- 1Sleep, Metabolism and Health Center, University of Chicago, Chicago, IL, United States
- 2Division of Endocrinology and Metabolism, Department of Internal Medicine, University of Iowa Carver College of Medicine, Iowa City, IA, United States
- 3Section of Endocrinology, Department of Medicine, University of Chicago, Chicago, IL, United States
- 4Section of Pulmonary and Critical Care, Department of Medicine, University of Chicago, Chicago, IL, United States
Background: Slow-wave activity (SWA) in non-rapid eye movement (NREM) sleep, obtained by spectral analysis of the electroencephalogram, is a marker of the depth or intensity of NREM sleep. Higher levels of SWA are associated with lower arousability during NREM sleep and protect against sleep fragmentation. Multiple studies have documented that SWA levels are higher in lean women, compared to age-matched lean men, but whether these differences persist in obese subjects is unclear. Obstructive sleep apnea (OSA), a condition associated with obesity, is more prevalent in men than in women. Sex differences in SWA could therefore be one of the factors predisposing men to OSA. Furthermore, we hypothesized that higher levels of testosterone may be associated with lower levels of SWA.
Objective: The aim of the current study was to identify sex differences in the determinants of SWA in young and middle-aged overweight and obese adults.
Methods: We enrolled 101 overweight and obese but otherwise healthy participants from the community (44 men, 57 women) in this cross-sectional study. Participants underwent an overnight in-laboratory polysomnogram. The recordings were submitted to sleep staging and spectral analysis. Sex differences and the potential contribution of testosterone levels were evaluated after adjusting for age, body mass index and race/ethnicity.
Results: OSA was present in 66% of men and in 44% of women. After adjustment for differences in age, race/ethnicity and BMI, the odds ratio for OSA in men vs. women was 3.17 (95% CI 1.14–9.43, p = 0.027). There was a graded inverse relationship between the apnea-hypopnea index (AHI) and SWA in men (β = −0.21, p = 0.018) but not in women (β = 0.10, p = 0.207). In a multivariate regression model, higher testosterone levels were independently associated with lower SWA in men after controlling for age, race/ethnicity and apnea-hypopnea index (β = −0.56, p = 0.025).
Conclusion: Increasing severity of OSA was associated with significant decrease in sleep intensity in men but not in women. Higher testosterone levels were associated with lower sleep intensity in men. Men with higher testosterone levels may therefore have lower arousal thresholds and higher ventilatory instability in NREM sleep, and be at greater risk of OSA.
Introduction
Slow-wave activity (SWA) in non-rapid eye movement (NREM) sleep, also known as delta activity, is a marker of the depth or intensity of NREM sleep. SWA may be quantified by spectral analysis of the electroencephalogram (EEG) in the low frequency range typical of slow waves (0.75–4.5 Hz) (1, 2). SWA measurements are highly reproducible from night to night in a given individual (3–5). Higher levels of SWA are associated with lower arousability during NREM sleep and protect against sleep fragmentation due to external or internal disturbances (6). Multiple studies have documented that SWA levels are higher in women, compared to age-matched men (7–9) but the majority of the findings were obtained in lean adults.
Observational studies have reported significant transient reduction of obstructive sleep apnea (OSA) severity during slow wave sleep (SWS), the deepest stage of NREM sleep, which is associated with higher levels of SWA (10, 11). Rigorous mechanistic studies examining the neuromechanical properties of the upper airway during NREM sleep have shown that the upper airway is indeed less collapsible during SWS (12, 13). A higher arousal threshold during SWS, which is partly determined by the intensity of NREM sleep, i.e., the level of SWA, has also been recognized as one of the factors leading to OSA improvement during SWS (14, 15).
Estimations of OSA prevalence have typically found that the disorder is much more common in men than in women, particularly in young and middle-aged adults (16, 17). Obesity is an important and well-recognized risk factor for OSA. However, women with OSA are typically heavier than men when matched for OSA severity (18–20). Thus, being a woman appears to offer a relative protection against the development of OSA, even in the presence of more severe obesity. The reasons for this sex disparity in OSA risk have not been clearly identified, and may lie in anatomical, neuronal, and/or hormonal differences (21). In particular, whether sex differences in SWA are also present in overweight and obese adults and are associated with differences in OSA presence and severity has not been systematically evaluated.
The potential role of testosterone levels, which are much lower in women than in men, in the regulation of SWA and in the risk of OSA is an important related question. Testosterone levels are known to be modulated by sleep duration, sleep restriction and sleep quality in men (22–24). Several small studies have examined the impact of OSA on testosterone levels in men and some have reported decreased testosterone concentrations in men with OSA compared to controls (25–27). On the other hand, multiple reports of exogenous testosterone administration triggering or worsening OSA have been published (28–32). However, it should be noted that participants received supraphysiologic doses of testosterone in all of these studies except one (32). In the latter study, the worsening of OSA severity was only transient (32). Conversely, pharmacologically induced reduction of testosterone in healthy men has been shown to increase breathing stability during NREM sleep, potentially leading to a reduction in the risk of OSA (33, 34), while a small study in premenopausal women demonstrated a decrease in breathing stability after <2 weeks of transdermal testosterone administration resulting in male testosterone levels (35).
The aim of the present study was therefore to examine sex differences in sleep architecture, assessed by visual scoring of all-night polysomnographic (PSG) laboratory recordings and quantitative EEG analysis, and to identify factors associated with SWA in overweight and obese men and women, with and without OSA. Additionally, we explored the possible contribution of endogenous testosterone levels to individual differences in SWA in both men and women.
Participants and Methods
Subjects aged 20–50 years with body mass index (BMI) >25 kg/m2 were recruited from the community between 2008 and 2014 using written advertisements inviting participation in research studies on sleep and metabolism. Sleep complaints or symptoms of OSA were not used as selection criteria for the study. Exclusion criteria were: self-reported habitual sleep duration of <6 h per night or more than 10 h per night, chronic insomnia, any sleep disorder other than OSA, any prior or current treatment for OSA (upper airway surgery, CPAP therapy, oral appliances or supplemental oxygen), history of substance abuse, hypnotic or psychotropic medications, tobacco use, caffeine intake above 300 mg per day, alcohol intake above 10 drinks per week, pregnancy, hormonal therapy, liver disease, renal insufficiency, heart failure, cancer, chronic infectious diseases, neurological or psychiatric diseases, diabetes, shift work within the last 3 months and travel across more than two time zones in the 4 weeks before the sleep recording. All female participants were premenopausal and were not taking hormonal contraceptives.
Study participants had a physical examination, and a complete medical history was obtained. Height and weight were measured. Self-reported race/ethnicity was recorded. One hundred and thirty participants underwent an overnight in-laboratory PSG with a minimum recording time of 7.5 h. Twenty nine subjects were excluded from analysis due to artifacts in the EEG (n = 22), total sleep duration <5 h (n = 5) or recording missing from server (n = 2). EEG recordings devoid of significant artifacts and with total sleep time of at least 5 h were obtained in 101 participants (44 men and 57 women). The following morning, fasting blood samples were taken to measure total and free testosterone and sex-hormone binding globulin.
