- 1Department of Psychology, University of British Columbia, Vancouver, BC, Canada
- 2Djavad Mowafaghian Centre for Brain Health, University of British Columbia, Vancouver, BC, Canada
- 3Neuroscience Graduate Program, University of Southern California, Los Angeles, CA, United States
- 4Department of Integrative Anatomical Sciences, Keck School of Medicine of the University of Southern California, Los Angeles, CA, United States
- 5Department of Zoology, University of British Columbia, Vancouver, BC, Canada
Multiple lines of evidence indicate that androgens, such as testosterone, modulate the mesocorticolimbic system and executive function. This review integrates neuroanatomical, molecular biological, neurochemical, and behavioral studies to highlight how endogenous and exogenous androgens alter behaviors, such as behavioral flexibility, decision making, and risk taking. First, we briefly review the neuroanatomy of the mesocorticolimbic system, which mediates executive function, with a focus on the ventral tegmental area (VTA), nucleus accumbens (NAc), medial prefrontal cortex (mPFC), and orbitofrontal cortex (OFC). Second, we present evidence that androgen receptors (AR) and other steroid receptors are expressed in the mesocorticolimbic system. Using sensitive immunohistochemistry and quantitative polymerase chain reaction (qPCR) techniques, ARs are detected in the VTA, NAc, mPFC, and OFC. Third, we describe recent evidence for local androgens (“neuroandrogens”) in the mesocorticolimbic system. Steroidogenic enzymes are expressed in mesocorticolimbic regions. Furthermore, following long-term gonadectomy, testosterone is nondetectable in the blood but detectable in the mesocorticolimbic system, using liquid chromatography tandem mass spectrometry. However, the physiological relevance of neuroandrogens remains unknown. Fourth, we review how anabolic-androgenic steroids (AAS) influence the mesocorticolimbic system. Fifth, we describe how androgens modulate the neurochemistry and structure of the mesocorticolimbic system, particularly with regard to dopaminergic signaling. Finally, we discuss evidence that androgens influence executive functions, including the effects of androgen deprivation therapy and AAS. Taken together, the evidence indicates that androgens are critical modulators of executive function. Similar to dopamine signaling, there might be optimal levels of androgen signaling within the mesocorticolimbic system for executive functioning. Future studies should examine the regulation and functions of neurosteroids in the mesocorticolimbic system, as well as the potential deleterious and enduring effects of AAS use.
Introduction
Berthold first reported the masculinizing effects of a bloodborne “substance” produced by the testes in male chicks (1). This substance is now known to belong to a class of steroids called androgens, which are synthesized by the male gonads and released into the circulatory system to regulate development, physiology, and behavior. Endogenous androgens are 19-carbon (C19) steroids and include testosterone (T) and its metabolite 5α-dihydrotestosterone (DHT), which have the most pronounced androgenic effects. Other C19 steroids include T precursors such as dehydroepiandrosterone (DHEA) and androstenedione (Figure 1). Androgen synthesis from cholesterol occurs in the Leydig cells of the testes, stromal, and thecal cells of the ovaries, and the zona reticularis of the adrenal cortices in some mammalian species (2, 3).
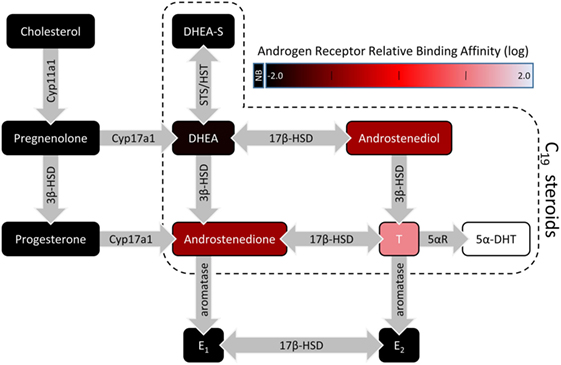
Figure 1. A simplified illustration of the steroidogenic pathway with a focus on C19 steroids. For C19 steroids, fill color represents the relative binding affinity to the androgen receptor (4–6). Steroidogenic enzymes are represented by the gray arrows. Abbreviations: 3β-HSD, 3β-hydroxysteroid dehydrogenase/isomerase; 5αR, 5α-reductase; 5α-DHT, 5α-dihydrotestosterone; 17β-HSD, 17β-hydroxysteroid dehydrogenase; DHEA, dehydroepiandrosterone; DHEA-S, dehydroepiandrosterone sulfate; E1, estrone; E2, 17β-estradiol; HST, hydroxysteroid sulfotransferase; STS, steroid sulfatase; NB, no binding.
Numerous studies examining the effects of gonadectomy (GDX), androgen receptor (AR) antagonists, androgen synthesis inhibitors, androgen replacement, and administration of supraphysiological amounts of androgens [i.e., anabolic-androgenic steroids (AAS)] demonstrate that androgens are critical for reproductive behavior [reviewed in Ref. (7)] and aggressive behavior [reviewed in Ref. (8, 9)]. However, recent research has revealed that more complex behaviors and cognitive processes, such as executive function, are also regulated by androgens. We will review research that examines the role of androgens in regulating the neural circuitry that mediates executive function and behaviors associated with executive function.
In the first section “The mesocorticolimbic system and executive function,” we give a brief overview of the mesocorticolimbic system and its involvement in various executive functions. In the second section, we describe evidence for the presence of sex steroid receptors in the mesocorticolimbic system. In the third section, we summarize recent work that provides strong evidence for local synthesis of androgens and estrogens within the mesocorticolimbic system. In the fourth section, we discuss how AAS modulate the mesocorticolimbic system. In the fifth section, we explore how androgens alter neurochemical signaling and cytoarchitecture in nodes of the mesocorticolimbic system. Finally, in the last section, we review preclinical and clinical studies demonstrating that GDX, AAS, and perhaps local production of androgens influence executive functions such as behavioral flexibility and inhibitory control. Like many other neuromodulator systems, androgen signaling levels are likely maintained at particular levels within different brain regions to achieve optimal executive function.
The Mesocorticolimbic System and Executive Function
Executive functions are a collection of cognitive operations that interact to facilitate selection and implementation of behaviors to attain chosen goals. More basic operations include selective attention, inhibitory control, and working memory (i.e., temporary maintenance and manipulation of information). These operations work in concert with those processed by other mnemonic, affective, and motivational systems to regulate more complex processes such as cognitive flexibility and cost/benefit decision making. It is well established from lesion and functional imaging studies in humans and non-human animals that various aspects of executive functioning are critically dependent on different regions of the prefrontal cortex (PFC) and its interactions with striatal regions, including the nucleus accumbens (NAc; Figure 2).
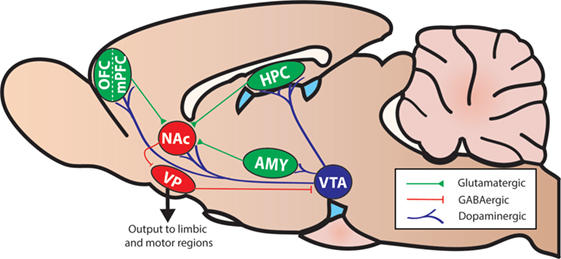
Figure 2. A simplified diagram of the mesocorticolimbic system and associated structures in the rodent brain (sagittal view). Abbreviations: AMY, amygdala; HPC, hippocampus; NAc, nucleus accumbens; mPFC, medial prefrontal cortex; OFC, orbitofrontal cortex; VP, ventral pallidum; VTA, ventral tegmental area.
The PFC and the NAc receive dopamine (DA) input from the ventral tegmental area (VTA) in the midbrain, and DA transmission within these regions plays a key role in facilitating both basic and more complex functions mediated by these circuits. Thus, the seminal findings of Brozoski et al. (10) revealed that DA depletion in the frontal lobes of monkeys markedly impairs working memory, and subsequent psychopharmacological studies revealed that these functions are dependent primarily on PFC D1 receptor (D1R) activity [reviewed in Ref. (11)]. Different forms of cognitive flexibility are also dependent on DA activity within the frontal lobes and/or striatal regions. For example, shifts between strategies, rules, or attentional sets are dependent on DA transmission in both the medial prefrontal cortex (mPFC) and NAc. D2 receptors (D2R) in the PFC facilitate suppression of old strategies, whereas D1R in the PFC and NAc facilitate establishment and maintenance of new strategies (11–14). In comparison, reversal learning is a simpler form of cognitive flexibility, entailing a shift between stimulus–reinforcement associations (i.e., use the same basic strategy, but approach a different stimulus). The orbitofrontal cortex (OFC) plays a key role in mediating reversal learning in both primates and rats (15, 16). Reversal learning is generally unimpaired by global depletion of PFC DA (17), and DA input to dorsal striatal regions appears more crucial to this form of flexibility (18, 19).
Dopamine transmission in prefrontal–striatal circuitry also mediates evaluative functions entailing a choice between a smaller, readily available reward vs. a larger/more palatable reward associated with some form of cost, which can diminish the subjective value of objectively larger or more-preferred rewards. These forms of decision making are exquisitely sensitive to manipulation of DA transmission, in that systemic treatment with DA antagonists reduces preference for larger rewards associated with a greater effort cost or uncertainty (20–22). However, the mechanisms through which DA regulates choice behavior can vary across different nodes of the mesocorticolimbic circuit. For example, blockade of D1R, but not D2R, in the NAc reduces risky choice (23), whereas blockade of either receptor in the NAc diminishes preference for more preferred rewards associated with a greater effort cost (24). Likewise, blockade of D1R, but not D2R, in different subregions of the mPFC shifts preference away from more costly rewards (25, 26) and also makes animals more risk-averse (27). Yet, blockade of PFC D2R impairs modifications of decision biases in response to changes in risk/reward contingencies (27, 28). Collectively, these studies indicate that DA transmission within different nodes of the mesocorticolimbic system helps to refine different types of decision making by promoting choice toward larger, yet more costly, rewards, and modifying decision biases when cost/benefit contingencies change. The critical involvement of DA in various executive functions suggests that other signals that can influence DA signaling, such as sex steroids, may also influence these functions.
The Mesocorticolimbic System Contains Sex-Steroid Receptors
Multiple lines of evidence indicate that receptors for sex steroids are present in the VTA, NAc, mPFC, and OFC. Here, we focus on the classical AR, the estrogen receptors (ER)α and ERβ, and more recently discovered membrane-associated androgen receptors (mAR) and ER (mER). We briefly discuss androgen metabolites that can act via allosteric binding sites on neurotransmitter receptors.
Androgens can act on target cells by binding to intracellular AR. Of the endogenous androgens, T and DHT have the highest binding affinities for AR, while DHEA, androstenedione, and androstenediol have weak binding affinities for AR [(4–6); Figure 1]. AAS have a wide range of binding affinities for AR, and users select different AAS according to the balance of desired anabolic (myotrophic) actions and unwanted side-effects (e.g., gynecomastia).
Androgens are lipophilic and non-polar, and thus they can pass through the blood–brain barrier and then the plasma membrane of cells to bind with AR in the cytosol. This ligand–receptor complex then dimerizes, is phosphorylated, and translocates to the cell nucleus, where the DNA-binding domain binds to a specific sequence of DNA called the hormone response element and acts as a transcription factor (29). Such genomic effects are responsible for many of the peripheral effects of androgens, such as enhancing muscle growth (30). ARs are also found in multiple brain regions. Generally, ARs are found in the highest concentrations in hypothalamic and limbic regions that regulate homeostatic functions, reproductive behaviors, and aggressive behaviors (31). For example, male mice with reduced AR in the nervous system show decreases in mating and aggression (32).
One way in which androgens might influence executive function is through direct actions on the mesocorticolimbic system. ARs are expressed in regions of the mesocorticolimbic system, albeit at lower levels than in the hypothalamus. In particular, the VTA, NAc, and mPFC express low to moderate levels of AR in male and female rodents (33–37), non-human primates (38, 39), and humans (40). Using microdissected tissue from mesocorticolimbic nodes, we recently demonstrated AR mRNA in the VTA, NAc, and mPFC using sensitive and specific probe-based quantitative polymerase chain reaction (qPCR) assays (36). The presence of AR protein immunoreactivity (AR-ir) in these regions has also been reported; however, the number of AR per cell is low, which results in immunohistochemical staining that is faint, challenging to quantify, and easy to overlook (41). One reason is that, in extrahypothalamic regions, androgen receptor immunoreactivity (AR-ir) is often located in neuronal processes and not concentrated in neuronal nuclei. Nonetheless, there are many processes and nuclei that express AR in the cerebral cortex, which has been verified by immunoelectron microscopy (35, 41). By adding a Tyramide Signal Amplification (TSA) step in the immunohistochemistry protocol, we recently showed that AR-ir cells are present in the VTA, NAc, mPFC, and OFC [(33); Figures 3 and 4]. Double-label immunofluorescence coupled with confocal microscopy demonstrates that AR-ir cells in the PFC are neurons (Figure 3). In the VTA, AR-ir cells express tyrosine hydroxylase (TH), a marker of DA-synthetic neurons (42). Furthermore, perikarya in the VTA that project to the NAc and mPFC express AR (43). Of the VTA neurons that project to the prelimbic mPFC (mPFC-PL), the proportion of DAergic (TH-positive) efferents containing AR is higher in male rats than female rats (~30 vs <5%), but the proportion of TH-negative efferents containing AR is similar between males and females (44). Thus, androgens can influence the male mPFC via actions on these DAergic projection neurons (42). Taken together, these data suggest that AR are well positioned to modulate executive function.
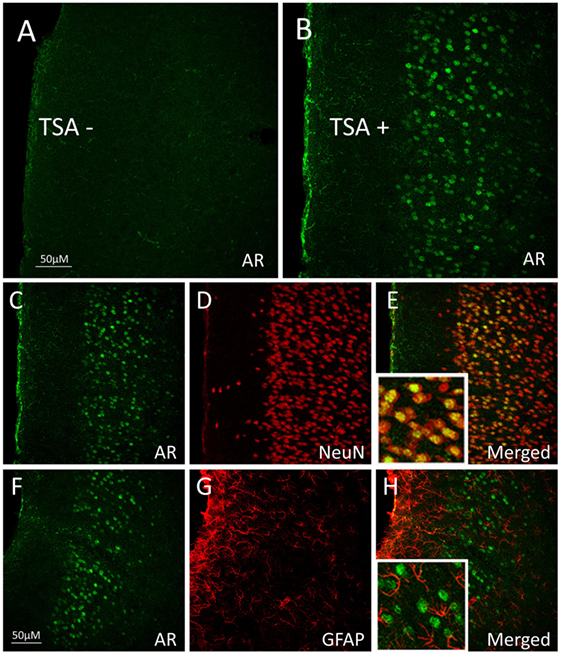
Figure 3. Androgen receptor (AR), neuronal nuclei (NeuN, neuronal marker), and glial fibrillary acidic protein (GFAP; glial marker) immunoreactivity (ir) in the medial prefrontal cortex (mPFC) of adult male rats. (A,B) Pseudocolored confocal photomicrographs of androgen receptor immunoreactivity (AR-ir) in coronal hemisections of the mPFC (A) without tyramide signal amplification (TSA−) and (B) with tyramide signal amplification (TSA+). TSA-enhanced detection of AR in the mPFC of male rats. (C–E) Confocal photomicrographs of mPFC with (C) AR-ir cells (green), (D) NeuN-ir cells (red), and (E) AR-ir and NeuN-ir cells merged. Cells that co-express AR-ir and NeuN-ir appear orange–yellow, suggesting that AR is primarily expressed in neurons. (F–H) Confocal photomicrographs of (F) AR-ir cells (green), (G) GFAP-ir cells (red), and (H) AR-ir and GFAP-ir cells merged. AR-ir is not co-expressed with GFAP. Adapted from Ref. (33); Reprinted by permission of SAGE Publications.
