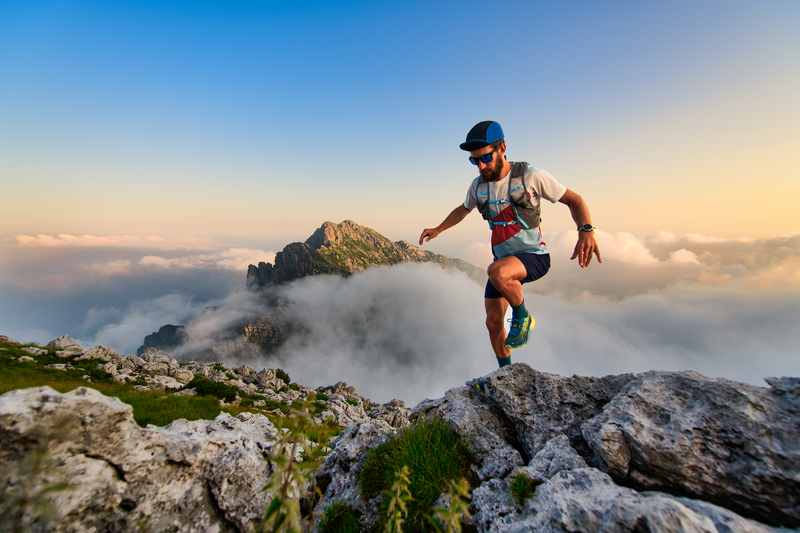
95% of researchers rate our articles as excellent or good
Learn more about the work of our research integrity team to safeguard the quality of each article we publish.
Find out more
REVIEW article
Front. Endocrinol. , 02 August 2017
Sec. Neuroendocrine Science
Volume 8 - 2017 | https://doi.org/10.3389/fendo.2017.00189
This article is part of the Research Topic The Vasopressin System and Behavior View all 18 articles
Nonapeptides, by modulating the activity of neural circuits in specific social contexts, provide an important mechanism underlying the evolution of diverse behavioral phenotypes across vertebrate taxa. Vasotocin-family nonapeptides, in particular, have been found to be involved in behavioral plasticity and diversity in social behavior, including seasonal variation, sexual dimorphism, and species differences. Although nonapeptides have been the focus of a great deal of research over the last several decades, the vast majority of this work has focused on adults. However, behavioral diversity may also be explained by the ways in which these peptides shape neural circuits and influence social processes during development. In this review, I synthesize comparative work on vasotocin-family peptides during development and classic work on early forms of social learning in developmental psychobiology. I also summarize recent work demonstrating that early life manipulations of the nonapeptide system alter attachment, affiliation, and vocal learning in zebra finches. I thus hypothesize that vasotocin-family peptides are involved in the evolution of social behaviors through their influence on learning during sensitive periods in social development.
Both extrinsic and intrinsic experience broadly shape the functional organization of the brain. Functional maturation critically depends on input at particular points in time to ensure that nervous systems are organized to enable the organism to survive and reproduce in maturity. The basic plan of the brain is, of course, governed by genetic expression. However, its development is influenced at every point along the way by the “environment,” broadly construed (1–5).
The development of the brain is shaped by experience that comes from outside the organism, but also by chemical signals that are generated by the organism itself. These signals include chemical gradients that guide the growth of neuronal projections to their targets, or longer distance chemical messengers that modulate the activity of complex neural circuits. Importantly, chemical messengers, such as steroid hormones or neuropeptides, provide a signal to coordinate the development of multiple tissues. Thus, there are important parallels between the organizational role that hormones play during development (intrinsic experience) and the organizational effects of experience that comes from outside of the organism (extrinsic experience).
There is, however, little research investigating the mechanisms that govern how organisms are so exquisitely sensitive to particular kinds of sensory input at specific points in development. In particular, there is a significant gap in our understanding of the neural and neuroendocrine mechanisms that modulate sensitive periods in development, even for potent forms of early learning, such as imprinting or vocal learning. What mechanisms shape the timing, existence, and nature of these sensitive periods in development, particularly given the significant impact that early social learning has on adult phenotype?
The nonapeptides, which provide an evolvable mechanism for modulating the activity of whole neural circuits in specific social contexts, may play an important role in the evolution and development of social phenotypes across vertebrate taxa. The majority of nonapeptide research in the last several decades has focused on adult organisms. Fortunately, there is a re-emerging interest in the effects of the nonapeptides during development (6, 7). The hypothesis that the nonapeptides may exert organizational effects on the brain by producing long term or permanent changes in neural structure was first proposed during the 1980s [arginine vasopressin (AVP) (8); oxytocin (OT) (9)]. Despite several intriguing findings which suggested that the nonapeptides are important during development, there was relatively little interest in this area until recently. This resurgence was largely driven by the realization that exogenous administration of synthetic OT to induce labor may have unknown side effects at a critical point in brain development (10, 11). Furthermore, it was realized that the nonapeptides may also have potential relevance to understanding social deficit and neurodevelopmental disorders (12).
Here, I argue that the nonapeptides, particularly in the vasotocin family, play a critical role during sensitive periods for social learning. Nonapeptides are known to influence many brain regions involved in multiple social behaviors. I present some speculative evidence that they may also do so during development in ways that have profound consequences for adult social behavior. Similar to discoveries in the newly emerging field of evolutionary developmental biology (evo-devo), this evidence suggests that subtle variations in nonapeptide circuitry during development may underlie species differences in social behaviors through their influence on social learning processes. To frame this argument, I attempt to link findings from several previously unconnected fields, including classical work in developmental psychobiology on sensitive periods, the evolution and development of the nonapeptide system, and several theories about how nonapeptides affect social processes. Finally, I will summarize recent work in the zebra finch (Taeniopygia guttata) supporting this claim which demonstrates that early life manipulations of the nonapeptide system alter attachment to parents, affiliative behavior in adult males, and vocal learning.
There is extensive evidence of early sensitive periods in development, in which an organism demonstrates a marked susceptibility or vulnerability to particular stimuli during a limited time window early in life (13, 14). This phenomenon reflects a developmental phase of built-in competence for exchange between an organism and its environment. Sensitive periods are most often observed in the context of what is known as experience-expectant learning, where an organism depends on certain types of experience in order to develop normally. By experimentally removing or altering the stimulation, we can reveal the extent to which an organism requires the species-typical input for species-typical development. For example, visual deprivation early in life in a number of species can cause disorganization of the cortical columns necessary to process visual stimuli (15). In addition, certain cues in an organism’s environment may provide information about which phenotypes will be most successful given the environment the organism is likely to encounter. In this case, developing organisms “sample” their environment during sensitive periods for cues which direct development in an adaptive direction. For example, early nutritional stress can serve to program physiological function in ways that would enhance postnatal survival under conditions of intermittent or poor nutrition (16).
