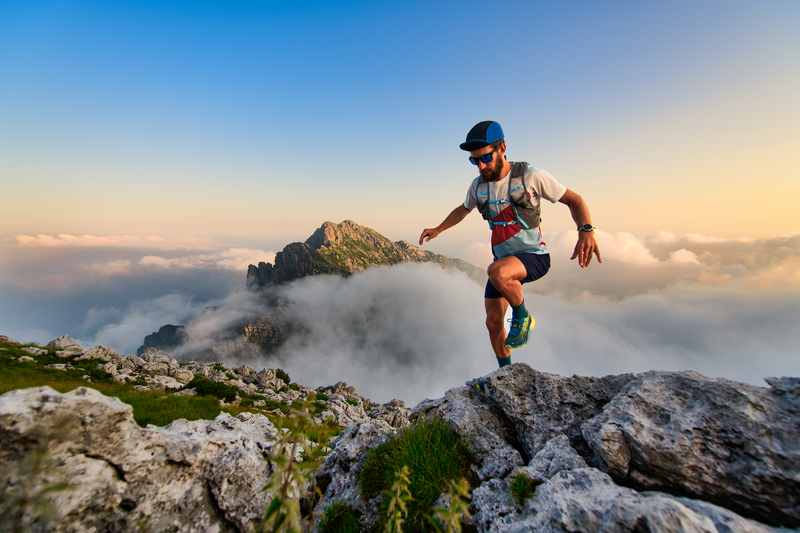
95% of researchers rate our articles as excellent or good
Learn more about the work of our research integrity team to safeguard the quality of each article we publish.
Find out more
REVIEW article
Front. Endocrinol. , 04 December 2017
Sec. Cancer Endocrinology
Volume 8 - 2017 | https://doi.org/10.3389/fendo.2017.00341
This article is part of the Research Topic Cell Signaling in Tumors of Endocrine and Endocrine-responsive Tissues View all 6 articles
The molecular events leading to gastroenteropancreatic neuroendocrine tumor (GEP-NET) formation are largely unknown. Over the past decades, systemic chemotherapies have been replaced by therapies directed at particular molecular targets such as the somatostatin receptors, mTOR complexes or proangiogenic molecules. These approaches have demonstrated some success in subtypes of this heterogeneous tumor group, but responses are still widely varied. This review highlights the clinical trials ongoing for neuroendocrine tumors (NETs) and includes emerging immunotherapy, which holds great promise for NETs based on successes in other tumor types. Current avenues of preclinical research, including Notch and PI3K/AKT, will lead to additional targeted therapies based on genome-wide studies that have cast a wide net in the search for driver mutations. Future preclinical and clinical investigations are required to identify those mutations predictive of therapeutic response or disease progression. Results of current clinical trials outlined here will better inform patient management with respect to agent selection, timing, duration and combination therapy in the treatment of NETs.
Gastroenteropancreatic neuroendocrine tumors (GEP-NETs) are clinically and biologically heterogeneous, resulting in widely varied clinical outcomes and challenges when establishing guidelines for tumor characterization and patient management. Over the past decade, significant effort has been expended to standardize the classification system used for these tumors in the clinical setting (1, 2). In 2010, guidelines published by the World Health Organization (WHO) recommended categorization of GEP-NETs based on clinical behavior, histopathological features and proliferation rate (3). These guidelines defined “neuroendocrine” as neoplastic cells that express markers of neural lineage (synaptophysin and chromogranin A). The term “neuroendocrine neoplasm (NEN)” was adopted which includes all tumors or carcinoma that derives from neuroendocrine cells. This led to the establishment of three morphologically distinct groups: (1) well-differentiated NENs, (2) poorly differentiated NENs [also called neuroendocrine carcinoma (NECs)], and (3) mixed adenoneurocarcinoma (MANECs). Well-differentiated NENs were further subdivided based on proliferative activity into either G1 (≤2% Ki67 index and mitoses <2/10 high-power field) or G2 (3–20% Ki67 index and mitoses 2–20/10 high-power field). All poorly differentiated NECs are G3 (>20% Ki67 index and >20/10 high-power field). MANECs were defined as having >30% of each component in the tumor and were defined as NEC G3 containing non-neuroendocrine components (typically adenocarcinoma) (3).
In recent months, the WHO2017 grading system was published which modifies the original WHO2010 classification for pancreatic NETs in several important ways (4). First, clinical practice noted a group of tumors that was discordant with their grade based on established criteria—meaning tumors that exhibited Ki67 indices of >20% even though they were well differentiated. Despite this discordance, these tumors were often classified as G3, yet behaved clinically as well-differentiated G1/2 tumors in that they had poor responses to platinum-based chemotherapy, surgery resulted in favorable outcomes, and these tumors responded well to somatostatin analogs, mTOR inhibitors, and alkylating agents such as temozolomide (5–7). This resulted in the generation of a new category called panNET G3 to account for these tumors. Second, the Ki67 index cutoff value was raised to 3% instead of 2% for pNET G1/2 distinction. Third, the MANEC classification was too narrow in that it assumed that the non-neuroendocrine component was glandular in nature, when in fact it can also be well-differentiated tumor such as pNET G1/2. Therefore, the term “mixed neuroendocrine non-neuroendocrine neoplasm” (MiNEN) was adopted (8). The WHO2017 grading recommendations are found in Table 1.
Staging for NETs has undergone repeated revision by groups in the US and Europe in attempt to gain consensus within the field and to accurately develop informative, prognostic, and biological classifications to aid in patient management, study comparisons, and to direct future clinical trial design (9, 10). A recent study of pancreatic NETs proposed amended guidelines that are a fusion of two of the most widely used staging criteria: the American Joint Commission on Cancer (AJCC; primarily used in North America) and the European Neuroendocrine Tumor Society (ENETS; primarily used in Europe) (11). This study addressed shortcomings in both systems and proposed a modified version, mENETs that uses the standard TNM classification from ENETS while incorporating the AJCC staging definitions (11). This mENETs system was tested on data from the SEER database and found to be more appropriate than either system alone for pancreatic NETs (11). In addition, guidelines for the surgical management of small bowel NETs were released recently by the North American Neuroendocrine Tumor Society (NANETS) (12), as were guidelines for medical management and follow-up surveillance of patients with GEP-NETs (13, 14).
The incidence of GEP-NETs is 2–5 cases per 100,000 and this number is expected to continue rising primarily due to improvements in diagnostic imaging and physician awareness (1, 15–17). Patients with GEP-NETs often present with advanced disease at diagnosis (18). Surgical resection is often the first option either for curative (for localized, non-metastatic disease) or palliative (for advanced metastatic disease) intent, followed by pathway-based, systemic chemotherapies such as those outlined below for patients with metastatic disease.
SSAs (octreotide, lanreotide, pasireotide) were initially developed to mimic the inhibitory action of somatostatin on cell surface G-protein-coupled receptors called SSTR1–5, which mediate downstream hormone release and cell growth via PI3K and MAPK signaling (19). These drugs are used successfully to control symptoms of the carcinoid syndrome that is clinically characterized by flushing, diarrhea and right-side heart valve disease as a result of hypersecretion of bioactive amines from small intestinal NETs (20). The ELECT trial (NCT00774930), a phase III double-blind study of lanreotide depot as a therapy for carcinoid syndrome, enrolled 115 patients—59 in the lanreotide depot arm and 56 in the placebo arm. Enrolled patients were randomized and given access to short-acting octreotide as a rescue medication, with the primary study endpoint being the utilization of the rescue medication and self-reported frequency of diarrhea and/or flushing episodes. The percentage of days using rescue medication was significantly decreased in the lanreotide depot group (33.7%, 95% CI 25–42.4) compared to placebo (48.5%, 95% CI 39.6–57.4) (21) indicating increased control of carcinoid syndrome with lanreotide.
SSA therapy was also used in a trial of patients with Hashimoto’s thyroiditis and enterochromaffin-like hyperplasia (22). Chronic atrophic gastritis is an autoimmune attack on the parietal cells that results in increased gastrin production. Chronic atrophic gastritis is also a risk factor for gastric endocrine carcinoma, especially when correlated with enterochromaffin-like cell hyperplasia. One study evaluated the prevalence of chronic atrophic gastritis, hypergastrinemia, and enterochromaffin-like cell hyperplasia in patients with Hashimoto’s. Treatment with SSAs caused regression of enterochromaffin-like cell hyperplasia in all patients suggesting that the early diagnosis of enterochromaffin-like cell hyperplasia, and the treatment with SSAs, may play a role in prevention of gastric endocrine carcinoma. However, it should be noted that one patient in this study with a type-1 gastric carcinoid at study start did not have significant tumor regression in response to SSA (22).
