- 1Department of Biological Sciences, North Carolina State University, Raleigh, NC, United States
- 2W.M. Keck Center for Behavioral Biology, North Carolina State University, Raleigh, NC, United States
- 3Graduate Program in Physiology, North Carolina State University, Raleigh, NC, United States
- 4Center for Human Health and the Environment, North Carolina State University, Raleigh, NC, United States
- 5Comparative Medicine Institute, North Carolina State University, Raleigh, NC, United States
Steroid sex hormones and biological sex influence how the brain regulates motivated behavior, reward, and sensorimotor function in both normal and pathological contexts. Investigations into the underlying neural mechanisms have targeted the striatal brain regions, including the caudate–putamen, nucleus accumbens core (AcbC), and shell. These brain regions are of particular interest to neuroendocrinologists given that they express membrane-associated but not nuclear estrogen receptors, and also the well-established role of the sex steroid hormone 17β-estradiol (estradiol) in modulating striatal dopamine systems. Indeed, output neurons of the striatum, the medium spiny neurons (MSNs), exhibit estradiol sensitivity and sex differences in electrophysiological properties. Here, we review sex differences in rat MSN glutamatergic synaptic input and intrinsic excitability across striatal regions, including evidence for estradiol-mediated sexual differentiation in the nucleus AcbC. In prepubertal animals, female MSNs in the caudate–putamen exhibit a greater intrinsic excitability relative to male MSNs, but no sex differences are detected in excitatory synaptic input. Alternatively, female MSNs in the nucleus AcbC exhibit increased excitatory synaptic input relative to male MSNs, but no sex differences in intrinsic excitability were detected. Increased excitatory synaptic input onto female MSNs in the nucleus AcbC is abolished after masculinizing estradiol or testosterone exposure during the neonatal critical period. No sex differences are detected in MSNs in prepubertal nucleus accumbens shell. Thus, despite possessing the same neuron type, striatal regions exhibit heterogeneity in sex differences in MSN electrophysiological properties, which likely contribute to the sex differences observed in striatal function.
Introduction
Steroid sex hormones and biological sex are important factors influencing neuron function (1–4). Historically, research into the roles of biological sex and hormones targeted brain regions directly involved in sex-specific reproduction-related behaviors in adult animals (5, 6). These regions display large sex differences in volume, neuron cellular anatomy, and/or electrophysiological properties. Examples of these regions include the spinal nucleus of the bulbocavernosus (7), the sexually dimorphic nucleus of the preoptic area (8), and the song control nuclei in sexually dimorphic songbirds (9). Years of work have built upon these early studies to make significant advances. Notable among these is the discovery that biological sex and steroid sex hormones can modulate brain regions not directly involved with sex-specific reproductive behaviors. Although the extent of the influence on biological sex in the nervous system remains vastly underexplored, especially since few studies documented experimental animal sex (10–12), contemporary research has detected sex differences in neural substrates across the brain (13–18). The newly appreciated role of sex in modulating neural substrate also includes the striatal brain regions, comprising the caudate–putamen (also called dorsal striatum), the nucleus accumbens core (AcbC) and the nucleus accumbens shell (AcbS).
Biological sex, exogenous 17β-estradiol (estradiol), and endogenously circulating hormones via the estrous or menstrual cycle can modulate striatal-mediated cognitive, locomotor, and sensorimotor behaviors, including those related to motivation and reward (19–30). Many striatal pathologies are sensitive to estradiol and/or show sex differences in incidence and/or phenotype. These include depression, Parkinson’s disease, drug addiction, schizophrenia, tardive dyskinesia, Huntington’s disease, ADHD, and Tourette’s syndrome, among others (31–41). The majority of published research in this field probes the link between sex and estradiol-induced influences on striatal-mediated normal and pathological behaviors (20, 42–46), especially regarding critical neurotransmitter/modulator systems such as dopamine and acetylcholine (43, 47–64). This research has established that sex and estradiol can influence striatal function via action on neurotransmitter and modulator systems, especially since the striatal regions show no robust sex differences in regional volume, neuron density, or soma size (65, 66). One area that has historically received less attention is how sex and estradiol modulate the electrophysiological properties of striatal neurons, including both the output neuron of the striatum, the medium spiny neuron (MSN) (67), and striatal interneurons (68). The term MSN is synonymous with striatal projection neuron. This is unfortunate, given that to change striatal circuit output and ultimately function, estradiol and sex must in some respect influence the electrophysiology of the MSN. This mini-review will focus upon the current state of knowledge regarding sex differences in rat MSN electrophysiological properties across striatal regions, with a focus on glutamatergic inputs and intrinsic excitability.
MSNs in Adult Caudate–Putamen Show Sex-Specific and Estradiol-Induced Differences in Excitability In Vivo
This research began in the 1980s, when Vincent and colleagues discovered that estradiol exposure increased in vivo spontaneous action potential generation and dopamine sensitivity in striatal neurons in ovariectomized adult female rat caudate–putamen (69). Tansey and colleagues then identified that the striatal neurons showing increased in vivo spontaneous action potential generation in response to high estradiol levels included nigrostriatal MSNs (other MSN subtypes and striatal interneurons were not examined). This increase in spontaneous action potential generation was induced either via exogenous estradiol exposure in ovariectomized animals or endogenously during specific phases of the estrous cycle (70). MSN spontaneous action potential firing rates were elevated in females compared with males only during the phases of the estrous cycle associated with the effects of increased estradiol and progesterone (proestrus and estrus). MSNs outside of the caudate–putamen were not examined in either study. The next breakthrough in targeting MSN electrophysiology came in the mid-1990s, when Mermelstein and colleagues established that estradiol rapidly decreases L-type calcium channel currents in both prepubertal and adult female rat caudate–putamen MSNs (71). In this case, estradiol acted within seconds in a steroid- and dose-dependent method on a membrane-associated estrogen receptor. The receptor was eventually identified as membrane-associated estrogen receptor β (72). Later research encompassing both the caudate–putamen and the nucleus accumbens established the presence of membrane-associated estrogen receptors α, β, and GPER-1 in MSNs, striatal interneurons, presynaptic terminals, and glia (72–78). The presence of aromatase, the enzyme that converts testosterone into estradiol, was also confirmed (79–82). There is little to no evidence of nuclear estrogen receptors in the striatal regions in adult rodents (83–85), although an exhaustive search across development, estrus cycle stages, and relevant species has not been performed.
These studies established several foundational themes for more recent research on the influences of estradiol and sex on MSN electrophysiology. First, estradiol can act directly on MSNs to modulate electrophysiological properties in addition to indirectly acting on MSNs by manipulating neuromodulatory influences such as those encompassed by the dopaminergic and cholinergic systems. Second, MSN sensitivity to estradiol can occur in a sex-specific fashion. Third, estradiol can manipulate MSN excitability, and in particular can increase MSN excitability in adult female animals. This sex-specific increase in MSN excitability could be potentially induced by multiple cellular mechanisms, broadly grouped into two types: mechanisms inducing alterations in synaptic input onto MSNs and mechanisms inducing alterations in the intrinsic electrophysiological properties of MSN. These mechanisms are not necessarily mutually exclusive and are not necessarily active in every striatal region. Recent research has uncovered that both mechanism types can influence MSN excitability, with heterogeneity across striatal regions (Table 1).