Assays
Plasma total testosterone was measured by a direct RIA kit (Coat-A-Count; Siemens Medical Solutions USA, Inc; Malvern, PA, USA) that has been validated against a liquid chromatography/tandem mass spectrometry method (36) with functional sensitivity of 10 ng/dl and normal range of 312–1240 ng/dl in men and 19–70 ng/dl in women. Total testosterone values were not obtained in 2 men and 1 woman. The plasma free testosterone concentration was computed as the product of total testosterone and percentage free testosterone, which was determined directly in plasma by competitive protein-binding assay with a sensitivity of 3 pg/ml (37, 38). Free testosterone levels were not obtained in 7 men and 1 woman. Sex hormone binding globulin (SHBG) was measured by an in-house assay based on a competitive protein binding procedure (37, 38). Intra-assay variation coefficients for testosterone, free testosterone and SHBG were 6, 6, and 19%, respectively, in men, and 12, 13, and 14%, respectively, in women.
Sleep Analysis
Sleep recordings were performed in the laboratory using a digital EEG acquisition system (Neurofax EEg-1100 A, Nihon Kohden, Tokyo, Japan). Lights-off time was tailored to match self-reported habitual bedtime (± 1 h) and total recording time was at least 6 h. Surface electrodes were used to collect the EEG signals and the acquisition montage included, in addition to a vertex central referential lead and one ground lead, two central EEG leads (C3 and C4), two occipital leads (O1 and O2), two mastoids leads (A1 and A2), one vertical and one horizontal electro-oculogram (EOG), one bipolar submental chin electromyogram (EMG), and one bipolar electrocardiogram (ECG). The presence or absence of OSA was evaluated by measuring oronasal airflow signal by thermal flow sensor and nasal pressure transducer, respiratory effort by thoracic and abdominal piezoelectric belts or resistance inductive plethysmography and arterial oxygen saturation by finger pulse oximetry. Limb movements were also recorded from one bipolar tibial EMG to exclude individuals with movement disorders. Polysomnographic recordings were visually scored in 30-s epochs by an experienced professional sleep technologist considering stages W, N1, N2, N3, and R in adherence with standardized criteria (39). Respiratory events, periodic limb movements and microarousals were scored according to established criteria (39, 40). The apnea-hypopnea index (AHI) was calculated as the total number of obstructive apneas and hypopneas per hour of sleep. Apneas were defined as total cessation of airflow for at least 10 s if respiratory effort was present. Hypopneas were defined as a decrease in nasal pressure signal of ≥30% of baseline, which was associated with either a ≥3% desaturation or an arousal. The presence of OSA was defined by an AHI ≥5 events/h. The microarousal index was defined as the number of microarousals per hour of sleep. The oxygen desaturation index (ODI) was defined as the number of oxygen desaturations ≥3% per hour of sleep.
During acquisition, all EEG signals were filtered between 0.3 and 35 Hz and digitally sampled and stored at 200 Hz with a 16-bit quantization range. Power spectral analysis of the EEG was performed on two central leads (C3, C4) re-referenced to the linked mastoids references (A1 and A2). All analyses were performed directly on the original recording files without data format conversion using the PRANA Software Suite (http://www.phitools.com, Strasbourg, France). After removal of muscular, ocular and movement artifacts by automatic and visual inspection, a fast Fourier transform using a 50% overlap between consecutive 4-s elementary windows was computed following the application of a Hanning taper and resulted in a spectral resolution of 0.25 Hz. The spectra of all elementary windows overlapping with artifacts were discarded prior averaging of the elementary spectra on a 30-s epoch basis in order to match the sleep/wake stage scores. When more than 50% of the elementary spectra windows were contaminated, averaging was skipped and the corresponding time series intervals replaced by missing vales in order to preserve continuity in the time series. Absolute power in the delta, theta, alpha and sigma frequency bands (0.75–4.5 Hz, 4.5–8.5 Hz, 8.5–12 Hz, and 12.5–15 Hz, respectively) were calculated by summing up powers of all frequency bins within each band, the lower bin being included and the upper one excluded. Finally, an average of spectral power in each frequency band was calculated using only the 30-epochs from NREM sleep during the first 6 h of sleep. Slow-wave activity in NREM sleep is equivalent to delta power in NREM sleep.
For illustrative purposes, the durations of NREM-REM cycles were normalized to account for individual differences, with each individual NREM period subdivided into 50 equal time bins and each REM period into 20 time bins, as previously described (41). NREM-REM cycles were defined according to the criteria of Feinberg and Floyd (42) and visually verified. For subjects with REM latency >120 min, the termination of the first cycle was identified as the first nadir of SWA. Three subjects had 3 NREM-REM cycles during the night of PSG, while all others had four cycles or more.
Statistical Analysis
Statistical analysis was performed using JMP version 8.0.2 (S.A.S Institute Inc., Cary, NC, USA) and confirmed with SPSS Statistics v20. All group data are expressed as means ± SEM for normally distributed variables, or median (interquartile range) for non-normally distributed variables. Transformation of raw data to achieve normal distribution was performed whenever appropriate prior to statistical analysis. In particular, BMI, AHI and EEG spectral powers were transformed in all analyses using the natural log (Ln). Sex differences for demographic and hormonal characteristics were assessed by Student's t-test for continuous variables and chi-square test for categorical variables. Comparisons were considered statistically significant at p < 0.05. Multivariate linear regression models adjusting for age, BMI, and race/ethnicity were fitted to examine sex differences in sleep stages, characteristics of OSA and spectral power in the different EEG frequency bands as well as the interaction sex x OSA and sex x AHI. When interactions were statistically significant, analyses were repeated separately in men and women. To identify the possible contribution of plasma levels of testosterone and SHBG to the observed sex differences, multivariate linear regression models including the hormonal variable in addition to age, BMI, AHI and race/ethnicity, were fitted separately in men and women.
Results
Demographic Characteristics and OSA Prevalence
In the present cohort of 101 participants, women (n = 57) were significantly more obese than men (n = 44) (BMI 38.0 ± 1.1 vs. 34.4 ± 0.9 kg/m2, p = 0.021) and more often African-American (74 vs. 50%, p = 0.022). The demographic characteristics of male and female participants, without and with OSA, are summarized in Table 1. Irrespective of sex, participants with OSA were significantly older than those without OSA (37 ± 1 vs. 31 ± 1 years, p < 0.0001). Consistent with previous reports (18–20), women with OSA had a higher BMI than men with OSA (median [IQR]: 38.7 [33.1–44.9] vs. 34.3 [32.1–36.8] kg/m2, p = 0.007). Given these differences in demographics, subsequent analyses were performed adjusting for age, BMI and race/ethnicity. After adjustment, the odds ratio for OSA in men as compared to women was 3.17 (95% CI 1.14–9.43, p = 0.027).
Men with OSA showed a trend for higher total testosterone (422[358-551]vs. 394[318-63] ng/dl, p = 0.095) and SHBG (13[10-16] vs. 9[7-13]nM, p = 0.070) concentrations compared to men without OSA, but free testosterone levels did not differ between these two groups. No difference in total testosterone, free testosterone or SHBG levels were found between women with or without OSA.