In clinical trials focused on NEN regression/control, SSAs have demonstrated efficacy in treatment arms compared to placebo controls. The phase 3 PROMID trial (NCT00171873) examined octreotide LAR (n = 42) compared to placebo (n = 43) in treatment-naïve, well-differentiated, metastatic midgut (mostly GI) NENs with time to tumor progression (TTP) as the primary endpoint. Octreotide LAR significantly lengthened TTP by 8.3 months over placebo [14.3 months TTP for octreotide LAR, 6 months in placebo (HR = 0.34; 95% CI 0.2–0.59; p = 0.000072)] (23). Long-term follow-up of these patients did not show a demonstrable increase in overall survival (OS) (24). Patients on placebo were offered octreotide LAR at study completion and the crossover design of this study may have confounded the data interpretation with respect to OS (23, 24). The CLARINET trial (NCT00353496) included lanreotide depot (n = 101) compared to placebo (n = 103) in metastatic, non-functioning, low-grade (G1 or G2) pancreatic and intestinal NETs with stable disease (25, 26). This trial resulted in significantly prolonged PFS in the lanreotide depot group compared to placebo (median not reached versus 18 months; HR = 0.47; 95% CI 0.3–0.73; p < 0.001). As in the PROMID trial, this improvement in PFS did not translate to increases in OS at 2 years (25). The CLARINET trial was continued for an additional 40 months with an open label extension that further confirmed the favorable safety and tolerability profile of lanreotide depot with long-term use (26). The ongoing phase 2 CLARINET FORTE study (NCT0265987) is investigating a reduced dosing interval (14 versus 28 days) for lanreotide depot in patients with well-differentiated pancreatic or midgut NENs with the primary endpoint of PFS.
Peptide receptor radionuclide therapy (PRRT) has tremendous potential for the treatment of NETs with elevated expression of SSTRs or other cell surface receptors. This therapy directs radionuclides directly to cancer cells by targeting the SSTRs expressed on their surface (27). It should be noted that SSAs have varying affinities for the different SSTR receptors and may be a compounding factor when comparing clinical trials involving different SSAs (28, 29). The NETTER-1 trial (NCT01578239), measured PFS in patients treated with octreotide LAR with and without 177Lu-Dotatate as a localized anticancer radiotherapy for advanced, SSTR-positive metastatic midgut (primarily jejuno, ileum, and proximal colon) NETs. In a preliminary analysis published recently, treatment with 177Lu-Dotatate plus octreotide LAR (n = 111) resulted in markedly increased PFS compared to the octreotide LAR (n = 110) alone (30). At the time of the planned interim analysis, the median PFS had not been reached for the 177Lu-Dotatate plus octreotide LAR group and was 8.4 months for the control group (95% CI 5.8–9.1). The estimated rate of PFS at month 20 was 65.2% (95% CI 50–76.8%) in the 177Lu-Dotatate plus octreotide LAR group versus 10.8% (95% CI 3.5–23) in control. PRRT has also been studied in combination with standard chemotherapeutic agents in NETs. For example, small published studies of 177Lu-dotatate in combination with capecitabine to radiosensitize in metastatic GEP-NETs, demonstrated safety and efficacy (31, 32). Other studies examined the combination of 177Lu-octreotate with capecitabine and temozolomide in advanced, low-grade NETs (n = 30), demonstrating an overall response rate of 80% (95% CI 66–93), with complete remission in 13% (95% CI 4–30) and partial response in 70% (95% CI 52–83) (33, 34). Among the many phase II trials in progress to study PRRT in NETs, the CONTROL NETS trial (NCT02358356) is testing the combination therapy of 177Lu-octreotate + capecitabine + temozolomide is compared with capecitabine + temozolomide in low- to intermediate-grade (G1/G2) pancreatic NENs and versus PRRT alone in low- to intermediate-grade midgut NENs. The LUNET trial is recruiting 98 patients for a non-comparison trial of two separate doses of 177Lu-Dotatate in advanced enteropancreatic NETs (NCT02489604), and the LUCAS trial (NCT02736448) is a randomized trial seeking to enroll 176 patients to compare 177Lu-PRRT + capecitabine versus 177Lu-PRRT alone in well-differentiated GEP-NENs. The PRELUDE trial (NCT02788578) is a retrospective study seeking to describe the effects on PFS of combination Lanreotide depot with PRRT in metastatic well-differentiated (G1 or G2) GEP- and bronchopulmonary NETs. There are many other PRRT trials in NETs currently recruiting (clinicaltrials.gov) and these trials are just the beginning of studies to understand combination interventions in NETs. It is unknown whether the timing and sequence of therapies is important, i.e., simultaneous versus sequential, direct effect versus radiosensitizing, octreotide versus pasireotide versus lanreotide (efficacy depends on the SSTR distribution present in tumor, if any), and many of the current clinical trials will yield informative results in coming years. Guidelines for therapy selection, timing, and combination may also be further clarified as we understand more about the genetics of the different subtypes and grades of NETs. Table 2 outlines the current phase III clinical trials for GEP-NETs.
Table 2. Current Phase III clinical trials for gastroenteropancreatic neuroendocrine tumors (GEP-NETs).
In addition to SSTR, other peptide receptor targets have also been identified and utilized for in vivo scintigraphy and targeted radiotherapy. Incretin receptors, including glucagon-like peptide-1 (GLP-1R) (35, 36) and glucose-dependent insulinotropic polypeptide receptor (GIPR) (37, 38), have been investigated with earnest in recent years, with putative utility in tumors such as insulinoma that often occur with low or absent expression of SSTR. Interestingly, in studies of SSTR-negative gastrointestinal or bronchial NETs, more than 88% were positive for GIPR (39). Recent studies from the Maecke lab (40) investigate a set of GIP-derived ligands for their ability to image a broad spectrum of GIPR-expressing NETs with positive results. Whereas biodistribution studies in mouse xenograft models indicate accumulation of these peptides in the kidney which could result in nephrotoxicity upon translation to clinical use, optimization of GIP-derived peptides demonstrates great potential for both imaging and PRRT in GIPR-positive NETs.
The mammalian target of rapamycin (mTOR) pathway was initially studied in NETs as a part of familial tumor syndromes known to have genetic mutations in genes upstream of the mTOR complexes. For example, the autosomal-dominant syndromes neurofibromatosis type 1 (NF1) and tuberous sclerosis (TS) are caused by inactivating mutations in NF-1 and TSC1/2, respectively. Patients with NF1 develop a spectrum of NETs in the ampulla of Vater, duodenum, and mediastinum, and demonstrate constitutive activation of the mTOR signaling pathway as a result of NF1 loss (41). TS has been associated with pancreatic NETs, and the loss of the TSC1/2 genes similarly leads to activation of mTOR signaling (42). Clinical genetic studies have identified that gastrointestinal (small intestine) NETs can have somatic mutations in MEN1, CDKN1B, and other genes involved in the PI3K/AKT/mTOR signaling pathway (43–45) and more recent whole-exome sequencing data have identified that 14% of pancreatic neuroendocrine tumors (NETs) have mutations in genes of the mTOR signaling pathway (46).
Mammalian target of rapamycin functions as an intracellular serine/threonine kinase that modulates key cellular processes such as nutrient sensing, proliferation, metabolism, and cell survival. mTOR exists as one of two known complexes, mTORC1 or mTORC2, in conjunction with several other proteins (47). Inhibitors of mTOR (rapamycin, everolimus/RAD001, temsirolimus) bind to the FK506 binding protein, which then binds to mTORC1 and inhibits pathway signaling. Everolimus has been investigated in NETs through the RADIANT series of trials. The RADIANT-1 trial was an open-label, phase 2 study that initially investigated everolimus in patients with advanced, low- to intermediate-grade GEP-NENs and included a small number of patients with carcinoids (n = 30) and islet cell tumors (n = 30). Patients received everolimus either alone or in combination with octreotide at the discretion of the study investigators and resulted in promising antitumor activity that was well tolerated (48). Octreotide is known to reduce serum IGF-1 levels, a pathway upstream of the mTOR pathway, so the combination of everolimus plus octreotide was considered a two-pronged approach to accomplish both upstream and downstream inhibition of mTOR signaling. Patients receiving the combination therapy had median PFS of 60 weeks (95% CI 54–66), compared to 50 weeks with 5 mg everolimus alone (95% CI 23–78) and 72 weeks with 10 mg everolimus alone (95% CI 60–83). However, this study was not powered nor designed to make the comparison between these study groups. RADIANT-1 was followed up with a larger study, the RADIANT-2 trial (NCT00412061) which was a randomized, double-blind, placebo-controlled phase III study (49). Patients with low- or intermediate-grade NENs (the majority small intestinal primary site) were treated with everolimus plus octreotide LAR (n = 216), or placebo plus octreotide LAR (n = 213). Everolimus plus octreotide LAR improved PFS by 5.1 months over the everolimus plus placebo group (16.4 months, 95% CI 13.7–21.2 versus 11.3, 95% CI 8.4–14.6), but did not meet statistical significance. Treatment with everolimus offered patients a 23% decrease in relative risk of progression (HR 0.77; p = 0.026) compared to placebo (49). The recently completed COOPERATE-2 trial, a randomized, open-label phase II study had similar results (50). Patients with advanced, well-differentiated progressive pancreatic NETs were treated with either everolimus alone (n = 81) or in combination with pasireotide (n = 79) and evaluated on the primary endpoint of PFS. The everolimus alone group had a median PFS of 16.6 months, while the combination group had median PFS of 16.8 months (HR 0.99; 95% CI 0.64–1.54), however, the combination group showed an advantage in partial responses with 20.3 versus 6.2% in the everolimus alone group (50), suggesting that there may be some low-level benefit to combination therapy.
