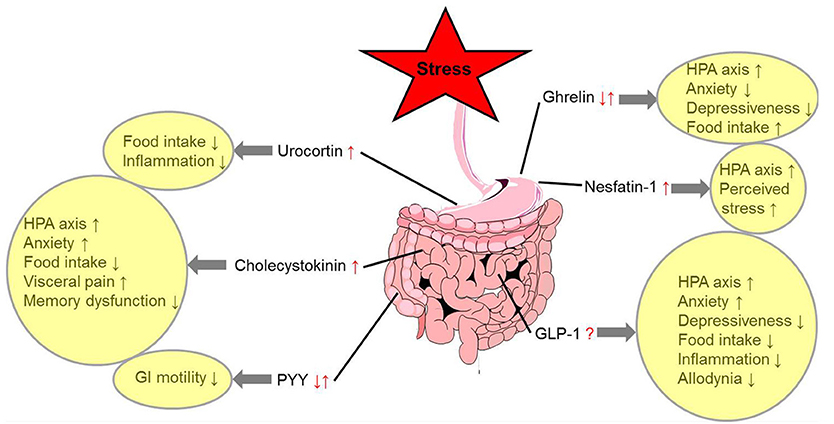
Figure 1. Alterations of gut-brain peptides under conditions of stress and functional implications. ↓, decrease; ↑, increase; ?, unknown effect; GLP-1, glucagon-like peptide 1; GI, gastrointestinal; HPA axis, hypothalamus-pituitary-adrenal axis; PYY, peptide YY.
Author Contributions
AS drafted the manuscript. AS and YT reviewed and finalized the manuscript.
Conflict of Interest Statement
The authors declare that the research was conducted in the absence of any commercial or financial relationships that could be construed as a potential conflict of interest.
Acknowledgments
This work was supported by funding of the German Research Foundation STE 1765/3-2, Charité University Funding UFF 89/441-176 (AS) and National Institute of Health grant DK-41301 (Animal Model Core) and VA Senior Career Scientist Award (YT).
References
1. Cummings DE, Overduin J. Gastrointestinal regulation of food intake. J Clin Invest. (2007) 117:13–23. doi: 10.1172/JCI30227
2. Dockray GJ. Gastrointestinal hormones and the dialogue between gut and brain. J Physiol. (2014) 592:2927–41. doi: 10.1113/jphysiol.2014.270850
3. de Lartigue G, Diepenbroek C. Novel developments in vagal afferent nutrient sensing and its role in energy homeostasis. Curr Opin Pharmacol. (2016) 31:38–43. doi: 10.1016/j.coph.2016.08.007
4. de Lartigue G. Putative roles of neuropeptides in vagal afferent signaling. Physiol Behav. (2014) 136:155–69. doi: 10.1016/j.physbeh.2014.03.011
5. Prinz P, Stengel A. Control of food intake by gastrointestinal peptides: mechanisms of action and possible modulation in the treatment of obesity. J Neurogastroenterol Motil. (2017) 23:180–96. doi: 10.5056/jnm16194
6. Stengel A, Wang L, Taché Y. Stress-related alterations of acyl and desacyl ghrelin circulating levels: mechanisms and functional implications. Peptides (2011) 32:2208–17. doi: 10.1016/j.peptides.2011.07.002
7. Kojima M, Hosoda H, Date Y, Nakazato M, Matsuo H, Kangawa K. Ghrelin is a growth-hormone-releasing acylated peptide from stomach. Nature (1999) 402:656–60. doi: 10.1038/45230
8. Ariyasu H, Takaya K, Tagami T, Ogawa Y, Hosoda K, Akamizu T, et al. Stomach is a major source of circulating ghrelin, and feeding state determines plasma ghrelin-like immunoreactivity levels in humans. J Clin Endocrinol Metab. (2001) 86:4753–8. doi: 10.1210/jcem.86.10.7885
9. Date Y, Kojima M, Hosoda H, Sawaguchi A, Mondal MS, Suganuma T, et al. Ghrelin, a novel growth hormone-releasing acylated peptide, is synthesized in a distinct endocrine cell type in the gastrointestinal tracts of rats and humans. Endocrinology (2000) 141:4255–61. doi: 10.1210/endo.141.11.7757
10. Davenport AP, Bonner TI, Foord SM, Harmar AJ, Neubig RR, Pin JP, et al. International union of pharmacology. LVI Ghrelin receptor nomenclature, distribution, and function Pharmacol Rev. (2005) 57:541–6. doi: 10.1124/pr.57.4.1
11. Gutierrez JA, Solenberg PJ, Perkins DR, Willency JA, Knierman MD, Jin Z, et al. Ghrelin octanoylation mediated by an orphan lipid transferase. Proc Natl Acad Sci USA. (2008) 105:6320–5. doi: 10.1073/pnas.0800708105
12. Yang J, Brown MS, Liang G, Grishin NV, Goldstein JL. Identification of the acyltransferase that octanoylates ghrelin, an appetite-stimulating peptide hormone. Cell (2008) 132:387–96. doi: 10.1016/j.cell.2008.01.017
13. Stengel A, Goebel M, Wang L, Taché Y, Sachs G, Lambrecht NW. Differential distribution of ghrelin-O-acyltransferase (GOAT) immunoreactive cells in the mouse and rat gastric oxyntic mucosa. Biochem Biophys Res Commun. (2010) 392:67–71. doi: 10.1016/j.bbrc.2009.12.169
14. Stengel A, Hofmann T, Goebel-Stengel M, Lembke V, Ahnis A, Elbelt U, et al. Ghrelin and NUCB2/nesfatin-1 are expressed in the same gastric cell and differentially correlated with body mass index in obese subjects. Histochem Cell Biol. (2013) 139:909–18. doi: 10.1007/s00418-013-1087-8
15. An W, Li Y, Xu G, Zhao J, Xiang X, Ding L, et al. Modulation of ghrelin O-acyltransferase expression in pancreatic islets. Cell Physiol Biochem. (2010) 26:707–16. doi: 10.1159/000322338
16. Goebel-Stengel M, Hofmann T, Elbelt U, Teuffel P, Ahnis A, Kobelt P, et al. The ghrelin activating enzyme ghrelin-O-acyltransferase (GOAT) is present in human plasma and expressed dependent on body mass index. Peptides (2013) 43:13–9. doi: 10.1016/j.peptides.2013.02.011
17. Wren AM, Small CJ, Ward HL, Murphy KG, Dakin CL, Taheri S, et al. The novel hypothalamic peptide ghrelin stimulates food intake and growth hormone secretion. Endocrinology (2000) 141:4325–8. doi: 10.1210/endo.141.11.7873
18. Wren AM, Seal LJ, Cohen MA, Brynes AE, Frost GS, Murphy KG, et al. Ghrelin enhances appetite and increases food intake in humans. J Clin Endocrinol Metab. (2001) 86:5992. doi: 10.1210/jcem.86.12.8111
19. Tschöp M, Smiley DL, Heiman ML. Ghrelin induces adiposity in rodents. Nature (2000) 407:908–13. doi: 10.1038/35038090
20. Date Y, Murakami N, Toshinai K, Matsukura S, Niijima A, Matsuo H, et al. The role of the gastric afferent vagal nerve in ghrelin-induced feeding and growth hormone secretion in rats. Gastroenterology (2002) 123:1120–8. doi: 10.1053/gast.2002.35954
21. Okada T, Waise TMZ, Toshinai K, Mita Y, Sakoda H, Nakazato M. Analysis of peripheral ghrelin signaling via the vagus nerve in ghrelin receptor-restored GHSR-null mice. Neurosci Lett. (2018) 681:50–5. doi: 10.1016/j.neulet.2018.05.035
22. Banks WA, Tschöp M, Robinson SM, Heiman ML. Extent and direction of ghrelin transport across the blood-brain barrier is determined by its unique primary structure. J Pharmacol Exp Ther. (2002) 302:822–7. doi: 10.1124/jpet.102.034827
23. Guan XM, Yu H, Palyha OC, Mckee KK, Feighner SD, Sirinathsinghji DJ, et al. Distribution of mRNA encoding the growth hormone secretagogue receptor in brain and peripheral tissues. Brain Res Mol Brain Res. (1997) 48:23–9.
