- 1Department of Physiological Sciences, Federal University of Espirito Santo, Vitoria, Brazil
- 2Pitagoras College, Guarapari, Brazil
- 3Department of Health Sciences, Federal University of Espirito Santo, São Mateus, Brazil
Organotins (OTs) are pollutants that are used widely by industry as disinfectants, pesticides, and most frequently as biocides in antifouling paints. This mini-review presents the main evidences from the literature about morphophysiological changes induced by OTs in the mammal endocrine system, focusing on the metabolism and reproductive control. Similar to other toxic compounds, the main effects with potential health risks to humans and experimental animals are not only related to dose and time of exposure but also to age, gender, and tissue/cell exposed. Regarding the underlying mechanisms, current literature indicates that OTs can directly damage endocrine glands, as well as interfere with neurohormonal control of endocrine function (i.e., in the hypothalamic–pituitary axis), altering hormone synthesis and/or bioavailability or activity of hormone receptors in the target cells. Importantly, OTs induces biochemical and morphological changes in gonads, abnormal steroidogenesis, both associated with reproductive dysfunctions such as irregular estrous cyclicity in female or spermatogenic disorders in male animals. Additionally, due to their role on endocrine systems predisposing to obesity, OTs are also included in the metabolism disrupting chemical hypothesis, either by central (e.g., accurate nucleus and lateral hypothalamus) or peripheral (e.g., adipose tissue) mechanisms. Thus, OTs should be indeed considered a major endocrine disruptor, being indispensable to understand the main toxic effects on the different tissues and its causative role for endocrine, metabolic, and reproductive dysfunctions observed.
Introduction
Organotins (OTs) belong to a class of pollutants described as organometallic used for various industrial purposes as disinfectants of water for industrial refrigeration, pesticides, biocides in antifouling paints, and wood preservatives (1–11).
Actually, tin-based compounds are known since the bronze age in the production of different metal alloys (12). However, the industrial use was consolidated only around 1940 as an efficient chemical stabilizer for plastic manufacture (5). Afterward, the biocidal effect of OTs was discovered and thus became intensively employed in a number of other commercial purposes. In this context, it is worth noting the use as an active principle of antifouling paints for boats and ships, reaching the apex in the 1990s, when about 80% of the boats worldwide used OT-based products (1, 3, 4).
Tin usually binds to non-polar radicals resulting in hydrophobic compounds; and due to their physicochemical properties, OTs are easily absorbed along the food chain. The effects depend greatly on the number and nature of radicals bound to the tin atom, being the tri-substituted (triorganostannic) forms, such as tributyltin (TBT) and triphenyltin (TPT) the most toxic. Fortunately, TBT is degraded in the environment to dibutyltin and then to monobutyltin (5, 12–16).
The TBT toxicology has become a major concern for the scientific community since the 1970s when toxic effects were discovered in different animal models, including mammals. As a result, researches were driven to better understand the actual impact of OT pollution for health and environmental risk (17, 18). These compounds can be easily assimilated by living organisms; in marine environment, for example, OTs are incorporated into soil and organic surface sediments such as phytoplankton, being absorbed by animals and plants of aquatic ecosystems (5, 19).
Figure 1 represents a visual summary of the main route of exposure to OTs for humans and the potential consequences for the endocrine system. Studies have shown that OTs cause several damages, including genetic, hepatic, renal, adrenal, neural, and immune toxicity (20–24). More importantly, recent reports indicate that TBT is a highly persistent chemical in the environment and food chain, being considered one of the largest existing endocrine disruptor with consequences to different hormonal functions (3, 20, 21, 25–27).
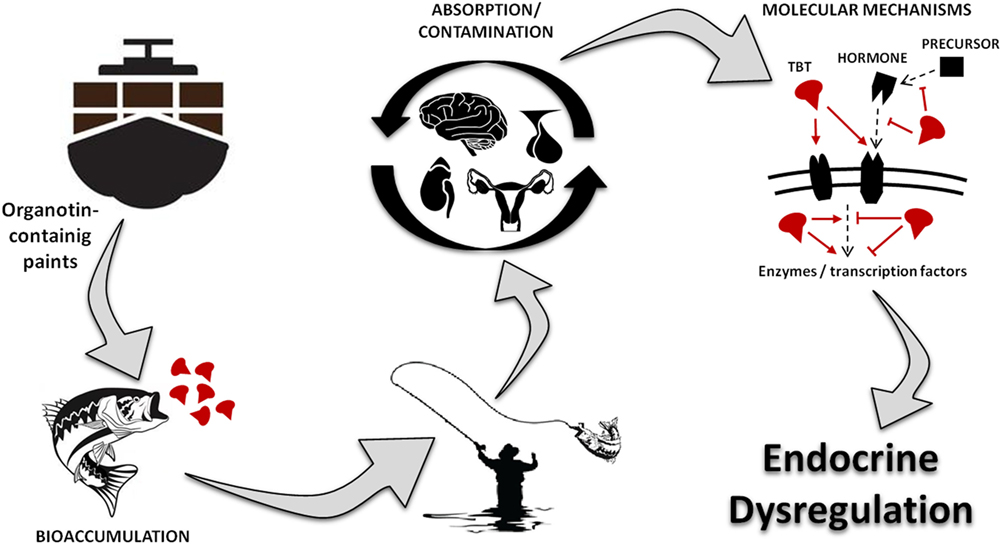
Figure 1. Visual abstract showing the main route of exposure to organotins for humans, underlying toxic mechanism and the potential consequences for the endocrine system. TBT, tributyltin.
As illustrated at Figure 2 and described in this mini-review, OTs are capable of altering the endocrine physiology at numerous levels: changing the pattern of hormone regulation, production, mechanisms of action or hormone elimination, and mimicking or blocking hormonal action (27–31). In this way, it is not possible to point out an exact toxic effect, whether acting directly on the endocrine glands, compromising hormonal receptors at the target cells, or both. Among all, one of the most iconic effects was noted in contaminated shellfish. These organisms undergo a phenomenon denominated “imposex,” that is the superposition of male genital organs in female individuals (32, 33). Notwithstanding, this endocrine disruptor has been proven as able to reduce circulating estrogen levels and cause morphophysiological damages also in reproductive organs of vertebrates, including mammals (5, 20, 21, 26, 34).