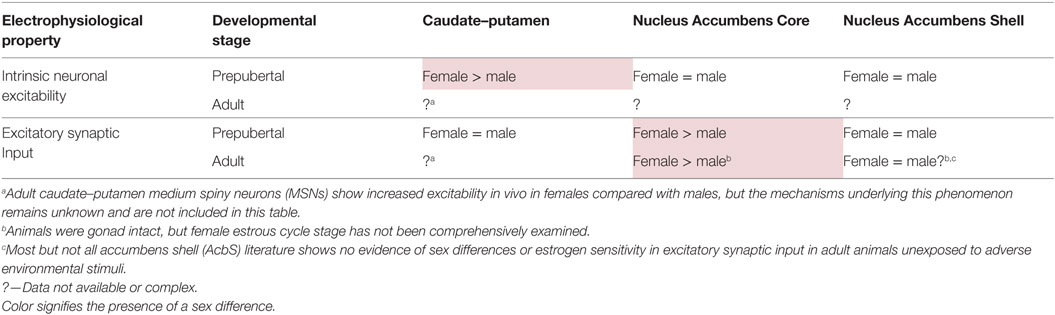
Table 1. Development of sex differences in MSN electrophysiological properties varies by striatal region.
Excitatory Synapse Number is Increased Onto Female Compared with Male AcbC MSNs, and These Synapses are Modulated by Estradiol in Adulthood
Regarding the AcbC, in the early part of this decade, Woolley and colleagues formulated the hypothesis that excitatory synapses onto MSNs in this region are increased in females compared with males (86). Anatomical studies employing electron microscopy, immunocytochemistry, and other techniques established that increased excitatory synapses are present on MSNs in the AcbC of adult rat females in proestrus compared with gonad-intact males (86–88). Alterations in excitatory synapse activity instruct AcbC function in many contexts (89–93), including the responsiveness to drugs of abuse (94). Even minor sex differences in these excitatory synaptic inputs are potentially influential on AcbC function.
One neuroanatomical correlate of increased excitatory synapse number onto female compared with male rat AcbC MSNs was concomitant increased dendritic spine density (87, 88). This sex difference in dendritic spine density was also identified in adult human AcbC, with increased dendritic spine density on female compared with male MSNs (95). In other brain regions, dendritic spine density has long been documented to be sensitive to estradiol exposure, either via endogenous exposure via the estrous or menstrual cycles, or exogenous (96–98). Regarding the AcbC, Staffend et al. elucidated that a 2-day exposure to exogenous estradiol altered dendritic spine density on MSNs in gonadectomized female hamsters (99). This exogenous estradiol-induced change in spine density is also detected in gonadectomized rat females and is dependent upon estradiol activating mGluR5 and endocannabinoid signaling via CB1 receptors (100, 101). This estrogen receptor/mGluR5 signaling pathway in the AcbC is speculated to induce an increased drive for sex (23), although this has not been tested in the context of the estrous cycle. Regarding neurological disorders, this pathway is implicated as one mechanism underlying estradiol-induced potentiation of cocaine-induced locomotor sensitization and cocaine self-administration (102, 103). This estradiol-induced change in dendritic spine density seems specific to the AcbC, at least in the absence of other interacting variables. No estradiol-induced changes in dendritic spine density were measured in the caudate–putamen, and only one of three experiments showed an estradiol-induced change in spine density in the AcbS (99, 101). There is select evidence that postsynaptic excitatory synapse markers are also increased in female AcbS (86), but this is not a robust finding (88, 104). There is evidence that sex differences in excitatory synapse in the AcbS are induced by the effects of stress and potentially other environmental factors (105). In AcbS MSNs, a stress paradigm induced sex-specific alterations in presynaptic but not postsynaptic excitatory synapse markers (106). Investigating the interactions between sex, hormones, and environmental inputs such as stress or environmental chemical exposure is an essential future line of research. Regarding male MSNs, another possibility is that testosterone regulates excitatory synaptic input onto nucleus accumbens neurons, as suggested by experiments analyzing dendritic spine density in response to week-long exogenous testosterone exposure in gonad-intact male rats (107), and the role of androgens in reward-related behaviors (108).
Increased Excitatory Synapse Activity in Female AcbC MSNs is Present Before Puberty and is Blocked by Neonatal Exposure to Estradiol
This body of data indicates that excitatory synapse number is increased onto adult female compared with male MSNs in the AcbC. Whether these differences in excitatory synapse number are functional has been assessed by analyzing miniature excitatory postsynaptic current (mEPSC) properties (Table 1). Wissman and colleagues discovered increased mEPSC frequency in adult gonad-intact female compare to male rat AcbC MSN, with estrous cycle not formally assessed (88). No differences were detected in mEPSC amplitude, decay, or in paired pulse properties, consistent with a model of increased excitatory synapse number in female compared with male AcbC, although sex differences in synaptic release probability remain possible. This increase in excitatory synaptic input could potentially be generated either in puberty or during the early natal sensitive period, and then modulated in adult females by the estrous cycle. Cao and coauthors addressed this question in three respects (109). First, increased mEPSC frequency onto female compared with male MSN in the rat AcbC was present prepuberty, determining that sex differences in excitatory synapse are generated before puberty and adulthood. Second, MSNs from females exposed to masculinizing levels of estradiol or testosterone during the neonatal sensitive period to organizational hormone action lacked increased mEPSC frequency compared with control females or males. This finding shows that neonatal masculinization/defeminization via estrogen exposure is sufficient to permanently downregulate excitatory synaptic input onto MSNs, an endocrine process which would normally occur only in male animals. This suggests an overall model that sex differences in AcbC excitatory synapse number are organized by steroid sex hormone action during early development, with estrogen exposure in males permanently decreasing AcbC synapse number and possibly adult estrogen sensitivity. Finally, Cao and colleagues found no evidence that MSN intrinsic membrane or action potential properties differed by sex, including those mediating intrinsic excitability. These findings tentatively focus the working model explaining sex differences in AcbC function toward the neuroanatomical inputs onto MSNs, most prominently glutamatergic synapse, but also dopamine inputs. Intriguing possibilities include interactions between sex, estradiol, glutamate, and dopamine. Consistent with this speculation, female compared with male AcbC MSNs demonstrate an increased proportion of large-head dendritic spines adjacent to dopaminergic terminals (87). Estradiol may also differentially modulate dopamine signaling across striatal regions, as anatomical studies revealed membrane-associated estrogen receptor α and GPER-1 expression in dopaminergic terminals in the nucleus accumbens, but not the caudate–putamen (73, 74). Dysregulation of sex differences in glutamatergic and dopaminergic signaling may potentially induce sex differences in the phenotype and incidence of AcbC-related disorders.
The anatomical source of increased excitatory input into the AcbC is unknown. One possibility of many is that the AcbC receives a sex-specific excitatory input distinct from other striatal regions. Consistent with this possibility, the AcbC receives a different set of glutamatergic inputs than other striatal regions (110, 111). These differential inputs are consistent with the AcbC mediating separate aspects of behavior than other striatal regions, including locomotor behaviors (112, 113), but also maternal, social, reward, learning, sensorimotor, and sex-related behaviors (112–121). Relatively new transgenic techniques could potentially be applied to address gaps in anatomical knowledge (122). However, inbred laboratory mice may not be effective tools to address the role of sex in modulating AcbC properties, as domestication induced female mice to lose sex- and AcbC-relevant behaviors compared with non-domesticated mice (123). In addition, many drug-abuse and other reward-mediated behaviors regulated by the nucleus accumbens are difficult or impossible to observe in mice (124). Regarding other avenues of investigation, it is unknown whether excitatory synaptic input, intrinsic excitability, and other components such as estrogen receptor α and β gene expression are modulated across estrous cycle stage. This should be a future avenue of research given the differences in striatal-mediated behaviors across the estrous and menstrual cycles. Another unknown is how GABA receptor activation and sex interact in MSNs. This question is interesting given that in select cases GABA receptor activation can facilitate MSN action potential generation (125), and that estradiol exposure can decrease extracellular GABA concentration in adult female rat caudate–putamen (126).