Macro-Architecture of Sleep
Polysomnographic variables are presented in Table 2. Median (IQR) recording time in the 101 participants was 8 h 06 min (8 h 01–8 h 30). After adjustment, men with OSA had less N3 slow wave sleep compared to men without OSA (11[2-37] vs. 33[7-82]min, p = 0.069), although the difference did not reach statistical significance. Moreover, men with OSA had significantly less N3 slow wave sleep than women with OSA (11[2-37] vs. 50[25-65] min, p < 0.001). In contrast, in women, N3 slow wave sleep duration was not influenced by the presence of OSA (61[28-82] min in women without OSA vs. 50[24-85] min in women with OSA, p = 0.822). Finally, compared to women without OSA, women with OSA had less REM sleep (90 ± 6 vs. 106 ± 5 min, p = 0.039).
Sex Differences in Characteristics of OSA
Figure 1 illustrates sex differences in the characteristics of OSA for the entire cohort, after adjusting for age, BMI and race/ethnicity. Men and women had similar total and NREM AHI. However, men had a significantly greater AHI in REM sleep compared to women (24.4 ± 1.3 vs. 8.5 ± 1.3 events/hour, p = 0.004), as well as higher total ODI (4.4 ± 1.3 vs. 1.3 ± 1.3 events/hour, p = 0.005), NREM ODI (2.1 ± 1.5 vs. 0.6 ± 1.5 events/hour, p = 0.041), and REM ODI (7.7 ± 1.5 vs. 2.0 ± 1.6 events/hour, p = 0.039). The percentage of sleep time spent below 90% oxygen saturation (T90%) was also higher in men than women (1.04 ± 2.34 vs. 0.07 ± 1.08%, p = 0.005), in both REM and NREM sleep. In contrast to variables quantifying hypoxemia, the overall microarousal index was similar in men and women. Although the microarousal index during NREM sleep was higher in women, it was still within the normal range.
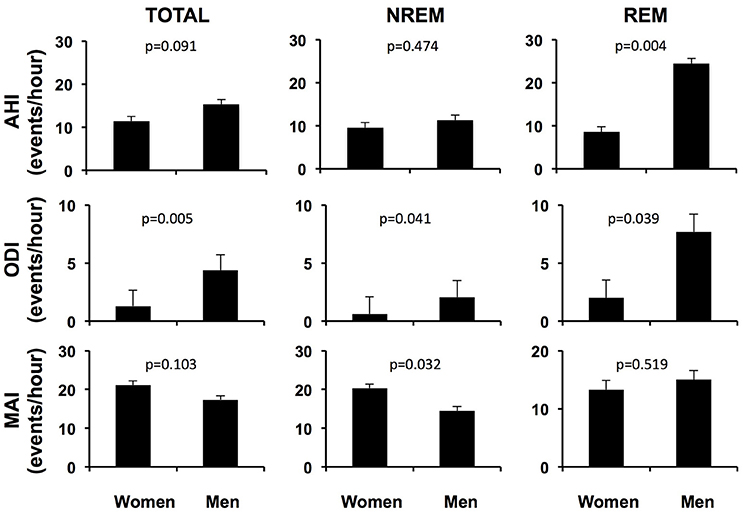
Figure 1. Adjusted respiratory variables and microarousal index of men and women with OSA over the entire night, in NREM sleep and in REM sleep. AHI apnea-hypopnea index; ODI oxygen desaturation index; MAI microarousal index; NREM non rapid eye movement sleep; REM rapid eye movement sleep. Adjusted for age, BMI and race/ethnicity.
Micro-Architecture of Sleep
Figure 2 illustrates the unadjusted profiles of SWA and theta activity normalized by NREM-REM cycles, in men and women with and without OSA. Profiles of alpha and sigma activity were similar in men and women, with and without OSA (not shown). In unadjusted analyses, the presence of OSA was associated with a 34% lower NREM SWA in men (non OSA 441 ± 51, vs. OSA 289 ± 24 μV2; p = 0.007) but did not significantly impact NREM SWA in women (non OSA 479 ± 37, vs. OSA 521 ± 53 μV2; p = 0.582). As summarized in Table 3, multivariate linear regression confirmed a sex-specific impact of OSA on NREM SWA as reflected by a significant sex x LnAHI interaction (p = 0.001). For NREM theta activity, a spectral power in an intermediate frequency range that is often contaminated by SWA, a trend for a greater impact of OSA in men than in women (p = 0.066 for sex x LnAHI interaction) was also present. In contrast, OSA had no significant influence on NREM alpha and sigma activity in either men or women, two power bands generated by neuronal systems distinct from those responsible for SWA.
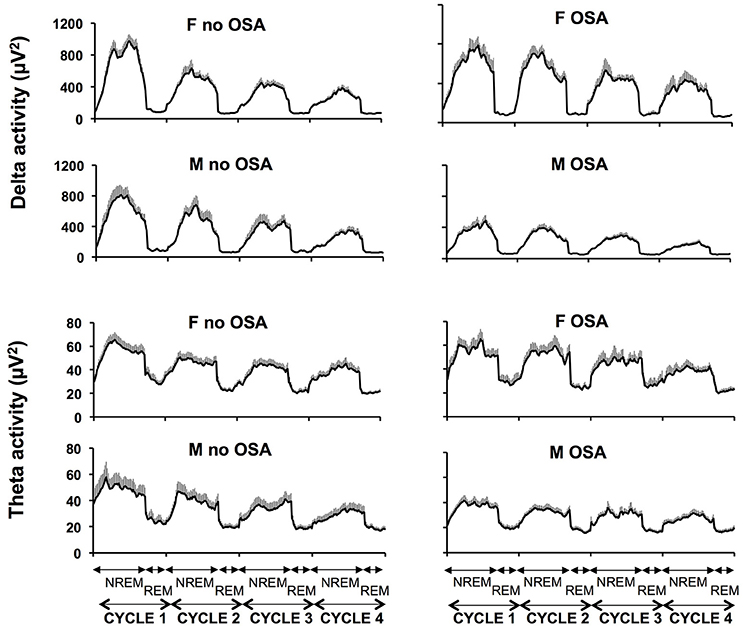
Figure 2. Unadjusted mean profiles (+SEM) of absolute SWA (4 upper panels) and theta activity (4 lower panels) during the first four NREM-REM cycles in women without and with OSA (upper panels) and men without and with OSA (lower panels). SWA slow wave activity; NREM non rapid eye movement sleep; REM rapid eye movement sleep.
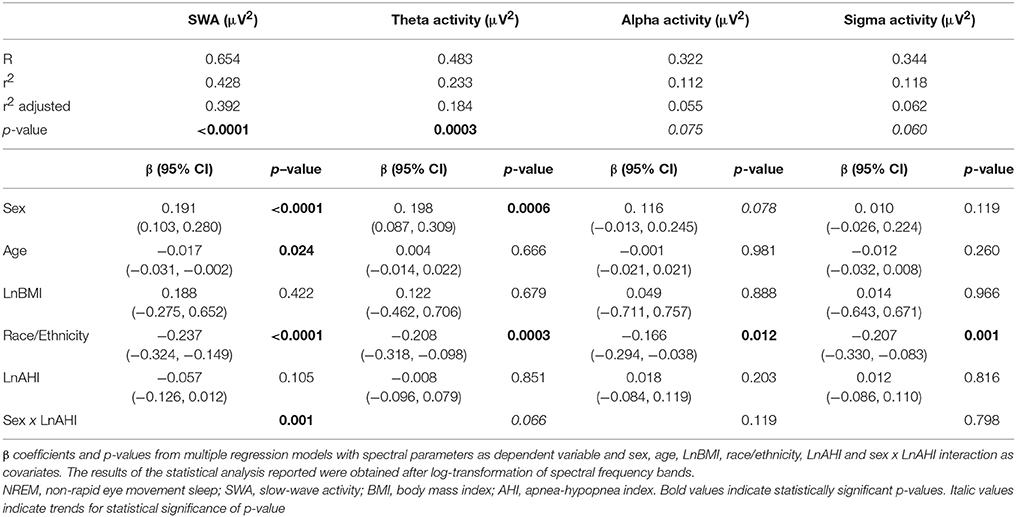
Table 3. Impact of demographic characteristics and OSA on EEG spectral activity in NREM sleep (first 6 h of sleep).