24. Willesen MG, Kristensen P, Romer J. Co-localization of growth hormone secretagogue receptor and NPY mRNA in the arcuate nucleus of the rat. Neuroendocrinology (1999) 70:306–16. doi: 10.1159/000054491
25. Cowley MA, Smith RG, Diano S, Tschöp M, Pronchuk N, Grove KL, et al. The distribution and mechanism of action of ghrelin in the CNS demonstrates a novel hypothalamic circuit regulating energy homeostasis. Neuron (2003) 37:649–61. doi: 10.1016/S0896-6273(03)00063-1
26. Zhang X, van den Pol AN. Hypothalamic arcuate nucleus tyrosine hydroxylase neurons play orexigenic role in energy homeostasis. Nat Neurosci. (2016) 19:1341–7. doi: 10.1038/nn.4372
27. Chen HY, Trumbauer ME, Chen AS, Weingarth DT, Adams JR, Frazier EG, et al. Orexigenic action of peripheral ghrelin is mediated by neuropeptide Y and agouti-related protein. Endocrinology (2004) 145:2607–12. doi: 10.1210/en.2003-1596
28. Patterson M, Murphy KG, Le Roux CW, Ghatei MA, Bloom SR. Characterization of ghrelin-like immunoreactivity in human plasma. J Clin Endocrinol Metab. (2005) 90:2205–11. doi: 10.1210/jc.2004-1641
29. Stengel A, Keire D, Goebel M, Evilevitch L, Wiggins B, Taché Y, et al. The RAPID method for blood processing yields new insight in plasma concentrations and molecular forms of circulating gut peptides. Endocrinology (2009) 150:5113–8. doi: 10.1210/en.2009-0697
30. Mahbod P, Smith EP, Fitzgerald ME, Morano RL, Packard BA, Ghosal S, et al. Desacyl ghrelin decreases anxiety-like behavior in male mice. Endocrinology (2018) 159:388–99. doi: 10.1210/en.2017-00540
31. Inhoff T, Mönnikes H, Noetzel S, Stengel A, Goebel M, Dinh QT, et al. Desacyl ghrelin inhibits the orexigenic effect of peripherally injected ghrelin in rats. Peptides (2008) 29:2159–68. doi: 10.1016/j.peptides.2008.09.014
32. Fernandez G, Cabral A, Cornejo MP, De Francesco PN, Garcia-Romero G, Reynaldo M, et al. Des-Acyl ghrelin directly targets the arcuate nucleus in a ghrelin-receptor independent manner and impairs the orexigenic effect of ghrelin. J Neuroendocrinol. (2016) 28:12349. doi: 10.1111/jne.12349
33. Asakawa A, Inui A, Kaga T, Yuzuriha H, Nagata T, Fujimiya M, et al. A role of ghrelin in neuroendocrine and behavioral responses to stress in mice. Neuroendocrinology (2001) 74:143–7. doi: 10.1159/000054680
34. Kristenssson E, Sundqvist M, Astin M, Kjerling M, Mattsson H, Dornonville De La Cour C, et al. Acute psychological stress raises plasma ghrelin in the rat. Regul Pept. (2006) 134:114–7. doi: 10.1016/j.regpep.2006.02.003
35. Rouach V, Bloch M, Rosenberg N, Gilad S, Limor R, Stern N, et al. The acute ghrelin response to a psychological stress challenge does not predict the post-stress urge to eat. Psychoneuroendocrinology (2007) 32:693–702. doi: 10.1016/j.psyneuen.2007.04.010
36. Maruna P, Gurlich R, Rosicka M. Ghrelin as an acute-phase reactant during postoperative stress response. Horm Metab Res. (2008) 40:404–9. doi: 10.1055/s-2008-1065329
37. Raspopow K, Abizaid A, Matheson K, Anisman H. Psychosocial stressor effects on cortisol and ghrelin in emotional and non-emotional eaters: influence of anger and shame. Horm Behav. (2010) 58:677–84. doi: 10.1016/j.yhbeh.2010.06.003
38. Kontoravdis N, Vassilikostas G, Lagoudianakis E, Pappas A, Seretis C, Panagiotopoulos N, et al. Effect of acute surgical stress on serum ghrelin levels. Gastroenterology Res. (2012) 5:97–102. doi: 10.4021/gr455e
39. Geliebter A, Carnell S, Gluck ME. Cortisol and ghrelin concentrations following a cold pressor stress test in overweight individuals with and without night eating. Int J Obes. (2013) 37:1104–8. doi: 10.1038/ijo.2012.166
40. Jung YS, Kim MY, Lee HS, Park SL, Lee KJ. Effect of DA-9701, a novel prokinetic agent, on stress-induced delayed gastric emptying and hormonal changes in rats. Neurogastroenterol Motil. (2013) 25:254–259:e166. doi: 10.1111/nmo.12053
41. Gluck ME, Yahav E, Hashim SA, Geliebter A. Ghrelin levels after a cold pressor stress test in obese women with binge eating disorder. Psychosom Med. (2014) 76:74–9. doi: 10.1097/PSY.0000000000000018
42. Wang L, Goebel-Stengel M, Yuan PQ, Stengel A, Taché Y. Corticotropin-releasing factor overexpression in mice abrogates sex differences in body weight, visceral fat, and food intake response to a fast and alters levels of feeding regulatory hormones. Biol Sex Differ (2017) 8:2. doi: 10.1186/s13293-016-0122-6
43. Carnell S, Grillot C, Ungredda T, Ellis S, Mehta N, Holst J, et al. Morning and afternoon appetite and gut hormone responses to meal and stress challenges in obese individuals with and without binge eating disorder. Int J Obes. (2018) 42:841–9. doi: 10.1038/ijo.2017.307
44. Lutter M, Sakata I, Osborne-Lawrence S, Rovinsky SA, Anderson JG, Jung S, et al. The orexigenic hormone ghrelin defends against depressive symptoms of chronic stress. Nat Neurosci. (2008) 11:752–3. doi: 10.1038/nn.2139
45. Zheng J, Dobner A, Babygirija R, Ludwig K, Takahashi T. Effects of repeated restraint stress on gastric motility in rats. Am J Physiol Regul Integr Comp Physiol. (2009) 296:R1358–1365. doi: 10.1152/ajpregu.90928.2008
46. Chuang JC, Perello M, Sakata I, Osborne-Lawrence S, Savitt JM, Lutter M, et al. Ghrelin mediates stress-induced food-reward behavior in mice. J Clin Invest. (2011) 121:2684–92. doi: 10.1172/JCI57660