No Evidence for Sex Differences in Excitatory Synaptic Input Onto MSNs in the Prepubertal AcbS and Caudate–Putamen
Prepubertal sex differences in excitatory synaptic input seem specific to the AcbC. MSNs in prepubertal male and female AcbS and caudate–putamen show no evidence of sex differences in mEPSC frequency, amplitude or decay (104, 127). Although there is no evidence of sex differences in excitatory synaptic properties so far, it is always a possibility that sex or estradiol modulates unexamined aspects of glutamatergic or other neurotransmission, including but not limited to NMDA/AMPA ratios/function, synaptic plasticity, and/or silent synapse formation. At this point it is unknown whether MSNs in adult AcbS or caudate–putamen exhibit sex differences in excitatory synaptic input, although one study used anterograde but not retrograde tracing methods to provide evidence of increased projections from the orbital frontal cortex to the caudate–putamen in adult gonad-intact female compared with male rats (128). Regarding synaptic plasticity, a recent study established that pharmacological inhibition of aromatase blocked the induction of long-term potentiation of glutamatergic excitatory inputs onto MSNs in adult gonad-intact male rat caudate–putamen neurons (81). This study did not include females, but increased estradiol concentrations in adult female caudate–putamen compared with estradiol concentrations in circulating plasma levels (129), along with the presence of aromatase in caudate–putamen and nucleus accumbens (79–82) hint that aromatase may be locally active across the striatum in both sexes.
MSNs Show Increased Intrinsic Excitability in Female Compared with Male Prepubertal Caudate–Putamen but Not the AcbC or AcbS
Up to this point, this review has focused on sex differences in excitatory synaptic input. Alternatively, sex differences could occur in the intrinsic electrophysiological properties of MSNs, including alterations in action potential properties, the frequency of evoked action potentials to injected current properties, and passive (membrane) properties. Intrinsic electrophysiological properties regulate action potential generation in response to synaptic input (130), making these values integral in producing the functional output of MSNs. For example, augmented MSN intrinsic excitability would increase the number of action potentials that an MSN generates in response to a unit current. Alterations in MSN intrinsic excitability have been implicated in a number of striatal functions and disorders, including the responsivity to abused drugs, homeostatic plasticity, and striatal neuron subtypes (94, 131–136).
Female MSNs in prepubertal caudate–putamen show increased intrinsic excitability compared with male MSNs (127), unlike MSNs in the AcbC and AcbS (104, 109). This excitability is manifested in several respects. Female MSNs on average produce more action potentials than male MSNs for similar moderate amounts of injected positive current. This difference in action potential to current ratio is generated by an increased initial action potential firing rate in female compared with male MSNs, along with a decreased action potential after hyperpolarization peak and a mildly hyperpolarized action potential threshold. No sex differences were detected in passive membrane properties such as input resistance or in mEPSC properties. This lack of difference in mEPSC properties is essential for interpreting sex differences in MSN intrinsic excitability, as intrinsic excitability can be altered either independently or in concert with other attributes such as changes in excitatory synaptic input (94, 137–140). These findings support a model where increased excitability in prepubertal caudate–putamen MSNs is driven by internal changes to MSN electrical properties, rather than changes in excitatory synaptic input. Elucidating the ionic and receptor basis underlying this increased intrinsic excitation in female MSNs will be critical future experiments, along with assessments of adult MSNs, possible regional differences between dorsolateral and dorsomedial caudate–putamen, and the rostral–caudal axis. Changes in intrinsic excitability may be responsible for the increased excitability of female compared with male MSNs in vivo recorded in adult caudate–putamen (50, 69, 70). However, it is possible that intrinsic excitability and/or excitatory synaptic input in the caudate–putamen may be reorganized during puberty, such as glutamatergic synaptic input in the medial amygdala (141, 142), or striatal dopamine receptors (31, 143, 144). Either or both of these properties may be modulated by estrous cycle stage (145, 146), as suggested by in vivo recordings of caudate–putamen MSNs (69, 70). Overall, data from caudate–putamen MSNs support the theme of increased excitability in female compared with male MSNs, but indicate that the mechanism underlying these differences varies by striatal region.
Conclusion
Medium spiny neuron electrophysiological properties are sensitive to sex and steroid sex hormone action in a striatal region-specific manner (Figure 1). In prepubertal caudate–putamen, MSNs show on average increased intrinsic excitation in females compared with males. In adult caudate–putamen, MSNs likewise show increased excitability in females compared with males, but the mechanism underlying this difference remains unknown. In both prepubertal and adult AcbC, MSNs receive augmented excitatory synaptic input in females compared with males, and early-life exposure to estradiol is instrumental in the sexual differentiation of this property. In the AcbS, there is little evidence for sex differences in intrinsic excitability or excitatory synaptic input in prepubertal animals unexposed to adverse environmental stimuli. These data argue for heterogeneity across striatal regions in the sensitivity to MSNs to sex and steroid sex hormones and the relative amount and nature of masculinization and feminization in MSN electrophysiological properties differs by striatal region. These findings highlight the importance of differentiating between striatal regions, developmental stage, sex, and estrous cycle. These data extend earlier mosaic models of brain sexuality, in that not only individual brain regions but also individual neuron types, in this instance MSNs, show differential degrees of masculinization, feminization, and homogeny (147, 148). These sex differences and similarities in MSN electrophysiological properties, along with developmental, individual, subtype, and the other documented variables in MSN properties, induce a dizzying variety within the same neuron type both between and within males and females.
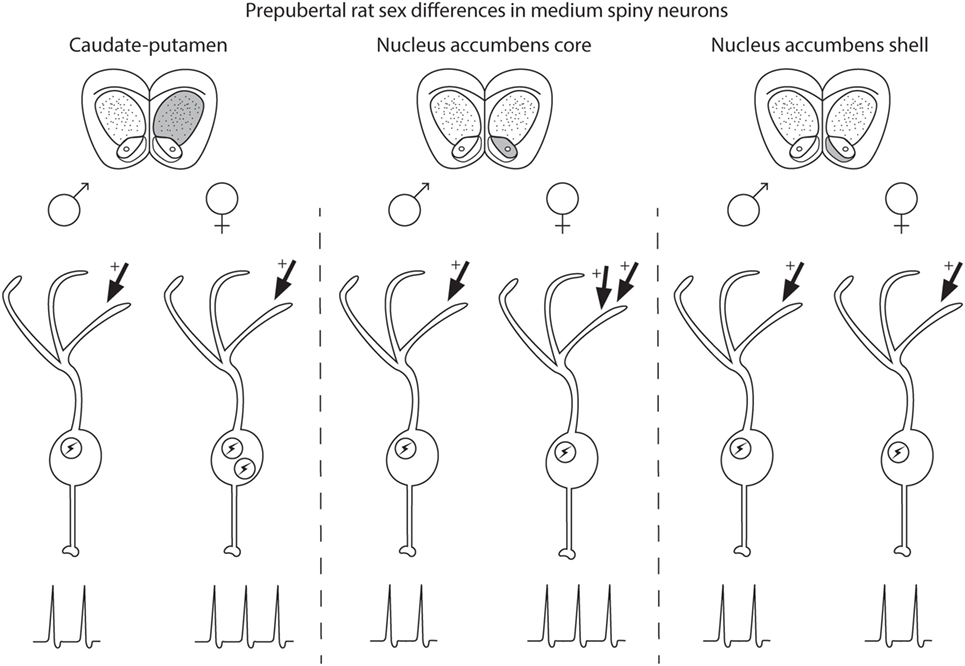
Figure 1. Schematic of sex differences in medium spiny neurons (MSNs) in prepubertal rat caudate–putamen, nucleus accumbens core (AcbC), and nucleus accumbens shell (AcbS). In general, MSNs recorded from prepubertal female rats exhibit increased excitation compared with male MSNs in the caudate–putamen and nucleus AcbC. However, the exact nature of the increased excitability in female MSNs differs by striatal region, encompassing changes in either intrinsic excitability (indicated in the schematic with an encircled lightning bolt) or excitatory synaptic input (indicated with arrows with plus signs). Differences in intrinsic excitability and excitatory synaptic input are indicated with more or less lightning bolts and arrows, respectively. Caudate–putamen MSNs show increased intrinsic excitability in prepubertal females compared with males. Nucleus AcbC MSNs receive augmented excitatory synaptic input in prepubertal females compared with males. Nucleus AcbS MSNs exhibit little evidence for sex differences in either intrinsic excitability or excitatory synaptic input in prepubertal animals.