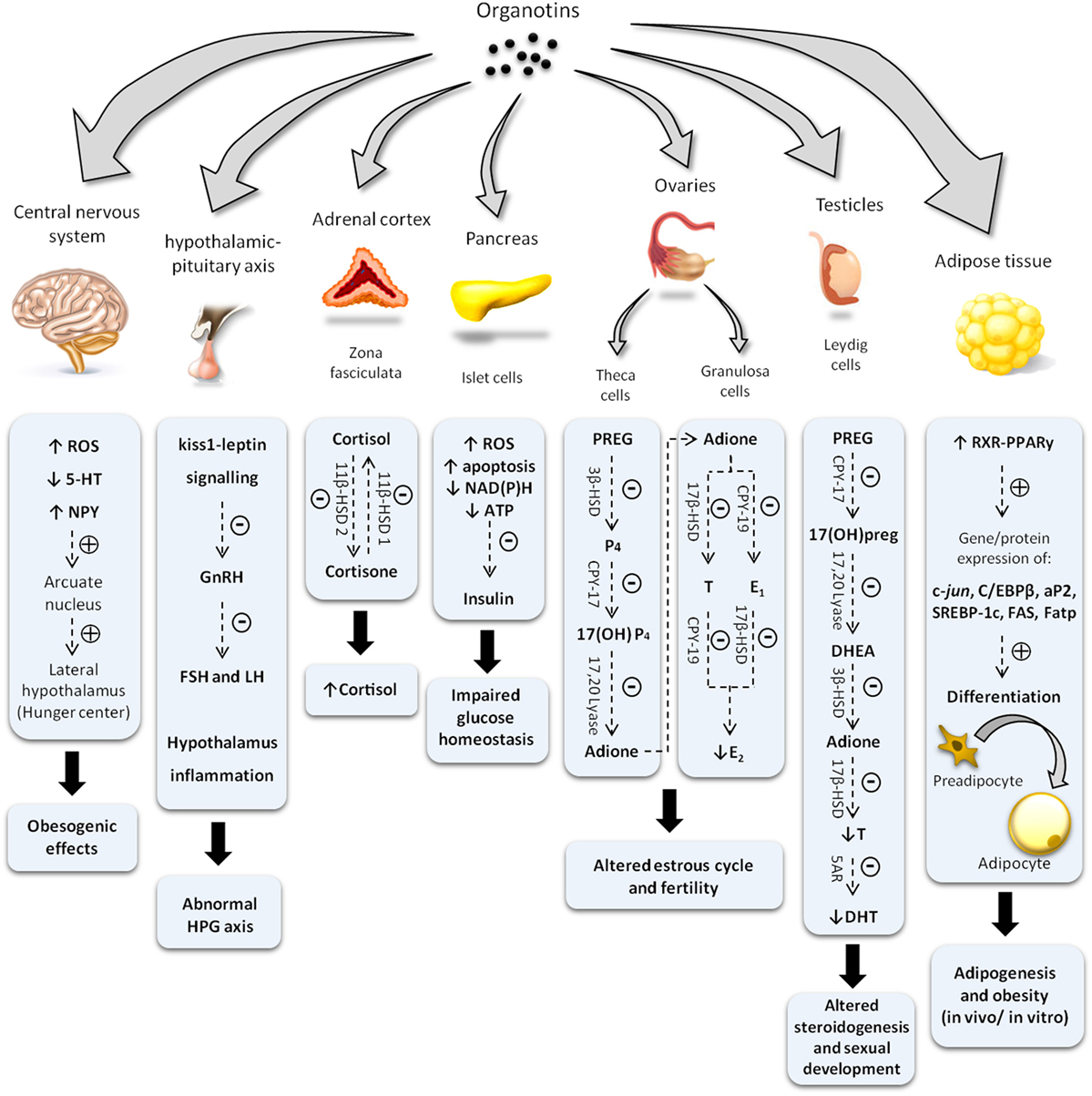
Figure 2. Diagram illustrating changes in endocrine system induced by organotin and major mechanisms involved. Symbols (−) or (+) indicate inhibitory and stimulatory effects, respectively. ROS, reactive oxygen species; 5-HT, serotonine; NPY, neuropeptide Y; GnRH, gonadotropin-releasing hormone; FSH, follicle-stimulating hormone; LH, luteinizing hormone; HPG, hypothalamus–pituitary–gonad; ATP, adenosine triphosphate; NAD(P)H, nicotinamide adenine dinucleotide phosphate; 11β-HSD, 11 β-hydroxysteroid dehydrogenase; PREG, pregnenolone; 3β-HSD, 3β-hydroxysteroid dehydrogenase; P4, progesterone; E1, estrone; E2, 17-β estradiol; T, testosterone; CPY-17, 17α-hydroxylase; Adione, androstenedione; CPY-19, aromatase; 17(OH)preg, 17(OH) pregnenolone; DHEA, dehydroepiandrosterone; 5AR, 5α-reductase; DHT, dihydrotestosterone; C/EBPβ, CCAAT/enhancer binding proteins; SREBP-1c, sterol regulatory element-binding protein 1c; aP2, adipocyte-protein 2; FAS, fatty acid synthase; Fatp, fatty acid transport proteins.
Neuroendocrine Changes in Mammals Induced by Organotin: A Focus on the Metabolism and the Reproductive Function
In mammals, OTs administered at different doses induce morphological and functional changes in several tissues involved in the control of endocrine function and metabolism, such as the, hypothalamus, pituitary, pancreas, gonads, adipose tissue, adrenal, and thyroid glands (35–38).
The role of environmental pollutants such as OTs on the endocrine system also supports the metabolism disrupting chemical hypothesis (formerly termed “obesogen hypothesis”), which postulates that several environmental toxic chemicals, by altering the endocrine function, can induce metabolic changes related to obesity, impaired glucose metabolism, and dyslipidemia (39, 40). These endocrine and metabolic disorders caused by OTs, particularly obesity, may occur by central and peripheral mechanisms (41, 42). In fact, there are evidences that morphofunctional changes in both fatty tissues and central nervous system may contribute to the deleterious effects of these compounds related to obesity and metabolic syndrome complications (3, 20, 42–47).
In the central nervous system, OTs promote important neurotoxic effects with changes on behavior, metabolism, and neuroendocrine control (48–51). Experimental studies have found decreased levels of dopamine, noradrenaline, and serotonin in mice brains (48), reduced neuron counting and increased glutamate-induced calcium permeability in neuronal membrane from rats (49), and an increase in reactive oxygen-derived species and oxidative damage associated with reduced antioxidant reserves in the nervous system exposed to TBT (50, 51). TBT also exerts its toxicity on other regions of the nervous system, as it is shown by disruption of the rat hypothalamic–pituitary–adrenal axis (22). In addition, the effects of OTs exposure on the brain are not restricted to general neurotoxic effects, but also to changes in neurohormonal control of metabolism and food intake. TBT administered acutely in mice activates the arcuate nucleus and the hunger center of the hypothalamus (46), and in rats increases neuropeptide Y (NPY) expression in the brain, in association with increased body weight, fat mass, and food intake (47). In addition, mice chronically exposed to low doses of TBT exhibited increased food efficiency and reduced leptin circulating levels associated with changes on the leptin–NPY–NPY–Y1 receptor axis in the hypothalamus (52). In this regard, it is well known the importance of leptin modulating the expression of NPY in the hypothalamus and, thus, the food intake. In fact, OT-induced changes on the leptin-NPY axis are associated with obesity due to increased food intake and decreased energy expenditure (53).