47. Patterson ZR, Khazall R, Mackay H, Anisman H, Abizaid A. Central ghrelin signaling mediates the metabolic response of C57BL/6 male mice to chronic social defeat stress. Endocrinology (2013) 154:1080–91. doi: 10.1210/en.2012-1834
48. Elbassuoni EA. Gender differences in ghrelin response to chronic immobilization stress in rats: possible role of estrogen. Gen Physiol Biophys. (2014) 33:111–20. doi: 10.4149/gpb_2013061
49. Huang HJ, Zhu XC, Han QQ, Wang YL, Yue N, Wang J, et al. Ghrelin alleviates anxiety- and depression-like behaviors induced by chronic unpredictable mild stress in rodents. Behav Brain Res. (2017) 326:33–43. doi: 10.1016/j.bbr.2017.02.040
50. Yousufzai M, Harmatz ES, Shah M, Malik MO, Goosens KA. Ghrelin is a persistent biomarker for chronic stress exposure in adolescent rats and humans. Transl Psychiatry (2018) 8:74. doi: 10.1038/s41398-018-0135-5
51. Labarthe A, Fiquet O, Hassouna R, Zizzari P, Lanfumey L, Ramoz N, et al. Ghrelin-derived peptides: a link between appetite/reward, GH axis, and psychiatric disorders? Front Endocrinol. (2014) 5:163. doi: 10.3389/fendo.2014.00163
52. Morin V, Hozer F, Costemale-Lacoste JF. The effects of ghrelin on sleep, appetite, and memory, and its possible role in depression: a review of the literature. Encephale (2018) 44:256–63. doi: 10.1016/j.encep.2017.10.012
53. Stengel A, Goebel M, Wang L, Reeve JR Jr, Taché Y, Lambrecht NW. Lipopolysaccharide differentially decreases plasma acyl and desacyl ghrelin levels in rats: potential role of the circulating ghrelin-acylating enzyme GOAT. Peptides (2010) 31:1689–96. doi: 10.1016/j.peptides.2010.06.015
54. Yuan PQ, Taché Y. Abdominal surgery induced gastric ileus and activation of M1-like macrophages in the gastric myenteric plexus: prevention by central vagal activation in rats. Am J Physiol Gastrointest Liver Physiol. (2017) 313:G320–29. doi: 10.1152/ajpgi.00121.2017
55. Stengel A, Goebel M, Luckey A, Yuan PQ, Wang L, Taché Y. Cold ambient temperature reverses abdominal surgery-induced delayed gastric emptying and decreased plasma ghrelin levels in rats. Peptides (2010) 31:2229–35. doi: 10.1016/j.peptides.2010.08.026
56. Stengel A, Goebel-Stengel M, Wang L, Shaikh A, Lambrecht NW, Rivier J, et al. Abdominal surgery inhibits circulating acyl ghrelin and ghrelin-O-acyltransferase levels in rats: role of the somatostatin receptor subtype 2. Am J Physiol Gastrointest Liver Physiol. (2011) 301:G239–48. doi: 10.1152/ajpgi.00018.2011
57. Stengel A, Goebel-Stengel M, Wang L, Luckey A, Hu E, Rivier J, et al. Central administration of pan-somatostatin agonist ODT8-SST prevents abdominal surgery-induced inhibition of circulating ghrelin, food intake and gastric emptying in rats. Neurogastroenterol Motil. (2011) 23:e294–308. doi: 10.1111/j.1365-2982.2011.01721.x
58. Wang L, Mogami S, Yakabi S, Karasawa H, Yamada C, Yakabi K, et al. Patterns of brain activation and meal reduction induced by abdominal surgery in mice and modulation by rikkunshito. PLoS ONE (2015) 10:e0139325. doi: 10.1371/journal.pone.0139325
59. Otero M, Nogueiras R, Lago F, Dieguez C, Gomez-Reino JJ, Gualillo O. Chronic inflammation modulates ghrelin levels in humans and rats. Rheumatology (2004) 43:306–10. doi: 10.1093/rheumatology/keh055
60. Saegusa Y, Takeda H, Muto S, Nakagawa K, Ohnishi S, Sadakane C, et al. Decreased plasma ghrelin contributes to anorexia following novelty stress. Am J Physiol Endocrinol Metab. (2011) 301:E685–696. doi: 10.1152/ajpendo.00121.2011
61. Yamada C, Saegusa Y, Nakagawa K, Ohnishi S, Muto S, Nahata M, et al. Rikkunshito, a Japanese kampo medicine, ameliorates decreased feeding behavior via ghrelin and serotonin 2B receptor signaling in a novelty stress murine model. Biomed Res Int. (2013) 2013:792940. doi: 10.1155/2013/792940
62. Jeong JY, Lee DH, Kang SS. Effects of chronic restraint stress on body weight, food intake, and hypothalamic gene expressions in mice. Endocrinol Metab. (2013) 28:288–96. doi: 10.3803/EnM.2013.28.4.288
63. Rostamkhani F, Zardooz H, Goshadrou F, Baveisi M, Hedayati M. Stress increased ghrelin secretion from pancreatic isolated islets in male rats. Gen Physiol Biophys. (2016) 35:109–17. doi: 10.4149/gpb_2015037
64. Ibrahim Abdalla MM. Ghrelin - physiological functions and regulation. Eur Endocrinol. (2015) 11:90–5. doi: 10.17925/EE.2015.11.02.90
65. Bale TL, Vale WW. CRF and CRF receptors: role in stress responsivity and other behaviors. Annu Rev Pharmacol Toxicol. (2004) 44:525–57. doi: 10.1146/annurev.pharmtox.44.101802.121410
66. Cabral A, Portiansky E, Sanchez-Jaramillo E, Zigman JM, Perello M. Ghrelin activates hypophysiotropic corticotropin-releasing factor neurons independently of the arcuate nucleus. Psychoneuroendocrinology (2016) 67:27–39. doi: 10.1016/j.psyneuen.2016.01.027
67. Kageyama K, Kumata Y, Akimoto K, Takayasu S, Tamasawa N, Suda T. Ghrelin stimulates corticotropin-releasing factor and vasopressin gene expression in rat hypothalamic 4B cells. Stress (2011) 14:520–9. doi: 10.3109/10253890.2011.558605
68. Oh IS, Shimizu H, Satoh T, Okada S, Adachi S, Inoue K, et al. Identification of nesfatin-1 as a satiety molecule in the hypothalamus. Nature (2006) 443:709–12. doi: 10.1038/nature05162
69. Goebel M, Stengel A, Wang L, Lambrecht NW, Taché Y. Nesfatin-1 immunoreactivity in rat brain and spinal cord autonomic nuclei. Neurosci Lett. (2009) 452:241–46. doi: 10.1016/j.neulet.2009.01.064.