The RADIANT-3 trial (NCT00510068) extended the study of everolimus alone to a larger number of patients with advanced, low, or intermediate grade pancreatic NETs with radiologic evidence of progression within the prior 12 months. Patients in this international, multicenter, double-blind phase 3 trial were randomized to receive everolimus (n = 207) or placebo (n = 203) in addition to supportive care (which involved SSA therapy in 40% of patients across both groups). Median PFS in the everolimus group was 11 months (95% CI 8.4–13.9) compared to the placebo group at 4.6 months (95% CI 3.1–5.4) and offered a reduction in relative risk of progression of 65% (HR = 0.35; 95% CI 0.27–0.45; p < 0.001) (51). The RADIANT-4 trial (NCT01524783) further studied everolimus in patients with advanced, progressive, well-differentiated, non-functional NETs of the lung or gastrointestinal tract (ileum and rectum) (52, 53). This randomized, placebo-controlled phase III trial investigated everolimus (n = 205) versus placebo (n = 97) on the background of best supportive care. Overall, patients receiving everolimus demonstrated an increase of 7.1 months PFS compared to placebo (11 months, 95% CI 9.2–13.3 versus 3.9 months, 95% CI 3.6–7.4) and a 52% decrease in relative risk of progression or death (52, 53). In the GI subset of these patients (175 of the 302 enrolled), PFS was increased by 7.7 months (13.1 months, 95% CI 9.2–17.2 in everolimus versus 5.4 months, 95% CI 3.6–9.3 in placebo), with no unexpected safety concerns (53). Taken together, the RADIANT-3 and -4 trials demonstrate that mTOR inhibitors provide durable antitumor effects in NETs from pancreatic, lung, and gastrointestinal origin suggesting that treatment with everolimus, with or without SSA therapy, may continue to be a promising treatment option for patients with progressive, advanced stage NETs. Indeed, there is now a phase 4 clinical trial in progress in China (NCT02842749) to understand the long-term safety of everolimus in locally advanced or metastatic, well-differentiated progressive pancreatic NETs.
Everolimus has also been studied in combination with PRRT. The NETTLE phase 1b study conducted in Australia was a proof of concept study for combination of everolimus with 177Lu-octreotate to identify the maximum tolerated dose, any dose limiting toxicities and to evaluate objective response in patients with progressive low-grade GEP-NENs (n = 16; 11 small bowel, 5 pancreatic). This study reported an overall response rate of 44% (7/16), and all patients maintained stable disease throughout the 6-month course of treatment with a manageable (and apparently reversible) side effect profile (54). Four of five pancreatic NEN patients achieved PR with this combination therapy, which sets the stage for an appropriately powered larger, phase 2 trial for statistical comparisons of combination versus monotherapy on the endpoint of PFS.
Neuroendocrine tumors are highly vascular tumors and overexpression of proangiogenic molecules and their receptors such as vascular endothelial growth factor (VEGF)/VEGF receptor (VEGFR), platelet-derived growth factor (PDGF)/PDGFR, fibroblast growth factor (FGF)/FGFR, and epithelial growth factor (EGF)/EGFR have been reported (55, 56). These observations have led to extensive preclinical and clinical investigations using tyrosine kinase inhibitors (TKI; either small molecule antagonists or blocking antibodies) that inhibit the activity of proangiogenic signals (57).
The oral TKI sunitinib (which targets both VEGFR and PDGFR) was studied in a prospective phase III clinical trial in patients with advanced, well-differentiated pancreatic NETs (NCT00428597). This trial resulted in a 6-month increase in PFS (11.4 months in the sunitinib group versus 5.5 months in the placebo arm; HR 0.42; 95% CI 0.26–0.66; p < 0.001) (58). This study was discontinued early by the independent ethics review panel because of a high number of serious adverse events and deaths in the placebo arm, and a significant trend toward improvement in PFS in the treatment group (58). Another small molecule TKI sulfatinib (which targets VEGFR and FGFR) has completed phase I studies (NCT02133157), with an objective response rate of 26.5% (9/34) and a disease control rate of 70.6% (24/34) (59). The phase I study identified 300 mg sulfatinib as the recommended dose for a phase Ib/II trial (NCT02267967) that is nearing completion. Randomized, double-blind phase III trials are currently recruiting to test sulfatinib versus placebo in patients with advanced pancreatic NETs (NCT02589821) and with low/intermediate-grade (G1/G2) advanced extrapancreatic NETs (any location except pancreatic; NCT02588170).
Pazopanib is an inhibitor of VEGFRs 1, 2, and 3 and showed clinical activity in phase II trials (NCT01280201, NCT01099540, NCT01841736) as a monotherapy for advanced GEP-NETS with stable disease or confirmed PR in 75.7% of patients (28/37; 95% CI 58.8–88.2) (60, 61). Combination of pazopanib with octreotide depot (NCT00454363) was associated with tumor response in advanced, well-differentiated pancreatic NETs but not in carcinoids (62), suggesting that a larger phase III trial in advanced pancreatic NETs is warranted. Axitinib (inhibits VEGF and PDGF) had disappointing results as a monotherapy in progressive, advanced grade G1/G2 extrapancreatic NETs (NCT01435122). Tumor growth was inhibited in advanced carcinoids, with radiographic evidence of stable disease in 21/30 (70%) patients, but there was an unacceptably high incidence of hypertension associated with this therapy (63). Lenvatinib is another pan-TKI in an ongoing phase II trial (NCT 02678780, TALENT study) evaluating ORR as the primary endpoint in patients with advanced pancreatic NETs after progression on other therapy (arm1) or in progressive gastrointestinal NETs after SSA therapy (arm2).
Bevacizumab is a recombinant human IgG1 monoclonal antibody that blocks VEGF from binding to VEGFR, resulting in decreased blood vessel density around tumors in rectal cancer studies (64). Initial phase II trials evaluating bevacizumab in advanced carcinoids and well-differentiated metastatic NETs were positive with increases in PFS (65, 66). Bevacizumab was studied in combination with temozolomide in patients with advanced NETs (56% carcinoid, 44% pancreatic; NCT00137774). Interestingly, only pancreatic NETs responded to therapy (5/15, 33%) with no responses in carcinoids (0/19, 0%) suggesting that this regimen may be more efficacious in patients with pancreatic NETs (67). More recently, the XELBEVOCT trial (NCT01203306) studied octreotide LAR + capecitabine ± bevacizumab in well to moderately differentiated metastatic NETs (multiple anatomic primary sites). Of the 45 patients enrolled in the study, partial response (most often in pancreatic NETs) was noted in 8 patients (17.8%; 95% CI 6.4–28.2) (68). A subsequent phase II trial followed this to evaluate the combination of octreotide LAR + everolimus ± bevacizumab (NCT01229943) in locally advanced or metastatic pancreatic NETs that cannot be treated by surgery. Surprisingly, preliminary data available on clinicaltrials.org indicate that there is no statistically significant improvement in PFS with the octreotide + everolimus group at 14 months (95% CI 9.1–16.9) versus 16.7 months (95% CI 12.6–19.7) in the octreotide + everolimus + bevacizumab group (p = 0.12). The phase III SWOG S0518 study (NCT00569127) investigated octreotide + bevacizumab compared to octreotide + interferon alpha-2b in advanced G1/G2 NETs (various anatomical sites). This study concluded there was no discernible difference in PFS between these two treatment groups, suggesting that bevacizumab had similar activity to that of interferon alpha-2b therapy (69).