Some of the most striking examples of sensitive periods in development occur in the social domain. One particularly potent form of an early sensitive period is imprinting. Imprinting, such as filial or sexual imprinting, is defined as a form of learning that (1) can only take place during a restricted window of time in an individual’s life, (2) is irreversible, (3) involves the learning of species specific or individual-specific characters, and (4) may occur at a time when the appropriate behavior itself is not yet performed (17). Visual imprinting phenomena have been best studied in birds (18–23), where the circuit underlying filial imprinting has been well characterized (24–26). Precocial birds, such as domestic chickens and ducks, will approach and follow any object which they were exposed to immediately after hatching, typically the mother. In addition, there is work from a number of fish species demonstrating imprinting phenomena used for species and kin recognition, primarily in the olfactory domain (27–34).
There are also more subtle forms of sensitive periods in social development, in which experience with caregivers early in life shapes later social relationships (35–38). Across taxa, isolation from conspecifics and caregivers results in significant disruptions to social functioning later in life (39–42). Furthermore, research in rats has demonstrated that early experiences of maternal care (e.g., licking and grooming behaviors) can alter both the responsiveness to stressors and maternal behavior in adulthood (37, 43, 44). Song learning in birds and vocal learning in humans, though they occur later in development, are also examples of sensitive periods in development in which the developing organism is dependent on interactions with adult caregivers to learn species-typical vocal structures (45, 46).
What appears to be common across these different kinds of sensitive periods in development is competitive exclusion—a particular class of sensory input from the environment is particularly influential, to the exclusion of others (47). These early forms of social learning, particularly about the identity, features, and valence of caregivers, provides an important foundation for later learning. Both learning the characteristics of and maintaining the motivation to be proximal to caregivers provide developing organisms with food and protection but also an abundance of opportunities for social learning. Indeed, these early social experiences provide the foundation upon which all future social interactions are built.
In each of these cases, we have a sense of what circuits are involved in the sensitive periods (as well as a number of ways to disrupt those circuits), but critically we do not know the mechanisms of how or why there is a particular kind of sensitivity in the first place. For example, the filial imprinting work in birds has focused on forebrain regions thought to be homologous to mammalian cortex which are involved in visual processing and multimodal association (24–26, 48). But why those particular inputs at that particular time?
One theory about how sensitive periods emerge is based on the observation that the sensory systems do not all become functional at the same time, but rather sequentially. In both birds and mammals, the first sensory modality to become functional is the tactile/vestibular system, followed in order by the chemosensory/olfactory, auditory, and visual systems (49, 50). Based on the pioneering work of Gilbert Gottlieb in avian development, Turkewitz and Kenny proposed that the invariance in the sequential onset of sensory function results in a reduction in the complexity of the sensory experience for the developing organism and a more reliable structure to the prenatal and early postnatal experience (51). Developing organisms are not bombarded with novel stimuli in all sensory modalities upon birth, but instead encounter a drastically reduced sensory experience. Earlier-developing systems (i.e., tactile and olfactory) in fact develop under reduced competition from other sensory modalities. This allows later-developing systems (i.e., visual and auditory) to build on associations formed in earlier-developing systems.
Furthermore, given that many sensory systems begin to develop prior to birth or hatching, the in utero and in ovo environments provide learning opportunities for the developing embryo. Precocial birds, which are born with all sensory systems functional at birth, benefit from extensive learning that takes place in the egg which they use to support the “emergence, maintenance, and transformation of behavior” (52). For example, in ducks, the preference for the maternal assembly call is dependent upon the prenatal exposure of the embryo either to its own vocalizations or those of its siblings in the days prior to hatching (53). Similarly, bob white quail denied interaction with broodmates after hatching fail to develop preferences for species-specific maternal cues (54). Even altricial rodents, which are less mature at birth, also use olfactory associations formed in utero to perform suckling behaviors (55).
In addition, generalized physiological arousal has been identified as a critical component of a young organism’s perceptual learning and development. In human infants, for example, there is a strong association between arousal levels and sensitivities to sensory stimulation (56–58). Physiological arousal can be manipulated neurochemically, or by simply making sensory stimuli more salient. For example, only rat pups receiving either tactile stimulation or injected with amphetamine while exposed to an artificial odor preferred to suckle nipples coated in the familiar odor (59). Furthermore, this process can be disrupted by a poorly timed change in arousal state. Injection of norepinephrine into quail embryos in the absence of exposure to appropriate auditory stimulation resulted in disrupted preference for the familiar maternal call (60). This work suggests that normal social development depends on physiological systems that mediate arousal and attention in the appropriate social environments early in life.
I propose that neuroendocrine mechanisms are also prime candidates for mediating sensitive periods in development. Hormones, which are typically defined as long-distance chemical signals, act directly on the cellular processes of neurons, but they also affect more general physiological systems, such as arousal, gonadal state, and metabolic function. Hormones influence multiple tissues simultaneously and modulate physiological and developmental processes across a wide spatial and temporal distance (61). This enables organisms to simultaneously coordinate many tissues or recruit whole neural circuits for an important task (62). Indeed, hormonal signals can provide a functional link between otherwise unconnected neuronal populations (63).
Most of the developmental effects of hormones have been studied in the context of steroid hormones and sexual differentiation (64–68). The focus of this work has been on how the hormones directly affect cellular function and the connectivity of neural circuits. However, many neuroendocrine signals have the potential to play a role in the organization of the social brain specifically by altering learning processes. Glucocorticoids, sex steroids, and neuropeptides have all been shown to be involved in learning and memory, both directly and indirectly (69). Nevertheless, there remains a gap in our understanding the role that such signals play in influencing the outcome of development in the context of important social experiences. Furthermore, the diversity of social phenotypes both within and between species begs the question as to how the unique features of both the organism’s early social experiences, as well as evolved differences in their neuroendocrine function, support the evolution and development of novel social phenotypes. For the purposes of this review, I focus on vasotocin-family neuropeptides, but many of the general principles of my argument may apply to other neuroendocrine signals, as well.