70. Stengel A, Goebel M, Yakubov I, Wang L, Witcher D, Coskun T, et al. Identification and characterization of nesfatin-1 immunoreactivity in endocrine cell types of the rat gastric oxyntic mucosa. Endocrinology (2009) 150:232–8. doi: 10.1210/en.2008-0747
71. Shimizu H, Oh IS, Hashimoto K, Nakata M, Yamamoto S, Yoshida N, et al. Peripheral administration of nesfatin-1 reduces food intake in mice: the leptin-independent mechanism. Endocrinology (2009) 150:662–71. doi: 10.1210/en.2008-0598
72. Brailoiu GC, Dun SL, Brailoiu E, Inan S, Yang J, Chang JK, et al. Nesfatin-1: distribution and interaction with a G protein-coupled receptor in the rat brain. Endocrinology (2007) 148:5088–94. doi: 10.1210/en.2007-0701
73. Ozcan M, Gok ZB, Kacar E, Serhatlioglu I, Kelestimur H. Nesfatin-1 increases intracellular calcium concentration by protein kinase C activation in cultured rat dorsal root ganglion neurons. Neurosci Lett. (2016) 619:177–81. doi: 10.1016/j.neulet.2016.03.018
74. Prinz P, Goebel-Stengel M, Teuffel P, Rose M, Klapp BF, Stengel A. Peripheral and central localization of the nesfatin-1 receptor using autoradiography in rats. Biochem Biophys Res Commun. (2016) 470:521–7. doi: 10.1016/j.bbrc.2016.01.113
75. Dore R, Levata L, Lehnert H, Schulz C. Nesfatin-1: functions and physiology of a novel regulatory peptide. J Endocrinol. (2017) 232:R45–65. doi: 10.1530/JOE-16-0361
76. Stengel A, Goebel M, Wang L, Rivier J, Kobelt P, Mönnikes H, et al. Central nesfatin-1 reduces dark-phase food intake and gastric emptying in rats: differential role of corticotropin-releasing factor2 receptor. Endocrinology (2009) 150:4911–9. doi: 10.1210/en.2009-0578
77. Goebel M, Stengel A, Wang L, Taché Y. Central nesfatin-1 reduces the nocturnal food intake in mice by reducing meal size and increasing inter-meal intervals. Peptides (2011) 32, 36–43. doi: 10.1016/j.peptides.2010.09.027
78. Heidarzadeh H, Zendehdel M, Babapour V, Gilanpour H. The effect of Nesfatin-1 on food intake in neonatal chicks: role of CRF1 /CRF2 and H1/ H3 receptors. Vet Res Commun. (2018) 42:39–47. doi: 10.1007/s11259-017-9706-9
79. Kerbel B, Unniappan S. Nesfatin-1 suppresses energy intake, co-localises ghrelin in the brain and gut, and alters ghrelin, cholecystokinin and orexin mRNA expression in goldfish. J Neuroendocrinol. (2012) 24:366–77. doi: 10.1111/j.1365-2826.2011.02246.x
80. Mortazavi S, Gonzalez R, Ceddia R, Unniappan S. Long-term infusion of nesfatin-1 causes a sustained regulation of whole-body energy homeostasis of male Fischer 344 rats. Front Cell Dev Biol. (2015) 3:22. doi: 10.3389/fcell.2015.00022
81. Yin Y, Li Z, Gao L, Li Y, Zhao J, Zhang W. AMPK-dependent modulation of hepatic lipid metabolism by nesfatin-1. Mol Cell Endocrinol. (2015) 417:20–6. doi: 10.1016/j.mce.2015.09.006
82. Gonzalez R, Reingold BK, Gao X, Gaidhu MP, Tsushima RG, Unniappan S. Nesfatin-1 exerts a direct, glucose-dependent insulinotropic action on mouse islet beta- and MIN6 cells. J Endocrinol. (2011) 208:R9–16. doi: 10.1530/JOE-10-0492
83. Riva M, Nitert MD, Voss U, Sathanoori R, Lindqvist A, Ling C, et al. Nesfatin-1 stimulates glucagon and insulin secretion and beta cell NUCB2 is reduced in human type 2 diabetic subjects. Cell Tissue Res. (2011) 346:393–405. doi: 10.1007/s00441-011-1268-5
84. Goebel M, Stengel A, Wang L, Taché Y. Restraint stress activates nesfatin-1-immunoreactive brain nuclei in rats. Brain Res. (2009) 1300:114–124. doi: 10.1016/j.brainres.2009.08.082
85. Okere B, Xu L, Roubos EW, Sonetti D, Kozicz T. Restraint stress alters the secretory activity of neurons co-expressing urocortin-1, cocaine- and amphetamine-regulated transcript peptide and nesfatin-1 in the mouse Edinger-Westphal nucleus. Brain Res. (2010) 1317:92–9. doi: 10.1016/j.brainres.2009.12.053
86. Yoshida N, Maejima Y, Sedbazar U, Ando A, Kurita H, Damdindorj B, et al. Stressor-responsive central nesfatin-1 activates corticotropin-releasing hormone, noradrenaline and serotonin neurons and evokes hypothalamic-pituitary-adrenal axis. Aging (Albany NY) (2010) 2:775–84. doi: 10.18632/aging.100207
87. Goebel-Stengel M, Wang L, Stengel A, Taché Y. Localization of nesfatin-1 neurons in the mouse brain and functional implication. Brain Res. (2011) 1396:20–34. doi: 10.1016/j.brainres.2011.04.031
88. Stengel A, Goebel M, Wang L, Taché Y. Abdominal surgery activates nesfatin-1 immunoreactive brain nuclei in rats. Peptides (2010) 31:263–270. doi: 10.1016/j.peptides.2009.11.015
89. Bonnet MS, Pecchi E, Trouslard J, Jean A, Dallaporta M, Troadec JD. Central nesfatin-1-expressing neurons are sensitive to peripheral inflammatory stimulus. J Neuroinflammation (2009) 6:27. doi: 10.1186/1742-2094-6-27
90. Xu L, Bloem B, Gaszner B, Roubos EW, Kozicz T. Stress-related changes in the activity of cocaine- and amphetamine-regulated transcript and nesfatin neurons in the midbrain non-preganglionic Edinger-Westphal nucleus in the rat. Neuroscience (2010) 170:478–88. doi: 10.1016/j.neuroscience.2010.07.001
91. Xu YY, Ge JF, Qin G, Peng YN, Zhang CF, Liu XR, et al. Acute, but not chronic, stress increased the plasma concentration and hypothalamic mRNA expression of NUCB2/nesfatin-1 in rats. Neuropeptides (2015) 54:47–53. doi: 10.1016/j.npep.2015.08.003
92. Stengel A, Goebel-Stengel M, Jawien J, Kobelt P, Taché Y, Lambrecht NW. Lipopolysaccharide increases gastric and circulating NUCB2/nesfatin-1 concentrations in rats. Peptides (2011) 32:1942–7. doi: 10.1016/j.peptides.2011.07.006
93. Hofmann T, Stengel A, Ahnis A, Busse P, Elbelt U, Klapp BF. NUCB2/nesfatin-1 is associated with elevated scores of anxiety in female obese patients. Psychoneuroendocrinology (2013) 38:2502–10. doi: 10.1016/j.psyneuen.2013.05.013
94. Bloem B, Xu L, Morava E, Faludi G, Palkovits M, Roubos EW, et al. Sex-specific differences in the dynamics of cocaine- and amphetamine-regulated transcript and nesfatin-1 expressions in the midbrain of depressed suicide victims vs. controls. Neuropharmacology (2012) 62:297–303. doi: 10.1016/j.neuropharm.2011.07.023
95. Vaughan J, Donaldson C, Bittencourt J, Perrin MH, Lewis K, Sutton S, et al. Urocortin, a mammalian neuropeptide related to fish urotensin I and to corticotropin-releasing factor. Nature (1995) 378:287–92. doi: 10.1038/378287a0
96. Reyes TM, Lewis K, Perrin MH, Kunitake KS, Vaughan J, Arias CA, et al. Urocortin II: a member of the corticotropin-releasing factor (CRF) neuropeptide family that is selectively bound by type 2 CRF receptors. Proc Natl Acad Sci USA. (2001) 98:2843–8. doi: 10.1073/pnas.051626398
97. Vaughan JM, Donaldson CJ, Fischer WH, Perrin MH, Rivier JE, Sawchenko PE, et al. Posttranslational processing of human and mouse urocortin 2: characterization and bioactivity of gene products. Endocrinology (2013) 154:1553–64. doi: 10.1210/en.2012-2011