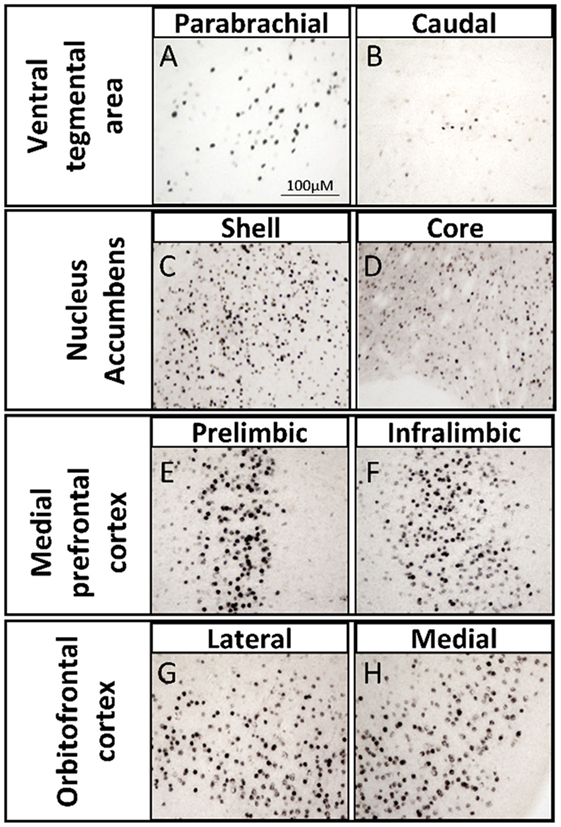
Figure 4. Brightfield photomicrographs depicting androgen receptor immunoreactivity (AR-ir) with tyramide signal amplification in nodes of the mesocorticolimbic system of adult male rats. AR-ir in the (A) parabrachial pigmented nucleus of the ventral tegmental area (VTA), (B) caudal VTA, (C) shell of the nucleus accumbens (NAc), (D) core of the NAc, (E) prelimbic subregion of the medial prefrontal cortex (mPFC), (F) infralimbic subregion of the mPFC, (G) lateral subregion of the orbitofrontal cortex (OFC), and (H) medial subregion of the OFC. Adapted from Ref. (45).
In addition, T can be locally aromatized to E2 and bind to ER in the mesocorticolimbic system (Figure 1). Many brain regions contain aromatase, the enzyme that catalyzes the conversion of androgens to estrogens (36). Aromatase expression is high in the hypothalamus (46, 47), and aromatase is also present in other regions including the mesocorticolimbic system (see below). The VTA, NAc, and mPFC contain some cells that express ERα or ERβ in female and male rats (48, 49). However, the VTA neurons that project to the NAc do not express ERβ. Instead, in both sexes, VTA neurons that express ERβ project principally to the ventral caudate putamen and amygdala (43). VTA neurons that project to mPFC-PL (TH-positive and TH-negative) lack ERα and less than 10% contain ERβ (44). In general, in female and male rodents, the NAc also has little intracellular ERα and ERβ (49–51).
In addition, androgens can modulate the mesocorticolimbic system through other mechanisms. First, hypothalamic nuclei that have high concentrations of AR, ERα, and ERβ directly innervate mesocorticolimbic nodes and influence DA release. For example, the medial preoptic area is rich in AR and ERs and projects to the VTA and modulates DAergic neurons (52–54). Second, mAR and mER might mediate the rapid, nongenomic effects of androgens in the mesocorticolimbic circuit. Two possible candidates for mAR are ZIP9 and GPRC6A (55–57). However, no studies have examined ZIP9 or GPRC6A in mesocorticolimbic nodes, and whole-brain analyses have not reported either transcript in the VTA, NAc, or mPFC in mice (58, 59) or humans (40). In addition, AR variants have been found in neuronal lipid rafts (60). Alternatively, the G protein–coupled estrogen receptor 1 (GPER1; formerly known as GPR30) is present in the VTA, NAc, and, to a lesser extent, the PFC in rats and humans (61–63). Thus, systemic and locally synthesized estrogens could act on the mesocorticolimbic system via GPER1. Third, some C19 steroids can rapidly (milliseconds to seconds) modulate neuronal excitability via allosteric binding sites on neurotransmitter receptors, voltage-gated channels, and neurotrophin receptors [reviewed in Ref. (64)]. For example, the γ-aminobutyric acid (GABA)-gated chloride channel GABAA receptor (GABAAR) and the glutamate-gated sodium/calcium channel N-methyl-D-aspartate receptor are sensitive to allosteric regulation by DHEA, DHEA-S, and 3α-androstanediol (65, 66).
The Mesocorticolimbic System Locally Synthesizes Androgens
Our understanding of the role of androgens in the brain changed dramatically with the first suggestion of steroid synthesis in the rodent brain. Baulieu, Robel, and colleagues (67, 68) originally suggested that levels of DHEA and pregnenolone and their sulfoconjugates were higher in grossly dissected regions of the male rat brain (i.e., divided into the “anterior” and “posterior” brain) than in the serum. Moreover, GDX and adrenalectomy did not eliminate these steroids in the brain. Later, Liere and colleagues described how these findings were actually artifacts resulting from sample preparation, including oxidation of cholesterol in brain tissue (69). Recent studies, however, have shown that androgens are present at higher levels in several brain regions than in the blood in male rats [e.g., (36)], are directly synthesized in the brain in female and male rats [e.g., (70, 71)], or metabolized in the brain [e.g., (72)]. Local production of neurosteroids serves to influence gene expression or neuron excitability in an intracrine, paracrine, autocrine, or synaptocrine manner under normal physiological conditions (73) or as a compensatory mechanism when circulating steroid levels are low (74).
The steroidogenic capacity of the brain is further corroborated by studies demonstrating that steroidogenic enzymes are present in the brain. In many of the initial studies, the lower sensitivity of Northern blots, in situ hybridization, immunohistochemistry, and even PCR was insufficient to detect some steroidogenic enzymes in the brain. For example, Goascogne and colleagues (75) attempted to detect Cyp17a1 (Figure 1), which catalyzes conversion of progestins into androgens, in the rodent brain via immunostaining, but it was not until 1995 that several groups detected Cyp17a1 transcripts and protein in the brain (76–78), and even then only at very low levels or only in embryonic brains. Several labs did find other steroidogenic enzymes in the brain, including Cyp11a1 (76, 79) and aromatase (78). Guennoun and colleagues (80) detected mRNA and protein of 3β-hydroxysteroid dehydrogenase/isomerase (3β-HSD) in the hippocampus (HPC), hypothalamus, cerebellum, and cerebral cortex. Current techniques, particularly PCR, can detect all the enzymes necessary for androgen synthesis and metabolism in multiple regions in the male and female rat brain (81–84) and human brain (85, 86).
Little is known about the androgenic capacity of the mesocorticolimbic system, and even less about the physiological relevance of these locally produced steroids. Most studies have measured steroidogenic enzymes in gross neuroanatomical regions (e.g., cerebral cortex, HPC), without specific attention to mesocorticolimbic regions [e.g., (81, 87, 88)]. Specifically, Cyp11a1, Cyp17a1, and aromatase have been reported in the frontal cortex and midbrain or tegmentum of birds (89, 90), rodents (79), and humans [reviewed in Ref. (85)]. However, these reports have low spatial resolution, so steroidogenic enzyme levels specifically in the mPFC or VTA are unclear. Raab and colleagues (91) detected aromatase mRNA in the VTA of male and female rats, but only during early development. More recently, one study showed a behavioral effect of Cyp11a1 overexpression in the VTA, but not in the NAc (92). These results suggest that, if present in the VTA, Cyp11a1 affects reward-seeking behavior, but they did not demonstrate the importance of endogenous Cyp11a1 in the VTA. In the NAc, 3α-HSD and 5α-reductase type I, both involved in synthesizing DHT, are present in GABAergic medium spiny neurons of male mice (93). The steroidogenic acute regulatory protein (StAR), which is essential for de novo steroid synthesis, is also present in the NAc of mice (84).
We have recently shown expression of Cyp17a1, Cyp19a1 (aromatase), and Hsd3b1 (3β-HSD type I) mRNA in microdissected mesocorticolimbic nodes in the adult male rat using exon-spanning, probe-based qPCR assays that are specific and sensitive [(36); Figure 5]. The VTA, NAc, and mPFC contained low levels of Cyp17a1 mRNA, compared to the preoptic area/hypothalamus (POA/HYP). In the VTA, GDX decreased Cyp17a1 mRNA at the 2 weeks time point. Compared to the VTA, the NAc and the mPFC contained much higher levels of aromatase mRNA. While GDX decreased aromatase mRNA in the POA/HYP, GDX had no effect on aromatase in VTA, NAc, or mPFC. 3β-HSD type I was expressed in trace amounts in the VTA, but was nondetectable in the NAc and mPFC. This is further evidence that the mesocorticolimbic system can synthesize androgens de novo from cholesterol or from circulating steroids (DHEA, progesterone).
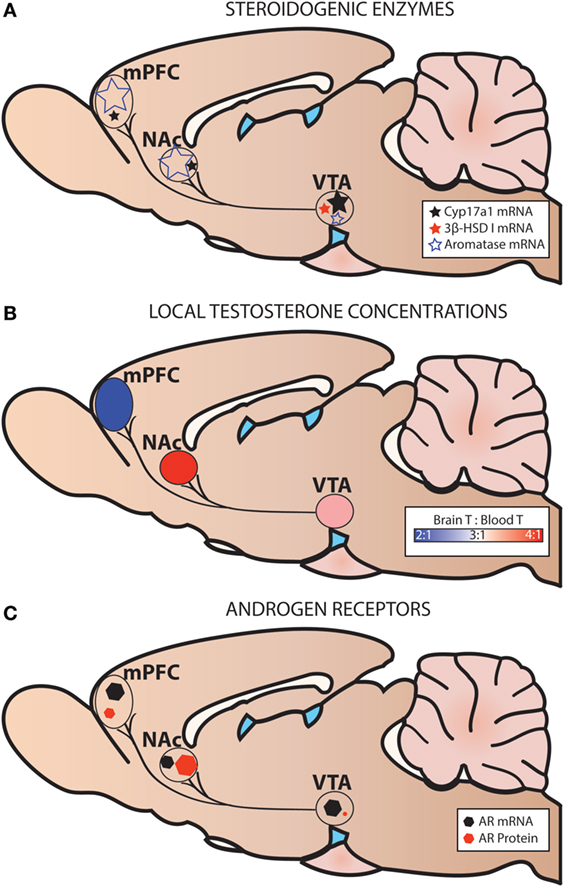
Figure 5. Steroidogenic enzymes, local testosterone concentrations, and androgen receptors (AR) in the mesocorticolimbic system of adult male rats. (A) Levels of steroidogenic enzyme mRNA are based on probe-based quantitative polymerase chain reaction (qPCR) assays (36). (B) Local testosterone (T) concentrations are based on Brain T: Blood T ratios in intact adult male rats [fed ad libitum or calorie restricted (36)]. (C) Levels of AR mRNA are based on probe-based qPCR assays (36), and levels of AR protein are based on immunohistochemistry (33). Levels of AR mRNA and AR protein are not shown relative to one another.
Using the contralateral side of the brain of the same subjects described above, we also examined steroid concentrations in mesocorticolimbic nodes via specific and ultra-sensitive liquid chromatography tandem mass spectrometry. Several results suggested local T synthesis. First, in sham-operated animals, T levels were 2–4× higher in the VTA, NAc and mPFC than in the blood (Figure 5B). Second, in all GDX subjects, T was nondetectable in the blood at 2 and 6 wks postoperatively (Figure 6). In the VTA, NAc and mPFC, T levels were lowered by GDX but nonetheless still detectable in ~50% of GDX subjects at 2 and 6 wks postoperatively (Figures 6A–D). Third, in subjects with detectable T, VTA T levels were similar in sham-operated and GDX subjects. Fourth, in GDX subjects, local T levels in the VTA might be driven by 3β-HSD type I, as Hsd3b1 mRNA was positively correlated with T levels (r = 0.316). We did not detect other significant correlations between local T concentrations and steroidogenic enzymes in GDX animals, but we did not examine all androgenic enzymes (e.g., 17β-HSD, 3β-HSD type 2). Overall, these data suggest that androgen synthesis occurs in mesocorticolimbic nodes and partially compensates for the loss of circulating T in GDX animals. Moreover, the fact that T remains at physiologically relevant levels long after GDX suggests that it exerts a significant physiological effect. Future studies should examine how other androgenic enzyme isoforms may contribute to regulation of local androgen synthesis, as well as the physiological relevance of neurally produced androgens.
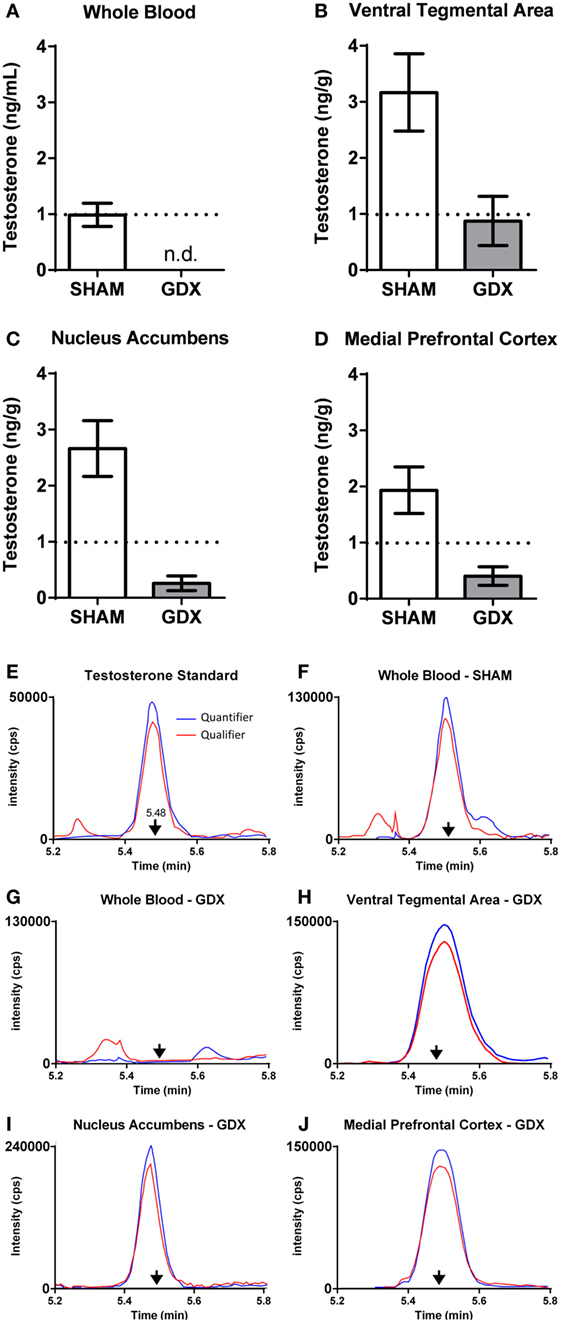
Figure 6. Testosterone is present in microdissected nodes of the mesocorticolimbic system of adult male rats at 6 weeks after GDX using LC–MS/MS. (A–D) Testosterone concentrations at 6 weeks after either SHAM surgery (n = 18–20) or GDX (n = 18–20) in the (A) whole blood, (B) ventral tegmental area (VTA), (C) nucleus accumbens (NAc), and (D) medial prefrontal cortex (mPFC). Values presented as mean ± SEM. (E–J) Representative chromatograms of testosterone quantifier ion (blue) and qualifier ion (red) for (E) testosterone standard (2 pg), (F) whole blood in a SHAM subject, (G) whole blood in a GDX subject, (H) VTA in a GDX subject, (I) NAc in a GDX subject, and (J) mPFC in a GDX subject. Arrows denote the retention time for testosterone. Note the differences in the intensity (counts per second, cps) on the y-axes. In (G–J), samples are from different subjects, as not all GDX subjects had detectable testosterone in all brain regions. Adapted from Ref. (36). Abbreviations: GDX, gonadectomy; SHAM, sham surgery; n.d., nondetectable.