To facilitate the interpretation of the impact of OSA severity on NREM SWA, we also fitted a linear regression model replacing LnAHI with AHI tertiles derived from the total of 101 subjects (first tertile or T1AHI < 2.3; T2AHI 2.3–11.7; T3AHI > 11.7 events/hour) after adjusting for age, race/ethnicity and BMI. A significant negative linear trend for adjusted SWA was clearly present in men (Figure 3; β = −0.206, p = 0.018) such that men in the highest tertile of AHI had SWA levels less than half of those found in men in the lower tertile of AHI. In contrast, in women there was no significant association between AHI tertiles and adjusted SWA (β = 0.104, p = 0.207). Similar results were obtained when subdividing the participants according to clinical cut offs of OSA severity (no [AHI < 5], mild [AHI 5–15] and moderate-severe OSA [AHI > 15], data not shown).
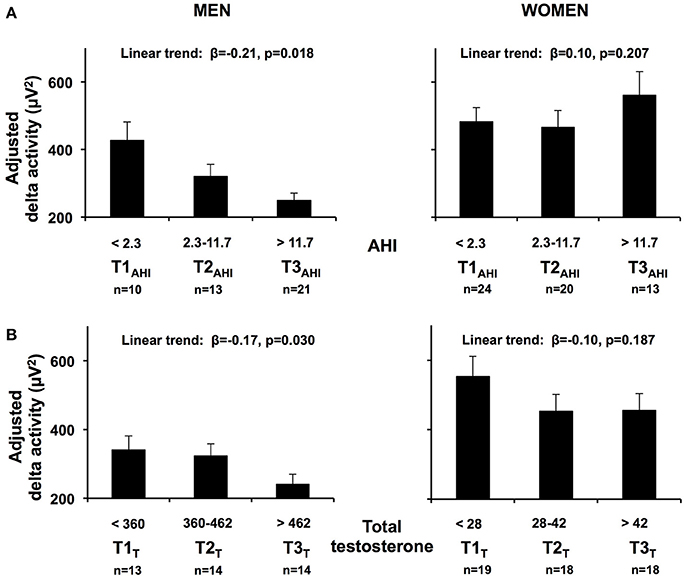
Figure 3. (A) Adjusted mean SWA (+SEM) in NREM sleep, in the first 6 h of sleep according to AHI tertiles (T1AHI, T2AHI, T3AHI) in men and women. (B) Adjusted mean SWA (+SEM) in NREM sleep, in the first 6 h of sleep according to total testosterone tertiles (T1T, T2 T, T3 T) in men and women. Results obtained from linear regression models including AHI tertiles derived from the total of 101 subjects. Age and BMI were centered at their means. An inverse log transformation was applied to beta coefficients for each AHI tertile to convert from Ln[NREM SWA] to the standard values adjusted for age, BMI and race/ethnicity. Standard errors were obtained by inverse log transformation of the upper and lower 95% confidence intervals of the model estimates and by dividing the difference by four, for each tertile. SWA, slow wave activity; AHI, apnea hypopnea index.
Determinants of NREM SWA in Men and Women
Because of the significant sex x LnAHI interaction present for SWA, we examined the determinants of NREM SWA separately in men and women, and explored the potential roles of circulating total and free testosterone levels as well as SHBG. The upper part of Table 4 describes the results of four multivariate linear regression models predicting NREM SWA in men. Model 1 includes demographic characteristics only, and reveals that age (p = 0.004) and race/ethnicity (p = 0.001), but not BMI, are significantly associated with SWA levels in this cohort of 20–50 years old overweight and obese men. Introducing AHI (model 2) improved the percentage of variance (r2) accounted for by the model; the association with age was no longer significant, while AHI (p = 0.002) was independently associated with SWA. The substitution of total testosterone levels for AHI (model 3) increased the r2 further, and testosterone levels significantly and negatively correlated with SWA (p = 0.005). Since we found no association with BMI in models 1–3, BMI was dropped for the last model to maintain statistical power and we examined simultaneously the contributions of AHI and testosterone levels. SWA remained strongly negatively associated with testosterone (p = 0.025), while the strength of the association with age and AHI was considerably reduced. The left lower part of Figure 3 illustrates the association between increasing tertiles of total testosterone levels and SWA in men, after adjusting for age, BMI and race/ethnicity (model 3).
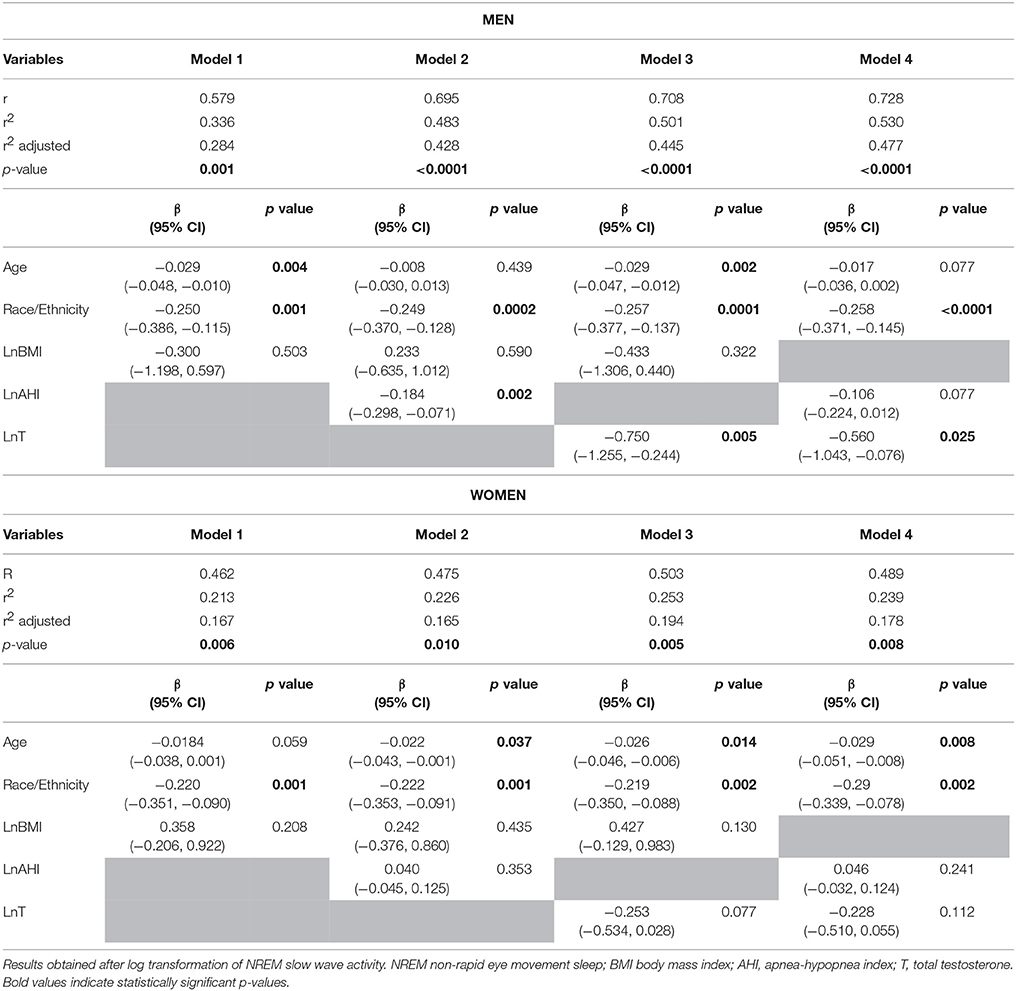
Table 4. Determinants of NREM slow wave activity (first 6 h of sleep) in men (top panel) and women (bottom panel).