98. Lewis K, Li C, Perrin MH, Blount A, Kunitake K, Donaldson C, et al. Identification of urocortin III, an additional member of the corticotropin-releasing factor (CRF) family with high affinity for the CRF2 receptor. Proc Natl Acad Sci USA. (2001) 98:7570–5. doi: 10.1073/pnas.121165198
99. Grace CR, Perrin MH, Cantle JP, Vale WW, Rivier JE, Riek R. Common and divergent structural features of a series of corticotropin releasing factor-related peptides. J Am Chem Soc. (2007) 129:16102–14. doi: 10.1021/ja0760933
100. Stengel A, Taché Y. CRF and urocortin peptides as modulators of energy balance and feeding behavior during stress. Front Neurosci. (2014) 8:52. doi: 10.3389/fnins.2014.00052
101. Boorse GC, Denver RJ. Widespread tissue distribution and diverse functions of corticotropin-releasing factor and related peptides. Gen Comp Endocrinol. (2006) 146:9–18. doi: 10.1016/j.ygcen.2005.11.014
102. Kozicz T, Arimura A. Distribution of urocortin in the rat's gastrointestinal tract and its colocalization with tyrosine hydroxylase. Peptides (2002) 23:515–21. doi: 10.1016/S0196-9781(01)00639-8
103. Li C, Chen P, Vaughan J, Blount A, Chen A, Jamieson PM, et al. Urocortin III is expressed in pancreatic beta-cells and stimulates insulin and glucagon secretion. Endocrinology (2003) 144:3216–24. doi: 10.1210/en.2002-0087
104. Porcher C, Peinnequin A, Pellissier S, Meregnani J, Sinniger V, Canini F, et al. Endogenous expression and in vitro study of CRF-related peptides and CRF receptors in the rat gastric antrum. Peptides (2006) 27:1464–75. doi: 10.1016/j.peptides.2005.10.023
105. Kimura T, Amano T, Uehara H, Ariga H, Ishida T, Torii A, et al. Urocortin I is present in the enteric nervous system and exerts an excitatory effect via cholinergic and serotonergic pathways in the rat colon. Am J Physiol Gastrointest Liver Physiol. (2007) 293:G903–10. doi: 10.1152/ajpgi.00066.2007
106. van der Meulen T, Xie R, Kelly OG, Vale WW, Sander M, Huising MO. Urocortin 3 marks mature human primary and embryonic stem cell-derived pancreatic alpha and beta cells. PLoS ONE (2012) 7:e52181. doi: 10.1371/journal.pone.0052181
107. Yuan PQ, Wu SV, Taché Y. Urocortins and CRF type 2 receptor isoforms expression in the rat stomach are regulated by endotoxin: role in the modulation of delayed gastric emptying. Am J Physiol Gastrointest Liver Physiol. (2012) 303:G20–31. doi: 10.1152/ajpgi.00547.2011
108. Yuan PQ, Wu SV, Pothoulakis C, Taché NY. Urocortins and CRF receptor type 2 variants in the male rat colon: gene expression and regulation by endotoxin and anti-inflammatory effect. Am J Physiol Gastrointest Liver Physiol. (2016) 310:G387–398. doi: 10.1152/ajpgi.00337.2015
109. Asakawa A, Inui A, Ueno N, Makino S, Fujino MA, Kasuga M. Urocortin reduces food intake and gastric emptying in lean and ob/ob obese mice. Gastroenterology (1999) 116:1287–92.
110. Wang L, Martinez V, Rivier JE, Taché Y. Peripheral urocortin inhibits gastric emptying and food intake in mice: differential role of CRF receptor 2. Am J Physiol Regul Integr Comp Physiol. (2001) 281:R1401–10. doi: 10.1152/ajpregu.2001.281.5.R1401
111. Tanaka C, Asakawa A, Ushikai M, Sakoguchi T, Amitani H, Terashi M, et al. Comparison of the anorexigenic activity of CRF family peptides. Biochem Biophys Res Commun. (2009) 390:887–91. doi: 10.1016/j.bbrc.2009.10.069
112. Weisinger RS, Blair-West JR, Burns P, Denton DA, Mckinley MJ, Purcell B, et al. The inhibitory effect of hormones associated with stress on Na appetite of sheep. Proc Natl Acad Sci USA. (2000) 97:2922–7. doi: 10.1073/pnas.040577997
113. Fekete EM, Zhao Y, Szucs A, Sabino V, Cottone P, Rivier J, et al. Systemic urocortin 2, but not urocortin 1 or stressin 1-A, suppresses feeding via CRF2 receptors without malaise and stress. Br J Pharmacol. (2011) 164:1959–75. doi: 10.1111/j.1476-5381.2011.01512.x
114. Gourcerol G, Wang L, Wang YH, Million M, Taché Y. Urocortins and cholecystokinin-8 act synergistically to increase satiation in lean but not obese mice: involvement of corticotropin-releasing factor receptor-2 pathway. Endocrinology (2007) 148:6115–23. doi: 10.1210/en.2007-0678
115. Wang L, Stengel A, Goebel M, Martinez V, Gourcerol G, Rivier J, et al. Peripheral activation of corticotropin-releasing factor receptor 2 inhibits food intake and alters meal structures in mice. Peptides (2011) 32:51–9. doi: 10.1016/j.peptides.2010.10.017
116. Kastin AJ, Akerstrom V. Differential interactions of urocortin/corticotropin-releasing hormone peptides with the blood-brain barrier. Neuroendocrinology (2002) 75:367–74. doi: 10.1159/000059433
117. Sakai N, Yamamoto T. Conditioned taste aversion and c-fos expression in the rat brainstem after administration of various USs. Neuroreport (1997) 8:2215–20. doi: 10.1097/00001756-199707070-00025
118. Bittencourt JC, Sawchenko PE. Do centrally administered neuropeptides access cognate receptors?: an analysis in the central corticotropin-releasing factor system. J Neurosci. (2000) 20:1142–56. doi: 10.1523/JNEUROSCI.20-03-01142.2000
119. Wang L, Martinez V, Vale W, Taché Y. Fos induction in selective hypothalamic neuroendocrine and medullary nuclei by intravenous injection of urocortin and corticotropin-releasing factor in rats. Brain Res. (2000) 855:47–57. doi: 10.1016/S0006-8993(99)02200-3
120. Terashi M, Asakawa A, Cheng KC, Koyama KI, Chaolu H, Ushikai M, et al. Effects of peripherally administered urocortin 3 on feeding behavior and gastric emptying in mice. Exp Ther Med. (2011) 2:333–5. doi: 10.3892/etm.2011.200
121. Zhang X, Wu Y, Hao J, Zhu J, Tang N, Qi J, et al. Intraperitoneal injection urocortin-3 reduces the food intake of Siberian sturgeon (Acipenser baerii). Peptides (2016) 85:80–8. doi: 10.1016/j.peptides.2016.09.007
122. Mercer JG, Lawrence CB, Copeland PA. Corticotropin-releasing factor binding sites undergo axonal transport in the rat vagus nerve. J Neuroendocrinol. (1992) 4:281–6. doi: 10.1111/j.1365-2826.1992.tb00169.x
123. Lawrence AJ, Krstew EV, Dautzenberg FM, Ruhmann A. The highly selective CRF(2) receptor antagonist K41498 binds to presynaptic CRF(2) receptors in rat brain. Br J Pharmacol. (2002) 136:896–904. doi: 10.1038/sj.bjp.0704783
124. Buckinx R, Bagyanszki M, Avula LR, Adriaensen D, Van Nassauw L, Timmermans JP. Expression of corticotropin-releasing factor and urocortins in the normal and Schistosoma mansoni-infected mouse ileum. Cell Tissue Res. (2015) 359:453–63. doi: 10.1007/s00441-014-2012-8
125. Koido S, Ohkusa T, Kan S, Takakura K, Saito K, Komita H, et al. Production of corticotropin-releasing factor and urocortin from human monocyte-derived dendritic cells is stimulated by commensal bacteria in intestine. World J Gastroenterol. (2014) 20:14420–9. doi: 10.3748/wjg.v20.i39.14420
126. Li Y, Song Z, Kerr KA, Moeser AJ. Chronic social stress in pigs impairs intestinal barrier and nutrient transporter function, and alters neuro-immune mediator and receptor expression. PLoS ONE (2017) 12:e0171617. doi: 10.1371/journal.pone.0171617.