Immunotherapy is a recent, rapidly emerging therapeutic option for all cancers, including NETs. Immune checkpoint inhibitors block interactions of programmed death-ligand 1 (PD-L1)/programmed cell death receptor 1 (PD1) or cytotoxic T lymphocyte antigen 4 (CTLA4) to block immune escape of tumor cells. PD-L1 is expressed on the surface of many cancer cells and interacts with its receptor PD1 on the surface of T cells. Immune escape occurs when antigens produced by the tumor inhibit T cells, allowing cancer cells to remain undetected by immune surveillance. Antibodies that target and block PD-L1 (avelumab), PD1 (pembrolizumab, nivolumab, JS001, or PDR001), or CTL4 (ipilimumab) have been used in a number of cancers with promising results, including melanoma, renal, lung, prostate, and bladder cancers, and equivalent trials are now in progress for GEP-NETs. Furthermore, durable responses have been obtained with the anti-PD-L1 antibody avelumab in clinical trials of Merkel Cell carcinoma, a NET of the skin, suggesting successful proof of concept for immune therapy in other NETs. Immunohistochemical analysis of PD-L1 expression in intermediate- to high-grade (G2/G3) GEP-NEN/NECs (n = 32, the majority from pancreas and rectum) was measured in 22% of patients and correlated specifically with the aggressive, high grade (G3) tumors. Based on these results, there are a number of clinical trials in progress or soon to open that investigate immunotherapy in GEP-NETs (Table 3).
Despite the abundance of activity in clinical research, the underlying molecular mechanisms of NET tumorigenesis are not fully understood and continue to be actively investigated in the preclinical setting. Recent whole-exome and whole-genome sequencing efforts have begun to reveal the mutational and epigenomic landscape of NET subtypes. The identification of new germline and somatic mutations, along with copy number variations and other changes, has identified novel mechanisms such as Notch, chromatin remodeling, histone modification, and promoter methylation that may be contributing to pathogenesis. These investigations with genetic underpinnings have the potential to be future precision approaches beyond the currently targeted methodologies outlined above.
There have been several reports published on the genetic profiles of different NET subtypes (70, 71). Gebauer et al. identified the degree of genomic instability and identified frequent copy number variations present in low-grade (G1/G2), well-differentiated pancreatic NETs. Using array comparative genomic hybridization, copy number gains were found on 6p22.2-p22.1, 17p13.1, 7p21.3-21.2, and 9q34.11, affecting regulatory genes involved in transcription, signaling, and epigenetic control (70). Recent whole-exome (46) and whole-genome (72) sequencing of pancreatic NETs identified that the most commonly mutated genes in this tumor type are genes involved in chromatin remodeling, such as multiple endocrine neoplasia type 1 (MEN1), death domain-associated protein (DAXX), α-thalassemia/mental retardation syndrome, X-linked (ATRX) (46), and DNA repair genes MUTYH, CHEK2, and BRCA2 (72). Interestingly, these studies demonstrated very little pathogenic role for oncogenes commonly mutated in cancer such as TP53 or RB1. Protein products from DAXX and ATRX form a heterodimer that is required for chromatin remodeling through histone H3.3 at telomeres. In the Jiao study, clinical NET samples with mutations in these genes were associated with improved prognosis (46). However, follow-up studies by other groups wherein telomere-specific FISH and DAXX/ATRX immunohistochemistry were performed, loss of these gene products was associated with worse prognosis for NET patients (73, 74). Other studies in patients with MEN1-related pancreatic NETs suggest that DAXX/ATRX mutations are not driver mutations, but rather later events in the pathogenesis of NET development (75). Although there is still some controversy over the clinical correlation of DAXX/ATRX mutations, this area of research has attracted a great deal of attention. Both the mutations in MEN1 and DAXX/ATRX suggest that targeting these epigenetic mechanisms with small molecule compounds may be a viable approach to developing new therapeutics for pancreatic NETs.
Small intestinal NETs have also been profiled at the genetic level (76–78). The study performed by Banck et al. on 48, G1/G2, well-differentiated SI-NETs identified single-nucleotide variants in a number of known cancer genes, including FGFR2, MEN1, HOOK3, EZH2, MLF1, VHL, NONO, and SMAD1 by whole-exome sequencing. The dysregulated genes implicated several altered cellular processes, such as chromatin remodeling, DNA damage pathways, apoptosis, and RAS signaling. 30% of SI-NETs have genetic mutations in the PI3K/AKT/mTOR pathway (see below) and amplification of AKT1 and 2 occurs the most frequently (44). In a subsequent study, CDKN1B mutations were identified in 8% of tumors, suggesting the gene for p27 to be a putative tumor suppressor in these tumors, with an obvious role in regulating the cell cycle (45, 79). Studies on copy number alterations in SI-NETs identified losses of 11q23.1-qter, 16q12.2-qter, 9pter-p13.2, and 9p13.1-11.2, and gains in 14q11.2, 14q32.2-32.31, 20pter-p11.21, 20q11.1-q11.21, and 20q12-qter (80). These regions impact tumor suppressor genes, growth factors (in particular FGF2/FGFR3/FGFB, PDGR), and signal transduction pathways (TGFB1, IGFBP3, AKT1, E2F1). DNA methylation studies have provided a global picture of gene regulation, highlighting genes, such as WIF1, RASSF1A, CTNNB1, CXCL14, NKX2-3, and others with increased promoter methylation, and at the same time noting a significant decrease in global methylation in tumors compared to normal reference controls (81). Furthermore, Karpathakis et al. performed a large, integrated analysis of these tumors and showed epigenetic-based mutations in 85% of tumors with 21 dysregulated genes, including CDX1, CELSR3, FBP1, and GIPR (79).
In general, GEP-NETs can be divided by location into gastrointestinal and pancreatic, but also by different copy number aberrations, gene expression profiles, and distinct DNA methylation patterns (82–84). Pancreatic NETs have variations in DAXX, ATRX, PTEN, and TSC2, whereas GI-NETs have identified CDKN1B and RASSF1A as the recurrent mutations. Ras-associated domain family 1 (RASSF1) is frequently hypermethylated in pancreatic NETs—75% of 48 well-differentiated tumors demonstrated hypermethylation at this site, with no hypermethylation in adjacent normal tissue (85). Furthermore, this hypermethylation correlated with larger tumors and widespread metastasis. Expression of O(6)-methylguanine DNA methyltransferase (MGMT), a DNA repair enzyme that guards cells against mutations caused by O6 alkylating agents, has also been studied in pancreatic NETs (86, 87). Temozolomide cytotoxicity is attributed to its ability to induce DNA methylation at the O6 position of guanine, leading to DNA mismatch and tumor cell death (88). MGMT promoter methylation was reported in 40% of pancreatic NETs (85), suggesting that this might be a biomarker for response to temozolomide. Others demonstrate that MGMT deficiency due to methylation was more prevalent in pancreatic NETs compared to gastrointestinal NETs (89). Subsequent studies have not identified a correlation between MGMT protein expression and promoter methylation, even though MGMT promoter methylation was significantly associated with response to temozolomide (86, 87). All of these genomic investigations have led to ongoing clinical tissue collection protocols to identify biomarkers (NCT02092714, NCT03130205) and complete molecular profiling (NCT02586844) of NET subtypes to aid in diagnosis and patient management.
Through genome-wide studies, the Notch signaling pathway has been implicated in pathogenesis of GEP-NETs as well. Notch has been studied for many years in the context of cancer; and over the years, the major players in the pathway have been identified, revealing a complex and sometimes redundant signaling network. The Notch pathway is widely recognized as a central player in proliferation, differentiation, and stem cell maintenance. Notch signaling is evolutionarily conserved and canonical signaling relies on the presence of a Notch receptor (in mammals called Notch1–4) binding to a ligand present on a neighboring cell. Ligand binding promotes intracellular cleavage of the receptor by metalloproteases (ADAM and gamma-secretase) to release the active form of the receptor, called the Notch intracellular domain (NICD). The NICD translocates into the nucleus, binds to transcription factor CBF-1/Suppressor of Hairless/LAG-1 (CSL, also known as RBP-Jκ) and activates transcription of Notch-responsive genes (90).
Notch is known to contribute to tumorigenesis in epithelial-derived cancers by inhibiting differentiation, promoting cellular proliferation, and/or inhibiting apoptosis. There is evidence that Notch can behave as a tumor suppressor or oncogene in these cancers depending on the cellular context. In the case of GEP-NETs, few studies have comprehensively examined the complement of Notch molecules and the mechanisms of Notch signaling. Those that have demonstrate that the presence of Notch signaling components varies across cells derived from the neuroendocrine lineage. Immunohistochemical staining for Notch1, Hes1, Hey1, pIGF1R, and FGF2 antibodies on a tissue microarray of 120 well-differentiated NETs arising from the pancreas (n = 74), ileum (n = 31), and rectum (n = 15), demonstrated elevated Notch1 expression in 100% rectal, 34% of pancreatic, and 0% of ileal NETs. Hes1 expression was present in 64% of rectal, 10% of pancreatic, and 0% of ileal NETs (91), exhibiting significant variability in Notch1 signaling across different tissue types. Due to the low/absent expression of Notch in ileal NETs/carcinoids, some have proposed that Notch functions as a tumor suppressor in these tumors. In the non-tumorigenic cell, Notch signaling activates a cascade of events that ultimately leads to the inhibition of a protein called ASCL1 (92). In ileal NETs, ASCL1 is overexpressed and transient overexpression of Notch1 in carcinoid cell lines in vitro can reverse ASCL1 overexpression, suggesting that activation of Notch1 may be therapeutic. In addition, organic extracts such as resveratrol activate Notch in vitro and have been proposed as therapy in patients with low-grade GI-NETs (NCT01476592 (93, 94)).