In relation to peripheral mechanisms involved in the obesogenic effect, it is well described the association between tin-based compounds and adipogenesis, through signaling between retinoid X receptor (RXR) and peroxisome proliferator-activator receptor gamma (PPARγ) (20, 25, 54–57). There are evidences that TBT increases adipocyte markers expression, lipid accumulation and glucose uptake in preadipocytes (36, 58, 59), and induces a differentiation to adipocytes by RXR/PPARγ activation (42–45). Notwithstanding, it is know that PPARγ plays also an orexigenic role, attributed to its central effects especially in both the NPY and agouti-related protein at the arcuate hypothalamic nucleus (60, 61). Since PPARγ is one of the peripheral targets of TBT, it is possible to speculate that this receptor may be also involved in the central effects related to OTs.
It is important to mention that there is a clear relationship between thyroid function and obesity, with changes in both thyroid-stimulating hormone and thyroxine (T4) associated with changes in body weight and fat mass (62). In spite of few studies investigating the OT effects on the hypothalamic–pituitary–thyroid axis, there is a body of evidences indicating that TBT can also be considered a thyroid disruptor, thus contributing to the development of metabolic disorders and obesity (38, 63–65).
Furthermore, pancreas is a key target organ of metabolic disrupter chemicals not only for controlling glucose metabolism but also for modulating digestion (i.e., releasing digestive enzymes) and food intake (e.g., insulin can modulate hypothalamic center of hunger). In fact, in addition to the endocrine and metabolic changes described above, it is known that OTs affect both exocrine (66) and endocrine functions of the pancreas (20, 67–70). Regarding the later, it is proposed that the impairment in the glucose homeostasis occurs probably due to the ability of OTs to reduce insulin secretion and/or signaling, through inhibition of β cell proliferation, increased apoptosis, and decreased production of NAD(P)H and adenosine triphosphate (ATP) in pancreatic islet cells, associated with local oxidative stress (20, 67–70).
It is well known that the impacts on neuroendocrine control caused by OTs also interfere with reproductive endocrine function: TBT exposure was accountable for morphological changes, such as weight loss of the male and female reproductive organs (30, 31, 71) and abnormal steroidogenesis on gametes (72), as well as reproductive dysfunctions such as changes in ovary morphology and abnormal estrous cyclicity (26, 36, 73–75). Interestingly, Podratz et al. (26) demonstrated that the ingestion of seafood homogenate with imposex indeed provoke important alterations in the rat reproductive organs, strengthening the hypothesis that the ingestion of contaminated shellfish is an important source of exposure to OTs (26).
In female rats, TBT oral administration not only induced estrous cycle and ovary morphological abnormalities (i.e., increased apoptotic cells in the corpus luteum and granulosa cells and increased cystic follicles) but also reduced 17β-estradiol and elevate progesterone serum levels (74, 75). Moreover, studies demonstrate that, depending on the dose, TBT can activate estrogen receptors (ER) in vivo and in vitro having estrogenic and adipogenic activities (3); reduce ER function on metabolic and reproductive controls (20, 26); or even change ER expression in different sites of the hypothalamus–pituitary–gonadal (HPG) axis (75). Actually, the effects of TBT on the gonad function may be, at least in part, due to changes on the HPG axis. Recent studies reported significant alterations in pituitary and hypothalamic morphophysiology and reduced GnRH expression that was related to an impaired Kisspeptin/leptin signaling (22, 75).
Similarly, male adult rats exposed to TBT exhibit varied endocrine damages, including effects on the reproductive endocrine system (30, 71, 76–80). Using different doses, studies with rodents evidenced changes in gonad weight (71, 80), reduced level of luteinizing hormone and testosterone, and spermatogenic disorders associated with reduced Leydig (30, 79) and Sertoli cells (81).
In view of the changes described in the HPG axis and gonads from both genders, an impaired reproductive function should be expected. In fact, several studies have shown that exposure to OTs, in a dose-dependent manner, reduces fertility and embryonic implantation and causes teratogenesis (75, 82–87). Moreover, when administered to pregnant females, TBT-induced weight loss in mothers and their offspring, as well as growth retardation (76, 88). The in utero exposure to TBT also leads to an impaired sexual development by affecting germ cells, which may lead to permanent damage in the adult gonads (77). However, there are evidences that perinatal exposure to OTs in rodents affects differentially male and female pups: while male postnatal development was severely affected with decreased weight of reproductive organs, testosterone level and sperm motility, suggesting that impacts may persist throughout adulthood; female pups exhibited more discreet changes such as initiation of estrous cycling and opening of the vagina occurring at an earlier stage. If considering that the enzyme cytochrome P450 aromatase (P450arom) activity is differentially influenced by OTs in male and female organisms, these studies strengthen the hypothesis of the greater susceptibility of males in the pre- and postnatal periods (72, 89–92).
Taking together, the current literature presents strong evidences of OT-induced endocrine dysfunctions, including significant differences between genders following chronic exposure. This is probably due to the ability of OTs causing not only general toxic effects but also specific molecular and cellular changes, thus altering cell signaling in different ways according to the physiology of each organism exposed.
Major Mechanisms on Metabolic and Endocrine Disrupting Induced by OTs
It is well known that OTs compounds induce their metabolic and endocrine-disrupting effects through interactions with transcriptional regulators such as nuclear and steroid receptors (42). Thus, OTs may affect different nuclear receptor signaling pathways inducing a variety of morphophysiological effects as reviewed herein. For example, as discussed above, OTs exerts obesogenic effect not only by stimulating adipogenesis as agonists of the PPARγ but also by central effects potentially via RXR/PPARγ signaling. Moreover, an equally well-described mechanism is to modulate the expression and/or activity of key enzymes for a number of biochemical processes involved in metabolism and endocrine function.