127. Estienne M, Claustre J, Clain-Gardechaux G, Paquet A, Taché Y, Fioramonti J, et al. Maternal deprivation alters epithelial secretory cell lineages in rat duodenum: role of CRF-related peptides. Gut (2010) 59:744–51. doi: 10.1136/gut.2009.190728
128. Sayegh AI. The role of cholecystokinin receptors in the short-term control of food intake. Prog Mol Biol Transl Sci. (2013) 114:277–316. doi: 10.1016/B978-0-12-386933-3.00008-X
129. Chandra R, Samsa LA, Vigna SR, Liddle RA. Pseudopod-like basal cell processes in intestinal cholecystokinin cells. Cell Tissue Res. (2010) 341:289–97. doi: 10.1007/s00441-010-0997-1
130. Goebel-Stengel M, Stengel A, Wang L, Ohning G, Taché Y, Reeve JR Jr. CCK-8 and CCK-58 differ in their effects on nocturnal solid meal pattern in undisturbed rats. Am J Physiol Regul Integr Comp Physiol. (2012) 303:R850–60. doi: 10.1152/ajpregu.00365.2011
131. Moos AB, Mclaughlin CL, Baile CA. Effects of CCK on gastrointestinal function in lean and obese Zucker rats. Peptides (1982) 3:619–22.
132. Chey WY, Hitanant S, Hendricks J, Lorber SH. Effect of secretin and cholecystokinin on gastric emptying and gastric secretion in man. Gastroenterology (1970) 58:820–7.
133. Dufresne M, Seva C, Fourmy D. Cholecystokinin and gastrin receptors. Physiol Rev. (2006) 86:805–47. doi: 10.1152/physrev.00014.2005
134. Liddle RA, Green GM, Conrad CK, Williams JA. Proteins but not amino acids, carbohydrates, or fats stimulate cholecystokinin secretion in the rat. Am J Physiol. (1986) 251:G243–8. doi: 10.1152/ajpgi.1986.251.2.G243
135. Greenberg D, Smith GP, Gibbs J. Intraduodenal infusions of fats elicit satiety in sham-feeding rats. Am J Physiol. (1990) 259:R110–18. doi: 10.1152/ajpregu.1990.259.1.R110
136. Brennan IM, Luscombe-Marsh ND, Seimon RV, Otto B, Horowitz M, Wishart JM, et al. Effects of fat, protein, and carbohydrate and protein load on appetite, plasma cholecystokinin, peptide YY, and ghrelin, and energy intake in lean and obese men. Am J Physiol Gastrointest Liver Physiol. (2012) 303:G129–40. doi: 10.1152/ajpgi.00478.2011
137. Bonaz B, De Giorgio R, Taché Y. Peripheral bombesin induces c-fos protein in the rat brain. Brain Res. (1993) 600:353–7.
138. Smith GP, Jerome C, Cushin BJ, Eterno R, Simansky KJ. Abdominal vagotomy blocks the satiety effect of cholecystokinin in the rat. Science (1981) 213:1036–7. doi: 10.1152/ajpregu.1993.264.5.R912
139. Hsu LT, Hung KY, Wu HW, Liu WW, She MP, Lee TC, et al. Gut-derived cholecystokinin contributes to visceral hypersensitivity via nerve growth factor-dependent neurite outgrowth. J Gastroenterol Hepatol. (2016) 31:1594–603. doi: 10.1111/jgh.13296
140. Sadeghi M, Radahmadi M, Reisi P. Effects of repeated treatment with cholecystokinin sulfated octapeptide on passive avoidance memory under chronic restraint stress in male rats. Adv Biomed Res. (2015) 4:150. doi: 10.4103/2277-9175.161577
141. Sadeghi M, Reisi P, Radahmadi M. The effects of CCK-8S on spatial memory and long-term potentiation at CA1 during induction of stress in rats. Iran J Basic Med Sci. (2017) 20:1368–76. doi: 10.22038/IJBMS.2017.9619
142. Hurwitz I, Malkesman O, Stern Y, Schroeder M, Lavi-Avnon Y, Shayit M, et al. Stress and pain responses in rats lacking CCK1 receptors. Peptides (2006) 27:1483–9. doi: 10.1016/j.peptides.2005.10.009
143. Juaneda C, Lafon-Dubourg P, Ciofi P, Sarrieau A, Wenger T, Tramu G, et al. CCK mRNA expression in neuroendocrine CRH neurons is increased in rats subjected to an immune challenge. Brain Res. (2001) 901:277–80. doi: 10.1016/S0006-8993(01)02365-4
144. Maniscalco JW, Rinaman L. Overnight food deprivation markedly attenuates hindbrain noradrenergic, glucagon-like peptide-1, and hypothalamic neural responses to exogenous cholecystokinin in male rats. Physiol Behav. (2013) 121:35–42. doi: 10.1016/j.physbeh.2013.01.012
145. Sander LD, Porter JR. Influence of bombesin, CCK, secretin and CRF on corticosterone concentration in the rat. Peptides (1988) 9:113–7.
146. Sherrin T, Todorovic C, Zeyda T, Tan CH, Wong PT, Zhu YZ, et al. Chronic stimulation of corticotropin-releasing factor receptor 1 enhances the anxiogenic response of the cholecystokinin system. Mol Psychiatry (2009) 14:291–307. doi: 10.1038/sj.mp.4002121
147. Macneil G, Sela Y, Mcintosh J, Zacharko RM. Anxiogenic behavior in the light-dark paradigm follwoing intraventricular administration of cholecystokinin-8S, restraint stress, or uncontrollable footshock in the CD-1 mouse. Pharmacol Biochem Behav. (1997) 58:737–46.