Similar associations with testosterone were found when free, rather than total concentrations were used. After controlling for age, race/ethnicity, and AHI (model 4), free testosterone level was significantly associated with SWA in men (p = 0.012, data not shown). There were no significant associations with SHBG levels in any of the models.
The lower part of Table 4 describes the results of the same four multivariate linear regression models predicting SWA in women. An association of NREM SWA with age and race/ethnicity was observed in all models, while no association between SWA and AHI was present. Testosterone levels were not a significant predictor but the beta coefficient was negative, similar to findings in men, and the significance level suggests the possibility that a trend may be found in a larger sample. The relationship between SWA and increasing tertiles of total testosterone is illustrated for the female participants in the left lower panels of Figure 3.
Of note, while model 4 accounts for nearly 50% of the inter-individual variability in SWA among men, the same covariates account for <20% of the variability among women. In this cohort of overweight and obese but otherwise healthy men and women ages 20–50 years, the degree of adiposity as assessed by the BMI was not associated with any measure of NREM sleep intensity and depth.
Discussion
In this study, we performed a comprehensive and rigorous laboratory assessment of sleep quality in overweight and obese men and women recruited from the community. Our sample was not selected based on prior diagnosis or symptoms of OSA. We observed a high prevalence of OSA, which was present in two-thirds of the men and nearly half of the women. After adjustment for age, BMI and race/ethnicity, the odds ratio of having OSA was 3 times higher in men as compared to women. Our results also reveal that the sex differences in duration of SWS (43–46) and in intensity of NREM sleep by spectral analysis (8, 47) previously reported in lean populations are also present in overweight and obese adults with OSA. We found a differential impact of OSA on the intensity of NREM sleep in men vs. women. Indeed, after adjusting for potential confounders, there was a graded inverse relationship between the severity of OSA and SWA in men, but not in women. A previous cross-sectional study with a small sample size has shown that in men, the presence of OSA is associated with lower SWA (48), likely because of fragmentation and arousals induced by respiratory events. In women, the impact of OSA on SWA has not been previously characterized. A possible explanation for the observed sex differences in the impact of OSA on the intensity of NREM sleep could be that women tend to have REM-related OSA, i.e., the majority of the obstructive events during REM sleep, more often than men (49–51). However, this was not the case in our cohort and therefore would not explain our findings. Sex differences in NREM SWA have also been hypothesized to be due to difference in skull size and thickness, as well as skin thickness (52), but anatomic differences would also not explain the different impact of OSA on men's sleep compared to women's. Finally, differences in neuronal activity could exist between men and women. This has been suggested by a few studies assessing sex differences in the impact of aging on EEG activity, as well as findings in patients with affective disorders. In men, SWA as well as SWS decline after the third decade, while such a decline occurs later in women (8, 53, 54). Similarly, decreased levels of SWA have been reported in men with major depression, compared to controls, but this was not observed in women (55). The exact mechanisms underlying these differences have yet to be identified, but these and other studies suggest a greater “neural slow wave synchronization” ability in women than men (56). In our case, this could explain why women are able to reach SWS despite recurrent respiratory events as well as why OSA is less prevalent or less severe in premenopausal women despite higher levels of obesity when compared to men.
Another important novel finding is that, in our cohort of overweight and obese young to middle-aged men, higher testosterone levels were strongly associated with a lower intensity of NREM sleep. Similar results were obtained with free testosterone concentrations. This observation suggests that common genetic or non-genetic pathways may link the intensity of NREM sleep, a stable phenotype that is highly variable across individuals, and the set point of the hypothalamo-pituitary-gonadal axis. A potential consequence of this inverse association might be that high testosterone levels are associated with a lower arousal threshold and a greater vulnerability to respiratory instability. This interpretation is consistent with reports of increased severity of OSA following exogenous intramuscular testosterone administration (28–32), and further suggests that the exacerbation of OSA may occur through reductions in NREM SWA. It is noteworthy that the findings of our cross-sectional analysis are not in contradiction with the results of the few intervention studies that showed that CPAP treatment of OSA may increase testosterone levels (26, 57). Indeed, for any given male OSA patient in whom SWA has been fragmented by repeated complete or partial obstruction of the upper airway, the restoration of sleep continuity, particularly during NREM sleep, should be associated with enhanced nocturnal testosterone release.
In our cohort, despite the fact that they were older and heavier, men with OSA had slightly higher total testosterone levels compared to men without OSA. SHBG was slightly higher as well in the former. SHBG is known to increase with age and this could explain our findings (58). No differences in free testosterone were present between groups, and results of the multivariate analysis were unchanged when free testosterone was used as a covariate in our models. Of note, most of our participants had moderate OSA, while studies that reported decreased testosterone levels in men with OSA vs. controls included a majority of patients with severe OSA (25–27). Furthermore, participants enrolled in those studies were older than our volunteers [average age over 50 in all but two studies (27, 59)]. In these studies, OSA and control participants were matched for either BMI (25) or age (26, 27). Furthermore, in all studies that examined testosterone levels in men with OSA (26, 27, 60–64) except one (25), the average or median testosterone levels in the OSA group were in the normal range [>300 ng/dl (65)], suggesting that a majority of men with OSA do have normal testosterone levels.
We have to acknowledge the limitations of the present study. First, the cross-sectional nature of the study limits any assessment of causality. Second, the study protocol did not include a habituation night and we cannot exclude an impact of an unfamiliar sleeping environment on the sleep variables. However, this would have affected all groups equally. Third, our cohort is relatively small. Fourth, we could not systematically control for the menstrual phase in women (who were all premenopausal and off hormonal contraceptives). However, no impact of menstrual phase on NREM SWA levels has been detected in previous studies (66, 67). By not controlling for menstrual phase, we may also have underestimated the prevalence of OSA in women, since upper airway resistance is decreased in the luteal phase (68). Furthermore, we may have overestimated T levels in women as androgen concentrations have been shown to peak in the late follicular phase (69). Fifth, testosterone levels in women were measured by RIA, which is reported to be less accurate at low values. However, our assay has been validated against a liquid chromatography/tandem mass spectrometry method, with correlation coefficients of 0.97 for all samples (men and women), and 0.91 for samples obtained from women (36). Finally, our testosterone assay was performed on a single morning sample for each participant. However, our study was not aimed at diagnosing hypogonadism, which does requires measurement of testosterone levels on two separate occasions at least (65). Furthermore, only one morning sample was obtained in most other studies that examined the impact of OSA on androgen levels (26, 27, 60–64).