Author Contributions
JM wrote the manuscript; DD prepared the figure; and JC, JW, DD, and JM edited and approved the manuscript and figure.
Conflict of Interest Statement
The authors declare that the research was conducted in the absence of any commercial or financial relationships that could be construed as a potential conflict of interest.
Funding
We acknowledge NIH MH109471 to JM and P30ES025128 (Center for Human Health and the Environment).
References
1. Cahill L. Fundamental sex difference in human brain architecture. Proc Natl Acad Sci U S A (2014) 111(2):577–8. doi:10.1073/pnas.1320954111
2. de Vries GJ, Forger NG. Sex differences in the brain: a whole body perspective. Biol Sex Differ (2015) 6:15. doi:10.1186/s13293-015-0032-z
3. McEwen BS, Milner TA. Understanding the broad influence of sex hormones and sex differences in the brain. J Neurosci Res (2017) 95(1–2):24–39. doi:10.1002/jnr.23809
4. McCarthy MM, Woolley CS, Arnold AP. Incorporating sex as a biological variable in neuroscience: what do we gain? Nat Rev Neurosci (2017) 18(12):707–8. doi:10.1038/nrn.2017.137
5. Breedlove SM, Hampson E. Sexual differentiation of the brain and behavior. In: Becker JB, Breedlove SM, Crews D, McCarthy MM, editors. Behavioral Endocrinology. London: MIT Press (2002). p. 75–114.
6. De Vries GJ. Minireview: sex differences in adult and developing brains: compensation, compensation, compensation. Endocrinology (2004) 145(3):1063–8. doi:10.1210/en.2003-1504
7. Breedlove SM, Arnold AP. Sexually dimorphic motor nucleus in the rat lumbar spinal cord: response to adult hormone manipulation, absence in androgen-insensitive rats. Brain Res (1981) 225(2):297–307. doi:10.1016/0006-8993(81)90837-4
8. Gorski RA, Gordon JH, Shryne JE, Southam AM. Evidence for a morphological sex difference within the medial preoptic area of the rat brain. Brain Res (1978) 148(2):333–46. doi:10.1016/0006-8993(78)90723-0
9. Nottebohm F, Arnold AP. Sexual dimorphism in vocal control areas of the songbird brain. Science (1976) 194(4261):211–3. doi:10.1126/science.959852
10. Beery AK, Zucker I. Sex bias in neuroscience and biomedical research. Neurosci Biobehav Rev (2011) 35(3):565–72. doi:10.1016/j.neubiorev.2010.07.002
11. Will TR, Proano SB, Thomas AM, Kunz LM, Thompson KC, Ginnari LA, et al. Problems and progress regarding sex bias and omission in neuroscience research. eNeuro (2017) 4(6):ENEURO.0278-17.2017. doi:10.1523/ENEURO.0278-17.2017
12. Shansky RM, Woolley CS. Considering sex as a biological variable will be valuable for neuroscience research. J Neurosci (2016) 36(47):11817–22. doi:10.1523/JNEUROSCI.1390-16.2016
13. Oberlander JG, Woolley CS. 17beta-estradiol acutely potentiates glutamatergic synaptic transmission in the hippocampus through distinct mechanisms in males and females. J Neurosci (2016) 36(9):2677–90. doi:10.1523/JNEUROSCI.4437-15.2016
14. Blume SR, Freedberg M, Vantrease JE, Chan R, Padival M, Record MJ, et al. Sex- and estrus-dependent differences in rat basolateral amygdala. J Neurosci (2017) 37(44):10567–86. doi:10.1523/JNEUROSCI.0758-17.2017
15. Salvatore M, Wiersielis K, Luz S, Waxler D, Bhatnagar S, Bangasser DA. Sex differences in circuits activated by corticotropin releasing factor in rats. Horm Behav (2018) 97:145–53.
16. Farrell MR, Holland FH, Shansky RM, Brenhouse HC. Sex-specific effects of early life stress on social interaction and prefrontal cortex dendritic morphology in young rats. Behav Brain Res (2016) 310:119–25. doi:10.1016/j.bbr.2016.05.009
17. Laman-Maharg A, Trainor BC. Stress, sex, and motivated behaviors. J Neurosci Res (2017) 95(1–2):83–92. doi:10.1002/jnr.23815
18. Terasaki LS, Gomez J, Schwarz JM. An examination of sex differences in the effects of early-life opiate and alcohol exposure. Philos Trans R Soc Lond B Biol Sci (2016) 371(1688):20150123. doi:10.1098/rstb.2015.0123
19. Beatty WW. Gonadal hormones and sex differences in nonreproductive behaviors in rodents: organizational and activational influences. Horm Behav (1979) 12(2):112–63. doi:10.1016/0018-506X(79)90017-5
20. Becker JB. Hormonal influences on sensorimotor function. In: Becker JB, Breedlove SM, Crews D, McCarthy MM, editors. Behavioral Endocrinology. Cambridge: MIT Press (2002). p. 497–525.
21. Carroll ME, Anker JJ. Sex differences and ovarian hormones in animal models of drug dependence. Horm Behav (2010) 58(1):44–56. doi:10.1016/j.yhbeh.2009.10.001
22. Yoest KE, Cummings JA, Becker JB. Estradiol, dopamine and motivation. Cent Nerv Syst Agents Med Chem (2014) 14(2):83–9. doi:10.2174/1871524914666141226103135
23. Tonn Eisinger KR, Larson EB, Boulware MI, Thomas MJ, Mermelstein PG. Membrane estrogen receptor signaling impacts the reward circuitry of the female brain to influence motivated behaviors. Steroids (2018) 133:53–9.
24. Becker JB, Snyder PJ, Miller MM, Westgate SA, Jenuwine MJ. The influence of estrous cycle and intrastriatal estradiol on sensorimotor performance in the female rat. Pharmacol Biochem Behav (1987) 27(1):53–9. doi:10.1016/0091-3057(87)90476-X
25. Smith SS. Female sex steroid hormones: from receptors to networks to performance – actions on the sensorimotor system. Prog Neurobiol (1994) 44(1):55–86. doi:10.1016/0301-0082(94)90057-4
26. Hampson E, Kimura D. Reciprocal effects of hormonal fluctuations on human motor and perceptual-spatial skills. Behav Neurosci (1988) 102(3):456–9. doi:10.1037/0735-7044.102.3.456
27. Hampson E. Estrogen-related variations in human spatial and articulatory-motor skills. Psychoneuroendocrinology (1990) 15(2):97–111. doi:10.1016/0306-4530(90)90018-5
28. Simic N, Tokic A, Pericic M. Performance of fine motor and spatial tasks during the menstrual cycle. Arh Hig Rada Toksikol (2010) 61(4):407–14. doi:10.2478/10004-1254-61-2010-2055
29. Jennings PJ, Janowsky JS, Orwoll E. Estrogen and sequential movement. Behav Neurosci (1998) 112(1):154–9. doi:10.1037/0735-7044.112.1.154
30. Zoghi M, Vaseghi B, Bastani A, Jaberzadeh S, Galea MP. The effects of sex hormonal fluctuations during menstrual cycle on cortical excitability and manual dexterity (a Pilot Study). PLoS One (2015) 10(8):e0136081. doi:10.1371/journal.pone.0136081
31. Andersen SL, Teicher MH. Sex differences in dopamine receptors and their relevance to ADHD. Neurosci Biobehav Rev (2000) 24(1):137–41. doi:10.1016/S0149-7634(99)00044-5
32. Bosse R, DiPaolo T. The modulation of brain dopamine and GABAA receptors by estradiol: a clue for CNS changes occurring at menopause. Cell Mol Neurobiol (1996) 16(2):199–212. doi:10.1007/BF02088176
33. Dluzen DE, McDermott JL. Gender differences in neurotoxicity of the nigrostriatal dopaminergic system: implications for Parkinson’s disease. J Gend Specif Med (2000) 3(6):36–42.