148. Kellner M, Yassouridis A, Jahn H, Wiedemann K. Influence of clonidine on psychopathological, endocrine and respiratory effects of cholecystokinin tetrapeptide in patients with panic disorder. Psychopharmacology (1997) 133:55–61.
149. Kellner M, Wiedemann K, Yassouridis A, Levengood R, Guo LS, Holsboer F, et al. Behavioral and endocrine response to cholecystokinin tetrapeptide in patients with posttraumatic stress disorder. Biol Psychiatry (2000) 47:107–11. doi: 10.1016/S0006-3223(99)00118-3
150. Levine AS, Morley JE. Cholecystokinin-octapeptide suppresses stress-induced eating by inducing hyperglycemia. Regul Pept. (1981) 2:353–7.
151. Goebel-Stengel M, Stengel A, Wang L, Taché Y. Orexigenic response to tail pinch: role of brain NPY(1) and corticotropin releasing factor receptors. Am J Physiol Regul Integr Comp Physiol. (2014) 306:R164–74. doi: 10.1152/ajpregu.00335.2013
152. Mojsov S, Heinrich G, Wilson IB, Ravazzola M, Orci L, Habener JF. Preproglucagon gene expression in pancreas and intestine diversifies at the level of post-translational processing. J Biol Chem. (1986) 261:11880–9.
153. Orskov C, Rabenhoj L, Wettergren A, Kofod H, Holst JJ. Tissue and plasma concentrations of amidated and glycine-extended glucagon-like peptide I in humans. Diabetes (1994) 43:535–9.
154. Roberge JN, Brubaker PL. Regulation of intestinal proglucagon-derived peptide secretion by glucose-dependent insulinotropic peptide in a novel enteroendocrine loop. Endocrinology (1993) 133:233–40. doi: 10.1210/endo.133.1.8319572
155. Reimer RA, Darimont C, Gremlich S, Nicolas-Metral V, Ruegg UT, Mace K. A human cellular model for studying the regulation of glucagon-like peptide-1 secretion. Endocrinology (2001) 142:4522–8. doi: 10.1210/endo.142.10.8415
156. Rocca AS, Brubaker PL. Role of the vagus nerve in mediating proximal nutrient-induced glucagon-like peptide-1 secretion. Endocrinology (1999) 140:1687–94. doi: 10.1210/endo.140.4.6643
157. Dailey MJ, Moran TH. Glucagon-like peptide 1 and appetite. Trends Endocrinol Metab. (2013) 24:85–91. doi: 10.1016/j.tem.2012.11.008
158. Tang-Christensen M, Larsen PJ, Goke R, Fink-Jensen A, Jessop DS, Moller M, et al. Central administration of GLP-1-(7-36) amide inhibits food and water intake in rats. Am J Physiol. (1996) 271:R848–56. doi: 10.1152/ajpregu.1996.271.4.R848
159. Chelikani PK, Haver AC, Reidelberger RD. Intravenous infusion of glucagon-like peptide-1 potently inhibits food intake, sham feeding, and gastric emptying in rats. Am J Physiol Regul Integr Comp Physiol. (2005) 288:R1695–706. doi: 10.1152/ajpregu.00870.2004
160. Gutzwiller JP, Goke B, Drewe J, Hildebrand P, Ketterer S, Handschin D, et al. Glucagon-like peptide-1: a potent regulator of food intake in humans. Gut (1999) 44:81–6.
161. Tolessa T, Gutniak M, Holst JJ, Efendic S, Hellstrom PM. Glucagon-like peptide-1 retards gastric emptying and small bowel transit in the rat: effect mediated through central or enteric nervous mechanisms. Dig Dis Sci. (1998) 43:2284–90.
162. Schirra J, Nicolaus M, Roggel R, Katschinski M, Storr M, Woerle HJ, et al. Endogenous glucagon-like peptide 1 controls endocrine pancreatic secretion and antro-pyloro-duodenal motility in humans. Gut (2006) 55:243–51. doi: 10.1136/gut.2004.059741
163. Nakagawa A, Satake H, Nakabayashi H, Nishizawa M, Furuya K, Nakano S, et al. Receptor gene expression of glucagon-like peptide-1, but not glucose-dependent insulinotropic polypeptide, in rat nodose ganglion cells. Auton Neurosci. (2004) 110:36–43. doi: 10.1016/j.autneu.2003.11.001
164. Abbott CR, Monteiro M, Small CJ, Sajedi A, Smith KL, Parkinson JR, et al. The inhibitory effects of peripheral administration of peptide YY(3-36) and glucagon-like peptide-1 on food intake are attenuated by ablation of the vagal-brainstem-hypothalamic pathway. Brain Res. (2005) 1044:127–31. doi: 10.1016/j.brainres.2005.03.011
165. Krieger JP, Arnold M, Pettersen KG, Lossel P, Langhans W, Lee SJ. Knockdown of GLP-1 receptors in vagal afferents affects normal food intake and glycemia. Diabetes (2016) 65:34–43. doi: 10.2337/db15-0973
166. Larsen PJ, Tang-Christensen M, Holst JJ, Orskov C. Distribution of glucagon-like peptide-1 and other preproglucagon-derived peptides in the rat hypothalamus and brainstem. Neuroscience (1997) 77:257–70.
167. Barrera JG, Jones KR, Herman JP, D'alessio DA, Woods SC, Seeley RJ. Hyperphagia and increased fat accumulation in two models of chronic CNS glucagon-like peptide-1 loss of function. J Neurosci. (2011) 31:3904–13. doi: 10.1523/JNEUROSCI.2212-10.2011
168. Orskov C, Poulsen SS, Moller M, Holst JJ. Glucagon-like peptide I receptors in the subfornical organ and the area postrema are accessible to circulating glucagon-like peptide I. Diabetes (1996) 45:832–5.
169. Hansen L, Deacon CF, Orskov C, Holst JJ. Glucagon-like peptide-1-(7-36)amide is transformed to glucagon-like peptide-1-(9-36)amide by dipeptidyl peptidase IV in the capillaries supplying the L cells of the porcine intestine. Endocrinology (1999) 140:5356–63. doi: 10.1210/endo.140.11.7143
170. Gil-Lozano M, Perez-Tilve D, Alvarez-Crespo M, Martis A, Fernandez AM, Catalina PA, et al. GLP-1(7-36)-amide and Exendin-4 stimulate the HPA axis in rodents and humans. Endocrinology (2010) 151:2629–40. doi: 10.1210/en.2009-0915
171. Ghosal S, Packard AEB, Mahbod P, Mcklveen JM, Seeley RJ, Myers B, et al. Disruption of glucagon-like peptide 1 signaling in Sim1 neurons reduces physiological and behavioral reactivity to acute and chronic stress. J Neurosci. (2017) 37:184–93. doi: 10.1523/JNEUROSCI.1104-16.2016
172. Terrill SJ, Maske CB, Williams DL. Endogenous GLP-1 in lateral septum contributes to stress-induced hypophagia. Physiol Behav. (2018) 192:17–22. doi: 10.1016/j.physbeh.2018.03.001
173. Maclusky NJ, Cook S, Scrocchi L, Shin J, Kim J, Vaccarino F, et al. Neuroendocrine function and response to stress in mice with complete disruption of glucagon-like peptide-1 receptor signaling. Endocrinology (2000) 141:752–62. doi: 10.1210/endo.141.2.7326
174. Isbil Buyukcoskun N, Gulec G, Cam Etoz B, Ozluk K. Central effects of glucagon-like peptide-1 on cold-restraint stress-induced gastric mucosal lesions. Turk J Gastroenterol. (2007) 18:150–6.