The presence of coactivators and corepressors also dictates Notch functionality. CSL coactivators such as MAML, SKIP, and p300 are well known to activate transcription of Notch target genes by binding to NICD, while in the absence of NICD, corepressors such as SMRT (95), SIRT (96), and others (97) inhibit such functions. Notch activator and repressor complexes can also actively remodel the chromatin at Notch-responsive target genes and provide an additional layer of reversible epigenetic regulation (98) by recruiting proteins with histone modification potential (99–101). A report by Liefke et al. (98) demonstrates that the histone demethylase KDM5A/RBP2 is a key component of the Notch CSL repressor complex. Recent studies demonstrate that RBP2 is upregulated in gastrointestinal NETs and in liver metastases from primary NET tumors, suggesting that RBP2 may be actively repressing canonical Notch activity (102) or perhaps remodeling chromatin in these tumors, resulting in aberrant expression of RBP2-regulated genes. Putative inhibitors of RBP2 demethylase activity and/or steric inhibitors to block protein–protein interaction may demonstrate efficacy for this tumor type. Notch receptor, ligand, coactivator, and corepressor function have not been fully analyzed across the spectrum NETs and the complete profile of Notch components present in these diverse tumors may begin to explain the wide variation in clinical responses observed with NETs.
Genome-wide studies in combination with older immunohistochemical studies have identified components of the PI3K/AKT pathway as dysregulated in NETs (70, 71). Although the PI3K/AKT pathway has been studied for decades in many solid tumors, it has more recently been in the spotlight with respect to GEP-NETs. The PI3K/AKT pathway integrates a multitude of extracellular signals and transmits these signals downstream via mTOR. PI3K/AKT/mTOR is the central regulator of a plethora of downstream events, including cell proliferation, apoptosis, cell survival, differentiation, angiogenesis, and cell migration. PI3K can be activated at the cell surface by tyrosine kinase receptors, G-coupled receptors or mutant RAS, and is antagonized by PTEN. Robbins and Hague have conducted a thorough review of PI3K/AKT in GEP-NETs (103), and a few points warrant reemphasis. Pancreatic NETs have a decrease in PTEN expression in 50% of patients, and a downregulation of TSC2 in 35% of patients, both of which correlate with poor survival (71). Small intestinal NETs also have dysregulated PI3K/AKT/mTOR pathway, but exhibit amplification of AKT in 33% of patients (44). This suggests that while there is clear evidence that this pathway is dysregulated in both pancreatic and intestinal NETs, the underlying molecular mechanisms are potentially different, and may explain the varied responses to targeted therapy in clinical trials.
Gastrointestinal NETs are a very heterogeneous group of tumors. Targeted therapies are available to treat these tumors but despite the many clinical approaches to NETs, and beyond surgery for localized disease, there is little consensus on first line therapy. Results of ongoing clinical trials will better inform patient management with respect to selection, timing, duration, and combination of available therapies, and immunotherapy holds great promise for NETs and other cancers. Furthermore, genetics-based approaches may hold the key toward precision therapies and future investigations into novel pathways may help define driver mutations present in the different subtypes of NETs. Future clinical utilization of gene panels, methylation screening tools and other molecular biomarker approaches in addition to classical neuroendocrine markers, will facilitate treatment and improve outcomes of this disease.
JC wrote and edited the entire manuscript.
The author declares that the research was conducted in the absence of any commercial or financial relationships that could be construed as a potential conflict of interest.
This work was supported by the Louisiana State University Health Sciences Center Department of Genetics.
1. Yao JC, Hassan M, Phan A, Dagohoy C, Leary C, Mares JE, et al. One hundred years after “carcinoid”: epidemiology of and prognostic factors for neuroendocrine tumors in 35,825 cases in the United States. J Clin Oncol (2008) 26:3063–72. doi:10.1200/JCO.2007.15.4377
2. Rindi G, Kloppel G, Alhman H, Caplin M, Couvelard A, de Herder WW, et al. TNM staging of foregut (neuro)endocrine tumors: a consensus proposal including a grading system. Virchows Arch (2006) 449:395–401. doi:10.1007/s00428-006-0250-1
3. Bosman F, Carneiro F, Hruban R, Theise N. WHO Classification of Tumours of the Digestive System. Geneva, Switzerland: IARC Press (2010).
4. Kloppel G, Klimstra DS, Hruban RH, Adsay NV, Capella C, Couvelard A, et al. Pancreatic neuroendocrine tumors: update on the new World Health Organization classification. AJSP Rev Rep (2017) 22:233–9. doi:10.1097/PCR.0000000000000211
5. Heetfeld M, Chougnet CN, Olsen IH, Rinke A, Borbath I, Crespo G, et al. Characteristics and treatment of patients with G3 gastroenteropancreatic neuroendocrine neoplasms. Endocr Relat Cancer (2015) 22:657–64. doi:10.1530/ERC-15-0119
6. Hijioka S, Hosoda W, Matsuo K, Ueno M, Furukawa M, Yoshitomi H, et al. Rb loss and KRAS mutation are predictors of the response to platinum-based chemotherapy in pancreatic neuroendocrine neoplasm with grade 3: a Japanese multicenter pancreatic NEN-G3 study. Clin Cancer Res (2017) 23:4625–32. doi:10.1158/1078-0432.CCR-16-3135
7. Sorbye H, Strosberg J, Baudin E, Klimstra DS, Yao JC. Gastroenteropancreatic high-grade neuroendocrine carcinoma. Cancer (2014) 120:2814–23. doi:10.1002/cncr.28721
8. La Rosa S, Sessa F, Uccella S. Mixed neuroendocrine-nonneuroendocrine neoplasms (MiNENs): unifying the concept of a heterogeneous group of neoplasms. Endocr Pathol (2016) 27:284–311. doi:10.1007/s12022-016-9432-9
9. Kidd M, Modlin I, Oberg K. Towards a new classification of gastroenteropancreatic neuroendocrine neoplasms. Nat Rev Clin Oncol (2016) 13:691–705. doi:10.1038/nrclinonc.2016.85
10. Kulke MH, Siu LL, Tepper JE, Fisher G, Jaffe D, Haller DG, et al. Future directions in the treatment of neuroendocrine tumors: consensus report of the national cancer institute neuroendocrine tumor clinical trials planning meeting. J Clin Oncol (2011) 29:934–43. doi:10.1200/JCO.2010.33.2056
11. Luo G, Javed A, Strosberg JR, Jin K, Zhang Y, Liu C, et al. Modified staging classification for pancreatic neuroendocrine tumors on the basis of the American Joint Committee on Cancer and European Neuroendocrine Tumor Society Systems. J Clin Oncol (2017) 35:274–80. doi:10.1200/JCO.2016.67.8193
12. Howe JR, Cardona K, Fraker DL, Kebebew E, Untch BR, Wang YZ, et al. The surgical management of small bowel neuroendocrine tumors: consensus guidelines of the North American Neuroendocrine Tumor Society. Pancreas (2017) 46:715–31. doi:10.1097/MPA.0000000000000846
13. Strosberg JR, Halfdanarson TR, Bellizzi AM, Chan JA, Dillon JS, Heaney AP, et al. The North American Neuroendocrine Tumor Society consensus guidelines for surveillance and medical management of midgut neuroendocrine tumors. Pancreas (2017) 46:707–14. doi:10.1097/MPA.0000000000000850
14. Singh S, Asa SL, Dey C, Kennecke H, Laidley D, Law C, et al. Diagnosis and management of gastrointestinal neuroendocrine tumors: an evidence-based Canadian consensus. Cancer Treat Rev (2016) 47:32–45. doi:10.1016/j.ctrv.2016.05.003
15. Garcia-Carbonero R, Capdevila J, Crespo-Herrero G, Diaz-Perez JA, Martinez Del Prado MP, Alonso Orduna V, et al. Incidence, patterns of care and prognostic factors for outcome of gastroenteropancreatic neuroendocrine tumors (GEP-NETs): results from the National Cancer Registry of Spain (RGETNE). Ann Oncol (2010) 21:1794–803. doi:10.1093/annonc/mdq022
16. Lawrence B, Gustafsson BI, Chan A, Svejda B, Kidd M, Modlin IM. The epidemiology of gastroenteropancreatic neuroendocrine tumors. Endocrinol Metab Clin North Am (2011) 40:1–18, vii. doi:10.1016/j.ecl.2010.12.005
17. Mocellin S, Nitti D. Gastrointestinal carcinoid: epidemiological and survival evidence from a large population-based study (n = 25 531). Ann Oncol (2013) 24:3040–4. doi:10.1093/annonc/mdt377
18. Modlin IM, Moss SF, Chung DC, Jensen RT, Snyderwine E. Priorities for improving the management of gastroenteropancreatic neuroendocrine tumors. J Natl Cancer Inst (2008) 100:1282–9. doi:10.1093/jnci/djn275
19. Wolin EM. The expanding role of somatostatin analogs in the management of neuroendocrine tumors. Gastrointest Cancer Res (2012) 5:161–8.