Over the last several decades, much research effort has been devoted to vasotocin family of neuropeptides (i.e., nonapeptides), which includes [arginine vasotocin (AVT), found in non-mammals and likely the ancestral peptide] and its mammalian homolog AVP; and the OT-like peptides [isotocin (IT), found in fish, mesotocin (MT), found in lung fish and non-eutherian tetrapods, including birds; and OT, found in mammals] (70, 71). The nonapeptides derive from an evolutionarily ancient neuromodulator. In the earliest vertebrates, only one gene was present (AVT), but sometime after Agnatha (lampreys and hagfish) a gene duplication event led to the divergence of the vasotocin (AVT/AVP) and OT (IT/MT/OT) lineages (72). Although these two lineages differ in only a single amino acid, the nonapeptides appear to have evolved quite distinct functions.
This is because the nonapeptides coevolved with their receptors, which are classic G-protein coupled receptors. There are typically four receptor subtypes for the nonapeptides within each species: V1a, V1b, V2, and OT (VT4, VT1, VT2, and VT3, respectively, in birds). The amino acid sequences of each receptor subtypes are more similar to each other across species (~90%) than they are to different subtypes within a single species (~45%) (73). When binding to their receptors, nonapeptides can have a multitude of effects on neurons, including changes to gene transcription, recruitment of intracellular calcium, neuroprotective effects, and alterations to long-term potentiation mechanisms (74). The sequencing of the vertebrate nonapeptide receptor genes suggests that the core–ligand receptor interaction sites have remained remarkably conserved, while varying the intracellular components, and thus their downstream effects (75). Receptors for nonapeptides are distributed throughout the brain, but importantly, the distribution of each of the receptor subtypes can vary widely by species, sex, age, and social context (76–78).
The primary sources of nonapeptides are the AVP/OT cell groups of the supraoptic nucleus (SON) and paraventricular nucleus (PVN) nuclei of the hypothalamus, as well as smaller extra-hypothalamic accessory cell groups, including the medial amygdala (MeA), medial bed nucleus of the stria terminalis (BSTm), lateral septum (LS), olfactory bulb (OB), and suprachiasmatic nucleus (SCN) (79, 80). The production of AVT/AVP, particularly in from the extra-hypothalamic cell groups, is often sexually dimorphic (usually male greater than female), organized by sex steroids during development, and sensitive to changes in gonadal state (81–88).
In order to understand the modulatory role of AVT/AVP during development, we need to consider the sources of the peptide, the sites of action in the body, and the functional consequences. There are three primary physiological systems influenced by AVT/AVP. The first can be summarized as AVT/AVP’s involvement in vasoconstriction and water balance. AVT/AVP, when released by magnocellular neurons in the PVN and SON of the hypothalamus, is released into the posterior pituitary. From there, AVT/AVP enters general circulation where exerts antidiuretic effects throughout the body.
The second is AVT/AVP’s involvement in the stress response. AVT/AVP, when it is released from the anterior pituitary by parvocellular neurons in the PVN, is at the top of the hypothalamic–pituitary–adrenal (HPA) axis. The HPA axis regulates the physiological response to stressors, helping the body mobilize resources in response to challenges in its environment. AVT/AVP, along with corticotropic releasing factor (CRF) serves as a releasing hormone for adrenocorticotropin-releasing hormone (ACTH) from the anterior pituitary, which is the chemical signal that leads to the release of glucocorticoids from adrenal tissue (89). AVT/AVP is not itself the major releasing hormone for ACTH, but it plays a critical role by potentiating the biological activity of CRF (90). Parvocellular AVT/AVP neurons are highly responsive to stress (91). Acute stress increases the production of both CRF and AVT/AVP in the PVN (92, 93).
Finally, multiple cell groups in the brain contribute to the central pool of nonapeptides with highly diverse functional consequences. Some of the same neurons that project into the pituitary also send projections back into the brain. In addition, the extra-hypothalamic accessory cell groups, including the MeA, BSTm, LS, OB, and SCN, contribute to the central pool of peptides (79, 80). Each of the AVT/AVP cell groups has a different pattern of activity and neural release, which is ultimately a function of the kinds of computation those neurons perform (87). Variation in how the nonapeptides affect the interconnected set of brain nuclei known as the social behavior network and other brain regions is thought to underlie the multiple effects of AVT/AVP across species (94, 95). Indeed, nonapeptides have been implicated in species differences in many diverse social behavioral domains (76, 77, 96–104).
The vast majority of this comparative work, however, has focused on nonapeptide function in adulthood. We in fact understand remarkably little about how nonapeptides shape social behaviors during development, particularly those behaviors for which plasticity, flexibility, and learning are critical. The effect that nonapeptides have on social learning in each species is influenced by when they act relative to important social experiences and in what brain regions. Across the few vertebrate species in which it has been investigated, the sequence of nonapeptide cell group maturation appears to be conserved. The first AVT/AVP immunostaining is consistently found in the SON followed by the PVN (105–112). In tetrapods, this is followed by production in the extra-hypothalamic cell groups, such as the BSTm and MeA, which exhibit steroid hormone-mediated sexual dimorphism in AVT/AVP staining (81, 113–115).
The most detailed developmental work in the nonapeptide system comes from rodents, particularly the rat. In rats, the neurons of the SON and PVN have formed before birth by 12–14 days postfertilization (dpf), gestation is 21 days in the rat (108). In the rat brain, the first AVP staining is observed between 14–18 dpf, which steadily increases to adult levels by postnatal day 30 (108, 113). Between birth and postnatal day 21, there is a 22- to 30-fold increase in AVP production by the pituitary, suggesting that the neurons that project from the hypothalamus to the pituitary are gradually coming on-line during development. By contrast, the cell groups of the BSTm and MeA show AVP staining only after birth, with the MeA delayed relative to the BSTm. AVP mRNA was only observed in the BSTm on postnatal day 3 and in the MeA on day 5 in male rats and day 14 and day 35, respectively, in female rats (113). The levels of AVP, thus, reach adult levels by postnatal day 35 in the BSTm and day 60 in the MeA in both sexes (113).
A similar developmental trajectory is found in the domestic chicken, despite substantial evolutionary distance and its precocial development. AVT is observed early in development in the chicken embryo SON and PVN, as early as 6 dpf (109–112). AVT is detectable in the BTSm by 12 dpf, which increases until hatching at 17 dpf before dropping precipitously in days after hatching (114). AVT then increases gradually in males until 129 dpf (114). Thus, the onset of function of all the cell groups in the SON, PVN, and BTSm occur while the chicken is still in the egg.