In summary, this cross-sectional analysis demonstrates a sex difference in the association between OSA severity and NREM SWA, as well as a robust negative association between total testosterone levels and intensity of NREM sleep in overweight and obese men. Further studies are needed to confirm and extend our results and elucidate the mechanisms linking circulating testosterone levels, SWA and OSA.
Ethics Statement
This study was carried out in accordance with the recommendations of the University of Chicago Institutional Review Board. The protocol was approved by the University of Chicago Institutional Review Board. All subjects gave written informed consent in accordance with the Declaration of Helsinki.
Author Contributions
EVC: designed the protocol; LLM, KAT, and RL: recruited subjects and collected data; LLM, EVC, and BM: analyzed the data; LLM: drafted the manuscript; EVC, DAE, and BM: reviewed and edited the manuscript. EVC and BM are both the senior authors for this submission.
Funding
Supported by NIH grants P50-HD057796, P60-DK20595 (The University of Chicago Diabetes Research and Training Center), P01 AG-11412, UL1-TR000430 (The University of Chicago CTSA), and the ResMed Foundation. BM is supported in part by NIH grant R01 HL-119161. EVC and DAE are supported by NIH grant P50HD057796.
Conflict of Interest Statement
BM is supported by National Institutes of Health grant R01HL119161 and the Merck Investigator Studies Program. EV is the principal investigator of an investigator-initiated study sponsored by Philips/Respironics.
The remaining authors declare that the research was conducted in the absence of any commercial or financial relationships that could be construed as a potential conflict of interest.
Acknowledgments
The authors wish to thank Harry Whitmore, R-PSGT, from the Sleep, Metabolism and Health Center (SMAHC) at the University of Chicago, for providing expert technical assistance with the collection and scoring of polysomnographic recordings. We are also grateful to the staff of the Sleep, Metabolism and Health Center and the General Clinical Resource Center of the University of Chicago, and to the study participants.
References
1. Daan S, Beersma DG, Borbely AA. Timing of human sleep: recovery process gated by a circadian pacemaker. Am J Physiol. (1984) 246:R161–83. doi: 10.1152/ajpregu.1984.246.2.R161
2. Achermann P, Dijk DJ, Brunner DP, Borbely AA. A model of human sleep homeostasis based on EEG slow-wave activity: quantitative comparison of data and simulations. Brain Res Bull. (1993) 31:97–113. doi: 10.1016/0361-9230(93)90016-5
3. Tan X, Campbell IG, Feinberg I. Internight reliability and benchmark values for computer analyses of non-rapid eye movement (NREM) and REM EEG in normal young adult and elderly subjects. Clin Neurophysiol. (2001) 112:1540–52. doi: 10.1016/S1388-2457(01)00570-3
4. Tan X, Campbell IG, Palagini L, Feinberg I. High internight reliability of computer-measured NREM delta, sigma, and beta: biological implications. Biol Psychiatry (2000) 48:1010–9. doi: 10.1016/S0006-3223(00)00873-8
5. Tucker AM, Dinges DF, Van Dongen HP. Trait interindividual differences in the sleep physiology of healthy young adults. J Sleep Res. (2007) 16:170–80. doi: 10.1111/j.1365-2869.2007.00594.x
6. Ermis U, Krakow K, Voss U. Arousal thresholds during human tonic and phasic REM sleep. J Sleep Res. (2010) 19:400–6. doi: 10.1111/j.1365-2869.2010.00831.x
7. Armitage R. The distribution of EEG frequencies in REM and NREM sleep stages in healthy young adults. Sleep (1995) 18:334–41. doi: 10.1093/sleep/18.5.334
8. Ehlers CL, Kupfer DJ. Slow-wave sleep: do young adult men and women age differently ? J Sleep Res. (1997) 6:211–5. doi: 10.1046/j.1365-2869.1997.00041.x
9. Dijk DJ. Regulation and functional correlates of slow wave sleep. J Clin Sleep Med. (2009) 5:S6–15.
10. Ratnavadivel R, Chau N, Stadler D, Yeo A, McEvoy RD, Catcheside PG. Marked reduction in obstructive sleep apnea severity in slow wave sleep. J Clin Sleep Med. (2009) 5:519–24.
11. Subramanian S, Hesselbacher S, Mattewal A, Surani S. Gender and age influence the effects of slow-wave sleep on respiration in patients with obstructive sleep apnea. Sleep Breath. (2013) 17:51–6. doi: 10.1007/s11325-011-0644-4
12. Basner RC, Ringler J, Schwartzstein RM, Weinberger SE, Weiss JW. Phasic electromyographic activity of the genioglossus increases in normals during slow-wave sleep. Respir Physiol. (1991) 83:189–200. doi: 10.1016/0034-5687(91)90028-H
13. McSharry DG, Saboisky JP, Deyoung P, Matteis P, Jordan AS, Trinder J, et al. A mechanism for upper airway stability during slow wave sleep. Sleep (2013) 36:555–63. doi: 10.5665/sleep.2544
14. Eckert DJ, Owens RL, Kehlmann GB, Wellman A, Rahangdale S, Yim-Yeh S, et al. Eszopiclone increases the respiratory arousal threshold and lowers the apnoea/hypopnoea index in obstructive sleep apnoea patients with a low arousal threshold. Clin Sci. (2011) 120:505–14. doi: 10.1042/CS20100588
15. Berry RB, Asyali MA, McNellis MI, Khoo MC. Within-night variation in respiratory effort preceding apnea termination and EEG delta power in sleep apnea. J Appl Physiol (1985). (1998) 85:1434–41.
16. Peppard PE, Young T, Barnet JH, Palta M, Hagen EW, Hla KM. Increased prevalence of sleep-disordered breathing in adults. Am J Epidemiol. (2013) 177:1006–14. doi: 10.1093/aje/kws342
17. Heinzer R, Vat S, Marques-Vidal P, Marti-Soler H, Andries D, Tobback N, et al. Prevalence of sleep-disordered breathing in the general population: the HypnoLaus study. Lancet Respir Med. (2015) 3:310–8. doi: 10.1016/S2213-2600(15)00043-0
18. Redline S, Kump K, Tishler P, Browner I, Ferrette V. Gender differences in sleep disordered breathing in a community-based sample. Am J Respir Crit Care Med. (1994) 149:722–6. doi: 10.1164/ajrccm.149.3.8118642
19. Young T, Hutton R, Finn L, Badr S, Palta M. The gender bias in sleep apnea diagnosis: are women missed because they have different symptoms? Arch Int Med. (1996) 156:2445–51. doi: 10.1001/archinte.1996.00440200055007
20. Vagiakis E, Kapsimalis F, Lagogianni I, Perraki H, Minaritzoglou A, Alexandropoulou K, et al. Gender differences on polysomnographic findings in Greek subjects with obstructive sleep apnea syndrome. Sleep Med. (2006) 7:424–30. doi: 10.1016/j.sleep.2005.12.014
21. Punjabi NM. The epidemiology of adult obstructive sleep apnea. Proc Am Thorac Soc. (2008) 5:136–43. doi: 10.1513/pats.200709-155MG
22. Penev P, Spiegel K, L'Hermite-Baleriaux M, Schneider R, Van Cauter E. Relationship between REM sleep and testosterone secretion in older men. Ann Endocrinol (Paris). (2003) 64:157.