20. Zandee WT, Kamp K, van Adrichem RC, Feelders RA, de Herder WW. Effect of hormone secretory syndromes on neuroendocrine tumor prognosis. Endocr Relat Cancer (2017) 24:R261–74. doi:10.1530/ERC-16-0538
21. Vinik AI, Wolin EM, Liyanage N, Gomez-Panzani E, Fisher GA; ELECT Study Group *. Evaluation of lanreotide depot/autogel efficacy and safety as a carcinoid syndrome treatment (Elect): a randomized, double-blind, placebo-controlled trial. Endocr Pract (2016) 22:1068–80. doi:10.4158/EP151172.OR
22. Renzullo A, Accardo G, Esposito D, de Bellis A, Bizzarro A, Romano M, et al. Hashimoto’s thyroiditis and entero-chromaffin-like cell hyperplasia: early detection and somatostatin analogue treatment. Eur J Inflamm (2013) 11:863–70. doi:10.1177/1721727X1301100329
23. Rinke A, Muller HH, Schade-Brittinger C, Klose KJ, Barth P, Wied M, et al. Placebo-controlled, double-blind, prospective, randomized study on the effect of octreotide LAR in the control of tumor growth in patients with metastatic neuroendocrine midgut tumors: a report from the PROMID study group. J Clin Oncol (2009) 27:4656–63. doi:10.1200/JCO.2009.22.8510
24. Rinke A, Wittenberg M, Schade-Brittinger C, Aminossadati B, Ronicke E, Gress TM, et al. Placebo-controlled, double-blind, prospective, randomized study on the effect of octreotide LAR in the control of tumor growth in patients with metastatic neuroendocrine midgut tumors (PROMID): results of long-term survival. Neuroendocrinology (2017) 104:26–32. doi:10.1159/000443612
25. Caplin ME, Pavel M, Cwikla JB, Phan AT, Raderer M, Sedlackova E, et al. Lanreotide in metastatic enteropancreatic neuroendocrine tumors. N Engl J Med (2014) 371:224–33. doi:10.1056/NEJMoa1316158
26. Caplin ME, Pavel M, Cwikla JB, Phan AT, Raderer M, Sedlackova E, et al. Anti-tumour effects of lanreotide for pancreatic and intestinal neuroendocrine tumours: the CLARINET open-label extension study. Endocr Relat Cancer (2016) 23:191–9. doi:10.1530/ERC-15-0490
27. Cives M, Strosberg J. Radionuclide therapy for neuroendocrine tumors. Curr Oncol Rep (2017) 19:9. doi:10.1007/s11912-017-0567-8
28. Reubi JC, Schonbrunn A. Illuminating somatostatin analog action at neuroendocrine tumor receptors. Trends Pharmacol Sci (2013) 34:676–88. doi:10.1016/j.tips.2013.10.001
29. Mohamed A, Blanchard MP, Albertelli M, Barbieri F, Brue T, Niccoli P, et al. Pasireotide and octreotide antiproliferative effects and sst2 trafficking in human pancreatic neuroendocrine tumor cultures. Endocr Relat Cancer (2014) 21:691–704. doi:10.1530/ERC-14-0086
30. Strosberg J, El-Haddad G, Wolin E, Hendifar A, Yao J, Chasen B, et al. Phase 3 trial of 177Lu-dotatate for midgut neuroendocrine tumors. N Engl J Med (2017) 376:125–35. doi:10.1056/NEJMoa1607427
31. Claringbold PG, Brayshaw PA, Price RA, Turner JH. Phase II study of radiopeptide 177Lu-octreotate and capecitabine therapy of progressive disseminated neuroendocrine tumours. Eur J Nucl Med Mol Imaging (2011) 38:302–11. doi:10.1007/s00259-010-1631-x
32. van Essen M, Krenning EP, Kam BL, de Herder WW, van Aken MO, Kwekkeboom DJ. Report on short-term side effects of treatments with 177Lu-octreotate in combination with capecitabine in seven patients with gastroenteropancreatic neuroendocrine tumours. Eur J Nucl Med Mol Imaging (2008) 35:743–8. doi:10.1007/s00259-007-0688-7
33. Claringbold PG, Price RA, Turner JH. Phase I-II study of radiopeptide 177Lu-octreotate in combination with capecitabine and temozolomide in advanced low-grade neuroendocrine tumors. Cancer Biother Radiopharm (2012) 27:561–9. doi:10.1089/cbr.2012.1276
34. Claringbold PG, Turner JH. Pancreatic neuroendocrine tumor control: durable objective response to combination 177Lu-octreotate-capecitabine-temozolomide radiopeptide chemotherapy. Neuroendocrinology (2016) 103:432–9. doi:10.1159/000434723
35. Christ E, Wild D, Forrer F, Brandle M, Sahli R, Clerici T, et al. Glucagon-like peptide-1 receptor imaging for localization of insulinomas. J Clin Endocrinol Metab (2009) 94:4398–405. doi:10.1210/jc.2009-1082
36. Wild D, Macke H, Christ E, Gloor B, Reubi JC. Glucagon-like peptide 1-receptor scans to localize occult insulinomas. N Engl J Med (2008) 359:766–8. doi:10.1056/NEJMc0802045
37. Sherman SK, Carr JC, Wang D, O’Dorisio MS, O’Dorisio TM, Howe JR. Gastric inhibitory polypeptide receptor (GIPR) is a promising target for imaging and therapy in neuroendocrine tumors. Surgery (2013) 154:1206–13; discussion 1214. doi:10.1016/j.surg.2013.04.052
38. Sherman SK, Maxwell JE, Carr JC, Wang D, O’Dorisio MS, O’Dorisio TM, et al. GIPR expression in gastric and duodenal neuroendocrine tumors. J Surg Res (2014) 190:587–93. doi:10.1016/j.jss.2014.01.044
39. Waser B, Rehmann R, Sanchez C, Fourmy D, Reubi JC. Glucose-dependent insulinotropic polypeptide receptors in most gastroenteropancreatic and bronchial neuroendocrine tumors. J Clin Endocrinol Metab (2012) 97:482–8. doi:10.1210/jc.2011-2454
40. Gourni E, Waser B, Clerc P, Fourmy D, Reubi JC, Maecke HR. The glucose-dependent insulinotropic polypeptide receptor: a novel target for neuroendocrine tumor imaging-first preclinical studies. J Nucl Med (2014) 55:976–82. doi:10.2967/jnumed.113.133744
41. Johannessen CM, Reczek EE, James MF, Brems H, Legius E, Cichowski K. The NF1 tumor suppressor critically regulates TSC2 and mTOR. Proc Natl Acad Sci U S A (2005) 102:8573–8. doi:10.1073/pnas.0503224102
42. Oberg K. The genetics of neuroendocrine tumors. Semin Oncol (2013) 40:37–44. doi:10.1053/j.seminoncol.2012.11.005
43. Chan J, Kulke M. Targeting the mTOR signaling pathway in neuroendocrine tumors. Curr Treat Options Oncol (2014) 15:365–79. doi:10.1007/s11864-014-0294-4
44. Banck MS, Kanwar R, Kulkarni AA, Boora GK, Metge F, Kipp BR, et al. The genomic landscape of small intestine neuroendocrine tumors. J Clin Invest (2013) 123:2502–8. doi:10.1172/JCI67963
45. Francis JM, Kiezun A, Ramos AH, Serra S, Pedamallu CS, Qian ZR, et al. Somatic mutation of CDKN1B in small intestine neuroendocrine tumors. Nat Genet (2013) 45:1483–6. doi:10.1038/ng.2821
46. Jiao Y, Shi C, Edil BH, de Wilde RF, Klimstra DS, Maitra A, et al. DAXX/ATRX, MEN1, and mTOR pathway genes are frequently altered in pancreatic neuroendocrine tumors. Science (2011) 331:1199–203. doi:10.1126/science.1200609
47. Zoncu R, Efeyan A, Sabatini DM. mTOR: from growth signal integration to cancer, diabetes and ageing. Nat Rev Mol Cell Biol (2011) 12:21–35. doi:10.1038/nrm3025
48. Yao JC, Phan AT, Chang DZ, Wolff RA, Hess K, Gupta S, et al. Efficacy of RAD001 (everolimus) and octreotide LAR in advanced low- to intermediate-grade neuroendocrine tumors: results of a phase II study. J Clin Oncol (2008) 26:4311–8. doi:10.1200/JCO.2008.16.7858
49. Pavel ME, Hainsworth JD, Baudin E, Peeters M, Horsch D, Winkler RE, et al. Everolimus plus octreotide long-acting repeatable for the treatment of advanced neuroendocrine tumours associated with carcinoid syndrome (RADIANT-2): a randomised, placebo-controlled, phase 3 study. Lancet (2011) 378:2005–12. doi:10.1016/S0140-6736(11)61742-X