Very limited information on the development of these cell groups exists from other species, but the sequential development of the hypothalamic cell groups appears similar. In humans, AVP is detectable in the SON and PVN at 77 and 91 dpf, respectively (107, 116). Thus, in humans, hypothalamic production of AVP begins before birth. Even in zebra fish, two cell groups in the rostral diencephalon and hypothalamic regions show AVT mRNA expression sequentially starting at 24 h postfertilization (106). The embryonic development of the nonapeptide system has not been explored in songbirds. An early paper that explored the development of the nonapeptide circuitry in canaries found that AVT was expressed in the PVN at 4 weeks, but no staining was observed in the BSTm or LS until later at 8–12 weeks of age (117).
Taken together, these data suggest that the relative timing of the onset of function of the AVT/AVP cell groups is, in fact, remarkably conserved throughout evolution. Furthermore, the sequence of general neurodevelopmental events (from neurogenesis to eye opening) is also very predictable (118). What changes more is the timing of birth or hatching and, thus, early social experiences relative to these ontological changes. Inspired by Workman et al., Figure 1 depicts the development of the nonapeptide system scaled according to when the brain reaches 20% total brain volume, a milestone that is highly correlated with other neurodevelopmental events (118). This is based on data from three species for which we have some information about the development of the nonapeptide system: rats, humans (SON and PVN only), and chickens. The fact that neurodevelopmental events occur in a highly stereotyped sequence allows us to make predictions about the maturation of the nonapeptide system for cell groups or other species for which we do not have data, as well. Figure 1 shows the predicted timing of AVT synthesis in the respective cell groups in the zebra finch brain, based on general data on zebra finch neurodevelopment. Data supporting the figure can be found in Tables 1 and 2.
Figure 1. Comparative timeline of arginine vasotocin (AVT)/arginine vasopressin (AVP) cell group development. Conceptual timeline illustrating the production of AVT/AVP across early development in rats, humans, chickens, and zebra finches in three main AVT/AVP cell groups: the supraoptic nucleus (SON, blue), paraventricular nucleus (PVN) of the hypothalamus (PVN, orange), and the medial bed nucleus of the stria terminalis (BSTm, green). The x-axis of the timeline is scaled to the neurodevelopmental time point of when the organism is estimated to reach 20% total adult brain volume (indicated by the gray dotted line intersecting each timeline). Days postfertilization (dpf) are indicated by hatchmarks along each timeline. Solid lines indicate solid data (see text for references), whereas dotted lines are predicted results. The data from which this illustration is created include counts of AVT/AVP immunoreactive cells, mRNA expression, and peptide concentrations (see Table 1). Thus, the y-axis does not have a scale, as it is not clear how these different data types are comparable across species, cell group, study, etc. The date of hatching or birth for each species is indicated by a red rectangle. Date of eye opening is indicated by an asterisk.
Table 1. Earliest day postfertilization of arginine vasotocin (AVT)/arginine vasopressin labeling found.
Table 2. Data are presented for 20% brain volume, eye opening, amygdala neurogenesis peak, and the date of birth or hatching.
A few notes of caution are required. First, it is important to note that these predictions are still quite speculative, given the available data. The Workman et al. model has not been applied to avian systems, so the extent to which we can extrapolate the findings to chickens and zebra finches is still unknown. More information about when key neurodevelopmental events occur in the zebra finch brain may also help to determine whether the maturation of the AVT system would be more similar to the altricial but distantly related rat or the precocial but more closely related chicken.
Second, Figure 1 was created using available data, which is a mix of immunohistochemistry (IHC) labeling of AVT or AVP protein and in situ hybridization (ISH) labeling AVT/AVP gene expression (see Table 1). It is also based on labeling of these in the cell bodies within the respective brain regions. AVT/AVP is first synthesized as a large protein precursor molecule, which is enzymatically cleaved into the active hormone (80, 128). The active hormone must then be packaged into specialized neurosecretory vesicles and transported to the nerve terminals where it is released. Thus, the presence of either ISH or IHC labeling in the cell bodies is not a definitive indicator that the hormone is being released, particularly during development (105).
Third, we have limited information about where nonapeptides are acting during development. Binding sites have been found in the developing mouse and rat brain in both the amygdala and septum between postnatal days 0 and 8, as well as several brain regions where AVP receptors are not expressed in adulthood, including the hippocampus, dentate gyrus, and caudate nucleus (129, 130). In rats, many brain regions also show significant differences in between juveniles and adult (131). The consequences of these brain-region specific changes in receptor expression across development are almost certainly important, but have proven difficult to explore experimentally.
Finally, sex differences in both AVT/AVP production and receptor expression also likely influence developmental processes. For example, there is a delay in AVT/AVP synthesis in females relative to males in the BSTm and MeA (113, 115). Given these differences, we might predict that males would be more affected by AVT/AVP manipulations or by manipulations earlier in development, as compared to females. Some sex differences in receptor expression have also been found, but we have a poor understanding of the functional consequences of these differences.
Nevertheless, manipulations of nonapeptides in rodents very early in life provide evidence that nonapeptides matter in development (6, 7). For example, vasopressin–deficient Brattleboro rat pups show hyperactivity, reduced huddling, and reduced proximity to other pups in the nest compared to wild-type rats (132). Wild-type rat pups treated with a nine-day exposure to AVP showed increased emotionality, activity levels, and grooming in an open field test as juveniles, as well as smaller overall brain size (133). Acute central administration of AVP in wild-type neonatal rat pups was found to decrease the number of ultrasonic vocalizations and reduced locomotor activity in a maternal isolation test (134). In juvenile male rats, both targeted infusion of AVP into the LS and intracerebroventricular infusion increased preference for investigating novel individuals, whereas a V1aR antagonist increased the preference for investigating familiar individuals (135). In addition, V1aR blockade in the LS increased social play behavior in males and decreased it in females, but only when it tested in a familiar environment (136, 137). Neonatal manipulation of AVT or OT in the socially monogamous prairie vole, leads to sex-specific changes in nonapeptide binding in several brain regions in adults and alterations to social behaviors (138–141). For example, sexually naïve males who were treated with AVP early in life were more aggressive than control males but females were less responsive to AVP treatment (140). It is unclear whether the developmental effects of these nonapeptide manipulations are mediated through physiological effects on the body versus binding within the brain. Nevertheless, these studies provide intriguing evidence that the nonapeptides are involved in the development of social behavior.