The synthesis of steroid hormones, for example, involves a number of steps catalyzed by enzymatic reactions that are potential targets for OTs including: (1) cholesterol metabolism, (2) chemical enzymatic conversions, and (3) trafficking of molecules between mitochondria and endoplasmic reticulum (93). Thus, OTs may induce biochemical and endocrine disorders due to this capability of up- or downregulate key enzymes of steroidogenesis (74, 75, 94–96). Studies have described a relationship between endocrine dysfunction induced by OTs and their effects on the enzyme P450arom, which converts androgens into estrogen (94, 96, 97). TBT is reported as a competitive inhibitor of P450arom by reducing its affinity for androstenedione, although this inhibitory effect depends on the exposed tissue, concentration, and time of exposure (94, 96, 97). Conversely, Nakanishi et al. (98) demonstrated that TBT and TPT can increase P450arom activity in a dose- and time-dependent manner in human placental choriocarcinoma cells (98). In addition, in male rats OTs increase P450arom activity and reduce testosterone levels, opposite effects to that found in females (30, 78, 89, 90). Thus, the effects of OTs on the enzymes activity vary not only with tissue or exposed cells, dose, and time of exposure but also according to gender, especially in the case of enzymes related to sex hormones. In animal studies are described an OTs-mediated inhibition of 17-hydroxylase, 3-β-HSD, and 17β-HSD, thus suppressing testosterone biosynthesis (99, 100). Furthermore, studies in human blood and tissue samples also evidenced an inhibitory effect on 5α-reductase 1 and 2, P450arom, 3β-HSD 2, 17β-HSD 1, 11β-HSD1, and 3 (101–103). In a molecular level, Lo et al. (101) suggest there is an interaction of OTs with critical cysteine residues of enzymes leading to disturbances in the steroid hormone levels (101).
It is worth noting that in addition to interaction with nuclear and steroid receptors or specific changes on enzymes involved with steroidogenesis as cited above, the endocrine dysfunction due to OTs exposure can be mediated also by general toxic effects, such as increased oxidative stress and damages to mitochondrial function and subsequent responses to cellular stress (104–107). In this regard, the inhibition of ATP synthesis evidenced by studies with OTs exposure could thereby trigger similar biochemical and/or endocrine dysfunctions (108–111).
Finally, based on studies with cells, tissues and living organisms including mammals exposed to OTs, there are strong evidences of the potential toxicity predisposing to metabolic syndrome complications and endocrine-reproductive disorders, due to changes in all components along the hypothalamus–pituitary axis and peripheral tissues. Notwithstanding, there are changes in different sites including adipose tissue, endocrine glands, neurohormonal, and metabolic control centers, which together can justify the role of OTs as an endocrine and metabolism disruptor in mammals.
Author Contributions
LS and VM idealized the general structure of the text, RF and VM did the literature review, LS, RF, and VM wrote the text, idealized and designed the figures. LS did the final revision of the text.
Conflict of Interest Statement
The authors declare that the research was conducted in the absence of any commercial or financial relationships that could be construed as a potential conflict of interest.
Acknowledgments
The authors acknowledge the continuous support of Conselho Nacional de Desenvolvimento Científico e Tecnológico (CNPq) and Fundação de Amparo à Pesquisa do Espírito Santo (FAPES). In addition, special acknowledgment is given to Sir Charles Gerard Rees, for assistance with language correction and style.
References
1. Airaksinen R, Rantakokko P, Turunen AW, Vartiainen T, Vuorinen PJ, Lappalainen A, et al. Organotin intake through fish consumption in Finland. Environ Res (2010) 110:544–7. doi:10.1016/j.envres.2010.06.004
2. Lee CC, Hsieh CY, Tien CJ. Factors influencing organotin distribution in different marine environmental compartments, and their potential health risk. Chemosphere (2006) 65:547–59. doi:10.1016/j.chemosphere.2006.02.037
3. Penza M, Jeremic M, Marrazzo E, Maggi A, Ciana P, Rando G, et al. The environmental chemical tributyltin chloride (TBT) shows both estrogenic and adipogenic activities in mice which might depend on the exposure dose. Toxicol Appl Pharmacol (2011) 255:65–75. doi:10.1016/j.taap.2011.05.017
4. Rantakokko P, Turunen A, Verkasalo PK, Kiviranta H, Mannisto S, Vartiainen T. Blood levels of organotin compounds and their relation to fish consumption in Finland. Sci Total Environ (2008) 399:90–5. doi:10.1016/j.scitotenv.2008.03.017
5. Hoch M. Organotin compounds in the environment – an overview. Appl Geochem (2001) 16:719–43. doi:10.1016/S0883-2927(00)00067-6
6. Ludgate J. Economic technological impact of TBT legislation on the USA marine industry. Proceedings of the Oceans e An InternationalWorkplace Conference. (Vol. 4), (1987). p. 1309–13.
8. Bryan GW, Gibbs PE, Hummerstone LG, Burt GR. The decline of the gastropod Nucella Lapillus around south-west England: evidence for the effect of tributyltin from antifouling paints. J Marine Biol Assoc U K (1986) 66:611–40. doi:10.1017/S0025315400042247
9. Choi M, Moon H, Yu J, Eom J, Choi H. Butyltin contamination in industrialized bays associated with intensive marine activities in Korea. Arch Environ Contam Toxicol (2009) 57:77–85.
10. Meng PJ, Lin J, Liu LL. Aquatic organotin pollution in Taiwan. J Environ Manage (2009) 90(Suppl 1):S8–15. doi:10.1016/j.jenvman.2008.06.008
11. Kegley SE, Hill BR, Orme S, Choi AH. PAN Pesticide Database. San Francisco, CA: Pesticide Action Network North America (2011).