50. Kulke MH, Ruszniewski P, Van Cutsem E, Lombard-Bohas C, Valle JW, De Herder WW, et al. A randomized, open-label, phase 2 study of everolimus in combination with pasireotide LAR or everolimus alone in advanced, well differentiated, progressive pancreatic neuroendocrine tumors: COOPERATE-2 trial. Ann Oncol (2017) 28:1309–15. doi:10.1093/annonc/mdx078
51. Yao JC, Shah MH, Ito T, Bohas CL, Wolin EM, Van Cutsem E, et al. Everolimus for advanced pancreatic neuroendocrine tumors. N Engl J Med (2011) 364:514–23. doi:10.1056/NEJMoa1009290
52. Yao JC, Fazio N, Singh S, Buzzoni R, Carnaghi C, Wolin E, et al. Everolimus for the treatment of advanced, non-functional neuroendocrine tumours of the lung or gastrointestinal tract (RADIANT-4): a randomised, placebo-controlled, phase 3 study. Lancet (2016) 387:968–77. doi:10.1016/S0140-6736(15)00817-X
53. Singh S, Carnaghi C, Buzzoni R, Pommier RF, Raderer M, Tomasek J, et al. Everolimus in neuroendocrine tumors of the gastrointestinal tract and unknown primary. Neuroendocrinology (2017). doi:10.1159/000477585
54. Claringbold PG, Turner JH. NeuroEndocrine tumor therapy with lutetium-177-octreotate and everolimus (NETTLE): a phase I study. Cancer Biother Radiopharm (2015) 30:261–9. doi:10.1089/cbr.2015.1876
55. Besig S, Voland P, Baur DM, Perren A, Prinz C. Vascular endothelial growth factors, angiogenesis, and survival in human ileal enterochromaffin cell carcinoids. Neuroendocrinology (2009) 90:402–15. doi:10.1159/000245900
56. Zhang J, Jia Z, Li Q, Wang L, Rashid A, Zhu Z, et al. Elevated expression of vascular endothelial growth factor correlates with increased angiogenesis and decreased progression-free survival among patients with low-grade neuroendocrine tumors. Cancer (2007) 109:1478–86. doi:10.1002/cncr.22554
57. Carrasco P, Zuazo-Gaztelu I, Casanovas O. Sprouting strategies and dead ends in anti-angiogenic targeting of NETs. J Mol Endocrinol (2017) 59:R77–91. doi:10.1530/JME-17-0029
58. Raymond E, Dahan L, Raoul JL, Bang YJ, Borbath I, Lombard-Bohas C, et al. Sunitinib malate for the treatment of pancreatic neuroendocrine tumors. N Engl J Med (2011) 364:501–13. doi:10.1056/NEJMoa1003825
59. Xu JM, Wang Y, Chen YL, Jia R, Li J, Gong JF, et al. Sulfatinib, a novel kinase inhibitor, in patients with advanced solid tumors: results from a phase I study. Oncotarget (2017) 8:42076–86. doi:10.18632/oncotarget.14942
60. Ahn HK, Choi JY, Kim KM, Kim H, Choi SH, Park SH, et al. Phase II study of pazopanib monotherapy in metastatic gastroenteropancreatic neuroendocrine tumours. Br J Cancer (2013) 109:1414–9. doi:10.1038/bjc.2013.470
61. Grande E, Capdevila J, Castellano D, Teule A, Duran I, Fuster J, et al. Pazopanib in pretreated advanced neuroendocrine tumors: a phase II, open-label trial of the Spanish task force group for neuroendocrine tumors (GETNE). Ann Oncol (2015) 26:1987–93. doi:10.1093/annonc/mdv252
62. Phan AT, Halperin DM, Chan JA, Fogelman DR, Hess KR, Malinowski P, et al. Pazopanib and depot octreotide in advanced, well differentiated neuroendocrine tumours: a multicentre, single-group, phase 2 study. Lancet Oncol (2015) 16:695–703. doi:10.1016/S1470-2045(15)70136-1
63. Strosberg JR, Cives M, Hwang J, Weber T, Nickerson M, Atreya CE, et al. A phase II study of axitinib in advanced neuroendocrine tumors. Endocr Relat Cancer (2016) 23:411–8. doi:10.1530/ERC-16-0008
64. Willett CG, Boucher Y, di Tomaso E, Duda DG, Munn LL, Tong RT, et al. Direct evidence that the VEGF-specific antibody bevacizumab has antivascular effects in human rectal cancer. Nat Med (2004) 10:145–7. doi:10.1038/nm0604-649c
65. Kulke MH, Chan JA, Meyerhardt JA, Zhu AX, Abrams TA, Blaszkowsky LS, et al. A prospective phase II study of 2-methoxyestradiol administered in combination with bevacizumab in patients with metastatic carcinoid tumors. Cancer Chemother Pharmacol (2011) 68:293–300. doi:10.1007/s00280-010-1478-7
66. Yao JC, Phan A, Hoff PM, Chen HX, Charnsangavej C, Yeung SC, et al. Targeting vascular endothelial growth factor in advanced carcinoid tumor: a random assignment phase II study of depot octreotide with bevacizumab and pegylated interferon alpha-2b. J Clin Oncol (2008) 26:1316–23. doi:10.1200/JCO.2007.13.6374
67. Chan JA, Stuart K, Earle CC, Clark JW, Bhargava P, Miksad R, et al. Prospective study of bevacizumab plus temozolomide in patients with advanced neuroendocrine tumors. J Clin Oncol (2012) 30:2963–8. doi:10.1200/JCO.2011.40.3147
68. Berruti A, Fazio N, Ferrero A, Brizzi MP, Volante M, Nobili E, et al. Bevacizumab plus octreotide and metronomic capecitabine in patients with metastatic well-to-moderately differentiated neuroendocrine tumors: the XELBEVOCT study. BMC Cancer (2014) 14:184. doi:10.1186/1471-2407-14-184
69. Yao JC, Guthrie KA, Moran C, Strosberg JR, Kulke MH, Chan JA, et al. Phase III prospective randomized comparison trial of depot octreotide plus interferon alfa-2b versus depot octreotide plus bevacizumab in patients with advanced carcinoid tumors: SWOG S0518. J Clin Oncol (2017) 35:1695–703. doi:10.1200/JCO.2016.70.4072
70. Gebauer N, Schmidt-Werthern C, Bernard V, Feller AC, Keck T, Begum N, et al. Genomic landscape of pancreatic neuroendocrine tumors. World J Gastroenterol (2014) 20:17498–506. doi:10.3748/wjg.v20.i46.17498
71. Missiaglia E, Dalai I, Barbi S, Beghelli S, Falconi M, della Peruta M, et al. Pancreatic endocrine tumors: expression profiling evidences a role for AKT-mTOR pathway. J Clin Oncol (2010) 28:245–55. doi:10.1200/JCO.2008.21.5988
72. Scarpa A, Chang DK, Nones K, Corbo V, Patch AM, Bailey P, et al. Whole-genome landscape of pancreatic neuroendocrine tumours. Nature (2017) 543:65–71. doi:10.1038/nature21063
73. Singhi AD, Liu TC, Roncaioli JL, Cao D, Zeh HJ, Zureikat AH, et al. Alternative lengthening of telomeres and loss of DAXX/ATRX expression predicts metastatic disease and poor survival in patients with pancreatic neuroendocrine tumors. Clin Cancer Res (2017) 23:600–9. doi:10.1158/1078-0432.CCR-16-1113
74. Marinoni I, Kurrer AS, Vassella E, Dettmer M, Rudolph T, Banz V, et al. Loss of DAXX and ATRX are associated with chromosome instability and reduced survival of patients with pancreatic neuroendocrine tumors. Gastroenterology (2014) 146:453–60.e5. doi:10.1053/j.gastro.2013.10.020
75. de Wilde RF, Heaphy CM, Maitra A, Meeker AK, Edil BH, Wolfgang CL, et al. Loss of ATRX or DAXX expression and concomitant acquisition of the alternative lengthening of telomeres phenotype are late events in a small subset of MEN-1 syndrome pancreatic neuroendocrine tumors. Mod Pathol (2012) 25:1033–9. doi:10.1038/modpathol.2012.53
76. Andersson E, Sward C, Stenman G, Ahlman H, Nilsson O. High-resolution genomic profiling reveals gain of chromosome 14 as a predictor of poor outcome in ileal carcinoids. Endocr Relat Cancer (2009) 16:953–66. doi:10.1677/ERC-09-0052
77. Nilsson O. Profiling of ileal carcinoids. Neuroendocrinology (2013) 97:7–18. doi:10.1159/000343232