The presence of steroidogenic enzyme mRNA or protein does not necessarily indicate steroidogenic enzyme activity. Few studies have demonstrated steroidogenic enzyme activity in brain cells in vitro or in vivo. In male rats, T in the cerebral cortex and tegmentum (including the VTA) is metabolized into 5α-androstanolone in vitro (94). Zwain and Yen (95) established that neonatal rat astrocytes and neurons synthesize pregnenolone, DHEA, androstenedione, T, and E2 from precursors in vitro. Furthermore, steroidogenesis was reduced when the steroidogenic enzymes were pharmacologically inhibited or when transcription was inhibited. In humans, adult and fetal brains are capable of metabolizing T and androstenedione in vitro to E2 and T, respectively (96, 97). More recently, studies demonstrate that androgens and estrogens are synthesized de novo in male and female rat hippocampal slices (70, 71, 98). Steroidogenic enzyme activity, the mesocorticolimbic system, however, has not yet been examined. What is more, whether these neurally-produced steroids modulate behavior remains largely unexplored.
AAS affect behavior via Action on the Mesocorticolimbic System
Recent studies have explored the consequences of androgen supplementation at supraphysiological (pharmacological) doses. This is relevant to the problem of AAS abuse. Importantly, when administered at pharmacological doses, AAS may act via different mechanisms from those under physiological conditions. AAS are performance-enhancing substances derived from T (99). The media focuses on AAS use among elite athletes and on steroid detection to ensure “fairness” in sport. In reality, use of AAS is far more widespread, and potential risks are only now becoming evident (100). As many as 3 million Americans have used AAS, which includes use in high schools, fitness centers, and “rejuvenation” clinics. A typical AAS user is a young man in his late teens or early 20s (100). Among U.S. high school students, 4–6% of boys have used AAS vs 1–2% of girls (101). This is comparable to the rates of crack cocaine or heroin use (101). It is estimated that AAS use among men in their 20s is even higher (100).
Commonly abused AAS include both aromatizable and non-aromatizable androgens (102). Elite athletes choose T because it is challenging to differentiate exogenous from endogenous sources (103). Rank-and-file users choose T because of its low cost and easy availability. Furthermore, most AAS users do not limit themselves to a single dose or type of steroid (104). Instead, users combine different steroids (“stacking”) in cycles of increasing and decreasing concentrations (“pyramiding”). AAS users take steroids orally, transdermally, or by intramuscular injection (105).
Recent research highlights a range of adverse health effects from chronic AAS abuse, including cardiovascular, hepatic, reproductive, and psychiatric dysfunction (105). However, the dangers of AAS abuse are not limited to the medical consequences of high-dose steroids themselves, but also result from risk-taking in non-social [e.g., drinking and driving (106)] and social contexts [e.g., aggression, sexual violence (107–110), and risky sex (106, 111, 112)]. Understanding the interplay of AAS and social behavior in risk-taking is particularly important in adolescents and young adults. This age group is strongly influenced by peer interactions (113, 114), exquisitely sensitive to rising levels of endogenous gonadal steroids (115), less risk-averse (116), and especially vulnerable to substance abuse (117). In part, this stems from adolescent immaturity in mPFC development (118).
Because it is not ethical to administer supraphysiological doses of AAS to normal volunteers, most of our knowledge of the behavioral effects of these drugs comes from studies of illicit users in the field and from animal studies. Furthermore, animal studies can explore consequences of AAS in an experimental context, where appearance and athletic performance are irrelevant. These studies have revealed that AAS appear to be rewarding and have potential to cause dependence. Rodents will voluntarily self-administer AAS orally (119) and by i.v. or i.c.v. injection (120). Moreover, they demonstrate tolerance, withdrawal, and fatal overdose with self-administration (121). T self-administration (i.c.v.) is blocked by the AR antagonist flutamide (121), although it appears that classical AR are not required for androgen reinforcement (122). The behavioral and physiological effects of supraphysiological doses of T resemble those of opioid overdose and are rapidly reversed by opioid antagonists (121). Likewise, many human AAS users meet DSM criteria for psychoactive substance dependence, including continued use despite negative side-effects, and withdrawal symptoms when steroids are discontinued (123).
The effects of AAS on reward and reinforcement strongly implicate involvement of the mesocorticolimbic system, since drugs of abuse act, in part, via DA release in NAc (124). Male rats form conditioned place preference (CPP) in response to intra-NAc infusion of T (125) or its metabolites (126), similar to the effects of DA-releasing drugs (127). Conversely, systemic or intra-NAc treatments with D1R and D2R antagonists block T-induced CPP (128, 129). Nonetheless, the manner in which androgens modulate DA release and signaling is still unclear. For example, acute administration of T does not induce NAc DA release (130), and in fact, AAS can reduce cocaine- or amphetamine-evoked DA release in NAc (131, 132). This latter finding is consistent with the observation that the acquisition of T self-administration is slow compared with cocaine or other addictive drugs (119). On the other hand, T upregulates the Fos protein, a marker of cellular activity, in regions of the mesolimbic DA system (133). Thus, the reinforcing effects of exogenous T may be due to its ability to modulate neural activity and DA signaling within the mesocorticolimbic circuit, but may do so without directly affecting DA release. Indeed, chronic AAS administration alters GABAAR subunit expression throughout the brain (including mesocorticolimbic regions), thus altering the physiological response to DA-independent GABAergic signaling (134, 135).
Androgens Modulate the Neurochemistry and Structure of the Mesocorticolimbic System
Many androgen-dependent behaviors are mediated by neurochemical changes and neuronal activity in the mesocorticolimbic system. In several mammalian species, GDX of adult males diminishes expression of copulatory behavior, which can be restored by chronic T treatment (136). Copulatory behavior, particularly ejaculation, is correlated with a T-dependent increase in DA release in the NAc (137, 138). In this section, we will discuss how androgen deprivation, AAS, and androgen synthesis influence the neurochemistry and structure of the mesocorticolimbic system.
Most studies examining T regulation of the mesocorticolimbic system have focused on DAergic transmission in the NAc and mPFC. GDX alters DA tone in the mPFC of male and female rats (139). In the mPFC, GDX decreases basal DA after 4 days but increases it after 28 days. This is likely a result of GDX increasing bursting of VTA DA neurons, altering activity of mPFC efferents to the VTA, and gradually increasing TH in the VTA (140, 141). In contrast, in the NAc, basal DA is unchanged after GDX, but the DA metabolites 3,4-dihydroxyphenylacetic acid and homovanillic acid are increased after GDX (142). This finding suggests that GDX increases DA turnover in the NAc, which might indicate faster clearance of DA from the synapse and higher rates of DA signaling at baseline. GDX also modulates evoked electrophysiological and DAergic responses in the mPFC and NAc. In superfused striatal tissue, K+-simulated DA release was higher in GDX compared to GDX + T adult male mice (143). In the same study, reserpine, a drug that depletes DA, had the opposite effect, whereby DA release was higher in GDX + T male mice. This is in line with studies demonstrating that GDX affects storage, uptake, and/or synthesis of catecholamines in mesocorticolimbic nodes (142, 144) and helps to maintain NAc DA levels when exposed to methamphetamine (145).
Androgen-mediated structural plasticity and alterations in neurotransmitter receptor densities in the mesocorticolimbic system are other potential mechanism through which these hormones may alter cognitive/behavioral functions of this system. GDX decreases and high doses of T increase dendritic spine density in limbic regions, including the amygdala and HPC, in male rats (146, 147) and male monkeys (148). In a recent study, male rats were treated chronically with high-dose T, and brains were stained by Golgi–Cox to analyze neuronal morphology in medium spiny neurons of nucleus accumbens shell (NAcS) (149). T decreased spine density throughout the dendritic tree in the NAcS. However, T treatment did not affect total spine number, dendritic length, or arborization. Similarly, in the mPFC, GDX reduces and DHT increases dendritic spine formation in male mice (150). The effect of DHT on dendritic spine formation was reduced, but not absent, in GDX testicular feminization mutant male rats (a naturally occurring mutant with severely attenuated AR binding capacity), which suggests both androgenic and non-androgenic influence on synaptic remodeling.
Androgens can influence the function of the mesocorticolimbic nodes by their local metabolism to more potent androgens (e.g., T→DHT), further metabolism to weak androgens (e.g., T→DHT→3α-androstanediol), or metabolism to estrogens (T→E2). 3α-androstanediol, for example, has weak androgenic effects but also acts as a robust and rapid neuromodulator via allosteric binding to GABAAR (66, 151). Indeed, 3α-androstanediol in the NAc facilitates CPP (a DA-dependent behavior) in rodents, likely through allosteric agonism of GABAAR in GABAergic medium spiny neurons (152). Concurrently, the aromatization of T into E2 may also influence activity in the mesocorticolimbic system. E2 decreases striatal DA transporter density, enhances DA synthesis and degradation (153, 154), and downregulates DA binding to D2R in the NAc (155). In contrast, systemic treatment with the aromatase inhibitor letrozole decreases basal DA turnover in the mPFC of male and female adult rats (156). The regulation of DA turnover in the brain may be directly related to changes in phasic DA signaling. Indeed, direct pulsatile application of E2 to striatal slices induces DA release (157) and enhances K+-mediated DA release (158). Patch clamp analysis of ion transfer across the membrane in dissociated NAc medium spiny neurons demonstrated that there is a prompt diminution of Ca++ currents in response to acute E2 (159). Taken together, these data suggest that local production of E2 in males and females modulates DA signaling and postsynaptic neural excitability in the mesocorticolimbic system.
Few studies have examined whether neurosteroids produced in the mesocorticolimbic system influence neurochemistry. Pharmacological inhibition of Cyp17a1 regulates a DA-dependent behavior [prepulse inhibition (PPI)], but the study did not examine the direct effect on DA signaling (160). Inhibition of Cyp17a1 would decrease both androgen and estrogen signaling. DHEA, a product of Cyp17a1, is present in human, but not in laboratory rat or mouse, circulation. DHEA has a wide range of neurochemical effects, but the source of DHEA is rarely determined (161). The mesocorticolimbic system is sensitive to DHEA. For example, DHEA decreases the activity of monoamine oxidase, an enzyme necessary for the degradation of monoamines, in the NAc in male rats in vivo and in vitro (162). Pharmacological inhibition of 5αR suggests that DHT influences neurochemistry, particularly DA signaling, in the mesocorticolimbic system (163–165). Overall, these data suggest neurosteroids regulate DA turnover and DA signaling in the mesocorticolimbic system, which is important for regulating executive functions.
Androgens Regulate Executive Function
Clinical and preclinical evidence suggest that hypogonadism and GDX have deleterious effects on executive functioning, which can often be ameliorated with androgen replacement. Furthermore, excessive androgen exposure (e.g., AAS) during adolescence and/or adulthood has detrimental effects on executive functioning. We also examine evidence that the brain compensates for a decrease in circulating androgens by increasing local androgen synthesis in the mesocorticolimbic system to mitigate deficits in executive functioning. These studies support the hypothesis that there is an optimal level of androgen signaling within the mesocorticolimbic system for proper executive functioning.
Low Androgen Signaling
Andrew and Rogers (166) were among the first to demonstrate that androgens affect executive function. In a foraging paradigm, young male chicks treated with T pecked grains of a familiar color and ignored unfamiliar, novel-colored grains, while vehicle-treated chicks demonstrated behavioral flexibility and pecked both grain colors without bias (166). The authors used the term “persistence” (also called “perseveration”) to describe the inability to stop using a response strategy when it is no longer relevant or advantageous. Rogers (167) then showed that antiandrogen treatment or GDX decreased persistence in adult male chickens, whereas systemic T replacement in GDX chickens reinstated persistence. Subsequent studies in adult male rodents revealed that GDX or an antiandrogen reduced persistence, supporting the initial findings in birds (168, 169). GDX also decreases male persistence during social investigation of female conspecifics, suggesting that T increases male persistence in gaining access to potential mates (170).
T increases perseveration in operant conditioning tasks that require behavioral flexibility. Using a reversal learning task, van Hest and colleagues (171) demonstrated that GDX male rats perseverated less on the previously reinforced lever, while administration of T to GDX subjects displayed the highest rates of perseveration. Additionally, GDX male rats exposed to a conditional discrimination task in a T-maze made fewer errors during the reversal phase (i.e., decrease in perseverative errors) compared to intact subjects (172). On a delayed spatial alternation test, GDX subjects made less perseverative errors than intact subjects, but only after a delay of 6 sec or more, suggesting a concurrent deficit in working memory (173).
In men, declines in executive functioning and visuospatial ability are the most commonly reported adverse cognitive effects of androgen deprivation therapy [ADT (174)]. ADTs are administered to nearly 50% of prostate cancer patients (175) and include GnRH analogs (e.g., Histrelin), AR antagonists (e.g., flutamide), and androgenic enzyme inhibitors (e.g., abiraterone, a Cyp17a1 inhibitor) [see (176) for review]. Many ADTs decrease systemic T but might not decrease local androgen synthesis equally across tissue types (e.g., GnRH analogs). If a clinical study includes only subjects on ADTs that inhibit androgen synthesis and/or signaling in the brain, which is often not the case, then the effects of ADTs on executive function might be more clear (see below).
There is contradictory evidence on the effect of ADTs on executive functioning. For example, ADT is associated with deficits in attention and cognitive control [i.e., Trail Making B task, Stroop Interference Test (177)]. Furthermore, ADT is associated with decreases in gray matter volume in the dorsolateral and frontopolar PFC (178) and decreased neural activity and connectivity in the mPFC during tasks requiring inhibitory control (179). ADT is also associated with changes in impulsivity, emotional lability, and working memory, compared to matched non-ADT subjects (180). These findings suggest that ADT disrupts PFC function, an area particularly sensitive to androgens in males (33, 39).