12. Rudel H. Case study: bioavailability of tin and tin compounds. Ecotoxicol Environ Saf (2003) 56:180–9. doi:10.1016/S0147-6513(03)00061-7
13. Godoi AFL, Favoreto R, Santiago-Silva M. Contaminação ambiental por compostos organoestânicos. Quím Nova (2003) 26:708–16. doi:10.1590/S0100-40422003000500015
14. Fent K. Ecotoxicology of organotin compounds. Crit Rev Toxicol (1996) 26:1–117. doi:10.3109/10408449609089891
16. Oliveira RC, Antelli RE. Occurrence and chemical speciation analysis of organotin compounds in the environment: a review. Talanta (2010) 82:9–24. doi:10.1016/j.talanta.2010.04.046
17. Alzieu C, Michel P, Tolosa I, Bacci E, Mee LD, Readman JW. Organotin com-pounds in the Mediterranean: a continuing cause for concern. Mar Environ (1991) 32:261–70. doi:10.1016/0141-1136(91)90047-C
18. Swennen C, Ruttanadakul N, Ardseungnern S, Singh HR, Mensink BP, Ten Hallers- Tjabbes CC. Imposex in sublittoral and littoral gastropods from the Gulf of Thailand and strait of Malacca in relation to shipping. Environ Tech (1997) 18:1245–54. doi:10.1080/09593331808616646
19. Gadd GM. Microbial interactions with tributyltin compounds: detoxification, accumulation, and environmental fate. Sci Total Environ (2000) 258:119–27. doi:10.1016/S0048-9697(00)00512-X
20. Bertuloso BD, Podratz PL, Merlo E, de Araujo JF, Lima LC, de Miguel EC, et al. Tributyltin chloride leads to adiposity and impairs metabolic functions in the rat liver and pancreas. Toxicol Lett (2015) 235:45–59. doi:10.1016/j.toxlet.2015.03.009
21. Coutinho JV, Freitas-Lima LC, Freitas FF, Freitas FP, Podratz PL, Magnago RP, et al. Tributyltin chloride induces renal dysfunction by inflammation and oxidative stress in female rats. Toxicol Lett (2016) 260:52–69. doi:10.1016/j.toxlet.2016.08.007
22. Merlo E, Podratz PL, Sena GC, de Araujo JF, Lima LC, Alves IS, et al. The environmental pollutant tributyltin chloride disrupts the hypothalamic-pituitary-adrenal axis at different levels in female rats. Endocrinology (2016) 157:2978–95. doi:10.1210/en.2015-1896
23. Krajnc EI, Wester PW, Loeber JG, van Leeuwen FX, Vos JG, Vaessen HA, et al. Toxicity of bis(tri-n-butyltin)oxide in the rat. I. Short-term effects on general parameters and on the endocrine and lymphoid systems. Toxicol Appl Pharmacol (1984) 75:363–86.
24. Wiebkin P, Prough RA, Bridges JW. The metabolism and toxicity of some organotin compounds in isolated rat hepatocytes. Toxicol Appl Pharmacol (1982) 62:409–20. doi:10.1016/0041-008X(82)90142-9
25. Grun F, Watanabe H, Zamanian Z, Maeda L, Arima K, Cubacha R, et al. Endocrine-disrupting organotin compounds are potent inducers of adipogenesis in vertebrates. Mol Endocrinol (2006) 20:2141–55. doi:10.1210/me.2005-0367
26. Podratz PL, Merlo E, Sena GC, Morozesk M, Bonomo MM, Matsumoto ST, et al. Accumulation of organotins in seafood leads to reproductive tract abnormalities in female rats. Reprod Toxicol (2015) 57:29–42. doi:10.1016/j.reprotox.2015.05.003
27. Tabb MM, Blumberg B. New modes of action for endocrine-disrupting chemicals. Mol Endocrinol (2006) 20:475–82. doi:10.1210/me.2004-0513
28. Nakanishi T, Hiromori Y, Yokoyama H, Koyanagi M, Itoh N, Nishikawa J, et al. Organotin compounds enhance 17beta-hydroxysteroid dehydrogenase type I activity in human choriocarcinoma JAr cells: potential promotion of 17beta-estradiol biosynthesis in human placenta. Biochem Pharmacol (2006) 71:1349–57. doi:10.1016/j.bcp.2006.01.014
29. Pagliarani A, Nesci S, Ventrella V. Toxicity of organotin compounds: shared and unshared biochemical targets and mechanisms in animal cells. Toxicol In Vitro (2013) 27:978–90. doi:10.1016/j.tiv.2012.12.002
30. Grote K, Stahlschmidt B, Talsness CE, Gericke C, Appel KE, Chahoud I. Effects of organotin compounds on pubertal male rats. Toxicology (2004) 202:145–58. doi:10.1016/j.tox.2004.05.003
31. Ogata R, Omura M, Shimasaki Y, Kubo K, Oshima Y, Aou S, et al. Two-generation reproductive toxicity study of tributyltin chloride in female rats. J Toxicol Environ Health A (2001) 63:127–44. doi:10.1080/15287390151126469
32. Matthiessen P, Gibbs PE. Critical appraisal of the evidence for tributyltin-mediated endocrine disruption in mollusks. Environ Toxicol Chem (1998) 17:37–43. doi:10.1002/etc.5620170106
33. Oehlmann J, Bauer B, Minchin D, Schulte-Oehlmann U, Fioroni P, Markert B. Imposex in Nucella lapillus and intersex in Littorina littorea: interspecific comparison of two TBT-induced effects and their geographical uniformity. Hydrobiologia (1998) 378:199–213. doi:10.1023/A:1003218411850
34. Grondin M, Marion M, Denizeau F, Averill-Bates DA. Tributyltin induces apoptotic signaling in hepatocytes through pathways involving the endoplasmic reticulum and mitochondria. Toxicol Appl Pharmacol (2007) 222:57–68. doi:10.1016/j.taap.2007.03.028
35. Wada O, Manabe S, Iwai H, Arakawa Y. [Recent progress in the study of analytical methods, toxicity, metabolism and health effects of organotin compounds]. Sangyo Igaku (1982) 24:24–54. doi:10.1539/joh1959.24.24
36. Graceli JB, Sena GC, Lopes PF, Zamprogno GC, da Costa MB, Godoi AF, et al. Organotins: a review of their reproductive toxicity, biochemistry, and environmental fate. Reprod Toxicol (2013) 36:40–52. doi:10.1016/j.reprotox.2012.11.008
37. Vos JG, Dybing E, Greim HA, Ladefoged O, Lambre C, Tarazona JV, et al. Health effects of endocrine-disrupting chemicals on wildlife, with special reference to the European situation. Crit Rev Toxicol (2000) 30:71–133. doi:10.1080/10408440091159176
38. Santos-Silva AP, Andrade MN, Pereira-Rodrigues P, Paiva-Melo FD, Soares P, Graceli JB, et al. Frontiers in endocrine disruption: impacts of organotin on the hypothalamus-pituitary-thyroid axis. Mol Cell Endocrinol (2017) 460:246–57. doi:10.1016/j.mce.2017.07.038