34. Elbaz A, Bower JH, Maraganore DM, McDonnell SK, Peterson BJ, Ahlskog JE, et al. Risk tables for parkinsonism and Parkinson’s disease. J Clin Epidemiol (2002) 55(1):25–31. doi:10.1016/S0895-4356(01)00425-5
35. Lynch WJ, Roth ME, Carroll ME. Biological basis of sex differences in drug abuse: preclinical and clinical studies. Psychopharmacology (2002) 164(2):121–37. doi:10.1007/s00213-002-1183-2
36. Pauls DL, Cohen DJ, Heimbuch R, Detlor J, Kidd KK. Familial pattern and transmission of Gilles de la Tourette syndrome and multiple tics. Arch Gen Psychiatry (1981) 38(10):1091–3. doi:10.1001/archpsyc.1981.01780350025002
37. Bode FJ, Stephan M, Suhling H, Pabst R, Straub RH, Raber KA, et al. Sex differences in a transgenic rat model of Huntington’s disease: decreased 17beta-estradiol levels correlate with reduced numbers of DARPP32+ neurons in males. Hum Mol Genet (2008) 17(17):2595–609. doi:10.1093/hmg/ddn159
38. Roos RA, Vegter-van der Vlis M, Hermans J, Elshove HM, Moll AC, van de Kamp JJ, et al. Age at onset in Huntington’s disease: effect of line of inheritance and patient’s sex. J Med Genet (1991) 28(8):515–9. doi:10.1136/jmg.28.8.515
39. Becker JB, McClellan ML, Reed BG. Sex differences, gender and addiction. J Neurosci Res (2017) 95(1–2):136–47. doi:10.1002/jnr.23963
40. Quinn NP, Marsden CD. Menstrual-related fluctuations in Parkinson’s disease. Mov Disord (1986) 1(1):85–7. doi:10.1002/mds.870010112
41. Castrioto A, Hulliger S, Poon YY, Lang AE, Moro E. A survey on the impact of the menstrual cycle on movement disorders severity. Can J Neurol Sci (2010) 37(4):478–81. doi:10.1017/S0317167100010490
42. Bobzean SA, DeNobrega AK, Perrotti LI. Sex differences in the neurobiology of drug addiction. Exp Neurol (2014) 259:64–74. doi:10.1016/j.expneurol.2014.01.022
43. Davis DM, Jacobson TK, Aliakbari S, Mizumori SJ. Differential effects of estrogen on hippocampal- and striatal-dependent learning. Neurobiol Learn Mem (2005) 84(2):132–7. doi:10.1016/j.nlm.2005.06.004
44. Fattore L, Melis M, Fadda P, Fratta W. Sex differences in addictive disorders. Front Neuroendocrinol (2014) 35:272–84. doi:10.1016/j.yfrne.2014.04.003
45. Pavon JM, Whitson HE, Okun MS. Parkinson’s disease in women: a call for improved clinical studies and for comparative effectiveness research. Maturitas (2010) 65(4):352–8. doi:10.1016/j.maturitas.2010.01.001
46. Zurkovsky L, Brown SL, Boyd SE, Fell JA, Korol DL. Estrogen modulates learning in female rats by acting directly at distinct memory systems. Neuroscience (2007) 144(1):26–37. doi:10.1016/j.neuroscience.2006.09.002
47. Gordon JH, Borison RL, Diamond BI. Modulation of dopamine receptor sensitivity by estrogen. Biol Psychiatry (1980) 15(3):389–96.
48. Hruska RE, Silbergeld EK. Increased dopamine receptor sensitivity after estrogen treatment using the rat rotation model. Science (1980) 208(4451):1466–8. doi:10.1126/science.7189902
49. Becker JB, Ramirez VD. Sex differences in the amphetamine stimulated release of catecholamines from rat striatal tissue in vitro. Brain Res (1981) 204(2):361–72. doi:10.1016/0006-8993(81)90595-3
50. Demotes-Mainard J, Arnauld E, Vincent JD. Estrogens modulate the responsiveness of in vivo recorded striatal neurons to lontophoretic application of dopamine in rats: role of D and D2 receptor activation. J Neuroendocrinol (1990) 2(6):825–32. doi:10.1111/j.1365-2826.1990.tb00647.x
51. Gordon JH, Perry KO. Pre- and postsynaptic neurochemical alterations following estrogen-induced striatal dopamine hypo- and hypersensitivity. Brain Res Bull (1983) 10(4):425–8. doi:10.1016/0361-9230(83)90137-5
52. Clopton J, Gordon JH. In vivo effects of estrogen and 2-hydroxyestradiol on D-2 dopamine receptor agonist affinity states in rat striatum. J Neural Transm (1986) 66(1):13–20. doi:10.1007/BF01262954
53. Levesque D, Gagnon S, Di Paolo T. Striatal D1 dopamine receptor density fluctuates during the rat estrous cycle. Neurosci Lett (1989) 98(3):345–50. doi:10.1016/0304-3940(89)90426-6
54. Levesque D, Di Paolo T. Rapid conversion of high into low striatal D2-dopamine receptor agonist binding states after an acute physiological dose of 17 beta-estradiol. Neurosci Lett (1988) 88(1):113–8. doi:10.1016/0304-3940(88)90324-2
55. Tonnaer JA, Leinders T, van Delft AM. Ovariectomy and subchronic estradiol-17 beta administration decrease dopamine D1 and D2 receptors in rat striatum. Psychoneuroendocrinology (1989) 14(6):469–76. doi:10.1016/0306-4530(89)90046-2
56. Bazzett TJ, Becker JB. Sex differences in the rapid and acute effects of estrogen on striatal D2 dopamine receptor binding. Brain Res (1994) 637(1–2):163–72. doi:10.1016/0006-8993(94)91229-7
57. Becker JB. Direct effect of 17 beta-estradiol on striatum: sex differences in dopamine release. Synapse (1990) 5(2):157–64. doi:10.1002/syn.890050211
58. Walker QD, Rooney MB, Wightman RM, Kuhn CM. Dopamine release and uptake are greater in female than male rat striatum as measured by fast cyclic voltammetry. Neuroscience (2000) 95(4):1061–70. doi:10.1016/S0306-4522(99)00500-X
59. Calipari ES, Juarez B, Morel C, Walker DM, Cahill ME, Ribeiro E, et al. Dopaminergic dynamics underlying sex-specific cocaine reward. Nat Commun (2017) 8:13877. doi:10.1038/ncomms13877
61. Euvrard C, Labrie F, Boissier JR. Effect of estrogen on changes in the activity of striatal cholinergic neurons induced by DA drugs. Brain Res (1979) 169(1):215–20. doi:10.1016/0006-8993(79)90392-5
62. Becker JB, Perry AN, Westenbroek C. Sex differences in the neural mechanisms mediating addiction: a new synthesis and hypothesis. Biol Sex Differ (2013) 3(1):14. doi:10.1186/2042-6410-3-14
63. Di Paolo T. Modulation of brain dopamine transmission by sex steroids. Rev Neurosci (1994) 5(1):27–41. doi:10.1515/REVNEURO.1994.5.1.27
64. Walker QD, Ray R, Kuhn CM. Sex differences in neurochemical effects of dopaminergic drugs in rat striatum. Neuropsychopharmacology (2006) 31(6):1193–202. doi:10.1038/sj.npp.1300915
65. Wong JE, Cao J, Dorris DM, Meitzen J. Genetic sex and the volumes of the caudate-putamen, nucleus accumbens core and shell: original data and a review. Brain Struct Funct (2016) 221(8):4257–67. doi:10.1007/s00429-015-1158-9