However, other studies have not found an association between ADT and executive function (181–183). A recent meta-analysis of the effects of ADT on a variety of cognitive functions found that only visuospatial ability was reliably affected by ADT, whereas assays of executive functioning (e.g., Trail Making Test B, Stroop Interference Task) did not detect any significant differences (184). These findings are in line with a study in menopausal women with low T who were then treated with T and did not show changes in a range of executive functions compared to untreated subjects (185). However, the assessment tools for these studies might not be sensitive enough to detect small, yet important, changes in executive functions [e.g., (186)]. In addition, studies on ADT and executive function may lack statistical power, neglect confounding variables (183, 187), or include subjects who have not received ADT for enough time [ADT is usually administered for 2–3 years (181)]. These issues reduce the ability to detect effects of ADT on executive functioning. For example, Alibhai and colleagues (181) conducted a small study that regularly administered a battery of neuropsychological tests to ADT patients for 36 months, and ADT was not associated with deficits in cognitive flexibility or working memory. However, this study might not have utilized cognitive tests sensitive enough to detect PFC-specific deficits in executive functioning [e.g., Iowa Gambling Task (IGT)]. Moreover, 95% of ADT subjects in this study were using GnRH analogs as their sole ADT, which may not affect androgen synthesis in the brain, as would androgenic enzyme inhibitors.
High Androgen Signaling
Supraphysiological levels of androgens typically seen with AAS use also impair executive function. This has been explored in rats treated with supraphysiological levels of T and trained to work for food reward (sugar pellets) in an operant chamber (Figures 7 and 8). T-treated rats display deficits in different forms of cognitive flexibility, including reversal learning and extra-dimensional set-shifting (188). These rats take longer to shift their behavior when stimuli associated with rewards are reversed, or when rats must employ a novel discrimination strategy to obtain rewards. Importantly, set-shifting behavior is dependent upon D1R in NAc (12), and AAS reduce NAc D1R (189).
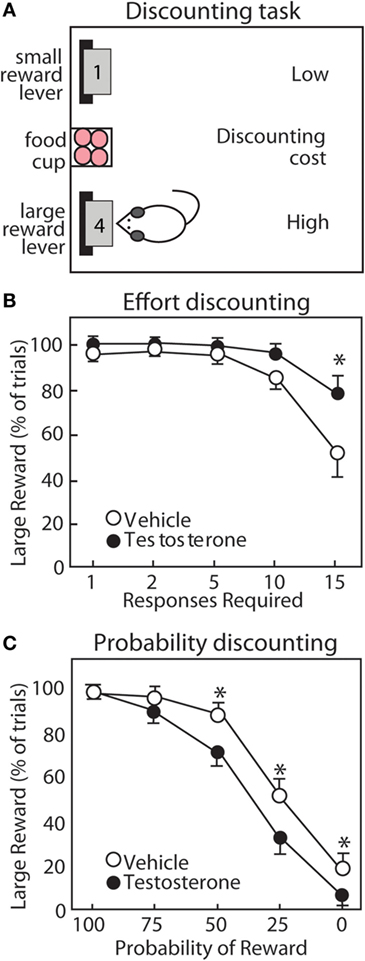
Figure 7. Testosterone influences discounting behavior of male rats. (A) Operant task for discounting behavior. Rats choose between two levers. The small reward lever delivers 1 pellet with minimal cost. The large reward lever delivers four pellets with increasing cost throughout the session. (B) For effort discounting behavior, the response requirement (number of lever presses) increases. (C) For probability discounting, the large reward is delivered with decreasing probability. Compared with vehicle controls (open circles), testosterone (closed circles) increases preference for the large reward lever in effort discounting, but reduces preference for the large reward lever in probability discounting. Adapted with permission from Ref. (190). Values presented as mean ± SEM. *p ≤ 0.05.
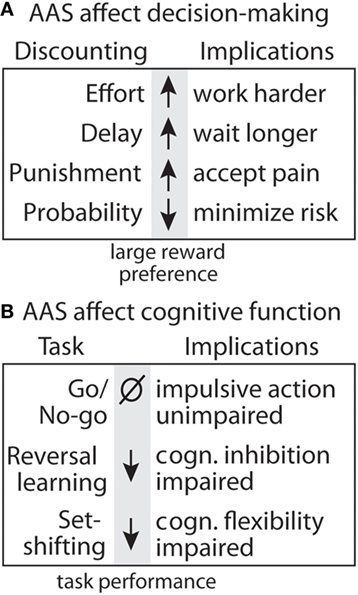
Figure 8. Summary of effects of anabolic-androgenic steroids (AAS) on decision making and cognitive function. (A) Testosterone effects on discounting behavior for effort (190), delay (191), punishment (192), and uncertainty (190). (B) Testosterone effects on cognitive function and motor impulsivity in the go/no-go task (192), cognitive inhibition in the reversal learning task, and cognitive flexibility in the set-shifting task (188).
Anabolic-androgenic steroids also alter different forms of cost/benefit decision making in operant discounting tasks. In these tasks, rats choose between two retractable levers, one of which is associated with a smaller, easily obtainable reward (1 pellet) and the other with a larger reward (3 or 4 pellets) associated some cost. These costs can include effort, delay, punishment, and probability, which results in discounting of the larger reward (i.e., making it less desirable; Figure 8). AAS and DA have site- and task-specific effects on discounting behavior. In particular, AAS do not cause impulsivity with a consistent preference for small rewards, nor do they produce a “win-at-all-costs” strategy that always favors the large reward. Instead, there is a selective effect of AAS. Specifically, AAS-treated rats are less sensitive to effort (188), punishment (192), and delay (191), but are more sensitive to uncertainty (190). In particular, AAS may diminish sensitivity to future negative consequences, even as they render users more sensitive to unpredictable outcomes.
A wealth of studies has mapped the neurotransmitters and brain regions responsible for discounting behavior using systemic treatment with neurotransmitter receptor agonists and antagonists and selective inactivation of NAc subregions. As discussed previously, D1R and D2R each promote preference for the large reward in effort discounting (delivery of the large reward requires more lever presses) and probability discounting [delivery of the large reward is uncertain (193)]. Studies using inactivation of NAc subregions have revealed that effort discounting is regulated by the NAc core (NAcC), while probability discounting is regulated more prominently by the NAc shell [NAcS (194, 195)]. These findings align with modulation of D1R and D2R in NAc subregions by the AAS nandrolone (189) and with recent studies of effort and probability discounting in response to high-dose T (190). T reduces preference for larger reward during probability discounting (190), and AAS reduce DA receptors in NAcS (189). Conversely, T treatment increases preference for the large reward during effort discounting (190), and nandrolone increases D2R in NAcC (189). Thus, AAS might reduce sensitivity to effort during effort discounting by increasing D2R in NAcC.
In animal studies, it is interesting that AAS selectively alter elements of risk-taking and impulsivity. In probability discounting, risk is reflected by the potential for reward omission, and T makes rats more risk-averse, an effect that may be driven by a reduction in NAc D1R. At the same time, they are less risk-averse in punishment discounting, whereby they risk a footshock with delivery of the large reward (190). Together, these results reveal a nuanced effect of supplemental T to increase sensitivity to reward omission and simultaneously decrease responsiveness to punishment. A similar picture emerges in assessment of how T alters different aspects of impulsivity. T has no effect on impulsive actions as measured in a go/no-go task (192), wherein rats must switch between initiating and inhibiting a response to obtain rewards. However, the same study showed that T reduces impulsive choice, assessed with a delay discounting task, in that it increases the subjects’ willingness to wait for a large, delayed reward. Given the complex manner in which increasing androgen activity can influence various forms of decision making, it is unlikely that the effects of these treatments are driven by uniform increases or decreases in mesolimbic DA activity. Rather, these findings suggest that the manner in which T influences the behavioral functions depends in part on the specific costs that are being evaluated and the underlying corticostriatal circuitry that is recruited in guiding these decisions.
Studies of executive function in human AAS users are limited and restricted to male subjects. AAS abusers show impaired visuospatial working memory compared to non-users, similar to deficits seen in ADT (184), and the level of impairment is correlated with lifetime AAS use (196). A variety of evidence further implicates androgens and AAS in risk-taking behavior in humans. In a study of American high school students, AAS use was associated with risky sex, drinking and driving, carrying a weapon, and not wearing a helmet or seat belt (106). Psychological evaluations of human users have also implicated AAS in impaired decision making, which may stem from feelings of invincibility (197). Deaths among AAS users show high rates of homicide, suicide, and drug overdose (110). These possible effects of AAS abuse on risk-taking in humans might be similar to punishment discounting in rats, in that androgens increase the appetite for reward despite a risk of punishment.
Risk taking induced by AAS has a potentially dangerous social dimension as well. AAS use has been implicated in several violent murders (107–110). In surveys of current AAS users and in studies of human volunteers, increased aggression is the most consistent behavioral effect of high-dose AAS exposure in humans (103, 105). Compared with non-users, AAS users report increased sex drive (198) and increases in risky sexual behaviors [i.e., increased numbers of partners, infrequent condom usage (111)], as well as unprotected anal intercourse among HIV-positive gay men (112). Among American high school students, AAS use correlated with not using a condom and a history of sexually transmitted disease (106). Thus, a key danger of AAS abuse is the likelihood that users will engage in behaviors that harm themselves and those around them.
Individual Variation in Circulating Androgen Levels and Executive Functioning
In normal, healthy individuals, circulating levels of androgens vary dramatically, allowing for correlational analyses of androgen levels and executive function. Interestingly, perseveration and risky behavior are positively correlated with endogenous androgens in adolescence and adulthood, similar to findings from animal studies (199). For example, adolescent males that exhibit external signs of high T (e.g., hirsutism) perform better on simple repetitive tasks than those without such external signs, independent of cognitive ability (200). This early study suggested a positive correlation between endogenous androgens and persistence. Furthermore, higher androgen levels in pubertal boys correlate with a greater probability of lifetime ethanol use (201). Therefore, circulating androgens may enhance ethanol effects on behavior, potentially increasing risk-taking in one or both sexes. To address this possibility, a study compared GDX male and female rats with and without hormone replacement in the probability discounting task, to investigate the influence of ethanol and gonadal steroids on the response to uncertainty (202). At baseline, GDX + T males showed a greater preference for the large reward than GDX males. Ethanol further increased large reward preference, but only in males. These results suggest that both ethanol and T at normal physiological levels increase tolerance for a large uncertain reward.
In adults, men with higher T are more likely to choose cards from decks offering large monetary gains paired with larger, infrequent losses in the IGT, a probabilistic, risk-based decision making task (203). This result is similar to patients with damage to the OFC and ventromedial PFC (204, 205). As a result, men with higher T earned less money throughout the session, relative to men with lower T. High levels of endogenous T also correlate with economic risk-taking outside of the lab. In a study of London stock traders, morning T levels predicted risk-taking throughout the day (206). In young and menopausal women, T is not associated with changes in any measure of executive function (207–209). Taken together, such studies suggest that individual variation in systemic T levels in males, but not females, is correlated with specific aspects of executive function.
Neuroandrogens and Executive Function
In addition to acting as endocrine signals, androgens also act as intracrine, paracrine, and autocrine signals. Specific nodes within the mesocorticolimbic system might require a particular androgen concentration to function appropriately. Similarly, McEwen and Wingfield (210) posited that local glucocorticoid signaling is tightly regulated to alleviate allostatic load imposed by high circulating glucocorticoid levels. Studies utilizing extreme changes to circulating androgen concentrations, such as AAS, GDX, or ADT demonstrate the importance of systemic androgens for executive function, but they can not reveal the role of local androgen synthesis. As discussed above, local levels of T in the mesocorticolimbic system vary greatly from circulating levels and from one neural node to another. Levels of T are often two or more times higher in the mesocorticolimbic system than in the blood in intact animals, and T is still present in the mesocorticolimbic system at 6 weeks after GDX (36). These results suggest that local T synthesis is important for neural activity in the mesocorticolimbic system and executive functioning.
There are few data on how the local production of androgens in the mesocorticolimbic system influences executive functioning. Several studies report changes in T precursors or androgenic enzymes in the mesocorticolimbic system of patients with mood disorders or in animal models of depression (211–213). Depression is frequently marked by deficits in executive functions (214, 215). In fact, clinicians refer to a disorder that occurs in geriatric populations as “depression-executive dysfunction syndrome” (214, 216). Low circulating DHEA and DHEA-S levels are correlated with depression in aged human populations, and DHEA has been suggested as treatment for depression (217, 218). In a rodent model of childhood depression, DHEA levels are lower in the VTA and NAc (but not amygdala or hypothalamus), suggesting mesolimbic-specific regulation of androgens (211). Expression of several steroidogenic enzymes are altered in post-mortem analyses of depressed individuals, which include a decrease of 5α-reductase type I in the PFC (213) and Cyp17a1 in the anterior cingulate cortex [ACC (212)], and an increase in hydroxysteroid sulfotransferase 2A1 (HST, Figure 1) in the ACC and StAR in the dorsolateral PFC (212). Changes in the expression of these specific steroidogenic enzymes suggest active androgen synthesis and metabolism in the VTA, NAc, and PFC.
Systemic administration of steroidogenic enzyme inhibitors that cross the blood–brain barrier hint at the role of neuroandrogens in modulating executive function. Using set-shifting and reversal learning tasks, the Soma laboratory has recently found that chronic systemic administration of abiraterone (a Cyp17a1 inhibitor) enhances behavioral flexibility in intact and gonadectomized subjects [unpublished results (219)]. Furthermore, systemic administration of letrozole, an aromatase inhibitor, increases risk-taking behavior in human males (220) and improves working memory in female rats (221). In particular, the study by Goudriaan et al. (220) administered letrozole to healthy men for 1 week and tested executive function and risk-taking before and after treatment. Importantly, this treatment was used to increase circulating T, but potentially influenced steroidogenesis in the mesocorticolimbic system. Letrozole-treated subjects demonstrated an increase in risk-taking on the Balloon Analog Risk-Taking task, but not the IGT or Game of Dice, compared to their baseline and estrogen-treated subjects. These findings highlight the importance of using a variety of sensitive neurocognitive assays to detect changes in executive function. This study, along with the studies of behavioral flexibility in male rats and working memory in female rats, suggests that androgens and local androgen synthesis, and not E2 or local androgen metabolism, have the most profound effects on executive functioning.
There have been no studies, to our knowledge, that directly (e.g., i.c.v. steroidogenic enzyme inhibitor) manipulated neural androgen synthesis and examined executive functioning. The most relevant studies examined the effects of androgenic enzyme (i.e., Cyp17a1 and 5αR) inhibitors on PPI of the acoustic startle reflex and DA signaling in the NAc (160, 163, 165). Frau and colleagues (160) administered apomorphine (a non-selective DA agonist; i.p.) to male rats to cause a deficit in PPI. The effects of apomorphine on PPI were attenuated by microinjecting (i.c.v.) the Cyp17a1 inhibitor abiraterone. Along with studies using systemic finasteride [5αR inhibitor (163, 164, 222, 223)], these results suggest that local androgen synthesis regulates DA signaling in the mesocorticolimbic system and DA-dependent behaviors. While these studies are informative, there still remains an important gap in our understanding of how neural androgen production specifically influences executive functioning.