39. Heindel JJ, Vom Saal FS, Blumberg B, Bovolin P, Calamandrei G, Ceresini G, et al. Correction to: Parma consensus statement on metabolic disruptors. Environ Health (2017) 16:130. doi:10.1186/s12940-017-0343-0
40. Heindel JJ, Vom Saal FS, Blumberg B, Bovolin P, Calamandrei G, Ceresini G, et al. Parma consensus statement on metabolic disruptors. Environ Health (2015) 14:54. doi:10.1186/s12940-015-0042-7
41. Grun F. The obesogen tributyltin. Vitam Horm (2014) 94:277–325. doi:10.1016/B978-0-12-800095-3.00011-0
42. Brtko J, Dvorak Z. Triorganotin compounds – ligands for "rexinoid" inducible transcription factors: biological effects. Toxicol Lett (2015) 234:50–8. doi:10.1016/j.toxlet.2015.02.009
43. Kanayama T, Kobayashi N, Mamiya S, Nakanishi T, Nishikawa J. Organotin compounds promote adipocyte differentiation as agonists of the peroxisome proliferator-activated receptor gamma/retinoid X receptor pathway. Mol Pharmacol (2005) 67:766–74. doi:10.1124/mol.104.008409
44. Yanik SC, Baker AH, Mann KK, Schlezinger JJ. Organotins are potent activators of PPARgamma and adipocyte differentiation in bone marrow multipotent mesenchymal stromal cells. Toxicol Sci (2011) 122:476–88. doi:10.1093/toxsci/kfr140
45. Nakanishi T. Endocrine disruption induced by organotin compounds; organotins function as a powerful agonist for nuclear receptors rather than an aromatase inhibitor. J Toxicol Sci (2008) 33:269–76. doi:10.2131/jts.33.269
46. Bo E, Viglietti-Panzica C, Panzica GC. Acute exposure to tributyltin induces c-fos activation in the hypothalamic arcuate nucleus of adult male mice. Neurotoxicology (2011) 32:277–80. doi:10.1016/j.neuro.2010.12.011
47. He K, Zhang J, Chen Z. Effect of tributyltin on the food intake and brain neuropeptide expression in rats. Endokrynol Pol (2014) 65:485–90. doi:10.5603/EP.2014.0068
48. Elsabbagh HS, Moussa SZ, El-tawil OS. Neurotoxicologic sequelae of tributyltin intoxication in rats. Pharmacol Res (2002) 45:201–6. doi:10.1006/phrs.2001.0909
49. Nakatsu Y, Kotake Y, Takishita T, Ohta S. Long-term exposure to endogenous levels of tributyltin decreases GluR2 expression and increases neuronal vulnerability to glutamate. Toxicol Appl Pharmacol (2009) 240:292–8. doi:10.1016/j.taap.2009.06.024
50. Mitra S, Gera R, Siddiqui WA, Khandelwal S. Tributyltin induces oxidative damage, inflammation and apoptosis via disturbance in blood-brain barrier and metal homeostasis in cerebral cortex of rat brain: an in vivo and in vitro study. Toxicology (2013) 310:39–52. doi:10.1016/j.tox.2013.05.011
51. Mitra S, Siddiqui WA, Khandelwal S. Early cellular responses against tributyltin chloride exposure in primary cultures derived from various brain regions. Environ Toxicol Pharmacol (2014) 37:1048–59. doi:10.1016/j.etap.2014.03.020
52. Bo E, Farinetti A, Marraudino M, Sterchele D, Eva C, Gotti S, et al. Adult exposure to tributyltin affects hypothalamic neuropeptide Y, Y1 receptor distribution, and circulating leptin in mice. Andrology (2016) 4:723–34. doi:10.1111/andr.12222
53. Robertson SA, Leinninger GM, Myers MG Jr. Molecular and neural mediators of leptin action. Physiol Behav (2008) 94:637–42. doi:10.1016/j.physbeh.2008.04.005
54. Kirchner S, Kieu T, Chow C, Casey S, Blumberg B. Prenatal exposure to the environmental obesogen tributyltin predisposes multipotent stem cells to become adipocytes. Mol Endocrinol (2010) 24:526–39. doi:10.1210/me.2009-0261
55. Ouadah-Boussouf N, Babin PJ. Pharmacological evaluation of the mechanisms involved in increased adiposity in zebrafish triggered by the environmental contaminant tributyltin. Toxicol Appl Pharmacol (2016) 294:32–42. doi:10.1016/j.taap.2016.01.014
56. Cui H, Okuhira K, Ohoka N, Naito M, Kagechika H, Hirose A, et al. Tributyltin chloride induces ABCA1 expression and apolipoprotein A-I-mediated cellular cholesterol efflux by activating LXRalpha/RXR. Biochem Pharmacol (2011) 81:819–24. doi:10.1016/j.bcp.2010.12.023
57. Le MA, Grimaldi M, Roecklin D, Dagnino S, Vivat-Hannah V, Balaguer P, et al. Activation of RXR-PPAR heterodimers by organotin environmental endocrine disruptors. EMBO Rep (2009) 10:367–73. doi:10.1038/embor.2009.8
58. Li X, Ycaza J, Blumberg B. The environmental obesogen tributyltin chloride acts via peroxisome proliferator activated receptor gamma to induce adipogenesis in murine 3T3-L1 preadipocytes. J Steroid Biochem Mol Biol (2011) 127:9–15. doi:10.1016/j.jsbmb.2011.03.012
59. Regnier SM, El-Hashani E, Kamau W, Zhang X, Massad NL, Sargis RM. Tributyltin differentially promotes development of a phenotypically distinct adipocyte. Obesity (Silver Spring) (2015) 23:1864–71. doi:10.1002/oby.21174
60. Garretson JT, Teubner BJ, Grove KL, Vazdarjanova A, Ryu V, Bartness TJ. Peroxisome proliferator-activated receptor gamma controls ingestive behavior, agouti-related protein, and neuropeptide Y mRNA in the arcuate hypothalamus. J Neurosci (2015) 35:4571–81. doi:10.1523/JNEUROSCI.2129-14.2015
61. Sarruf DA, Yu F, Nguyen HT, Williams DL, Printz RL, Niswender KD, et al. Expression of peroxisome proliferator-activated receptor-gamma in key neuronal subsets regulating glucose metabolism and energy homeostasis. Endocrinology (2009) 150:707–12. doi:10.1210/en.2008-0899
62. Laurberg P, Knudsen N, Andersen S, Carle A, Pedersen IB, Karmisholt J. Thyroid function and obesity. Eur Thyroid J (2012) 1:159–67. doi:10.1159/000342994
63. Sharan S, Nikhil K, Roy P. Disruption of thyroid hormone functions by low dose exposure of tributyltin: an in vitro and in vivo approach. Gen Comp Endocrinol (2014) 206:155–65. doi:10.1016/j.ygcen.2014.07.027
64. Decherf S, Demeneix BA. The obesogen hypothesis: a shift of focus from the periphery to the hypothalamus. J Toxicol Environ Health B Crit Rev (2011) 14:423–48. doi:10.1080/10937404.2011.578561