23. Leproult R, Van Cauter E. Effect of 1 week of sleep restriction on testosterone levels in young healthy men. JAMA (2011) 305:2173–4. doi: 10.1001/jama.2011.710
24. Luboshitzky R, Zabari Z, Shen-Orr Z, Herer P, Lavie P. Disruption of the nocturnal testosterone rhythm by sleep fragmentation in normal men. J Clin Endocrinol Metab. (2001) 86:1134–9. doi: 10.1210/jcem.86.3.7296
25. Santamaria JD, Prior JC, Fleetham JA. Reversible reproductive dysfunction in men with obstructive sleep apnoea. Clin Endocrinol (Oxf). (1988) 28:461–70. doi: 10.1111/j.1365-2265.1988.tb03680.x
26. Grunstein RR, Handelsman DJ, Lawrence SJ, Blackwell C, Caterson ID, Sullivan CE. Neuroendocrine dysfunction in sleep apnea: reversal by continuous positive airways pressure therapy. J Clin Endocrinol Metab. (1989) 68:352–8. doi: 10.1210/jcem-68-2-352
27. Zhang XB, Lin QC, Zeng HQ, Jiang XT, Chen B, Chen X. Erectile dysfunction and sexual hormone levels in men with obstructive sleep apnea: efficacy of continuous positive airway pressure. Arch Sex Behav. (2016) 45:235–40. doi: 10.1007/s10508-015-0593-2
28. Sandblom RE, Matsumoto AM, Schoene RB, Lee KA, Giblin EC, Bremner WJ, et al. Obstructive sleep apnea syndrome induced by testosterone administration. New Eng J Med. (1983) 308:508–10. doi: 10.1056/NEJM198303033080908
29. Schneider BK, Pickett CK, Zwillich CW, Weil JV, McDermott MT, Santen RJ, et al. Influence of testosterone on breathing during sleep. J Appl Physiol (1985). (1986) 61:618–23.
30. Cistulli PA, Grunstein RR, Sullivan CE. Effect of testosterone administration on upper airway collapsibility during sleep. Am J Respir Crit Care Med. (1994) 149:530–2. doi: 10.1164/ajrccm.149.2.8306057
31. Liu PY, Yee B, Wishart SM, Jimenez M, Jung DG, Grunstein RR, et al. The short-term effects of high-dose testosterone on sleep, breathing, and function in older men. J Clin Endocrinol Metab. (2003) 88:3605–13. doi: 10.1210/jc.2003-030236
32. Hoyos CM, Killick R, Yee BJ, Grunstein RR, Liu PY. Effects of testosterone therapy on sleep and breathing in obese men with severe obstructive sleep apnoea: a randomized placebo-controlled trial. Clin Endocrinol (Oxf). (2012) 77:599–607. doi: 10.1111/j.1365-2265.2012.04413.x
33. Mateika JH, Omran Q, Rowley JA, Zhou XS, Diamond MP, Badr MS. Treatment with leuprolide acetate decreases the threshold of the ventilatory response to carbon dioxide in healthy males. J Physiol. (2004) 561:637–46. doi: 10.1113/jphysiol.2004.071811
34. Chowdhuri S, Bascom A, Mohan D, Diamond MP, Badr MS. Testosterone conversion blockade increases breathing stability in healthy men during NREM sleep. Sleep (2013) 36:1793–8. doi: 10.5665/sleep.3202
35. Zhou XS, Rowley JA, Demirovic F, Diamond MP, Badr MS. Effect of testosterone on the apneic threshold in women during NREM sleep. J Appl Physiol (1985). (2003) 94:101–7. doi: 10.1152/japplphysiol.00264.2002
36. Rosenfield RL, Mortensen M, Wroblewski K, Littlejohn E, Ehrmann DA. Determination of the source of androgen excess in functionally atypical polycystic ovary syndrome by a short dexamethasone androgen-suppression test and a low-dose ACTH test. Hum. Reprod. (2011) 26:3138–46. doi: 10.1093/humrep/der291
37. Moll GW Jr., Rosenfield RL. Testosterone binding and free plasma androgen concentrations under physiological conditons: chararacterization by flow dialysis technique. J Clin Endocrinol Metab. (1979) 49:730–6. doi: 10.1210/jcem-49-5-730
38. Moll GW Jr., Rosenfield RL, Helke JH. Estradiol-testosterone binding interactions and free plasma estradiol under physiological conditions. J Clin Endocrinol Metab. (1981) 52:868–74. doi: 10.1210/jcem-52-5-868
39. Iber C, Ancoli-Israel S, Chesson AJ, Quan SF. The AASM Manual for the Scoring of Sleep and Associated Events: Rules, Terminology and Technical Specifications. Westchester, IL: American Academy of Sleep Medicine (2007).
40. Berry R, Budhiraja R, Gottlieb D, Gozal D, Iber C, Kapur V, et al. Rules for scoring respiratory events in sleep: update of the 2007 AASM manual for the scoring of sleep and associated events. Deliberations of the sleep apnea definitions task force of the American Academy of Sleep Medicine. J Clin Sleep Med JCSM Off Publ Am Acad Sleep Med. (2012) 8:597–619. doi: 10.5664/jcsm.2172
41. Latta F, Leproult R, Tasali E, Hofmann E, Van Cauter E. Sex differences in delta and alpha EEG activities in healthy older adults. Sleep (2005) 28:1525–34. doi: 10.1093/sleep/28.12.1525
42. Feinberg I, Floyd TC. Systematic trends across the night in human sleep cycles. Psychophysiology (1979) 16:283–91. doi: 10.1111/j.1469-8986.1979.tb02991.x
43. Hume KI, Van F, Watson A. A field study of age and gender differences in habitual adult sleep. J Sleep Res. (1998) 7:85–94. doi: 10.1046/j.1365-2869.1998.00103.x
44. Kobayashi R, Kohsaka M, Fukuda N, Honma H, Sakakibara S, Koyama T. Gender differences in the sleep of middle-aged individuals. Psychiatry Clin Neurosci. (1998) 52:186–7. doi: 10.1111/j.1440-1819.1998.tb01021.x
45. Redline S, Kirchner HL, Quan SF, Gottlieb DJ, Kapur V, Newman A. The effects of age, sex, ethnicity, and sleep-disordered breathing on sleep architecture. Arch Intern Med. (2004) 164:406–18. doi: 10.1001/archinte.164.4.406
46. Bixler EO, Papaliaga MN, Vgontzas AN, Lin HM, Pejovic S, Karataraki M, et al. Women sleep objectively better than men and the sleep of young women is more resilient to external stressors: effects of age and menopause. J Sleep Res. (2009) 18:221–8. doi: 10.1111/j.1365-2869.2008.00713.x
47. Mourtazaev MS, Kemp B, Zwinderman AH, Kamphuisen HAC. Age and gender affect different characteristics of slow waves in the sleep EEG. Sleep (1995) 18:557–64. doi: 10.1093/sleep/18.7.557
48. Guilleminault C, Do Kim Y, Chowdhuri S, Horita M, Ohayon M, Kushida C. Sleep and daytime sleepiness in upper airway resistance syndrome compared to obstructive sleep apnoea syndrome. Eur Respir J. (2001) 17:838–47. doi: 10.1183/09031936.01.17508380