Conclusion
Androgens influence a variety of behaviors and cognitive functions, which include executive functioning. Converging lines of evidence suggest that androgens can influence executive functioning via actions on the mesocorticolimbic system. Multiple nodes of the mesocorticolimbic system (VTA, NAc, mPFC, and OFC) contain AR and ER. Emerging evidence suggests that multiple nodes of the mesocorticolimbic system also locally synthesize androgens, estrogens, and other steroids. However, the physiological role of these neuroandrogens still remains to be determined. Reducing endogenous androgens (GDX, ADT) and administering exogenous androgens (AAS) alter the neurochemistry (e.g., DA signaling) and cytoarchitecture of the mesocorticolimbic system. In animal studies, both a reduction of endogenous androgens and pharmacological administration of exogenous androgens lead to alterations in behavioral flexibility and inhibitory control. In human studies, evidence suggests that ADT or AAS abuse can also lead to deficits in executive functioning. Future studies should investigate the roles of systemic and locally produced androgens in the mesocorticolimbic system and cognition. Taken together, such studies broaden our understanding of androgen regulation of behavior to include decision making and executive function, and also highlight neurosteroid and AAS action within the mesocorticolimbic system.
Author Contributions
All authors listed have made a substantial, direct and intellectual contribution to the work and approved it for publication.
Conflict of Interest Statement
The authors declare that the research was conducted in the absence of any commercial or financial relationships that could be construed as a potential conflict of interest.
Acknowledgments
The authors thank Ryan Tomm, Jordan Hamden, and Katelyn Low for comments on the manuscript.
Funding
This review was supported by a Bluma Tischler Postdoctoral Fellowship to DT; a grant from the National Institute on Drug Abuse of the U.S. National Institutes of Health (R01-DA029613) to RW; grants from the Natural Sciences and Engineering Research Council of Canada and the Canadian Institutes of Health Research (MOP 133579) to SF; grants from the Canadian Institutes of Health Research (MOP 133606), Canada Foundation for Innovation, and British Columbia Knowledge Development Fund to KS.
Abbreviations
17β-HSD, 17β-hydroxysteroid dehydrogenase; 3β-HSD, 3β-hydroxysteroid dehydrogenase/isomerase; 5αR, 5α-reductase; AAS, anabolic androgenic steroids; ACC, anterior cingulate cortex; ADT, androgen deprivation therapy; AR, androgen receptor; CPP, conditioned place preference; D1R, dopamine receptor D1; D2R, dopamine receptor D2; DA, dopamine; DAT, dopamine transporter; DHEA, dehydroepiandrosterone; DHEA-S, dehydroepiandrosterone sulfate; DHT, 5α-dihydrotestosterone; E2, 17β-estradiol; ER, estrogen receptor; ERα, estrogen receptor α; ERβ, estrogen receptor β; GABA, γ-aminobutyric acid; GABAAR, Type A γ-aminobutyric acid receptor; GDX, gonadectomy; GPER1, G protein–coupled estrogen receptor 1; GPRC6A, G protein–coupled receptor family C group 6 member A; HST, hydroxysteroid sulfotransferase 2A1; i.c.v., intracerebroventricular; i.v., intravenous; IGT, Iowa Gambling Task; ir, immunoreactivity; mAR, membrane-associated androgen receptor; mER, membrane-associated estrogen receptor; mPFC, medial prefrontal cortex; mPFC-PL, prelimbic medial prefrontal cortex; NAc, nucleus accumbens; NAcC, nucleus accumbens core; NAcS, nucleus accumbens shell; NMDA-R, N-methyl-D-aspartate receptor; OFC, orbitofrontal cortex; PCR, polymerase chain reaction; PFC, prefrontal cortex; POA/HYP, preoptic area/hypothalamus; PPI, prepulse inhibition; qPCR, quantitative polymerase chain reaction; StAR, steroidogenic acute regulatory protein; T, testosterone; TH, tyrosine hydroxylase; TSA, tyramide signal amplification; VTA, ventral tegmental area; ZIP9, Zrt- and Irt-like protein 9.
References
2. Burger HG. Androgen production in women. Fertil Steril (2002) 77:S3–5. doi:10.1016/S0015-0282(02)02985-0
4. Chen F, Knecht K, Birzin E, Fisher J, Wilkinson H, Mojena M, et al. Direct agonist/antagonist functions of dehydroepiandrosterone. Endocrinology (2005) 146:4568–76. doi:10.1210/en.2005-0368
5. Fang H, Tong W, Branham WS, Moland CL, Dial SL, Hong H, et al. Study of 202 natural, synthetic, and environmental chemicals for binding to the androgen receptor. Chem Res Toxicol (2003) 16:1338–58. doi:10.1021/tx030011g
6. Mo Q, Lu S, Simon NG. Dehydroepiandrosterone and its metabolites: differential effects on androgen receptor trafficking and transcriptional activity. J Steroid Biochem Mol Biol (2006) 99:50–8. doi:10.1016/j.jsbmb.2005.11.011
7. Hull EM, Dominguez JM. Sexual behavior in male rodents. Horm Behav (2007) 52:45–55. doi:10.1016/j.yhbeh.2007.03.030
8. Soma KK, Scotti M-AL, Newman AEM, Charlier TD, Demas GE. Novel mechanisms for neuroendocrine regulation of aggression. Front Neuroendocrinol (2008) 29:476–89. doi:10.1016/j.yfrne.2007.12.003
10. Brozoski T, Brown R, Rosvold H, Goldman P. Cognitive deficit caused by regional depletion of dopamine in prefrontal cortex of rhesus monkey. Science (1979) 205:929–32. doi:10.1126/science.112679
11. Floresco SB. Prefrontal dopamine and behavioral flexibility: shifting from an “inverted-U” toward a family of functions. Front Neurosci (2013) 7:62. doi:10.3389/fnins.2013.00062
12. Haluk DM, Floresco SB. Ventral striatal dopamine modulation of different forms of behavioral flexibility. Neuropsychopharmacology (2009) 34:2041–52. doi:10.1038/npp.2009.21
13. Ragozzino ME. The effects of dopamine D1 receptor blockade in the prelimbic – infralimbic areas on behavioral flexibility. Learn Mem (2002) 9:18–28. doi:10.1101/lm.45802
14. Floresco SB, Magyar O, Ghods-Sharifi S, Vexelman C, Tse MTL. Multiple dopamine receptor subtypes in the medial prefrontal cortex of the rat regulate set-shifting. Neuropsychopharmacology (2006) 31:297–309. doi:10.1038/sj.npp.1300825
15. Dias R, Robbins TW, Roberts AC. Dissociable forms of inhibitory control within prefrontal cortex with an analog of the Wisconsin Card Sort Test: restriction to novel situations and independence from “on-line” processing. J Neurosci (1997) 17:9285–97. doi:10.1523/JNEUROSCI.17-23-09285.1997
16. McAlonan K, Brown VJ. Orbital prefrontal cortex mediates reversal learning and not attentional set shifting in the rat. Behav Brain Res (2003) 146:97–103. doi:10.1016/j.bbr.2003.09.019
17. Roberts AC, De Salvia MA, Wilkinson LS, Collins P, Muir JL, Everitt BJ, et al. 6-Hydroxydopamine lesions of the prefrontal cortex in monkeys enhance performance on an analog of the Wisconsin Card Sort Test: possible interactions with subcortical dopamine. J Neurosci (1994) 14:2531–44. doi:10.1523/JNEUROSCI.14-05-02531.1994
18. O’Neill M, Brown VJ. The effect of striatal dopamine depletion and the adenosine A2A antagonist KW-6002 on reversal learning in rats. Neurobiol Learn Mem (2007) 88:75–81. doi:10.1016/j.nlm.2007.03.003
19. Clarke HF, Hill GJ, Robbins TW, Roberts AC. Dopamine, but not serotonin, regulates reversal learning in the marmoset caudate nucleus. J Neurosci (2011) 31:4290–7. doi:10.1523/JNEUROSCI.5066-10.2011
20. Floresco SB, Tse MTL, Ghods-Sharifi S. Dopaminergic and glutamatergic regulation of effort- and delay-based decision making. Neuropsychopharmacology (2008) 33:1966–79. doi:10.1038/sj.npp.1301565
21. St. Onge JR, Floresco SB. Dopaminergic modulation of risk-based decision making. Neuropsychopharmacology (2009) 34:681–97. doi:10.1038/npp.2008.121
22. St. Onge JR, Chiu YC, Floresco SB. Differential effects of dopaminergic manipulations on risky choice. Psychopharmacology (Berl) (2010) 211:209–21. doi:10.1007/s00213-010-1883-y
23. Stopper CM, Khayambashi S, Floresco SB. Receptor-specific modulation of risk-based decision making by nucleus accumbens dopamine. Neuropsychopharmacology (2013) 38:715–28. doi:10.1038/npp.2012.240
24. Nowend KL, Arizzi M, Carlson BB, Salamone JD. D1 or D2 antagonism in nucleus accumbens core or dorsomedial shell suppresses lever pressing for food but leads to compensatory increases in chow consumption. Pharmacol Biochem Behav (2001) 69:373–82. doi:10.1016/S0091-3057(01)00524-X
25. Schweimer J, Hauber W. Dopamine D1 receptors in the anterior cingulate cortex regulate effort-based decision making. Learn Mem (2006) 13:777–82. doi:10.1101/lm.409306
26. Loos M, Pattij T, Janssen MCW, Counotte DS, Schoffelmeer ANM, Smit AB, et al. Dopamine receptor D1/D5 gene expression in the medial prefrontal cortex predicts impulsive choice in rats. Cereb Cortex (2010) 20:1064–70. doi:10.1093/cercor/bhp167
27. St Onge JR, Abhari H, Floresco SB. Dissociable contributions by prefrontal D1 and D2 receptors to risk-based decision making. J Neurosci (2011) 31:8625–33. doi:10.1523/JNEUROSCI.1020-11.2011
28. Jenni NL, Larkin JD, Floresco SB. Prefrontal dopamine D1 and D2 receptors regulate dissociable aspects of decision making via distinct ventral striatal and amygdalar circuits. J Neurosci (2017) 37:6200–13. doi:10.1523/JNEUROSCI.0030-17.2017
29. Gao W, Bohl CE, Dalton JT. Chemistry and structural biology of androgen receptor. Chem Rev (2005) 105:3352–70. doi:10.1021/cr020456u
30. Griggs RC, Kingston W, Jozefowicz RF, Herr BE, Forbes G, Halliday D. Effect of testosterone on muscle mass and muscle protein synthesis. J Appl Physiol (1989) 66:498–503. doi:10.1152/jappl.1989.66.1.498
31. O’Connell LA, Hofmann HA. The vertebrate mesolimbic reward system and social behavior network: a comparative synthesis. J Comp Neurol (2011) 519:3599–639. doi:10.1002/cne.22735
32. Raskin K, de Gendt K, Duittoz A, Liere P, Verhoeven G, Tronche F, et al. Conditional inactivation of androgen receptor gene in the nervous system: effects on male behavioral and neuroendocrine responses. J Neurosci (2009) 29:4461–70. doi:10.1523/JNEUROSCI.0296-09.2009
33. Low KL, Ma C, Soma KK. Tyramide signal amplification permits immunohistochemical analyses of androgen receptors in the rat prefrontal cortex. J Histochem Cytochem (2017) 65:295–308. doi:10.1369/0022155417694870
34. Simerly RB, Chang C, Muramatsu M, Swanson LW. Distribution of androgen and estrogen receptor mRNA-containing cells in the rat brain: an in situ hybridization study. J Comp Neurol (1990) 294:76–95. doi:10.1002/cne.902940107
35. Kritzer MF. The distribution of immunoreactivity for intracellular androgen receptors in the cerebral cortex of hormonally intact adult male and female rats: localization in pyramidal neurons making corticocortical connections. Cereb Cortex (2004) 14:268–80. doi:10.1093/cercor/bhg127
36. Tobiansky DJ, Korol AM, Ma C, Hamden JE, Jalabert C, Tomm RJ, et al. Testosterone and corticosterone in the mesocorticolimbic system of male rats: effects of gonadectomy and caloric restriction. Endocrinology (2018) 159:450–64. doi:10.1210/en.2017-00704
37. Fuxjager MJ, Forbes-Lorman RM, Coss DJ, Auger CJ, Auger AP, Marler CA. Winning territorial disputes selectively enhances androgen sensitivity in neural pathways related to motivation and social aggression. Proc Natl Acad Sci U S A (2010) 107:12393–8. doi:10.1073/pnas.1001394107
38. Abdelgadir SE, Roselli CE, Choate JV, Resko JA. Distribution of aromatase cytochrome P450 messenger ribonucleic acid in adult rhesus monkey brains. Biol Reprod (1997) 57:772–7. doi:10.1095/biolreprod57.4.772
39. Finley SK, Kritzer MF. Immunoreactivity for intracellular androgen receptors in identified subpopulations of neurons, astrocytes and oligodendrocytes in primate prefrontal cortex. J Neurobiol (1999) 40:446–57. doi:10.1002/(SICI)1097-4695(19990915)40:4<446::AID-NEU3>3.0.CO;2-J
40. Hawrylycz MJ, Lein ES, Guillozet-Bongaarts AL, Shen EH, Ng L, Miller JA, et al. An anatomically comprehensive atlas of the adult human brain transcriptome. Nature (2012) 489:391–9. doi:10.1038/nature11405
41. DonCarlos LL, Sarkey S, Lorenz B, Azcoitia I, Garcia-Ovejero D, Huppenbauer C, et al. Novel cellular phenotypes and subcellular sites for androgen action in the forebrain. Neuroscience (2006) 138:801–7. doi:10.1016/j.neuroscience.2005.06.020
42. Aubele T, Kritzer MF. Androgen influence on prefrontal dopamine systems in adult male rats: localization of cognate intracellular receptors in medial prefrontal projections to the ventral tegmental area and effects of gonadectomy and hormone replacement on glutamate-stimulated. Cereb Cortex (2012) 22:1799–812. doi:10.1093/cercor/bhr258
43. Creutz LM, Kritzer MF. Mesostriatal and mesolimbic projections of midbrain neurons immunoreactive for estrogen receptor beta or androgen receptors in rats. J Comp Neurol (2004) 476:348–62. doi:10.1002/cne.20229
44. Kritzer MF, Creutz LM. Region and sex differences in constituent dopamine neurons and immunoreactivity for intracellular estrogen and androgen receptors in mesocortical projections in rats. J Neurosci (2008) 28:9525–35. doi:10.1523/JNEUROSCI.2637-08.2008