Several researchers have proposed that one way to understand the outsize role of neuropeptides such as AVT/AVP in the evolution of behavioral diversity is through studying the differential expression of the peptides and their receptors (142, 143). The evolution of neuropeptide signaling systems may be highly constrained within the nervous system because mutations altering the proteins themselves would have deleterious effects on the receptor–ligand interactions. However, tweaks to where and in what quantities the peptides and their receptors are expressed via changes to gene regulation provide opportunities to modify the activity of neural circuits (142). Indeed, a number of examples have been identified. For example, variation in the production of AVT has been associated with species differences in social behaviors in birds (144). The number of IT-producing cells in the POA has been associated with cooperative breeding in cichlid fish (103). And the expression of V1aR is associated with variation in mating behaviors in voles (76, 145, 146). However, perhaps an important mechanism underlying this behavioral diversity across species is not just where but also when nonapeptides are acting during development.
Much of the research for the role of nonapeptides in social behavior has focused on brain regions with more generalized roles in social processing. However, the “compartmentalization” of AVT/AVP function can extend to include the modulation of species-specific behavioral circuits throughout the central nervous system (142, 147). In their sensorimotor hypothesis, Rose and Moore posit that AVT/AVP can act on sensory pathways to modulate the responsiveness of neurons to particular kinds of sensory stimuli as well as act on motor pathways to modulate behavioral output (147). In this case, the nonapeptide signal is directly modulating the specific circuits that are necessary for the production of the behavior. This theory emerged from comparative work which suggests that AVT modulates the activity of neurons in each step of a sensorimotor processing circuit which controls a complex courtship behavior in male newts (Taricha granulosa) (147). In these newts, AVT enhances the highly stereotyped sexual behavior, in which the male embraces the female with all four limbs to induce receptivity (148). AVT enhances this behavior by modulating sensory processing in the visual and olfactory domains as well as motor output at the level of the spinal cord (149–151).
A parallel story may also be true in the case of complex learned behaviors, such as bird song. Interestingly, there is limited evidence that anterior forebrain song learning pathway is sensitive to AVT, at least in adults AVT (88, 152–154). However, each step in the circuit controlling the expression of vocal behavior in birds appears to be partially modulated by AVT (152, 154, 155). Several auditory structures in the forebrain, including the caudomedial mesopallium and the caudomedial nidopallium, highly express V1aR in zebra finches (154). The robust nucleus of the arcopallium (RA, homologous to laryngeal motor cortex) exhibits limited receptor expression, but two nuclei involved in the motor pathway of song production contain AVT receptors. There is AVT immunoreactivity and binding in the intercollicular nucleus (ICo, a region implicated in vocal control) in several songbird species (152, 153, 156, 157). In addition, the key motor nucleus, nXIIts, which innervates the syrinx and is considered part of the song system, contains high levels of mRNA for all three subtypes of AVT receptor (154). However, a sensorimotor account of the role played by these regions during song learning remains to be tested.
Evolutionary novelty in behavior may also arise when new structures or circuits are modulated in new ways starting early in development. Syal and Finlay claim that what is necessary for the evolution of novel behaviors is changes to how the sensory and motor circuits are attached to the socio-motivational circuitry during early social interactions with caregivers, family, and conspecifics (158). In fact, they view the reciprocally connected network of brain nuclei known as the social behavior network (which includes the major nonapeptide cell groups), as the conserved neural structure that assembles the relevant sensory dimensions of a representation of other individuals (i.e., caregivers, mating partners, rivals) and attaches that representation to motivations and actions appropriate to their social context (158). The social behavior network is highly connected to the mesolimbic reward system via the BSTm, MeA, and LS, which all contain nonapeptide cell groups. Other structures commonly associated with reward and motivation, such as the ventral tegmental area, ventral pallidum, and nucleus accumbens, also densely express receptors for nonapeptides (159).
In this context, the modulatory signal produced by the nonapeptide cell groups, by acting on receptors throughout the brain, can be used to bias attention toward certain kinds of sensory stimuli or to reward the performance of certain behaviors. Consequently, it is easy to imagine how even tiny tweaks to the system, such as gene mutations that change the regulation of a receptor gene or slightly alter its downstream functions, might have large effects on whole neural circuits. Thus, the nonapeptide system may provide a mechanism whereby evolution generates novel social behavior using an otherwise highly conserved brain.
The effect of changes to the nonapeptide system would, thus, be expected to be even more consequential in development, particularly when coupled with salient social experiences. If indeed nonapeptides are gating social learning, then the nonapeptides may function by biasing a young organisms’ attention toward the behaviors exhibited by their family or other socially relevant conspecifics. For example, a primary reason why the development of the nonapeptide system may underlie important social development is because of its central role in olfactory processing. Early social experiences are often highly olfactory and thermotactile in nature. For example, suckling behavior in rat pups is dependent upon odor processing in the accessory olfactory system and MeA, which allows them to learn the odor of their mother’s amniotic fluid and of the saliva of their broodmates to guide nipple attachment (160). Even zebra finches, which do not have an accessory olfactory system and which are thought to be more responsive to auditory and visual stimuli, show olfactory preferences for their natal nest (161). Thus, early olfactory experiences provide some of the first forms of social learning about conspecifics at the same time that the relevant behavioral circuits begin responding to nonapeptides.
Increased attention to relevant social stimuli would provide opportunities for social learning, which could also be reinforced by socio-motivational circuits. Early sensitivity to social stimuli would support future social learning, leading to accumulating effects. On the other hand, genetic mutations that reduce social approach or attention during development might reduce the probability that predictable aspects of the social environment are learned at all. Of course, these kinds of effects depend critically on the kinds of input that an organism receives from its environment during these social interactions. It is possible to think of the organizational effects of nonapeptide circuitry independent of its social environment, but more likely, the kinds of social experiences an organism has—and their sensitivity to those social experience—coevolved with each other. Neuropeptide systems during development may have, thus, evolved to allow organisms to plastically respond to their environment as they mature. However, by allowing for variable outcomes in adulthood, these evolvable systems also provide the raw material for evolution to act.