66. Meitzen J, Pflepsen KR, Stern CM, Meisel RL, Mermelstein PG. Measurements of neuron soma size and density in rat dorsal striatum, nucleus accumbens core and nucleus accumbens shell: differences between striatal region and brain hemisphere, but not sex. Neurosci Lett (2011) 487(2):177–81. doi:10.1016/j.neulet.2010.10.017
68. Rapanelli M, Frick LR, Xu M, Groman SM, Jindachomthong K, Tamamaki N, et al. Targeted interneuron depletion in the dorsal striatum produces autism-like behavioral abnormalities in male but not female mice. Biol Psychiatry (2017) 82(3):194–203. doi:10.1016/j.biopsych.2017.01.020
69. Arnauld E, Dufy B, Pestre M, Vincent JD. Effects of estrogens on the responses of caudate neurons to microiontophoretically applied dopamine. Neurosci Lett (1981) 21(3):325–31. doi:10.1016/0304-3940(81)90225-1
70. Tansey EM, Arbuthnott GW, Fink G, Whale D. Oestradiol-17 beta increases the firing rate of antidromically identified neurones of the rat neostriatum. Neuroendocrinology (1983) 37(2):106–10. doi:10.1159/000123527
71. Mermelstein PG, Becker JB, Surmeier DJ. Estradiol reduces calcium currents in rat neostriatal neurons via a membrane receptor. J Neurosci (1996) 16(2):595–604. doi:10.1523/JNEUROSCI.16-02-00595.1996
72. Grove-Strawser D, Boulware MI, Mermelstein PG. Membrane estrogen receptors activate the metabotropic glutamate receptors mGluR5 and mGluR3 to bidirectionally regulate CREB phosphorylation in female rat striatal neurons. Neuroscience (2010) 170:1045–55. doi:10.1016/j.neuroscience.2010.08.012
73. Almey A, Filardo EJ, Milner TA, Brake WG. Estrogen receptors are found in glia and at extranuclear neuronal sites in the dorsal striatum of female rats: evidence for cholinergic but not dopaminergic colocalization. Endocrinology (2012) 153(11):5373–83. doi:10.1210/en.2012-1458
74. Almey A, Milner TA, Brake WG. Estrogen receptors in the central nervous system and their implication for dopamine-dependent cognition in females. Horm Behav (2015) 74:125–38. doi:10.1016/j.yhbeh.2015.06.010
75. Almey A, Milner TA, Brake WG. Estrogen receptor alpha and G-protein coupled estrogen receptor 1 are localised to GABAergic neurons in the dorsal striatum. Neurosci Lett (2016) 622:118–23. doi:10.1016/j.neulet.2016.04.023
76. Bourque M, Morissette M, Di Paolo T. Raloxifene activates G protein-coupled estrogen receptor 1/Akt signaling to protect dopamine neurons in 1-methyl-4-phenyl-1,2,3,6-tetrahydropyridine mice. Neurobiol Aging (2014) 35(10):2347–56. doi:10.1016/j.neurobiolaging.2014.03.017
77. Schultz KN, von Esenwein SA, Hu M, Bennett AL, Kennedy RT, Musatov S, et al. Viral vector-mediated overexpression of estrogen receptor-alpha in striatum enhances the estradiol-induced motor activity in female rats and estradiol-modulated GABA release. J Neurosci (2009) 29(6):1897–903. doi:10.1523/JNEUROSCI.4647-08.2009
78. Kuppers E, Beyer C. Expression of estrogen receptor-alpha and beta mRNA in the developing and adult mouse striatum. Neurosci Lett (1999) 276(2):95–8. doi:10.1016/S0304-3940(99)00815-0
79. Foidart A, Harada N, Balthazart J. Aromatase-immunoreactive cells are present in mouse brain areas that are known to express high levels of aromatase activity. Cell Tissue Res (1995) 280(3):561–74. doi:10.1007/BF00318360
80. Stanic D, Dubois S, Chua HK, Tonge B, Rinehart N, Horne MK, et al. Characterization of aromatase expression in the adult male and female mouse brain. I. Coexistence with oestrogen receptors alpha and beta, and androgen receptors. PLoS One (2014) 9(3):e90451. doi:10.1371/journal.pone.0090451
81. Tozzi A, de Iure A, Tantucci M, Durante V, Quiroga-Varela A, Giampa C, et al. Endogenous 17beta-estradiol is required for activity-dependent long-term potentiation in the striatum: interaction with the dopaminergic system. Front Cell Neurosci (2015) 9:192. doi:10.3389/fncel.2015.00192
82. Kuppers E, Beyer C. Expression of aromatase in the embryonic and postnatal mouse striatum. Brain Res Mol Brain Res (1998) 63(1):184–8. doi:10.1016/S0169-328X(98)00279-4
83. Warembourg M, Jolivet A, Milgrom E. Immunohistochemical evidence of the presence of estrogen and progesterone receptors in the same neurons of the guinea pig hypothalamus and preoptic area. Brain Res (1989) 480(1–2):1–15. doi:10.1016/0006-8993(89)91561-8
84. Lauber AH, Romano GJ, Pfaff DW. Gene expression for estrogen and progesterone receptor mRNAs in rat brain and possible relations to sexually dimorphic functions. J Steroid Biochem Mol Biol (1991) 40(1–3):53–62. doi:10.1016/0960-0760(91)90167-4
85. Milner TA, Thompson LI, Wang G, Kievits JA, Martin E, Zhou P, et al. Distribution of estrogen receptor beta containing cells in the brains of bacterial artificial chromosome transgenic mice. Brain Res (2010) 1351:74–96. doi:10.1016/j.brainres.2010.06.038
86. Forlano PM, Woolley CS. Quantitative analysis of pre- and postsynaptic sex differences in the nucleus accumbens. J Comp Neurol (2010) 518(8):1330–48. doi:10.1002/cne.22279
87. Wissman AM, May RM, Woolley CS. Ultrastructural analysis of sex differences in nucleus accumbens synaptic connectivity. Brain Struct Funct (2012) 217(2):181–90. doi:10.1007/s00429-011-0353-6
88. Wissman AM, McCollum AF, Huang GZ, Nikrodhanond AA, Woolley CS. Sex differences and effects of cocaine on excitatory synapses in the nucleus accumbens. Neuropharmacology (2011) 61:217–27. doi:10.1016/j.neuropharm.2011.04.002
89. Huang YH, Schluter OM, Dong Y. Silent synapses speak up: updates of the neural rejuvenation hypothesis of drug addiction. Neuroscientist (2015) 21(5):451–9. doi:10.1177/1073858415579405
90. Grueter BA, Rothwell PE, Malenka RC. Integrating synaptic plasticity and striatal circuit function in addiction. Curr Opin Neurobiol (2012) 22(3):545–51. doi:10.1016/j.conb.2011.09.009
91. Robinson TE, Kolb B. Structural plasticity associated with exposure to drugs of abuse. Neuropharmacology (2004) 47:33–46. doi:10.1016/j.neuropharm.2004.06.025
92. Roitman MF, Na E, Anderson G, Jones TA, Bernstein IL. Induction of a salt appetite alters dendritic morphology in nucleus accumbens and sensitizes rats to amphetamine. J Neurosci (2002) 22(11):RC225.