45. Low KL. Effects of Age on Mesocorticolimbic Testosterone Levels and Androgen Receptors in Male Rats. Vancouver: The University of British Columbia (2016). Available from: https://open.library.ubc.ca/cIRcle/collections/ubctheses/24/items/1.0300233
46. Stanić D, Dubois S, Chua HK, Tonge B, Rinehart N, Horne MK, et al. Characterization of aromatase expression in the adult male and female mouse brain. I. Coexistence with oestrogen receptors α and β, and androgen receptors. PLoS One (2014) 9:e90451. doi:10.1371/journal.pone.0090451
47. Roselli CE, Resko JA. Sex differences in androgen-regulated expression of cytochrome P450 aromatase in the rat brain. J Steroid Biochem Mol Biol (1997) 61:365–74. doi:10.1016/S0960-0760(97)80034-9
48. Pérez SE, Chen E-Y, Mufson EJ. Distribution of estrogen receptor alpha and beta immunoreactive profiles in the postnatal rat brain. Brain Res Rev (2003) 145:117–39. doi:10.1016/S0165-3806(03)00223-2
49. Österlund M. Differential distribution and regulation of estrogen receptor-α and -β mRNA within the female rat brain. Mol Brain Res (1998) 54:175–80. doi:10.1016/S0169-328X(97)00351-3
50. DonCarlos LL, Monroy E, Morrell JI. Distribution of estrogen receptor-immunoreactive cells in the forebrain of the female guinea pig. J Comp Neurol (1991) 305:591–612. doi:10.1002/cne.903050406
51. Mitra SW, Hoskin E, Yudkovitz J, Pear L, Wilkinson HA, Hayashi S, et al. Immunolocalization of estrogen receptor beta in the mouse brain: comparison with estrogen receptor alpha. Endocrinology (2003) 144:2055–67. doi:10.1210/en.2002-221069
52. Tobiansky DJ, Roma PG, Hattori T, Will RG, Nutsch VL, Dominguez JM. The medial preoptic area modulates cocaine-induced activity in female rats. Behav Neurosci (2013) 127:293–302. doi:10.1037/a0031949
53. Tobiansky DJ, Will RG, Lominac KD, Turner JM, Hattori T, Krishnan K, et al. Estradiol in the preoptic area regulates the dopaminergic response to cocaine in the nucleus accumbens. Neuropsychopharmacology (2016) 41:1897–906. doi:10.1038/npp.2015.360
54. McHenry JA, Otis JM, Rossi MA, Robinson JE, Kosyk O, Miller NW, et al. Hormonal gain control of a medial preoptic area social reward circuit. Nat Neurosci (2017) 20:449–58. doi:10.1038/nn.4487
55. Thomas P, Converse A, Berg HA. ZIP9, a novel membrane androgen receptor and zinc transporter protein. Gen Comp Endocrinol (2018) 257:130–6. doi:10.1016/j.ygcen.2017.04.016
56. Pi M, Parrill AL, Quarles LD. GPRC6A mediates the non-genomic effects of steroids. J Biol Chem (2010) 285:39953–64. doi:10.1074/jbc.M110.158063
57. Berg AH, Rice CD, Rahman MS, Dong J, Thomas P. Identification and characterization of membrane androgen receptors in the ZIP9 zinc transporter subfamily: I. Discovery in female Atlantic croaker and evidence ZIP9 mediates testosterone-induced apoptosis of ovarian follicle cells. Endocrinology (2014) 155:4237–49. doi:10.1210/en.2014-1198
58. Luo J, Liu Z, Liu J, Eugene CY. Distribution pattern of GPRC6A mRNA in mouse tissue by in situ hybridization. Zhong Nan Da Xue Xue Bao Yi Xue Ban (2010) 35:1–10. doi:10.3969/j.issn.1672-7347.2010.01.001
59. Lein ES, Hawrylycz MJ, Ao N, Ayres M, Bensinger A, Bernard A, et al. Genome-wide atlas of gene expression in the adult mouse brain. Nature (2007) 445:168–76. doi:10.1038/nature05453
60. Garza-Contreras J, Duong P, Snyder BD, Schreihofer DA, Cunningham RL. Presence of androgen receptor variant in neuronal lipid rafts. eNeuro (2017) 4:ENEURO.109–17. doi:10.1523/ENEURO.0109-17.2017
61. Brailoiu E, Dun SL, Brailoiu GC, Mizuo K, Sklar LA, Oprea TI, et al. Distribution and characterization of estrogen receptor G protein-coupled receptor 30 in the rat central nervous system. J Endocrinol (2007) 193:311–21. doi:10.1677/JOE-07-0017
62. O’Dowd BF, Nguyen T, Marchese A, Cheng R, Lynch KR, Heng HH, et al. Discovery of three novel G-protein-coupled receptor genes. Genomics (1998) 47:310–3. doi:10.1006/geno.1998.5095
63. Almey A, Milner TA, Brake WG. Estrogen receptors in the central nervous system and their implication for dopamine-dependent cognition in females. Horm Behav (2015) 74:125–38. doi:10.1016/j.yhbeh.2015.06.010
64. Zorumski CF, Paul SM, Izumi Y, Covey DF, Mennerick S. Neurosteroids, stress and depression: potential therapeutic opportunities. Neurosci Biobehav Rev (2013) 37:109–22. doi:10.1016/j.neubiorev.2012.10.005
65. Prough RA, Clark BJ, Klinge CM. Novel mechanisms for DHEA action. J Mol Endocrinol (2016) 56:R139–55. doi:10.1530/JME-16-0013
66. Reddy DS, Estes WA. Clinical potential of neurosteroids for CNS disorders. Trends Pharmacol Sci (2016) 37:543–61. doi:10.1016/j.tips.2016.04.003
67. Corpéchot C, Robel P, Axelson M, Sjövall J, Baulieu EE. Characterization and measurement of dehydroepiandrosterone sulfate in rat brain. Proc Natl Acad Sci U S A (1981) 78:4704–7. doi:10.1073/pnas.78.8.4704
68. Corpéchot C, Synguelakis M, Talha S, Axelson M, Sjövall J, Vihko R, et al. Pregnenolone and its sulfate ester in the rat brain. Brain Res (1983) 270:119–25. doi:10.1016/0006-8993(83)90797-7
69. Liere P, Pianos A, Eychenne B, Cambourg A, Bodin K, Griffiths W, et al. Analysis of pregnenolone and dehydroepiandrosterone in rodent brain: cholesterol autoxidation is the key. J Lipid Res (2009) 50:2430–44. doi:10.1194/jlr.M900162-JLR200
70. Hojo Y, Hattori T-A, Enami T, Furukawa A, Suzuki K, Ishii H-T, et al. Adult male rat hippocampus synthesizes estradiol from pregnenolone by cytochromes P45017alpha and P450 aromatase localized in neurons. Proc Natl Acad Sci U S A (2004) 101:865–70. doi:10.1073/pnas.2630225100
71. Munetsuna E, Hojo Y, Hattori M, Ishii H, Kawato S, Ishida A, et al. Retinoic acid stimulates 17beta-estradiol and testosterone synthesis in rat hippocampal slice cultures. Endocrinology (2009) 150:4260–9. doi:10.1210/en.2008-1644
72. Remage-Healey L, Dong SM, Chao A, Schlinger BA. Sex-specific, rapid neuroestrogen fluctuations and neurophysiological actions in the songbird auditory forebrain. J Neurophysiol (2012) 107:1621–31. doi:10.1152/jn.00749.2011
73. Saldanha CJ, Remage-Healey L, Schlinger BA. Synaptocrine signaling: steroid synthesis and action at the synapse. Endocr Rev (2011) 32:532–49. doi:10.1210/er.2011-0004
74. Kristensen DM, Desdoits-Lethimonier C, Mackey AL, Dalgaard MD, De Masi F, Munkbøl CH, et al. Ibuprofen alters human testicular physiology to produce a state of compensated hypogonadism. Proc Natl Acad Sci U S A (2018) 115:E715–24. doi:10.1073/pnas.1715035115
75. Le Goascogne C, Sananes N, Gouezou M, Takemori S, Kominami S, Baulieu EE, et al. Immunoreactive cytochrome P-45017alpha in rat and guinea-pig gonads, adrenal glands and brain. J Reprod Fertl (1991) 93:609–22. doi:10.1530/jrf.0.0930609
76. Sanne JL, Krueger KE. Aberrant splicing of rat steroid 17α-hydroxylase transcripts. Gene (1995) 165:327–8. doi:10.1016/0378-1119(95)00536-F
77. Compagnone NA, Bulfone A, Rubenstein JLR, Mellon SH. Steroidogenic enzyme p450c17 is expressed in the embryonic central nervous system. Endocrinology (1995) 136:5212–23. doi:10.1210/endo.136.6.7750493
78. Strömstedt M, Waterman MR. Messenger RNAs encoding steroidogenic enzymes are expressed in rodent brain. Brain Res Mol Brain Res (1995) 34:75–88. doi:10.1016/0169-328X(95)00140-N
79. Mellon SH, Deschepper CF. Neurosteroid biosynthesis: genes for adrenal steroidogenic enzymes are expressed in the brain. Brain Res (1993) 629:283–92. doi:10.1016/0006-8993(93)91332-M
80. Guennoun R, Fiddes RJ, Gouézou M, Lombès M, Baulieu EE. A key enzyme in the biosynthesis of neurosteroids, 3β-hydroxysteroid dehydrogenase/Δ5-Δ4-isomerase (3β-HSD), is expressed in rat brain. Brain Res Mol Brain Res (1995) 30:287–300. doi:10.1016/0169-328X(95)00016-L
81. Kimoto T, Ishii H, Higo S, Hojo Y, Kawato S. Semicomprehensive analysis of the postnatal age-related changes in the mRNA expression of sex steroidogenic enzymes and sex steroid receptors in the male rat hippocampus. Endocrinology (2010) 151:5795–806. doi:10.1210/en.2010-0581
82. Hojo Y, Okamoto M, Kato A, Higo S, Sakai F, Soya H, et al. Neurosteroid synthesis in adult female rat hippocampus, including androgens and allopregnanolone. J Steroids Horm Sci (2014) S4:2. doi:10.4172/2157-7536.S4-002
83. Munetomo A, Hojo Y, Higo S, Kato A, Yoshida K, Shirasawa T, et al. Aging-induced changes in sex-steroidogenic enzymes and sex-steroid receptors in the cortex, hypothalamus and cerebellum. J Physiol Sci (2015) 65:253–63. doi:10.1007/s12576-015-0363-x
84. Furukawa A, Miyatake A, Ohnishi T, Ichikawa Y. Steroidogenic acute regulatory protein (StAR) transcripts constitutively expressed in the adult rat central nervous system: colocalization of StAR, cytochrome P-450SCC (CYP XIA1), and 3beta-hydroxysteroid dehydrogenase in the rat brain. J Neurochem (1998) 71:2231–8. doi:10.1046/j.1471-4159.1998.71062231.x
85. Stoffel-Wagner B. Neurosteroid biosynthesis in the human brain and its clinical implications. Ann N Y Acad Sci (2003) 1007:64–78. doi:10.1196/annals.1286.007
86. Stoffel-Wagner B. Neurosteroid metabolism in the human brain. Eur J Endocrinol (2001) 145:669–79. doi:10.1530/eje.0.1450669
87. Wagner CK, Morrell JI. Neuroanatomical distribution of aromatase mRNA in the rat brain: indications of regional regulation. J Steroid Biochem Mol Biol (1997) 61:307–14. doi:10.1016/S0960-0760(97)80028-3
88. Hatanaka Y, Hojo Y, Mukai H, Murakami G, Komatsuzaki Y, Kim J, et al. Rapid increase of spines by dihydrotestosterone and testosterone in hippocampal neurons: dependence on synaptic androgen receptor and kinase networks. Brain Res (2015) 1621:121–32. doi:10.1016/j.brainres.2014.12.011
89. Matsunaga M, Ukena K, Tsutsui K. Androgen biosynthesis in the quail brain. Brain Res (2002) 948:180–5. doi:10.1016/S0006-8993(02)03147-5
90. Shen P, Schlinger BA, Campagnoni AT, Arnold AP. An atlas of aromatase mRNA expression in the zebra finch brain. J Comp Neurol (1995) 360:172–84. doi:10.1002/cne.903600113
91. Raab H, Beyer C, Wozniak A, Hutchison JB, Pilgrim C, Reisert I. Ontogeny of aromatase messenger ribonucleic acid and aromatase activity in the rat midbrain. Brain Res Mol Brain Res (1995) 34:333–6. doi:10.1016/0169-328X(95)00196-Y
92. Cook JB, Werner DF, Maldonado-Devincci AM, Leonard MN, Fisher KR, O’Buckley TK, et al. Overexpression of the steroidogenic enzyme cytochrome P450 side chain cleavage in the ventral tegmental area increases 3α,5α-THP and reduces long-term operant ethanol self-administration. J Neurosci (2014) 34:5824–34. doi:10.1523/JNEUROSCI.4733-13.2014
93. Agís-Balboa RC, Pinna G, Zhubi A, Maloku E, Veldic M, Costa E, et al. Characterization of brain neurons that express enzymes mediating neurosteroid biosynthesis. Proc Natl Acad Sci U S A (2006) 103:14602–7. doi:10.1073/pnas.0606544103
94. Snipes CA, Shore LS. Metabolism of testosterone in vitro by hypothalamus and other areas of rat brain. Andrologia (1982) 14:81–5. doi:10.1111/j.1439-0272.1982.tb03101.x
95. Zwain IH, Yen SS. Neurosteroidogenesis in astrocytes, oligodendrocytes, and neurons of cerebral cortex of rat brain. Endocrinology (1999) 140:3843–52. doi:10.1210/endo.140.8.6907
96. Schindler AE. Metabolism of androstenedione and testosterone in human fetal brain. Prog Brain Res (1975) 42:330. doi:10.1016/S0079-6123(08)63689-4
97. Jenkins JS, Hall CJ. Metabolism of [14C]testosterone by human foetal and adult brain tissue. J Endocrinol (1977) 74:425–9. doi:10.1677/joe.0.0740425
98. Hojo Y, Higo S, Ishii H, Ooishi Y, Mukai H, Murakami G, et al. Comparison between hippocampus-synthesized and circulation-derived sex steroids in the hippocampus. Endocrinology (2009) 150:5106–12. doi:10.1210/en.2009-0305
99. Kicman AT. Pharmacology of anabolic steroids. Br J Pharmacol (2008) 154:502–21. doi:10.1038/bjp.2008.165
100. Pope HG, Kanayama G, Athey A, Ryan E, Hudson JI, Baggish A. The lifetime prevalence of anabolic-androgenic steroid use and dependence in Americans: current best estimates. Am J Addict (2014) 23:371–7. doi:10.1111/j.1521-0391.2013.12118.x
101. Johnston L, O’Malley P, Miech R, Bachman J, Schulenberg J. Monitoring the Future: National Survey Results on Drug Use: 1975-2014. Overview, Key Findings on Adolescent Drug Use. Ann Arbor: Institute for Social Research, The University of Michigan (2014). p. 1–98.
102. World Anti-Doping Agency. 2014 anti-doping testing figures report. Lab Rep (2014) 16:202. Available from: https://www.wada-ama.org/en/resources/laboratories/anti-doping-testing-figures
103. Wood RI, Stanton SJ. Testosterone and sport: current perspectives. Horm Behav (2012) 61:147–55. doi:10.1016/j.yhbeh.2011.09.010
105. Pope HG, Wood RI, Rogol A, Nyberg F, Bowers L, Bhasin S. Adverse health consequences of performance-enhancing drugs: an endocrine society scientific statement. Endocr Rev (2014) 35:341–75. doi:10.1210/er.2013-1058
106. Middleman AB, Faulkner AH, Woods ER, Emans SJ, DuRant RH. High-risk behaviors among high school students in Massachusetts who use anabolic steroids. Pediatrics (1995) 96:268–72.