Recent experimental evidence from manipulating the nonapeptide system early in life in zebra finch provides support for the idea nonapeptides play an organizational role in on a broad suite of social behaviors (162–164). Intracranial injections of either AVT or [Manning Compound (MC), a V1aR antagonist] in hatchlings (days 2–8 post-hatching) altered social interest in the parents and conspecifics after fledging, suggesting that the nonapeptides are serving to gate a number of social approach behaviors in juvenile zebra finches (162). In addition, early life nonapeptide treatment also altered affiliative behaviors and courtship song in adult male, but not female, zebra finches. Both AVT and Control males showed an increased affiliative interest in females as they reached reproductive maturity (162). However, AVT-treated males showed less sexually motivated courting of females compared to Controls and instead formed highly affiliative pair bonds with their female partner (163). By contrast, MC males did not show the normal increase in affiliative interest in females as they reached maturity and showed only modest levels of both courtship and affiliation in their interactions with females (162, 163). Furthermore, nonapeptide treatment also altered neural activity and the expression of V1aR in the BSTm and MeA (163). Taken together, these findings suggest that AVT-injected males may have had more experience attending to social cues or a stronger association between affiliative interactions and reward compared to both MC and Control males, resulting in different approaches to reproduction.
This change in the affiliative interest in parents and conspecifics also had functional consequences for social learning. Male zebra finches injected with MC as hatchlings both showed decreased interest in their parents during development and ultimately sang a song that was a worse acoustic match to their father’s song in adulthood compared to Controls (162, 164). By contrast, AVT males showed increased affiliative interest in their parents and family and more effectively copy their father’s song (162, 164). Interestingly, affiliation with parents at 30 days post-hatch was correlated with song quality in adulthood. These data suggest that the nonapeptides may bias the motivation of developing zebra finches to attend to the behaviors of the father during development, which ultimately allows them to more accurately learn courtship song from their father. This is ultimately consistent with Syal and Finlay’s hypothesis that the nonapeptides gate complex vocal learning in song birds by altering social motivation, supporting their suggestion that the nonapeptides may play an equally critical role in language learning in humans (158).
Thus, social phenotypes may evolve via relatively simple alterations to the actions of a single nonapeptide during development. In zebra finches, AVT altered early social behaviors, potentially affecting the opportunities for social learning. However, it also affected the organization of the neural substrate underlying these social behaviors. It will likely prove impossible to disentangle the direct effects of nonapeptides on the brain during development from their indirect effects resulting from how they alter the trajectory of learning from early social experiences. Indeed, this conceptual challenge is at the heart of the nature “versus” nurture debate (4).
Nevertheless, these results provide support for the idea that the actions of nonapeptides in development may play an important role in the evolution of novel social behavior. The field of evolutionary developmental biology (evo-devo) has long been concerned with how evolution shapes developmental processes to generate phenotypic novelty. However, the insights from evo-devo have rarely expanded into the social domain (165–167). Neuropeptides and hormonal systems are well-situated to play that role, given that they alter the activities of whole neural circuits. However, we are just scratching the surface in our understanding of the diversity of mechanisms which may facilitate the evolution and development of social behaviors.
Indeed, the nonapeptides are almost certainly not the only chemicals that play a role in the evolution of diverse social phenotypes. We now know of more than 100 different peptides and other signaling molecules, each of which is expressed in only a small population of neurons, and all of which signal to neurons throughout the brain via specific receptors. The endless forms of neural systems and behavior appear to be result of evolutionary changes to compartmentalization of neuropeptide signaling systems (142). However, the complex nature of diverse signaling systems suggests that they can only be fully understood by integrating research at all levels of analysis—investigating both their molecular and developmental mechanisms, as well as their adaptive significance in the life of an organism.
NB wrote the article as part of her dissertation work at Cornell University.
The author declares that the research was conducted in the absence of any commercial or financial relationships that could be construed as a potential conflict of interest.
I am grateful for the feedback of E. Adkins-Regan and M. H. Goldstein on this manuscript, as well as their support and insight throughout my graduate research.
This research was supported by an NSF Doctoral Dissertation Improvement Grant (NSF, IOS – 1310908); NSF, IOS – 1146891; and NIH Training Grant 5T32HD055177-05.
1. Stiles J. The Fundamentals of Brain Development: Integrating Nature and Nurture. 1sr ed. Cambridge, MA: Harvard University Press (2008).
2. Oyama S, Lewontin R. The Ontogeny of Information: Developmental Systems and Evolution. 2nd ed. Durham, NC: Duke University Press Books (2000).
3. Lickliter R, Honeycutt H. Developmental dynamics: toward a biologically plausible evolutionary psychology. Psychol Bull (2003) 129:819–35. doi:10.1037/0033-2909.129.6.819
4. Lehrman DS. Semantic and conceptual issues in the nature-nurture problem. In: Aronson LR, Tobach E, Lehrman DS, Rosenblatt JS, editors. Development and Evolution of Behavior. San Francisco, CA: W.H.Freeman and Company (2012). p. 17–52.
5. Gottlieb G. Experiential canalization of behavioral development: theory. Dev Psychol (1991) 27:4–13. doi:10.1037/0012-1649.27.1.4
6. Cushing BS. The organizational effects of oxytocin and vasopressin. In: Choleris E, Pfaff DW, Kavaliers M, editors. Oxytocin, Vasopressin and Related Peptides in the Regulation of Behavior. Cambridge, UK: Cambridge University Press (2013). p. 56–72.
7. Miller TV, Caldwell HK. Oxytocin during development: possible organizational effects on behavior. Front Endocrinol (2015) 6:76. doi:10.3389/fendo.2015.00076
8. Boer GJ. Vasopressin and brain development: studies using the Brattleboro rat. Peptides (1985) 6:49–62. doi:10.1016/0196-9781(85)90011-7
9. Noonan LR, Continella G, Pedersen CA. Neonatal administration of oxytocin increases novelty-induced grooming in the adult rat. Pharmacol Biochem Behav (1989) 33:555–8. doi:10.1016/0091-3057(89)90386-9
10. Carter CS, Boone EM, Pournajafi-Nazarloo H, Bales KL. Consequences of early experiences and exposure to oxytocin and vasopressin are sexually dimorphic. Dev Neurosci (2009) 31:332–41. doi:10.1159/000216544
11. Kenkel WM, Yee JR, Carter CS. Is oxytocin a maternal-foetal signalling molecule at birth? Implications for development. J Neuroendocrinol (2014) 26:739–49. doi:10.1111/jne.12186
12. Insel TR. The challenge of translation in social neuroscience: a review of oxytocin, vasopressin, and affiliative behavior. Neuron (2010) 65:768–79. doi:10.1016/j.neuron.2010.03.005
13. Bornstein MH, editor. Sensitive Periods in Development: Interdisciplinary Perspectives. Hillsdale, NJ: Lawrence Erlbaum Associates, Inc. (1987).