65. Decherf S, Seugnet I, Fini JB, Clerget-Froidevaux MS, Demeneix BA. Disruption of thyroid hormone-dependent hypothalamic set-points by environmental contaminants. Mol Cell Endocrinol (2010) 323:172–82. doi:10.1016/j.mce.2010.04.010
66. Hara K, Yoshizuka M, Fujimoto S. Toxic effects of bis (tributyltin) oxide on the synthesis and secretion of zymogen granules in the rat exocrine pancreas. Arch Histol Cytol (1994) 57:201–12. doi:10.1679/aohc.57.201
67. Ogino K, Inukai T, Miura Y, Matsui H, Takemura Y. Triphenyltin chloride induces glucose intolerance by the suppression of insulin release from hamster pancreatic beta-cells. Exp Clin Endocrinol Diabetes (1996) 104:409–11. doi:10.1055/s-0029-1211476
68. Zuo Z, Wu T, Lin M, Zhang S, Yan F, Yang Z, et al. Chronic exposure to tributyltin chloride induces pancreatic islet cell apoptosis and disrupts glucose homeostasis in male mice. Environ Sci Technol (2014) 48:5179–86. doi:10.1021/es404729p
69. Miura Y, Hori Y, Kimura S, Hachiya H, Sakurai Y, Inoue K, et al. Triphenyltin impairs insulin secretion by decreasing glucose-induced NADP(H) and ATP production in hamster pancreatic beta-cells. Toxicology (2012) 299:165–71. doi:10.1016/j.tox.2012.05.021
70. Chen YW, Lan KC, Tsai JR, Weng TI, Yang CY, Liu SH. Tributyltin exposure at noncytotoxic doses dysregulates pancreatic beta-cell function in vitro and in vivo. Arch Toxicol (2017) 91:3135–44. doi:10.1007/s00204-017-1940-y
71. Omura M, Ogata R, Kubo K, Shimasaki Y, Aou S, Oshima Y, et al. Two-generation reproductive toxicity study of tributyltin chloride in male rats. Toxicol Sci (2001) 64:224–32. doi:10.1093/toxsci/64.2.224
72. Si J, Li P, Xin Q, Li X, An L, Li J. Perinatal exposure to low doses of tributyltin chloride reduces sperm count and quality in mice. Environ Toxicol (2015) 30:44–52. doi:10.1002/tox.21892
73. Delgado Filho VS, Lopes PF, Podratz PL, Graceli JB. Triorganotin as a compound with potential reproductive toxicity in mammals. Braz J Med Biol Res (2011) 44:958–65. doi:10.1590/S0100-879X2011007500110
74. Lang PP, Delgado Filho VS, Lopes PF, Cavati SG, Matsumoto ST, Samoto VY, et al. Tributyltin impairs the reproductive cycle in female rats. J Toxicol Environ Health A (2012) 75:1035–46. doi:10.1080/15287394.2012.697826
75. Sena GC, Freitas-Lima LC, Merlo E, Podratz PL, de Araujo JF, Brandao PA, et al. Environmental obesogen tributyltin chloride leads to abnormal hypothalamic-pituitary-gonadal axis function by disruption in kisspeptin/leptin signaling in female rats. Toxicol Appl Pharmacol (2017) 319:22–38. doi:10.1016/j.taap.2017.01.021
76. Adeeko A, Li D, Forsyth DS, Casey V, Cooke GM, Barthelemy J, et al. Effects of in utero tributyltin chloride exposure in the rat on pregnancy outcome. Toxicol Sci (2003) 74:407–15. doi:10.1093/toxsci/kfg131
77. Kishta O, Adeeko A, Li D, Luu T, Brawer JR, Morales C, et al. In utero exposure to tributyltin chloride differentially alters male and female fetal gonad morphology and gene expression profiles in the Sprague-Dawley rat. Reprod Toxicol (2007) 23:1–11. doi:10.1016/j.reprotox.2006.08.014
78. Wang BA, Li M, Mu YM, Lu ZH, Li JY. [Effects of tributyltin chloride (TBT) and triphenyltin chloride (TPT) on rat testicular Leydig cells]. Zhonghua Nan Ke Xue (2006) 12:516–9.
79. Yu WJ, Lee BJ, Nam SY, Kim YC, Lee YS, Yun YW. Spermatogenetic disorders in adult rats exposed to tributyltin chloride during puberty. J Vet Med Sci (2003) 65:1331–5. doi:10.1292/jvms.65.1331
80. Yu WJ, Nam SY, Kim YC, Lee BJ, Yun YW. Effects of tributyltin chloride on the reproductive system in pubertal male rats. J Vet Sci (2003) 4:29–34.
81. Mitra S, Srivastava A, Khandelwal S. Tributyltin chloride induced testicular toxicity by JNK and p38 activation, redox imbalance and cell death in sertoli-germ cell co-culture. Toxicology (2013) 314:39–50. doi:10.1016/j.tox.2013.09.003
82. Ema M, Miyawaki E, Kawashima K. Suppression of uterine decidualization as a cause of implantation failure induced by triphenyltin chloride in rats. Arch Toxicol (1999) 73:175–9. doi:10.1007/s002040050603
83. Ema M, Harazono A, Miyawaki E, Ogawa Y. Effect of the day of administration on the developmental toxicity of tributyltin chloride in rats. Arch Environ Contam Toxicol (1997) 33:90–6. doi:10.1007/s002449900228
84. Ema M, Miyawaki E. Suppression of uterine decidualization correlated with reduction in serum progesterone levels as a cause of preimplantation embryonic loss induced by diphenyltin in rats. Reprod Toxicol (2002) 16:309–17. doi:10.1016/S0890-6238(02)00018-7
85. Harazono A, Ema M, Ogawa Y. Pre-implantation embryonic loss induced by tributyltin chloride in rats. Toxicol Lett (1996) 89:185–90. doi:10.1016/S0378-4274(96)03805-2
86. Itami T, Ema M, Amano H, Murai T, Kawasaki H. Teratogenic evaluation of tributyltin chloride in rats following oral exposure. Drug Chem Toxicol (1990) 13:283–95. doi:10.3109/01480549009032287
87. Sarpa M, Tavares Lopes CM, Delgado IF, Paumgartten FJ. Postnatal development and fertility of offspring from mice exposed to triphenyltin (fentin) hydroxide during pregnancy and lactation. J Toxicol Environ Health A (2010) 73:965–71. doi:10.1080/15287391003751752
88. Makita Y, Omura M, Tanaka A, Kiyohara C. Effects of concurrent exposure to tributyltin and 1,1-dichloro-2,2 bis (p-chlorophenyl) ethylene (p,p’-DDE) on immature male Wistar rats. Basic Clin Pharmacol Toxicol (2005) 97:364–8. doi:10.1111/j.1742-7843.2005.pto_199.x
89. Grote K, Hobler C, Andrade AJ, Grande SW, Gericke C, Talsness CE, et al. Sex differences in effects on sexual development in rat offspring after pre- and postnatal exposure to triphenyltin chloride. Toxicology (2009) 260:53–9. doi:10.1016/j.tox.2009.03.006