49. O'Connor C, Thornley KS, Hanly PJ. Gender differences in the polysomnographic features of obstructive sleep apnea. Am J Respir Crit Care Med. (2000) 161:1465–72. doi: 10.1164/ajrccm.161.5.9904121
50. Koo BB, Dostal J, Ioachimescu O, Budur K. The effects of gender and age on REM-related sleep-disordered breathing. Sleep Breath. (2008) 12:259–64. doi: 10.1007/s11325-007-0161-7
51. Conwell W, Patel B, Doeing D, Pamidi S, Knutson KL, Ghods F, et al. Prevalence, clinical features, and CPAP adherence in REM-related sleep-disordered breathing: a cross-sectional analysis of a large clinical population. Sleep Breath. (2012) 16:519–26. doi: 10.1007/s11325-011-0537-6
52. Dijk DJ, Beersma DG, Bloem GM. Sex differences in the sleep EEG of young adults: visual scoring and spectral analysis. Sleep (1989) 12:500–7. doi: 10.1093/sleep/12.6.500
53. Robillard R, Massicotte-Marquez J, Kawinska A, Paquet J, Frenette S, Carrier J. Topography of homeostatic sleep pressure dissipation across the night in young and middle-aged men and women. J Sleep Res. (2010) 19:455–65. doi: 10.1111/j.1365-2869.2010.00820.x
54. Van Cauter E, Leproult R, Plat L. Age-related changes in slow wave sleep and REM sleep and relationship with growth hormone and cortisol levels in healthy men. J Am Med Assoc. (2000) 284:861–8. doi: 10.1001/jama.284.7.861
55. Armitage R, Hoffmann RF. Sleep EEG, depression and gender. Sleep Med. Rev. (2001) 5:237–46. doi: 10.1053/smrv.2000.0144
56. Carrier J, Viens I, Poirier G, Robillard R, Lafortune M, Vandewalle G, et al. Sleep slow wave changes during the middle years of life. Eur J Neurosci. (2011) 33:758–66. doi: 10.1111/j.1460-9568.2010.07543.x
57. Luboshitzky R, Lavie L, Shen-Orr Z, Lavie P. Pituitary-gonadal function in men with obstructive sleep apnea. The effect of continuous positive airways pressure treatment. Neuro Endocrinol Lett. (2003) 24:463–7.
58. Harman SM, Metter EJ, Tobin JD, Pearson J, Blackman MR, Baltimore Longitudinal Study of A. Longitudinal effects of aging on serum total and free testosterone levels in healthy men. Baltimore Longitudinal Study of Aging. J Clin Endocrinol Metab. (2001) 86:724–31. doi: 10.1210/jcem.86.2.7219
59. Luboshitzky R, Aviv A, Hefetz A, Herer P, Shen-Orr Z, Lavie L, et al. Decreased pituitary-gonadal secretion in men with obstructive sleep apnea. J Clin Endocrinol Metab. (2002) 87:3394–8. doi: 10.1210/jcem.87.7.8663
60. Bratel T, Wennlund A, Carlstrom K. Pituitary reactivity, androgens and catecholamines in obstructive sleep apnoea. Effects of continuous positive airway pressure treatment (CPAP). Respir Med. (1999) 93:1–7. doi: 10.1016/S0954-6111(99)90068-9
61. Meston N, Davies RJ, Mullins R, Jenkinson C, Wass JA, Stradling JR. Endocrine effects of nasal continuous positive airway pressure in male patients with obstructive sleep apnoea. J Intern Med. (2003) 254:447–54. doi: 10.1046/j.1365-2796.2003.01212.x
62. Hoekema A, Stel AL, Stegenga B, van der Hoeven JH, Wijkstra PJ, van Driel MF, et al. Sexual function and obstructive sleep apnea-hypopnea: a randomized clinical trial evaluating the effects of oral-appliance and continuous positive airway pressure therapy. J Sex Med. (2007) 4:1153–62. doi: 10.1111/j.1743-6109.2006.00341.x
63. Celec P, Mucska I, Ostatnikova D, Hodosy J. Testosterone and estradiol are not affected in male and female patients with obstructive sleep apnea treated with continuous positive airway pressure. J Endocrinol Invest. (2014) 37:9–12. doi: 10.1007/s40618-013-0003-3
64. Knapp A, Myhill PC, Davis WA, Peters KE, Hillman D, Hamilton EJ, et al. Effect of continuous positive airway pressure therapy on sexual function and serum testosterone in males with type 2 diabetes and obstructive sleep apnoea. Clin Endocrinol (Oxf). (2014) 81:254–8. doi: 10.1111/cen.12401
65. Bhasin S, Cunningham GR, Hayes FJ, Matsumoto AM, Snyder PJ, Swerdloff RS, et al., Task Force ES. Testosterone therapy in men with androgen deficiency syndromes: an Endocrine Society clinical practice guideline. J Clin Endocrinol Metab. (2010) 95:2536–59. doi: 10.1210/jc.2009-2354
66. Ishizuka Y, Pollak CP, Shirakawa S, Kakuma T, Azumi K, Usui A, et al. Sleep spindle frequency changes during the menstrual cycle. J Sleep Res. (1994) 3:26–9. doi: 10.1111/j.1365-2869.1994.tb00100.x
67. Driver HS, Dijk DJ, Werth E, Biedermann K, Borbely AA. Sleep and the sleep electroencephalogram across the menstrual cycle in young healthy women. J Clin Endocrinol Metab. (1996) 81:728–35.
68. Driver HS, McLean H, Kumar DV, Farr N, Day AG, Fitzpatrick MF. The influence of the menstrual cycle on upper airway resistance and breathing during sleep. Sleep (2005) 28:449–56. doi: 10.1093/sleep/28.4.449
Keywords: obstructive sleep apnea, sex differences, slow wave sleep, slow wave activity, delta activity, testosterone, spectral analysis
Citation: Morselli LL, Temple KA, Leproult R, Ehrmann DA, Van Cauter E and Mokhlesi B (2018) Determinants of Slow-Wave Activity in Overweight and Obese Adults: Roles of Sex, Obstructive Sleep Apnea and Testosterone Levels. Front. Endocrinol. 9:377. doi: 10.3389/fendo.2018.00377
Received: 28 March 2018; Accepted: 22 June 2018;
Published: 12 July 2018.
Edited by:
GianLuca Colussi, Università degli Studi di Udine, ItalyReviewed by:
Licio A. Velloso, Universidade Estadual de Campinas, BrazilThomas Scherer, Medizinische Universität Wien, Austria
Copyright © 2018 Morselli, Temple, Leproult, Ehrmann, Van Cauter and Mokhlesi. This is an open-access article distributed under the terms of the Creative Commons Attribution License (CC BY). The use, distribution or reproduction in other forums is permitted, provided the original author(s) and the copyright owner(s) are credited and that the original publication in this journal is cited, in accordance with accepted academic practice. No use, distribution or reproduction is permitted which does not comply with these terms.
*Correspondence: Babak Mokhlesi, Ym1va2hsZXNAbWVkaWNpbmUuYnNkLnVjaGljYWdvLmVkdQ==
†Co-senior authors.