107. Pope HG, Kouri EM, Powell KF, Campbell C, Katz DL. Anabolic-androgenic steroid use among 133 prisoners. Compr Psychiatry (1996) 37:322–7. doi:10.1016/S0010-440X(96)90013-9
108. Conacher GN, Workman DG. Violent crime possibly associated with anabolic steroid use. Am J Psychiatry (1989) 146:679. doi:10.1176/ajp.146.5.679b
109. Schulte HM, Hall MJ, Boyer M. Domestic violence associated with anabolic steroid abuse. Am J Psychiatry (1993) 150:348. doi:10.1176/ajp.150.2.348a
110. Thiblin I, Lindquist O, Rajs J. Cause and manner of death among users of anabolic androgenic steroids. J Forensic Sci (2000) 45:16–23. doi:10.1520/JFS14635J
111. Midgley SJ, Heather N, Best D, Henderson D, McCarthy S, Davies JB. Risk behaviours for HIV and hepatitis infection among anabolic-androgenic steroid users. AIDS Care (2000) 12:163–70. doi:10.1080/09540120050001832
112. Bolding G, Sherr L, Elford J. Use of anabolic steroids and associated health risks among gay men attending London gyms. Addiction (2002) 97:195–203. doi:10.1046/j.1360-0443.2002.00031.x
113. Sherman AM, de Vries B, Lansford JE. Friendship in childhood and adulthood: lessons across the life span. Int J Aging Hum Dev (2000) 51:31–51. doi:10.2190/4QFV-D52D-TPYP-RLM6
114. Sumter SR, Bokhorst CL, Steinberg L, Westenberg PM. The developmental pattern of resistance to peer influence in adolescence: will the teenager ever be able to resist? J Adolesc (2009) 32:1009–21. doi:10.1016/j.adolescence.2008.08.010
115. Sato SM, Schulz KM, Sisk CL, Wood RI. Adolescents and androgens, receptors and rewards. Horm Behav (2008) 53:647–58. doi:10.1016/j.yhbeh.2008.01.010
116. Kuntsche E, Gmel G. Alcohol consumption in late adolescence and early adulthood – where is the problem? Swiss Med Wkly (2013) 143:w13826. doi:10.4414/smw.2013.13826
117. Martin CA, Mainous AG, Curry T, Martin D. Alcohol use in adolescent females: correlates with estradiol and testosterone. Am J Addict (1999) 8:9–14. doi:10.1080/105504999306036
118. Spear LP. Consequences of adolescent use of alcohol and other drugs: studies using rodent models. Neurosci Biobehav Rev (2016) 70:228–43. doi:10.1016/j.neubiorev.2016.07.026
119. Johnson LR, Wood RI. Oral testosterone self-administration in male hamsters. Neuroendocrinology (2001) 73:285–92. doi:10.1159/000054645
120. Wood RI. Reinforcing aspects of androgens. Physiol Behav (2004) 83:279–89. doi:10.1016/j.physbeh.2004.08.012
121. Peters KD, Wood RI. Androgen dependence in hamsters: overdose, tolerance, and potential opioidergic mechanisms. Neuroscience (2005) 130:971–81. doi:10.1016/j.neuroscience.2004.09.063
122. Sato SM, Johansen JA, Jordan CL, Wood RI. Membrane androgen receptors may mediate androgen reinforcement. Psychoneuroendocrinology (2010) 35:1063–73. doi:10.1016/j.psyneuen.2010.01.007
123. Brower KJ, Blow FC, Young JP, Hill EM. Symptoms and correlates of anabolic-androgenic steroid dependence. Br J Addict (1991) 86:759–68. doi:10.1111/j.1360-0443.1991.tb03101.x
124. Volkow ND, Morales M. The brain on drugs: from reward to addiction. Cell (2015) 162:712–25. doi:10.1016/j.cell.2015.07.046
125. Packard MG, Cornell AH, Alexander GM. Rewarding affective properties of intra-nucleus accumbens injections of testosterone. Behav Neurosci (1997) 111:219–24. doi:10.1037/0735-7044.111.1.219
126. Frye CA, Rhodes ME, Rosellini R, Svare B. The nucleus accumbens as a site of action for rewarding properties of testosterone and its 5alpha-reduced metabolites. Pharmacol Biochem Behav (2002) 74:119–27. doi:10.1016/S0091-3057(02)00968-1
127. White NM, Packard MG, Hiroi N. Place conditioning with dopamine D1 and D2 agonists injected peripherally or into nucleus accumbens. Psychopharmacology (Berl) (1991) 103:271–6. doi:10.1007/BF02244216
128. Packard MG, Schroeder JP, Alexander GM. Expression of testosterone conditioned place preference is blocked by peripheral or intra-accumbens injection of α-flupenthixol. Horm Behav (1998) 34:39–47. doi:10.1006/hbeh.1998.1461
129. Schroeder JP, Packard MG. Role of dopamine receptor subtypes in the acquisition of a testosterone conditioned place preference in rats. Neurosci Lett (2000) 282:17–20. doi:10.1016/S0304-3940(00)00839-9
130. Triemstra JL, Sato SM, Wood RI. Testosterone and nucleus accumbens dopamine in the male Syrian hamster. Psychoneuroendocrinology (2008) 33:386–94. doi:10.1016/j.psyneuen.2007.12.006
131. Kailanto S, Kankaanpää A, Seppälä T. Subchronic steroid administration induces long lasting changes in neurochemical and behavioral response to cocaine in rats. Steroids (2011) 76:1310–6. doi:10.1016/j.steroids.2011.06.011
132. Kurling S, Kankaanpää A, Seppälä T. Sub-chronic nandrolone treatment modifies neurochemical and behavioral effects of amphetamine and 3,4-methylenedioxymethamphetamine (MDMA) in rats. Behav Brain Res (2008) 189:191–201. doi:10.1016/j.bbr.2007.12.021
133. Dimeo AN, Wood RI. Self-administration of estrogen and dihydrotestosterone in male hamsters. Horm Behav (2006) 49:519–26. doi:10.1016/j.yhbeh.2005.11.003
134. Henderson LP, Penatti CAA, Jones BL, Yang P, Clark AS. Anabolic androgenic steroids and forebrain GABAergic transmission. Neuroscience (2006) 138:793–9. doi:10.1016/j.neuroscience.2005.08.039
135. McIntyre KL, Porter DM, Henderson LP. Anabolic androgenic steroids induce age-, sex-, and dose-dependent changes in receptor subunit mRNAs in the mouse forebrain. Neuropharmacology (2002) 43:634–45. doi:10.1016/S0028-3908(02)00154-5
136. Beach FA, Pauker RS. Effects of castration and subsequent androgen administration upon mating behavior in the male hamster (Cricetus auratus). Endocrinology (1949) 45:211–21. doi:10.1210/endo-45-3-211
137. Pfaus JG, Damsma G, Nomikos GG, Wenkstern DG, Blaha CD, Phillips AG, et al. Sexual behavior enhances central dopamine transmission in the male rat. Brain Res (1990) 530:345–8. doi:10.1016/0006-8993(90)91309-5
138. van Erp AM, Miczek KA. Aggressive behavior, increased accumbal dopamine, and decreased cortical serotonin in rats. J Neurosci (2000) 20:9320–5. doi:10.1523/JNEUROSCI.20-24-09320.2000
139. Aubele T, Kritzer MF. Gonadectomy and hormone replacement affects in vivo basal extracellular dopamine levels in the prefrontal cortex but not motor cortex of adult male rats. Cereb Cortex (2011) 21:222–32. doi:10.1093/cercor/bhq083
140. Adler A, Vescovo P, Robinson JK, Kritzer MF. Gonadectomy in adult life increases tyrosine hydroxylase immunoreactivity in the prefrontal cortex and decreases open field activity in male rats. Neuroscience (1999) 89:939–54. doi:10.1016/S0306-4522(98)00341-8
141. Locklear MN, Michealos M, Collins WF, Kritzer MF. Gonadectomy but not biological sex affects burst-firing in dopamine neurons of the ventral tegmental area and in prefrontal cortical neurons projecting to the ventral tegmentum in adult rats. Eur J Neurosci (2016) 45:1–15. doi:10.1111/ejn.13380
142. Bitar MS, Ota M, Linnoila M, Shapiro BH. Modification of gonadectomy-induced increases in brain monoamine metabolism by steroid hormones in male and female rats. Psychoneuroendocrinology (1991) 16:547–57. doi:10.1016/0306-4530(91)90038-U
143. Shemisa K, Kunnathur V, Liu B, Salvaterra TJ, Dluzen DE. Testosterone modulation of striatal dopamine output in orchidectomized mice. Synapse (2006) 60:347–53. doi:10.1002/syn.20309
144. Meyers B, Kritzer MF. In vitro binding assays using 3H nisoxetine and 3H WIN 35,428 reveal selective effects of gonadectomy and hormone replacement in adult male rats on norepinephrine but not dopamine transporter sites in the cerebral cortex. Neuroscience (2009) 159:271–82. doi:10.1016/j.neuroscience.2008.12.010
145. Lewis C, Dluzen DE. Testosterone enhances dopamine depletion by methamphetamine in male, but not female, mice. Neurosci Lett (2008) 448:130–3. doi:10.1016/j.neulet.2008.10.011
146. Leranth C, Petnehazy O, MacLusky NJ. Gonadal hormones affect spine synaptic density in the CA1 hippocampal subfield of male rats. J Neurosci (2003) 23:1588–92. doi:10.1523/JNEUROSCI.23-05-01588.2003
147. de Castilhos J, Forti CD, Achaval M, Rasia-Filho AA. Dendritic spine density of posterodorsal medial amygdala neurons can be affected by gonadectomy and sex steroid manipulations in adult rats: a Golgi study. Brain Res (2008) 1240:73–81. doi:10.1016/j.brainres.2008.09.002
148. Hajszan T, MacLusky NJ, Leranth C. Role of androgens and the androgen receptor in remodeling of spine synapses in limbic brain areas. Horm Behav (2008) 53:638–46. doi:10.1016/j.yhbeh.2007.12.007
149. Wallin-Miller K, Li G, Kelishani D, Wood RI. Anabolic-androgenic steroids decrease dendritic spine density in the nucleus accumbens of male rats. Neuroscience (2016) 330:72–8. doi:10.1016/j.neuroscience.2016.05.045
150. Hajszan T, MacLusky NJ, Johansen JA, Jordan CL, Leranth C. Effects of androgens and estradiol on spine synapse formation in the prefrontal cortex of normal and testicular feminization mutant male rats. Endocrinology (2007) 148:1963–7. doi:10.1210/en.2006-1626
151. Reddy DS, Jian K. The testosterone-derived neurosteroid androstanediol is a positive allosteric modulator of GABAA receptors. J Pharmacol Exp Ther (2010) 334:1031–41. doi:10.1124/jpet.110.169854
152. Frye CA. Some rewarding effects of androgens may be mediated by actions of its 5alpha-reduced metabolite 3alpha-androstanediol. Pharmacol Biochem Behav (2007) 86:354–67. doi:10.1016/j.pbb.2006.10.003
153. McArthur S, McHale E, Gillies GE. The size and distribution of midbrain dopaminergic populations are permanently altered by perinatal glucocorticoid exposure in a sex- region- and time-specific manner. Neuropsychopharmacology (2007) 32:1462–76. doi:10.1038/sj.npp.1301277
154. Pasqualini C, Olivier V, Guibert B, Frain O, Leviel V. Acute stimulatory effect of estradiol on striatal dopamine synthesis. J Neurochem (2002) 65:1651–7. doi:10.1046/j.1471-4159.1995.65041651.x
155. Bazzett TJ, Becker JB. Sex differences in the rapid and acute effects of estrogen on striatal D2 dopamine receptor binding. Brain Res (1994) 637:163–72. doi:10.1016/0006-8993(94)91229-7
156. Kokras N, Pastromas N, Papasava D, de Bournonville C, Cornil CA, Dalla C. Sex differences in behavioral and neurochemical effects of gonadectomy and aromatase inhibition in rats. Psychoneuroendocrinology (2018) 87:93–107. doi:10.1016/j.psyneuen.2017.10.007
157. Becker JB. Estrogen rapidly potentiates amphetamine-induced striatal dopamine release and rotational behavior during microdialysis. Neurosci Lett (1990) 118:169–71. doi:10.1016/0304-3940(90)90618-J
158. Thompson TL, Moss RL. Estrogen regulation of dopamine release in the nucleus accumbens: genomic- and nongenomic-mediated effects. J Neurochem (1994) 62:1750–6. doi:10.1046/j.1471-4159.1994.62051750.x
159. Mermelstein PG, Becker JB. Increased extracellular dopamine in the nucleus accumbens and striatum of the female rat during paced copulatory behavior. Behav Neurosci (1995) 109:354–65. doi:10.1037/0735-7044.109.2.354
160. Frau R, Bini V, Pes R, Pillolla G, Saba P, Devoto P, et al. Inhibition of 17α-hydroxylase/C17,20 lyase reduces gating deficits consequent to dopaminergic activation. Psychoneuroendocrinology (2014) 39:204–13. doi:10.1016/j.psyneuen.2013.09.014
161. Kibaly C, Patte-Mensah C, Mensah-Nyagan AG. Molecular and neurochemical evidence for the biosynthesis of dehydroepiandrosterone in the adult rat spinal cord. J Neurochem (2005) 93:1220–30. doi:10.1111/j.1471-4159.2005.03113.x
162. Pérez-Neri I, Montes S, Ríos C. Inhibitory effect of dehydroepiandrosterone on brain monoamine oxidase activity: in vivo and in vitro studies. Life Sci (2009) 85:652–6. doi:10.1016/j.lfs.2009.09.008
163. Devoto P, Frau R, Bini V, Pillolla G, Saba P, Flore G, et al. Inhibition of 5α-reductase in the nucleus accumbens counters sensorimotor gating deficits induced by dopaminergic activation. Psychoneuroendocrinology (2012) 37:1630–45. doi:10.1016/j.psyneuen.2011.09.018
164. Soggiu A, Piras C, Greco V, Devoto P, Urbani A, Calzetta L, et al. Exploring the neural mechanisms of finasteride: a proteomic analysis in the nucleus accumbens. Psychoneuroendocrinology (2016) 74:387–96. doi:10.1016/j.psyneuen.2016.10.001
165. Frau R, Abbiati F, Bini V, Casti A, Caruso D, Devoto P, et al. Targeting neurosteroid synthesis as a therapy for schizophrenia-related alterations induced by early psychosocial stress. Schizophr Res (2015) 168:640–8. doi:10.1016/j.schres.2015.04.044
166. Andrew RJ, Rogers LJ. Testosterone, search behaviour and persistence. Nature (1972) 237:343–6. doi:10.1038/237343a0
167. Rogers LJ. Persistence and search influenced by natural levels of androgens in young and adult chickens. Physiol Behav (1974) 12:197–204. doi:10.1016/0031-9384(74)90173-5
168. Thompson WR, Wright JS. “Persistence” in rats: effects of testosterone. Physiol Psychol (1979) 7:291–4. doi:10.3758/BF03326643
169. Archer J. Testosterone and persistence in mice. Anim Behav (1977) 25:479–88. doi:10.1016/0003-3472(77)90023-9