93. Meisel RL, Mullins AJ. Sexual experience in female rodents: cellular mechanisms and functional consequences. Brain Res (2006) 1126(1):56–65. doi:10.1016/j.brainres.2006.08.050
94. Wolf ME. The Bermuda Triangle of cocaine-induced neuroadaptations. Trends Neurosci (2010) 33(9):391–8. doi:10.1016/j.tins.2010.06.003
95. Sazdanovic M, Slobodanka M, Zivanovic-Macuzic I, Jeremic D, Tanaskovic I, Milosavljevic Z, et al. Sexual dimorphism of medium-sized neurons with spines in human nucleus accumbens. Arch Biol Sci Belgrade (2013) 65(3):1149–55. doi:10.2298/ABS1303149S
96. Meisel RL, Luttrell VR. Estradiol increases the dendritic length of ventromedial hypothalamic neurons in female Syrian hamsters. Brain Res Bull (1990) 25(1):165–8. doi:10.1016/0361-9230(90)90269-6
97. Gould E, Woolley CS, Frankfurt M, McEwen BS. Gonadal steroids regulate dendritic spine density in hippocampal pyramidal cells in adulthood. J Neurosci (1990) 10(4):1286–91. doi:10.1523/JNEUROSCI.10-04-01286.1990
98. Woolley CS, McEwen BS. Estradiol mediates fluctuation in hippocampal synapse density during the estrous cycle in the adult rat. J Neurosci (1992) 12(7):2549–54. doi:10.1523/JNEUROSCI.12-07-02549.1992
99. Staffend NA, Loftus CM, Meisel RL. Estradiol reduces dendritic spine density in the ventral striatum of female Syrian hamsters. Brain Struct Funct (2011) 215(3–4):187–94. doi:10.1007/s00429-010-0284-7
100. Peterson BM, Martinez LA, Meisel RL, Mermelstein PG. Estradiol impacts the endocannabinoid system in female rats to influence behavioral and structural responses to cocaine. Neuropharmacology (2016) 110:118–24. doi:10.1016/j.neuropharm.2016.06.002
101. Peterson BM, Mermelstein PG, Meisel RL. Estradiol mediates dendritic spine plasticity in the nucleus accumbens core through activation of mGluR5. Brain Struct Funct (2015) 220:2415–22. doi:10.1007/s00429-014-0794-9
102. Martinez LA, Gross KS, Himmler BT, Emmitt NL, Peterson BM, Zlebnik NE, et al. Estradiol facilitation of cocaine self-administration in female rats requires activation of mGluR5. eNeuro (2016) 3(5):ENEURO.0140-16.2016. doi:10.1523/ENEURO.0140-16.2016
103. Martinez LA, Peterson BM, Meisel RL, Mermelstein PG. Estradiol facilitation of cocaine-induced locomotor sensitization in female rats requires activation of mGluR5. Behav Brain Res (2014) 271:39–42. doi:10.1016/j.bbr.2014.05.052
104. Willett JA, Will TR, Hauser CA, Dorris DM, Cao J, Meitzen J. No evidence for sex differences in the electrophysiological properties and excitatory synaptic input onto nucleus accumbens shell medium spiny neurons. eNeuro (2016) 3(1):ENEURO.0147-15.2016. doi:10.1523/ENEURO.0147-15.2016
105. Hodes GE, Pfau ML, Purushothaman I, Ahn HF, Golden SA, Christoffel DJ, et al. Sex differences in nucleus accumbens transcriptome profiles associated with susceptibility versus resilience to subchronic variable stress. J Neurosci (2015) 35(50):16362–76. doi:10.1523/JNEUROSCI.1392-15.2015
106. Brancato A, Bregman D, Ahn HF, Pfau ML, Menard C, Cannizzaro C, et al. Sub-chronic variable stress induces sex-specific effects on glutamatergic synapses in the nucleus accumbens. Neuroscience (2017) 350(14 May):180–9. doi:10.1016/j.neuroscience.2017.03.014
107. Wallin-Miller K, Li G, Kelishani D, Wood RI. Anabolic-androgenic steroids decrease dendritic spine density in the nucleus accumbens of male rats. Neuroscience (2016) 330:72–8. doi:10.1016/j.neuroscience.2016.05.045
108. Frye CA, Rhodes ME, Rosellini R, Svare B. The nucleus accumbens as a site of action for rewarding properties of testosterone and its 5alpha-reduced metabolites. Pharmacol Biochem Behav (2002) 74(1):119–27. doi:10.1016/S0091-3057(02)00968-1
109. Cao J, Dorris DM, Meitzen J. Neonatal masculinization blocks increased excitatory synaptic input in female rat nucleus accumbens core. Endocrinology (2016) 157(8):3181–96. doi:10.1210/en.2016-1160
110. Groenewegen HJ, Wright CI, Beijer AV, Voorn P. Convergence and segregation of ventral striatal inputs and outputs. Ann N Y Acad Sci (1999) 877:49–63. doi:10.1111/j.1749-6632.1999.tb09260.x
111. Britt JP, Benaliouad F, McDevitt RA, Stuber GD, Wise RA, Bonci A. Synaptic and behavioral profile of multiple glutamatergic inputs to the nucleus accumbens. Neuron (2012) 76(4):790–803. doi:10.1016/j.neuron.2012.09.040
112. Ito R, Robbins TW, Everitt BJ. Differential control over cocaine-seeking behavior by nucleus accumbens core and shell. Nat Neurosci (2004) 7(4):389–97. doi:10.1038/nn1217
113. Pulvirenti L, Berrier R, Kreifeldt M, Koob GF. Modulation of locomotor activity by NMDA receptors in the nucleus accumbens core and shell regions of the rat. Brain Res (1994) 664(1–2):231–6. doi:10.1016/0006-8993(94)91977-1
114. Bradley KC, Boulware MB, Jiang H, Doerge RW, Meisel RL, Mermelstein PG. Changes in gene expression within the nucleus accumbens and striatum following sexual experience. Genes Brain Behav (2005) 4(1):31–44. doi:10.1111/j.1601-183X.2004.00093.x
115. Henschen CW, Palmiter RD, Darvas M. Restoration of dopamine signaling to the dorsal striatum is sufficient for aspects of active maternal behavior in female mice. Endocrinology (2013) 154(11):4316–27. doi:10.1210/en.2013-1257
116. Pena CJ, Neugut YD, Calarco CA, Champagne FA. Effects of maternal care on the development of midbrain dopamine pathways and reward-directed behavior in female offspring. Eur J Neurosci (2014) 39(6):946–56. doi:10.1111/ejn.12479
117. O’Connell LA, Hofmann HA. The vertebrate mesolimbic reward system and social behavior network: a comparative synthesis. J Comp Neurol (2011) 519(18):3599–639. doi:10.1002/cne.22735
118. West AE, Carelli RM. Nucleus accumbens core and shell differentially encode reward-associated cues after reinforcer devaluation. J Neurosci (2016) 36(4):1128–39. doi:10.1523/JNEUROSCI.2976-15.2016
119. Corbit LH, Balleine BW. The general and outcome-specific forms of Pavlovian-instrumental transfer are differentially mediated by the nucleus accumbens core and shell. J Neurosci (2011) 31(33):11786–94. doi:10.1523/JNEUROSCI.2711-11.2011
120. Corbit LH, Muir JL, Balleine BW. The role of the nucleus accumbens in instrumental conditioning: evidence of a functional dissociation between accumbens core and shell. J Neurosci (2001) 21(9):3251–60.