14. Bornstein MH. Sensitive periods in development: structural characteristics and causal interpretations. Psychol Bull (1989) 105:179–97. doi:10.1037/0033-2909.105.2.179
15. Wiesel TN, Hubel DH. Effects of visual deprivation on morphology and physiology of cells in the cat’s lateral geniculate body. J Neurophysiol (1963) 26:978–93.
16. McMillen IC, Robinson JS. Developmental origins of the metabolic syndrome: prediction, plasticity, and programming. Physiol Rev (2005) 85:571–633. doi:10.1152/physrev.00053.2003
17. Immelmann K. Sexual and other long-term aspects of imprinting in birds and other species. Adv Study Behav (1972) 4:147–74. doi:10.1016/S0065-3454(08)60009-1
18. Bolhuis JJ. Mechanisms of avian imprinting: a review. Biol Rev Camb Philos Soc (1991) 66:303–45. doi:10.1111/j.1469-185X.1991.tb01145.x
19. Burley NT. An eye for detail: selective sexual imprinting in zebra finches. Evolution (2006) 60:1076–85. doi:10.1111/j.0014-3820.2006.tb01184.x
21. ten Cate C, Vos DR. Sexual imprinting and evolutionary processes in birds: a reassessment. In: Peter JSR, Slater JB, editors. Advances in the Study of Behavior. San Diego, CA: Academic Press (1999). p. 1–31.
22. Vos DR, Prijs J, ten Cate C. Sexual imprinting in zebra finch males: a differential effect of successive and simultaneous experience with two colour morphs. Behaviour (1993) 126:137–54. doi:10.2307/4535128
23. Witte K, Caspers B. Sexual imprinting on a novel blue ornament in zebra finches. Behaviour (2006) 143:969–91. doi:10.2307/4536389
24. Horn G. Visual imprinting and the neural mechanisms of recognition memory. Trends Neurosci (1998) 21:300–5. doi:10.1016/S0166-2236(97)01219-8
25. Knudsen E. Sensitive periods in the development of the brain and behavior. J Cogn Neurosci (2004) 16:1412–25. doi:10.1162/0898929042304796
26. Nakamori T, Maekawa F, Sato K, Tanaka K, Ohki-Hamazaki H. Neural basis of imprinting behavior in chicks. Dev Growth Differ (2013) 55:198–206. doi:10.1111/dgd.12028
27. Barnett C. Rearing conditions affect chemosensory preferences in young cichlid fish. Ethology (1986) 72:227–35. doi:10.1111/j.1439-0310.1986.tb00623.x
28. Gerlach G, Hodgins-Davis A, Avolio C, Schunter C. Kin recognition in zebrafish: a 24-hour window for olfactory imprinting. Proc R Soc Lond B Biol Sci (2008) 275:2165–70. doi:10.1098/rspb.2008.0647
29. Gerlach G, Lysiak N. Kin recognition and inbreeding avoidance in zebrafish, Danio rerio, is based on phenotype matching. Anim Behav (2006) 71:1371–7. doi:10.1016/j.anbehav.2005.10.010
30. Hasler AD, Scholz AT, Horrall RM. Olfactory imprinting and homing in salmon: recent experiments in which salmon have been artificially imprinted to a synthetic chemical verify the olfactory hypothesis for salmon homing. Am Sci (1978) 66:347–55.
31. Keller-Costa T, Canário AVM, Hubbard PC. Chemical communication in cichlids: a mini-review. Gen Comp Endocrinol (2015) 221:64–74. doi:10.1016/j.ygcen.2015.01.001
32. Kozak GM, Head ML, Boughman JW. Sexual imprinting on ecologically divergent traits leads to sexual isolation in sticklebacks. Proc R Soc Lond B Biol Sci (2011) 278:2604–10. doi:10.1098/rspb.2010.2466
33. Mann KD, Turnell ER, Atema J, Gerlach G. Kin recognition in juvenile zebrafish (Danio rerio) based on olfactory cues. Biol Bull (2003) 205:224–5. doi:10.2307/1543264
34. Verzijden MN, ten Cate C. Early learning influences species assortative mating preferences in Lake Victoria cichlid fish. Biol Lett (2007) 3:134–6. doi:10.1098/rsbl.2006.0601
35. Ainsworth MS. Attachments beyond infancy. Am Psychol (1989) 44:709–16. doi:10.1037/0003-066X.44.4.709
36. Bowlby J. Separation anxiety: a critical review of the literature. J Child Psychol Psychiatry (1960) 1:251–69. doi:10.1111/j.1469-7610.1960.tb01999.x
37. Champagne FA, Francis DD, Mar A, Meaney MJ. Variations in maternal care in the rat as a mediating influence for the effects of environment on development. Physiol Behav (2003) 79:359–71. doi:10.1016/S0031-9384(03)00149-5
38. Zayas V, Mischel W, Shoda Y, Aber JL. Roots of adult attachment: maternal caregiving at 18 months predicts adult peer and partner attachment. Soc Psychol Personal Sci (2011) 2:289–97. doi:10.1177/1948550610389822
39. Bertin A, Richard-Yris M-A. Mothering during early development influences subsequent emotional and social behaviour in Japanese quail. J Exp Zoolog A Comp Exp Biol (2005) 303A:792–801. doi:10.1002/jez.a.202
40. Harlow HF, Dodsworth RO, Harlow MK. Total social isolation in monkeys. Proc Natl Acad Sci U S A (1965) 54:90–7. doi:10.1073/pnas.54.1.90
41. Lévy F, Melo AI, Galef BG, Madden M, Fleming AS. Complete maternal deprivation affects social, but not spatial, learning in adult rats. Dev Psychobiol (2003) 43:177–91. doi:10.1002/dev.10131
42. Rutter M. Developmental catch-up, and deficit, following adoption after severe global early privation. J Child Psychol Psychiatry (1998) 39:465–76. doi:10.1111/1469-7610.00343
43. Liu D, Diorio J, Tannenbaum B, Caldji C, Francis D, Freedman A, et al. Maternal care, hippocampal glucocorticoid receptors, and hypothalamic-pituitary-adrenal responses to stress. Science (1997) 277:1659–62. doi:10.1126/science.277.5332.1659
44. Meaney MJ. Maternal care, gene expression, and the transmission of individual differences in stress reactivity across generations. Annu Rev Neurosci (2001) 24:1161–92. doi:10.1146/annurev.neuro.24.1.1161
45. Goldstein MH, Schwade JA. From birds to words. In: Blumberg MS, Freeman JH, Robinson SR, editors. Oxford Handbook of Developmental Behavioral Neuroscience. Oxford University Press (2014). p. 708–29. Available from: http://www.oxfordhandbooks.com/view/10.1093/oxfordhb/9780195314731.001.0001/oxfordhb-9780195314731-e-035
46. Lipkind D, Marcus GF, Bemis DK, Sasahara K, Jacoby N, Takahasi M, et al. Stepwise acquisition of vocal combinatorial capacity in songbirds and human infants. Nature (2013) 498:104–8. doi:10.1038/nature12173
47. Bateson P, Hinde RA. Developmental changes in sensitivity to experience. In: Bornstein MH, editor. Sensitive Periods in Development: Interdisciplinary Perspectives. Hillsdale, NJ: Lawrence Erlbaum Associates, Inc. (1987). p. 19–38.