78. Andersson E, Arvidsson Y, Sward C, Hofving T, Wangberg B, Kristiansson E, et al. Expression profiling of small intestinal neuroendocrine tumors identifies subgroups with clinical relevance, prognostic markers and therapeutic targets. Mod Pathol (2016) 29:616–29. doi:10.1038/modpathol.2016.48
79. Karpathakis A, Dibra H, Pipinikas C, Feber A, Morris T, Francis J, et al. Prognostic impact of novel molecular subtypes of small intestinal neuroendocrine tumor. Clin Cancer Res (2016) 22:250–8. doi:10.1158/1078-0432.CCR-15-0373
80. Hashemi J, Fotouhi O, Sulaiman L, Kjellman M, Hoog A, Zedenius J, et al. Copy number alterations in small intestinal neuroendocrine tumors determined by array comparative genomic hybridization. BMC Cancer (2013) 13:505. doi:10.1186/1471-2407-13-505
81. Fotouhi O, Adel Fahmideh M, Kjellman M, Sulaiman L, Hoog A, Zedenius J, et al. Global hypomethylation and promoter methylation in small intestinal neuroendocrine tumors: an in vivo and in vitro study. Epigenetics (2014) 9:987–97. doi:10.4161/epi.28936
82. Cives M, Simone V, Rizzo FM, Silvestris F. NETs: organ-related epigenetic derangements and potential clinical applications. Oncotarget (2016) 7:57414–29. doi:10.18632/oncotarget.10598
83. Karpathakis A, Dibra H, Thirlwell C. Neuroendocrine tumours: cracking the epigenetic code. Endocr Relat Cancer (2013) 20:R65–82. doi:10.1530/ERC-12-0338
84. How-Kit A, Dejeux E, Dousset B, Renault V, Baudry M, Terris B, et al. DNA methylation profiles distinguish different subtypes of gastroenteropancreatic neuroendocrine tumors. Epigenomics (2015) 7:1245–58. doi:10.2217/epi.15.85
85. House MG, Herman JG, Guo MZ, Hooker CM, Schulick RD, Lillemoe KD, et al. Aberrant hypermethylation of tumor suppressor genes in pancreatic endocrine neoplasms. Ann Surg (2003) 238:423–31; discussion 431–2. doi:10.1097/01.sla.0000086659.49569.9e
86. Schmitt AM, Pavel M, Rudolph T, Dawson H, Blank A, Komminoth P, et al. Prognostic and predictive roles of MGMT protein expression and promoter methylation in sporadic pancreatic neuroendocrine neoplasms. Neuroendocrinology (2014) 100:35–44. doi:10.1159/000365514
87. Yang QC, Wang YH, Lin Y, Xue L, Chen YJ, Chen MH, et al. Expression of O(6)-methylguanine DNA methyltransferase (MGMT) and its clinical significance in gastroenteropancreatic neuroendocrine neoplasm. Int J Clin Exp Pathol (2014) 7:4204–12.
88. Liu L, Gerson SL. Targeted modulation of MGMT: clinical implications. Clin Cancer Res (2006) 12:328–31. doi:10.1158/1078-0432.CCR-05-2543
89. Kulke MH, Hornick JL, Frauenhoffer C, Hooshmand S, Ryan DP, Enzinger PC, et al. O6-methylguanine DNA methyltransferase deficiency and response to temozolomide-based therapy in patients with neuroendocrine tumors. Clin Cancer Res (2009) 15:338–45. doi:10.1158/1078-0432.CCR-08-1476
90. Crabtree JS, Singleton CS, Miele L. Notch signaling in neuroendocrine tumors. Front Oncol (2016) 6:94. doi:10.3389/fonc.2016.00094
91. Wang H, Chen Y, Fernandez-Del Castillo C, Yilmaz O, Deshpande V. Heterogeneity in signaling pathways of gastroenteropancreatic neuroendocrine tumors: a critical look at notch signaling pathway. Mod Pathol (2013) 26:139–47. doi:10.1038/modpathol.2012.143
92. Kunnimalaiyaan M, Chen H. Tumor suppressor role of Notch-1 signaling in neuroendocrine tumors. Oncologist (2007) 12:535–42. doi:10.1634/theoncologist.12-5-535
93. Pinchot SN, Jaskula-Sztul R, Ning L, Peters NR, Cook MR, Kunnimalaiyaan M, et al. Identification and validation of Notch pathway activating compounds through a novel high-throughput screening method. Cancer (2011) 117:1386–98. doi:10.1002/cncr.25652
94. Kunnimalaiyaan M, Traeger K, Chen H. Conservation of the Notch1 signaling pathway in gastrointestinal carcinoid cells. Am J Physiol Gastrointest Liver Physiol (2005) 289:G636–42. doi:10.1152/ajpgi.00146.2005
95. Kao HY, Ordentlich P, Koyano-Nakagawa N, Tang Z, Downes M, Kintner CR, et al. A histone deacetylase corepressor complex regulates the Notch signal transduction pathway. Genes Dev (1998) 12:2269–77. doi:10.1101/gad.12.15.2269
96. Mulligan P, Yang F, Di Stefano L, Ji JY, Ouyang J, Nishikawa JL, et al. A SIRT1-LSD1 corepressor complex regulates Notch target gene expression and development. Mol Cell (2011) 42:689–99. doi:10.1016/j.molcel.2011.04.020
97. Borggrefe T, Liefke R. Fine-tuning of the intracellular canonical Notch signaling pathway. Cell Cycle (2012) 11:264–76. doi:10.4161/cc.11.2.18995
98. Liefke R, Oswald F, Alvarado C, Ferres-Marco D, Mittler G, Rodriguez P, et al. Histone demethylase KDM5A is an integral part of the core Notch-RBP-J repressor complex. Genes Dev (2010) 24:590–601. doi:10.1101/gad.563210
99. Miele L. Transcription factor RBPJ/CSL: a genome-wide look at transcriptional regulation. Proc Natl Acad Sci U S A (2011) 108:14715–6. doi:10.1073/pnas.1110570108
100. Wang H, Zou J, Zhao B, Johannsen E, Ashworth T, Wong H, et al. Genome-wide analysis reveals conserved and divergent features of Notch1/RBPJ binding in human and murine T-lymphoblastic leukemia cells. Proc Natl Acad Sci U S A (2011) 108:14908–13. doi:10.1073/pnas.1109023108
101. Zhao B, Zou J, Wang H, Johannsen E, Peng CW, Quackenbush J, et al. Epstein-Barr virus exploits intrinsic B-lymphocyte transcription programs to achieve immortal cell growth. Proc Natl Acad Sci U S A (2011) 108:14902–7. doi:10.1073/pnas.1108892108
102. Maggi EC, Trillo-Tinoco J, Struckhoff AP, Vijayaraghavan J, Del Valle L, Crabtree JS. Retinoblastoma-binding protein 2 (RBP2) is frequently expressed in neuroendocrine tumors and promotes the neoplastic phenotype. Oncogenesis (2016) 5:e257. doi:10.1038/oncsis.2016.58
Keywords: neuroendocrine tumor, Notch, small intestinal NET, pancreatic neuroendocrine tumor, carcinoid
Citation: Crabtree JS (2017) Clinical and Preclinical Advances in Gastroenteropancreatic Neuroendocrine Tumor Therapy. Front. Endocrinol. 8:341. doi: 10.3389/fendo.2017.00341
Received: 26 May 2017; Accepted: 21 November 2017;
Published: 04 December 2017
Edited by:
Angela Hague, University of Bristol, United KingdomReviewed by:
Daniela Pasquali, Università degli Studi della Campania “Luigi Vanvitelli” Caserta, ItalyCopyright: © 2017 Crabtree. This is an open-access article distributed under the terms of the Creative Commons Attribution License (CC BY). The use, distribution or reproduction in other forums is permitted, provided the original author(s) or licensor are credited and that the original publication in this journal is cited, in accordance with accepted academic practice. No use, distribution or reproduction is permitted which does not comply with these terms.
*Correspondence: Judy S. Crabtree, amNyYWJ0QGxzdWhzYy5lZHU=
Disclaimer: All claims expressed in this article are solely those of the authors and do not necessarily represent those of their affiliated organizations, or those of the publisher, the editors and the reviewers. Any product that may be evaluated in this article or claim that may be made by its manufacturer is not guaranteed or endorsed by the publisher.
Research integrity at Frontiers
Learn more about the work of our research integrity team to safeguard the quality of each article we publish.