121. Willett JA, Johnson AG, Vogel AR, Patisaul HB, McGraw LA, Meitzen J. Nucleus accumbens core medium spiny neuron electrophysiological properties and partner preference behavior in the adult male prairie vole, Microtus ochrogaster. J Neurophysiol (2018). doi:10.1152/jn.00737.2017
122. Lerner TN, Ye L, Deisseroth K. Communication in neural circuits: tools, opportunities, and challenges. Cell (2016) 164(6):1136–50. doi:10.1016/j.cell.2016.02.027
123. Chalfin L, Dayan M, Levy DR, Austad SN, Miller RA, Iraqi FA, et al. Mapping ecologically relevant social behaviours by gene knockout in wild mice. Nat Commun (2014) 5:4569. doi:10.1038/ncomms5569
124. Parker CC, Chen H, Flagel SB, Geurts AM, Richards JB, Robinson TE, et al. Rats are the smart choice: rationale for a renewed focus on rats in behavioral genetics. Neuropharmacology (2014) 76(Pt B):250–8. doi:10.1016/j.neuropharm.2013.05.047
125. Bracci E, Panzeri S. Excitatory GABAergic effects in striatal projection neurons. J Neurophysiol (2006) 95(2):1285–90. doi:10.1152/jn.00598.2005
126. Hu M, Watson CJ, Kennedy RT, Becker JB. Estradiol attenuates the K+-induced increase in extracellular GABA in rat striatum. Synapse (2006) 59(2):122–4. doi:10.1002/syn.20221
127. Dorris DM, Cao J, Willett JA, Hauser CA, Meitzen J. Intrinsic excitability varies by sex in pre-pubertal striatal medium spiny neurons. J Neurophysiol (2015) 113(3):720–9. doi:10.1152/jn.00687.2014
128. Bayless DW, Daniel JM. Sex differences in myelin-associated protein levels within and density of projections between the orbital frontal cortex and dorsal striatum of adult rats: implications for inhibitory control. Neuroscience (2015) 300:286–96. doi:10.1016/j.neuroscience.2015.05.029
129. Morissette M, Garcia-Segura LM, Belanger A, Di Paolo T. Changes of rat striatal neuronal membrane morphology and steroid content during the estrous cycle. Neuroscience (1992) 49(4):893–902. doi:10.1016/0306-4522(92)90365-9
131. Ishikawa M, Mu P, Moyer JT, Wolf JA, Quock RM, Davies NM, et al. Homeostatic synapse-driven membrane plasticity in nucleus accumbens neurons. J Neurosci (2009) 29(18):5820–31. doi:10.1523/JNEUROSCI.5703-08.2009
132. Kourrich S, Thomas MJ. Similar neurons, opposite adaptations: psychostimulant experience differentially alters firing properties in accumbens core versus shell. J Neurosci (2009) 29(39):12275–83. doi:10.1523/JNEUROSCI.3028-09.2009
133. Mu P, Moyer JT, Ishikawa M, Zhang Y, Panksepp J, Sorg BA, et al. Exposure to cocaine dynamically regulates the intrinsic membrane excitability of nucleus accumbens neurons. J Neurosci (2010) 30(10):3689–99. doi:10.1523/JNEUROSCI.4063-09.2010
134. Zhang XF, Hu XT, White FJ. Whole-cell plasticity in cocaine withdrawal: reduced sodium currents in nucleus accumbens neurons. J Neurosci (1998) 18(1):488–98. doi:10.1523/JNEUROSCI.18-01-00488.1998
135. Gertler TS, Chan CS, Surmeier DJ. Dichotomous anatomical properties of adult striatal medium spiny neurons. J Neurosci (2008) 28(43):10814–24. doi:10.1523/JNEUROSCI.2660-08.2008
136. Planert H, Berger TK, Silberberg G. Membrane properties of striatal direct and indirect pathway neurons in mouse and rat slices and their modulation by dopamine. PLoS One (2013) 8(3):e57054. doi:10.1371/journal.pone.0057054
137. Otaka M, Ishikawa M, Lee BR, Liu L, Neumann PA, Cui R, et al. Exposure to cocaine regulates inhibitory synaptic transmission in the nucleus accumbens. J Neurosci (2013) 33(16):6753–8. doi:10.1523/JNEUROSCI.4577-12.2013
138. Schulz DJ. Plasticity and stability in neuronal output via changes in intrinsic excitability: it’s what’s inside that counts. J Exp Biol (2006) 209(Pt 24):4821–7. doi:10.1242/jeb.02567
139. Suska A, Lee BR, Huang YH, Dong Y, Schluter OM. Selective presynaptic enhancement of the prefrontal cortex to nucleus accumbens pathway by cocaine. Proc Natl Acad Sci U S A (2013) 110(2):713–8. doi:10.1073/pnas.1206287110
140. Zakon HH. The effects of steroid hormones on electrical activity of excitable cells. Trends Neurosci (1998) 21(5):202–7. doi:10.1016/S0166-2236(97)01209-5
141. Cooke BM. Synaptic reorganisation of the medial amygdala during puberty. J Neuroendocrinol (2011) 23(1):65–73. doi:10.1111/j.1365-2826.2010.02075.x
142. Cooke BM, Woolley CS. Effects of prepubertal gonadectomy on a male-typical behavior and excitatory synaptic transmission in the amygdala. Dev Neurobiol (2009) 69(2–3):141–52. doi:10.1002/dneu.20688
143. Andersen SL, Rutstein M, Benzo JM, Hostetter JC, Teicher MH. Sex differences in dopamine receptor overproduction and elimination. Neuroreport (1997) 8(6):1495–8. doi:10.1097/00001756-199704140-00034
144. Andersen SL, Thompson AP, Krenzel E, Teicher MH. Pubertal changes in gonadal hormones do not underlie adolescent dopamine receptor overproduction. Psychoneuroendocrinology (2002) 27(6):683–91. doi:10.1016/S0306-4530(01)00069-5
145. Woolley CS. Acute effects of estrogen on neuronal physiology. Annu Rev Pharmacol Toxicol (2007) 47:657–80. doi:10.1146/annurev.pharmtox.47.120505.105219
146. Wu WW, Adelman JP, Maylie J. Ovarian hormone deficiency reduces intrinsic excitability and abolishes acute estrogen sensitivity in hippocampal CA1 pyramidal neurons. J Neurosci (2011) 31(7):2638–48. doi:10.1523/JNEUROSCI.6081-10.2011
147. Joel D, McCarthy MM. Incorporating sex as a biological variable in neuropsychiatric research: where are we now and where should we be? Neuropsychopharmacology (2017) 42(2):379–85. doi:10.1038/npp.2016.79
Keywords: sex, estradiol, hormones, striatum, medium spiny neuron, nucleus accumbens, caudate–putamen, electrophysiology
Citation: Cao J, Willett JA, Dorris DM and Meitzen J (2018) Sex Differences in Medium Spiny Neuron Excitability and Glutamatergic Synaptic Input: Heterogeneity Across Striatal Regions and Evidence for Estradiol-Dependent Sexual Differentiation. Front. Endocrinol. 9:173. doi: 10.3389/fendo.2018.00173
Received: 19 December 2017; Accepted: 03 April 2018;
Published: 18 April 2018
Edited by:
Vance Trudeau, University of Ottawa, CanadaReviewed by:
Richard Anthony DeFazio, University of Michigan, United StatesWudu E. Lado, University of Toronto, Canada
Copyright: © 2018 Cao, Willett, Dorris and Meitzen. This is an open-access article distributed under the terms of the Creative Commons Attribution License (CC BY). The use, distribution or reproduction in other forums is permitted, provided the original author(s) and the copyright owner are credited and that the original publication in this journal is cited, in accordance with accepted academic practice. No use, distribution or reproduction is permitted which does not comply with these terms.
*Correspondence: John Meitzen, amVtZWl0emVAbmNzdS5lZHU=