175. Nozu T, Miyagishi S, Kumei S, Nozu R, Takakusaki K, Okumura T. Glucagon-like peptide-1 analog, liraglutide, improves visceral sensation and gut permeability in rats. J Gastroenterol Hepatol. (2018) 33:232–9. doi: 10.1111/jgh.13808
176. Farokhnia M, Sheskier MB, Lee MR, Le AN, Singley E, Bouhlal S, et al. Neuroendocrine response to GABA-B receptor agonism in alcohol-dependent individuals: results from a combined outpatient and human laboratory experiment. Neuropharmacology (2018) 137:230–9. doi: 10.1016/j.neuropharm.2018.04.011
177. Zhu E, Hu L, Wu H, Piao L, Zhao G, Inoue A, et al. Dipeptidyl peptidase-4 regulates hematopoietic stem cell activation in response to chronic stress. J Am Heart Assoc. (2017) 6:e006394. doi: 10.1161/JAHA.117.006394
178. Zhao Y, Li H, Fang F, Qin T, Xiao W, Wang Z, et al. Geniposide improves repeated restraint stress-induced depression-like behavior in mice by ameliorating neuronal apoptosis via regulating GLP-1R/AKT signaling pathway. Neurosci Lett. (2018) 676:19–26. doi: 10.1016/j.neulet.2018.04.010
179. Yoshino Y, Ishisaka M, Tsujii S, Shimazawa M, Hara H. Glucagon-like peptide-1 protects the murine hippocampus against stressors via Akt and ERK1/2 signaling. Biochem Biophys Res Commun. (2015) 458:274–9. doi: 10.1016/j.bbrc.2015.01.098
180. Suzuki K, Iwasaki K, Murata Y, Harada N, Yamane S, Hamasaki A, et al. Distribution and hormonal characterization of primary murine L cells throughout the gastrointestinal tract. J Diabetes Investig. (2018) 9:25–32. doi: 10.1111/jdi.12681
181. Grandt D, Schimiczek M, Beglinger C, Layer P, Goebell H, Eysselein VE, et al. Two molecular forms of peptide YY (PYY) are abundant in human blood: characterization of a radioimmunoassay recognizing PYY 1-36 and PYY 3-36. Regul Pept. (1994) 51:151–9.
182. Mentlein R, Dahms P, Grandt D, Kruger R. Proteolytic processing of neuropeptide Y and peptide YY by dipeptidyl peptidase IV. Regul Pept. (1993) 49:133–44.
183. Batterham RL, Cowley MA, Small CJ, Herzog H, Cohen MA, Dakin CL, et al. Gut hormone PYY(3-36) physiologically inhibits food intake. Nature (2002) 418:650–4. doi: 10.1038/nature00887
184. Abbott CR, Small CJ, Kennedy AR, Neary NM, Sajedi A, Ghatei MA, et al. Blockade of the neuropeptide Y Y2 receptor with the specific antagonist BIIE0246 attenuates the effect of endogenous and exogenous peptide YY(3-36) on food intake. Brain Res. (2005) 1043:139–44. doi: 10.1016/j.brainres.2005.02.065
185. Burdyga G, De Lartigue G, Raybould HE, Morris R, Dimaline R, Varro A, et al. Cholecystokinin regulates expression of Y2 receptors in vagal afferent neurons serving the stomach. J Neurosci. (2008) 28:11583–92. doi: 10.1523/JNEUROSCI.2493-08.2008
186. Koda S, Date Y, Murakami N, Shimbara T, Hanada T, Toshinai K, et al. The role of the vagal nerve in peripheral PYY3-36-induced feeding reduction in rats. Endocrinology (2005) 146:2369–75. doi: 10.1210/en.2004-1266
187. Nonaka N, Shioda S, Niehoff ML, Banks WA. Characterization of blood-brain barrier permeability to PYY3-36 in the mouse. J Pharmacol Exp Ther. (2003) 306:948–53. doi: 10.1124/jpet.103.051821
188. Bonaz B, Taylor I, Taché Y. Peripheral peptide YY induces c-fos-like immunoreactivity in the rat brain. Neurosci Lett. (1993) 163:77–80.
189. Stadlbauer U, Arnold M, Weber E, Langhans W. Possible mechanisms of circulating PYY-induced satiation in male rats. Endocrinology (2013) 154:193–204. doi: 10.1210/en.2012-1956
190. Broberger C, Landry M, Wong H, Walsh JN, Hokfelt T. Subtypes Y1 and Y2 of the neuropeptide Y receptor are respectively expressed in pro-opiomelanocortin- and neuropeptide-Y-containing neurons of the rat hypothalamic arcuate nucleus. Neuroendocrinology (1997) 66:393–408. doi: 10.1159/000127265
191. Liang C, Luo H, Liu Y, Cao J, Xia H. Plasma hormones facilitated the hypermotility of the colon in a chronic stress rat model. PLoS ONE (2012) 7:e31774. doi: 10.1371/journal.pone.0031774
192. Kiessl GR, Laessle RG. Stress inhibits PYY secretion in obese and normal weight women. Eat Weight Disord. (2016) 21:245–9. doi: 10.1007/s40519-015-0231-y
193. Yamada C, Mogami S, Kanno H, Hattori T. Peptide YY causes apathy-like behavior via the dopamine D2 receptor in repeated water-immersed mice. Mol Neurobiol. (2018) 55:7555–66. doi: 10.1007/s12035-018-0931-1
194. Forbes SC, Cox HM. Peptide YY, neuropeptide Y and corticotrophin-releasing factor modulate gastrointestinal motility and food intake during acute stress. Neurogastroenterol Motil. (2014) 26:1605–14. doi: 10.1111/nmo.12428
Keywords: food intake, gastrointestinal functions, gut-brain axis, hypothalamus, peptides
Citation: Stengel A and Taché Y (2018) Gut-Brain Neuroendocrine Signaling Under Conditions of Stress—Focus on Food Intake-Regulatory Mediators. Front. Endocrinol. 9:498. doi: 10.3389/fendo.2018.00498
Received: 31 May 2018; Accepted: 08 August 2018;
Published: 28 August 2018.
Edited by:
Jeff M. P. Holly, University of Bristol, United KingdomReviewed by:
Bruno Bonaz, Centre Hospitalier Universitaire de Grenoble, FranceBalazs Gaszner, University of Pécs, Hungary
Copyright © 2018 Stengel and Taché. This is an open-access article distributed under the terms of the Creative Commons Attribution License (CC BY). The use, distribution or reproduction in other forums is permitted, provided the original author(s) and the copyright owner(s) are credited and that the original publication in this journal is cited, in accordance with accepted academic practice. No use, distribution or reproduction is permitted which does not comply with these terms.
*Correspondence: Andreas Stengel, YW5kcmVhcy5zdGVuZ2VsQG1lZC51bmktdHVlYmluZ2VuLmRl