170. Thor DH. Testosterone and persistence of social investigation in laboratory rats. J Comp Physiol Psychol (1980) 94:970–6. doi:10.1037/h0077831
171. van Hest A, van Haaren F, van de Poll NE. Perseverative responding in male and female Wistar rats: effects of gonadal hormones. Horm Behav (1989) 23:57–67. doi:10.1016/0018-506X(89)90074-3
172. Guillamón A, Valencia A, Calés J, Segovia S. Effects of early postnatal gonadal steroids on the successive conditional discrimination reversal learning in the rat. Physiol Behav (1986) 38:845–9. doi:10.1016/0031-9384(86)90052-1
173. Neese SL, Schantz SL. Testosterone impairs the acquisition of an operant delayed alternation task in male rats. Horm Behav (2012) 61:57–66. doi:10.1016/j.yhbeh.2011.10.003
174. Nelson CJ, Lee JS, Gamboa MC, Roth AJ. Cognitive effects of hormone therapy in men with prostate cancer. Cancer (2008) 113:1097–106. doi:10.1002/cncr.23658
175. Shahinian VB, Kuo Y, Freeman JL, Orihuela E, Goodwin JS. Increasing use of gonadotropin-releasing hormone agonists for the treatment of localized prostate carcinoma. Cancer (2005) 103:1615–24. doi:10.1002/cncr.20955
176. Fokidis HB, Adomat HH, Kharmate G, Hosseini-Beheshti E, Guns ES, Soma KK. Regulation of local steroidogenesis in the brain and in prostate cancer: lessons learned from interdisciplinary collaboration. Front Neuroendocrinol (2015) 36:108–29. doi:10.1016/j.yfrne.2014.08.005
177. Yang J, Zhong F, Qiu J, Cheng H, Wang K. Dissociation of event-based prospective memory and time-based prospective memory in patients with prostate cancer receiving androgen-deprivation therapy: a neuropsychological study. Eur J Cancer Care (Engl) (2015) 24:198–204. doi:10.1111/ecc.12299
178. Chao HH, Hu S, Ide JS, Uchio E, Zhang S, Rose M, et al. Effects of androgen deprivation on cerebral morphometry in prostate cancer patients – an exploratory study. PLoS One (2013) 8:e72032. doi:10.1371/journal.pone.0072032
179. Chao HH, Uchio E, Zhang S, Hu S, Bednarski SR, Luo X, et al. Effects of androgen deprivation on brain function in prostate cancer patients – a prospective observational cohort analysis. BMC Cancer (2012) 12:1. doi:10.1186/1471-2407-12-371
180. Wu LM, Tanenbaum ML, Dijkers MPJM, Amidi A, Hall SJ, Penedo FJ, et al. Cognitive and neurobehavioral symptoms in patients with non-metastatic prostate cancer treated with androgen deprivation therapy or observation: a mixed methods study. Soc Sci Med (2016) 156:80–9. doi:10.1016/j.socscimed.2016.03.016
181. Alibhai SMH, Timilshina N, Duff-Canning S, Breunis H, Tannock IF, Naglie G, et al. Effects of long-term androgen deprivation therapy on cognitive function over 36 months in men with prostate cancer. Cancer (2017) 123:237–44. doi:10.1002/cncr.30320
182. Alibhai SMH, Breunis H, Timilshina N, Marzouk S, Stewart D, Tannock I, et al. Impact of androgen-deprivation therapy on cognitive function in men with nonmetastatic prostate cancer. J Clin Oncol (2010) 28:5030–7. doi:10.1200/JCO.2010.30.8742
183. Morote J, Tabernero J, Álvarez-Ossorio JL, Ciria JP, Domínguez-Escrig JL, Vázquez F, et al. Cognitive function in patients on androgen suppression: a prospective, multicentric study. Actas Urol Esp (2018) 42(2):114–20. doi:10.1016/j.acuro.2017.04.007
184. McGinty HL, Phillips KM, Jim HSL, Cessna JM, Asvat Y, Cases MG, et al. Cognitive functioning in men receiving androgen deprivation therapy for prostate cancer: a systematic review and meta-analysis. Support Care Cancer (2014) 22:2271–80. doi:10.1007/s00520-014-2285-1
185. Huang G, Wharton W, Travison TG, Ho MH, Gleason C, Asthana S, et al. Effects of testosterone administration on cognitive function in hysterectomized women with low testosterone levels: a dose-response randomized trial. J Endocrinol Invest (2015) 38:455–61. doi:10.1007/s40618-014-0213-3
186. Donovan KA, Walker LM, Wassersug RJ, Thompson LMA, Robinson JW. Psychological effects of androgen-deprivation therapy on men with prostate cancer and their partners. Cancer (2015) 121:4286–99. doi:10.1002/cncr.29672
187. Wu LM, Amidi A, Tanenbaum ML, Winkel G, Gordon WA, Hall SJ, et al. Computerized cognitive training in prostate cancer patients on androgen deprivation therapy: a pilot study. Support Care Cancer (2018) 26(6):1917–26. doi:10.1007/s00520-017-4026-8
188. Wallin KG, Wood RI. Anabolic–androgenic steroids impair set-shifting and reversal learning in male rats. Eur Neuropsychopharmacol (2015) 25:583–90. doi:10.1016/j.euroneuro.2015.01.002
189. Kindlundh AMS, Lindblom J, Bergström L, Wikberg JES, Nyberg F. The anabolic-androgenic steroid nandrolone decanoate affects the density of dopamine receptors in the male rat brain. Eur J Neurosci (2001) 13:291–6. doi:10.1046/j.0953-816X.2000.01402.x
190. Wallin KG, Alves JM, Wood RI. Anabolic-androgenic steroids and decision making: probability and effort discounting in male rats. Psychoneuroendocrinology (2015) 57:84–92. doi:10.1016/j.psyneuen.2015.03.023
191. Wood RI, Armstrong A, Fridkin V, Shah V, Najafi A, Jakowec M. Roid rage in rats? Testosterone effects on aggressive motivation, impulsivity and tyrosine hydroxylase. Physiol Behav (2013) 11(0–111):6–12. doi:10.1016/j.physbeh.2012.12.005
192. Cooper SE, Goings SP, Kim JY, Wood RI. Testosterone enhances risk tolerance without altering motor impulsivity in male rats. Psychoneuroendocrinology (2014) 40:201–12. doi:10.1016/j.psyneuen.2013.11.017
193. Winstanley CA, Floresco SB. Deciphering decision making: variation in animal models of effort- and uncertainty-based choice reveals distinct neural circuitries underlying core cognitive processes. J Neurosci (2016) 36:12069–79. doi:10.1523/JNEUROSCI.1713-16.2016
194. Ghods-Sharifi S, Floresco SB. Differential effects on effort discounting induced by inactivations of the nucleus accumbens core or shell. Behav Neurosci (2010) 124:179–91. doi:10.1037/a0018932
195. Stopper CM, Floresco SB. Contributions of the nucleus accumbens and its subregions to different aspects of risk-based decision making. Cogn Affect Behav Neurosci (2011) 11:97–112. doi:10.3758/s13415-010-0015-9
196. Kanayama G, Kean J, Hudson JI, Pope HG. Cognitive deficits in long-term anabolic-androgenic steroid users. Drug Alcohol Depend (2013) 130:208–14. doi:10.1016/j.drugalcdep.2012.11.008
197. Pope HG, Katz DL. Homicide and near-homicide by anabolic steroid users. J Clin Psychiatry (1990) 51:28–31.
198. Moss HB, Panzak GL, Tarter RE. Sexual functioning of male anabolic steroid abusers. Arch Sex Behav (1993) 22:1–12. doi:10.1007/BF01552908
199. Welker KM, Carré JM. Individual differences in testosterone predict persistence in men. Eur J Pers (2015) 29:83–9. doi:10.1002/per.1958
200. Broverman DM, Broverman IK, Vogel W, Palmer RD, Klaiber EL. The automatization cognitive style and physical development. Child Dev (1964) 35:1343. doi:10.1111/j.1467-8624.1964.tb05272.x
201. de Water E, Braams BR, Crone EA, Peper JS. Pubertal maturation and sex steroids are related to alcohol use in adolescents. Horm Behav (2013) 63:392–7. doi:10.1016/j.yhbeh.2012.11.018
202. Wallin-Miller KG, Chesley J, Castrillon J, Wood RI. Sex differences and hormonal modulation of ethanol-enhanced risk taking in rats. Drug Alcohol Depend (2017) 174:137–44. doi:10.1016/j.drugalcdep.2017.01.023
203. Stanton SJ, Liening SH, Schultheiss OC. Testosterone is positively associated with risk taking in the Iowa Gambling Task. Horm Behav (2011) 59:252–6. doi:10.1016/j.yhbeh.2010.12.003
204. Bechara A, Tranel D, Damasio H. Characterization of the decision-making deficit of patients with ventromedial prefrontal cortex lesions. Brain (2000) 123:2189–202. doi:10.1093/brain/123.11.2189
205. Bechara A, Damasio H, Damasio AR. Emotion, decision making and the orbitofrontal cortex. Cereb Cortex (2000) 10:295–307. doi:10.1093/cercor/10.3.295
206. Coates JM, Herbert J. Endogenous steroids and financial risk taking on a London trading floor. Proc Natl Acad Sci U S A (2008) 105:6167–72. doi:10.1073/pnas.0704025105
207. Castanho TC, Moreira PS, Portugal-Nunes C, Novais A, Costa PS, Palha JA, et al. The role of sex and sex-related hormones in cognition, mood and well-being in older men and women. Biol Psychol (2014) 103:158–66. doi:10.1016/j.biopsycho.2014.08.015
208. Henderson VW, St John JA, Hodis HN, Mccleary CA, Stanczyk FZ, Karim R, et al. Cognition, mood, and physiological concentrations of sex hormones in the early and late postmenopause. Proc Natl Acad Sci U S A (2013) 110:20290–5. doi:10.1073/pnas.1312353110
209. Bove RM, Brick DJ, Healy BC, Mancuso SM, Gerweck AV, Bredella MA, et al. Metabolic and endocrine correlates of cognitive function in healthy young women. Obesity (Silver Spring) (2013) 21:1343–9. doi:10.1002/oby.20212
210. McEwen BS, Wingfield JC. The concept of allostasis in biology and biomedicine. Horm Behav (2003) 43:2–15. doi:10.1016/S0018-506X(02)00024-7
211. Malkesman O, Braw Y, Ram E, Maayan R, Weizman A, Kinor N, et al. Dehydroepiandrosterone and monoamines in the limbic system of a genetic animal model of childhood depression. Eur Neuropsychopharmacol (2008) 18:255–61. doi:10.1016/j.euroneuro.2007.06.007
212. Qi X-R, Luchetti S, Verwer RWH, Sluiter AA, Mason MRJ, Zhou J-N, et al. Alterations in the steroid biosynthetic pathways in the human prefrontal cortex in mood disorders: a postmortem study. Brain Pathol (2017) 1–12. doi:10.1111/bpa.12548
213. Agis-Balboa RC, Guidotti A, Pinna G. 5α-reductase type I expression is downregulated in the prefrontal cortex/Brodmann’s area 9 (BA9) of depressed patients. Psychopharmacology (Berl) (2014) 231:3569–80. doi:10.1007/s00213-014-3567-5
214. Alexopoulos GS. Role of executive function in late-life depression. J Clin Psychiatry (2003) 64(Suppl 1):18–23.
215. McDermott LM, Ebmeier KP. A meta-analysis of depression severity and cognitive function. J Affect Disord (2009) 119:1–8. doi:10.1016/j.jad.2009.04.022
216. Alexopoulos GS. “The depression-executive dysfunction syndrome of late life”: a specific target for D3 agonists? Am J Geriatr Psychiatry (2001) 9:22–9. doi:10.1176/appi.ajgp.9.1.22
217. Souza-Teodoro LH, de Oliveira C, Walters K, Carvalho LA. Higher serum dehydroepiandrosterone sulfate protects against the onset of depression in the elderly: findings from the English Longitudinal Study of Aging (ELSA). Psychoneuroendocrinology (2016) 64:40–6. doi:10.1016/j.psyneuen.2015.11.005
218. Veronese N, De Rui M, Bolzetta F, Zambon S, Corti MC, Baggio G, et al. Serum dehydroepiandrosterone sulfate and incident depression in the elderly: the Pro.V.A. study. Am J Geriatr Psychiatry (2015) 23:863–71. doi:10.1016/j.jagp.2014.10.009
219. Tomm RJ, Schweitzer HR, Tobiansky DJ, Kachkovski GV, Ma CQ, Adomat HH, et al. Local Androgen Synthesis in the Male Rat Brain and its Modulation of Behavioral Flexibility. Washington D.C: Society for Neuroscience Conference (2017).
220. Goudriaan AE, Lapauw B, Ruige J, Feyen E, Kaufman JM, Brand M, et al. The influence of high-normal testosterone levels on risk-taking in healthy males in a 1-week letrozole administration study. Psychoneuroendocrinology (2010) 35:1416–21. doi:10.1016/j.psyneuen.2010.04.005
221. Taylor GT, Manzella FM, Huffman J, Cabrera OH, Hoffman J. Hormones and behavior cognition in female rats after blocking conversion of androgens to estrogens. Horm Behav (2017) 90:84–9. doi:10.1016/j.yhbeh.2017.02.011
222. Li L, Kang Y-X, Ji X-M, Li Y-K, Li S-C, Zhang X-J, et al. Finasteride inhibited brain dopaminergic system and open-field behaviors in adolescent male rats. CNS Neurosci Ther (2018) 24:115–25. doi:10.1111/cns.12781
Keywords: 3β-hydroxysteroid dehydrogenase, aromatase, cognition, Cyp17a1, estradiol, neurosteroid, dehydroepiandrosterone, LC–MS/MS
Citation: Tobiansky DJ, Wallin-Miller KG, Floresco SB, Wood RI and Soma KK (2018) Androgen Regulation of the Mesocorticolimbic System and Executive Function. Front. Endocrinol. 9:279. doi: 10.3389/fendo.2018.00279
Received: 16 February 2018; Accepted: 11 May 2018;
Published: 05 June 2018
Edited by:
Takayoshi Ubuka, Monash University Malaysia, MalaysiaReviewed by:
Graziano Pinna, University of Illinois at Chicago, United StatesMichael Schumacher, Institut National de la Santé et de la Recherche Médicale (INSERM), France
Copyright: © 2018 Tobiansky, Wallin-Miller, Floresco, Wood and Soma. This is an open-access article distributed under the terms of the Creative Commons Attribution License (CC BY). The use, distribution or reproduction in other forums is permitted, provided the original author(s) and the copyright owner are credited and that the original publication in this journal is cited, in accordance with accepted academic practice. No use, distribution or reproduction is permitted which does not comply with these terms.
*Correspondence: Daniel J. Tobiansky, ZGp0b2JpYW5za3lAZ21haWwuY29t
†Present address: Kathryn G. Wallin-Miller, Department of Behavioral Neuroscience, Oregon Health & Science University, Portland, OR, United States