90. Hobler C, Andrade AJ, Grande SW, Gericke C, Talsness CE, Appel KE, et al. Sex-dependent aromatase activity in rat offspring after pre- and postnatal exposure to triphenyltin chloride. Toxicology (2010) 276:198–205. doi:10.1016/j.tox.2010.08.003
91. American Diabetes Association. Diagnosis and classification of diabetes mellitus. Diabetes Care (2006) 29(Suppl 1):S43–8. doi:10.2337/dc10-S062
92. Si J, Han X, Zhang F, Xin Q, An L, Li G, et al. Perinatal exposure to low doses of tributyltin chloride advances puberty and affects patterns of estrous cyclicity in female mice. Environ Toxicol (2012) 27:662–70. doi:10.1002/tox.21756
93. Whitehead SA, Rice S. Endocrine-disrupting chemicals as modulators of sex steroid synthesis. Best Pract Res Clin Endocrinol Metab (2006) 20:45–61. doi:10.1016/j.beem.2005.09.003
94. Cooke GM. Effect of organotins on human aromatase activity in vitro. Toxicol Lett (2002) 126:121–30. doi:10.1016/S0378-4274(01)00451-9
95. Grote K, Andrade AJ, Grande SW, Kuriyama SN, Talsness CE, Appel KE, et al. Effects of peripubertal exposure to triphenyltin on female sexual development of the rat. Toxicology (2006) 222:17–24. doi:10.1016/j.tox.2006.01.008
96. Saitoh M, Yanase T, Morinaga H, Tanabe M, Mu YM, Nishi Y, et al. Tributyltin or triphenyltin inhibits aromatase activity in the human granulosa-like tumor cell line KGN. Biochem Biophys Res Commun (2001) 289:198–204. doi:10.1006/bbrc.2001.5952
97. Heidrich DD, Steckelbroeck S, Klingmuller D. Inhibition of human cytochrome P450 aromatase activity by butyltins. Steroids (2001) 66:763–9. doi:10.1016/S0039-128X(01)00108-8
98. Nakanishi T, Kohroki J, Suzuki S, Ishizaki J, Hiromori Y, Takasuga S, et al. Trialkyltin compounds enhance human CG secretion and aromatase activity in human placental choriocarcinoma cells. J Clin Endocrinol Metab (2002) 87:2830–7. doi:10.1210/jcem.87.6.8540
99. McVey MJ, Cooke GM. Inhibition of rat testis microsomal 3beta-hydroxysteroid dehydrogenase activity by tributyltin. J Steroid Biochem Mol Biol (2003) 86:99–105. doi:10.1016/S0960-0760(03)00256-5
100. Ohno S, Nakajima Y, Nakajin S. Triphenyltin and tributyltin inhibit pig testicular 17beta-hydroxysteroid dehydrogenase activity and suppress testicular testosterone biosynthesis. Steroids (2005) 70:645–51. doi:10.1016/j.steroids.2005.03.005
101. Lo S, Allera A, Albers P, Heimbrecht J, Jantzen E, Klingmuller D, et al. Dithioerythritol (DTE) prevents inhibitory effects of triphenyltin (TPT) on the key enzymes of the human sex steroid hormone metabolism. J Steroid Biochem Mol Biol (2003) 84:569–76. doi:10.1016/S0960-0760(03)00074-8
102. Ohshima M, Ohno S, Nakajin S. Inhibitory effects of some possible endocrine-disrupting chemicals on the isozymes of human 11beta-hydroxysteroid dehydrogenase and expression of their mRNA in gonads and adrenal glands. Environ Sci (2005) 12:219–30.
103. Doering DD, Steckelbroeck S, Doering T, Klingmuller D. Effects of butyltins on human 5alpha-reductase type 1 and type 2 activity. Steroids (2002) 67:859–67. doi:10.1016/S0039-128X(02)00051-X
104. Nishikimi A, Kira Y, Kasahara E, Sato EF, Kanno T, Utsumi K, et al. Tributyltin interacts with mitochondria and induces cytochrome c release. Biochem J (2001) 356:621–6. doi:10.1042/bj3560621
105. Powers MF, Beavis AD. Triorganotins inhibit the mitochondrial inner membrane anion channel. J Biol Chem (1991) 266:17250–6.
106. Gennari A, Viviani B, Galli CL, Marinovich M, Pieters R, Corsini E. Organotins induce apoptosis by disturbance of [Ca(2+)](i) and mitochondrial activity, causing oxidative stress and activation of caspases in rat thymocytes. Toxicol Appl Pharmacol (2000) 169:185–90. doi:10.1006/taap.2000.9076
107. Yamada S, Kotake Y, Nakano M, Sekino Y, Kanda Y. Tributyltin induces mitochondrial fission through NAD-IDH dependent mitofusin degradation in human embryonic carcinoma cells. Metallomics (2015) 7:1240–6. doi:10.1039/c5mt00033e
108. Aldridge WN. The biochemistry of organotin compounds: trialkyltins and oxidative phosphorylation. Biochem J (1958) 69:367–76. doi:10.1042/bj0690481b
109. Cain K, Partis MD, Griffiths DE. Dibutylchloromethyltin chloride, a covalent inhibitor of the adenosine triphosphate synthase complex. Biochem J (1977) 166:593–602. doi:10.1042/bj1660593
110. Matsuno-Yagi A, Hatefi Y. Studies on the mechanism of oxidative phosphorylation. ATP synthesis by submitochondrial particles inhibited at F0 by venturicidin and organotin compounds. J Biol Chem (1993) 268:6168–73.
Keywords: tributyltin, triphenyltin, impossex, endocrine disruptor, obesogen, metabolic disrupting chemicals, hypothalamus–pituitary axis
Citation: Marques VB, Faria RA and Dos Santos L (2018) Overview of the Pathophysiological Implications of Organotins on the Endocrine System. Front. Endocrinol. 9:101. doi: 10.3389/fendo.2018.00101
Received: 15 December 2017; Accepted: 01 March 2018;
Published: 16 March 2018
Edited by:
Leandro Miranda-Alves, Universidade Federal do Rio de Janeiro, BrazilReviewed by:
Giancarlo Panzica, Università degli Studi di Torino, ItalyRen-Shan Ge, Wenzhou Medical University, China
Copyright: © 2018 Marques, Faria and Dos Santos. This is an open-access article distributed under the terms of the Creative Commons Attribution License (CC BY). The use, distribution or reproduction in other forums is permitted, provided the original author(s) and the copyright owner are credited and that the original publication in this journal is cited, in accordance with accepted academic practice. No use, distribution or reproduction is permitted which does not comply with these terms.
*Correspondence: Leonardo Dos Santos, bGVvZG9zc2FudG9zQGhvdG1haWwuY29t