48. Yamaguchi S, Aoki N, Kitajima T, Iikubo E, Katagiri S, Matsushima T, et al. Thyroid hormone determines the start of the sensitive period of imprinting and primes later learning. Nat Commun (2012) 3:1081. doi:10.1038/ncomms2088
50. Gottlieb G. Ontogenesis of sensory function in birds and mammals. In: Tobach E, Aronson LR, Shaw E, editors. The Biopsychology of Development. New York, NY: Academic Press (1971). p. 67–128.
51. Turkewitz G, Kenny PA. Limitations on input as a basis for neural organization and perceptual development: a preliminary theoretical statement. Dev Psychobiol (1982) 15:357–68. doi:10.1002/dev.420150408
52. Lickliter R. Prenatal sensory ecology and experience: implications for perceptual and behavioral development in precocial birds. In: Slater PJB, Snowdon CT, Roper TJ, Brockmann HJ, Naguib M, editors. Advances in the Study of Behavior. New York, NY: Academic Press (2005). p. 235–74. doi:10.1016/S0065-3454(05)35006-6
53. Gottlieb G. Synthesizing Nature–Nurture: Prenatal Roots of Instinctive Behavior. Mahwah, NJ: Lawrence Erlbaum Associates, Inc (1997).
54. McBride TC, Lickliter R. Social experience with siblings fosters species specific responsiveness to maternal visual cues in bobwhite quail chicks (Colinus virginianus). J Comp Psychol (1993) 107:320–7. doi:10.1037/0735-7036.107.3.320
55. Blass EM. Critical events during sensitive periods in social development in rats. In: Bornstein MH, editor. Sensitive Periods in Development: Interdisciplinary Perspectives. Hillsdale, NJ: Lawrence Erlbaum Associates, Inc. (1987). p. 81–98.
56. Lewkowicz DJ, Turkewitz G. Cross-modal equivalence in early infancy: auditory–visual intensity matching. Dev Psychol (1980) 16:597–607. doi:10.1037/0012-1649.16.6.597
57. Gardner JM, Karmel BZ. Development of arousal-modulated visual preferences in early infancy. Dev Psychol (1995) 31:473–82. doi:10.1037/0012-1649.31.3.473
58. Kaplan PS, Bachorowski J-A, Smoski MJ, Hudenko WJ. Infants of depressed mothers, although competent learners, fail to learn in response to their own mothers’ infant-directed speech. Psychol Sci (2002) 13:268–71. doi:10.1111/1467-9280.00449
59. Pedersen PE, Williams CL, Blass EM. Activation and odor conditioning of suckling behavior in 3-day-old albino rats. J Exp Psychol Anim Behav Process (1982) 8:329–41. doi:10.1037/0097-7403.8.4.329
60. Markham RG, Toth G, Lickliter R. Prenatally elevated physiological arousal interferes with perceptual learning in bobwhite quail (Colinus virginianus) embryos. Behav Neurosci (2006) 120:1315–25. doi:10.1037/0735-7044.120.6.1315
61. Ketterson ED, Atwell JW, McGlothlin JW. Phenotypic integration and independence: hormones, performance, and response to environmental change. Integr Comp Biol (2009) 49:365–79. doi:10.1093/icb/icp057
62. Adkins-Regan E. Hormones and Animal Social Behavior. Princeton, NJ: Princeton University Press (2005).
63. Baran NM, McGrath PT, Streelman JT. Applying gene regulatory network logic to the evolution of social behavior. Proc Natl Acad Sci U S A (2017) 114:5886–93. doi:10.1073/pnas.1610621114
64. Phoenix CH, Goy RW, Gerall AA, Young WC. Organizing action of prenatally administered testosterone propionate on the tissues mediating mating behavior in the female guinea pig. Endocrinology (1959) 65:369–82. doi:10.1210/endo-65-3-369
65. Adkins EK. Embryonic exposure to an antiestrogen masculinizes behavior of female quail. Physiol Behav (1976) 17:357–9. doi:10.1016/0031-9384(76)90088-3
66. Adkins-Regan E, Pickett P, Koutnik D. Sexual differentiation in quail: conversion of androgen to estrogen mediates testosterone-induced demasculinization of copulation but not other male characteristics. Horm Behav (1982) 16:259–78. doi:10.1016/0018-506X(82)90026-5
67. Adkins-Regan E. Hormones and sexual differentiation. In: Norris DO, Jones RE, editors. Hormones and Reproduction in Fishes, Amphibians, and Reptiles. Springer US (1987). p. 1–29. Available from: http://link.springer.com/chapter/10.1007/978-1-4613-1869-9_1
68. Balthazart J, Adkins-Regan E. 66 – sexual differentiation of brain and behavior in birds. In: Pfaff DW, Arnold AP, Fahrbach SE, Etgen AM, Rubin RT, editors. Hormones, Brain and Behavior. San Diego: Academic Press (2009). p. 223–301.
69. Martinez JL Jr, Meilandt WJ, Peng H, Barea-Rodriguez EJ. Hormones, learning and memory. Encyclopedia of Cognitive Science. John Wiley & Sons, Ltd (2006). Available from: http://onlinelibrary.wiley.com/doi/10.1002/0470018860.s00442/abstract
70. Acher R, Chauvet J, Chauvet M. Phylogeny of the neurohypophysial hormones. Eur J Biochem (1970) 17:509–13. doi:10.1111/j.1432-1033.1970.tb01193.x
71. Acher R, Chauvet J, Chauvet M-T. Phylogeny of the neurohypophysial hormones. Eur J Biochem (1972) 29:12–9. doi:10.1111/j.1432-1033.1972.tb01951.x
72. Moore FL, Lowry CA. Comparative neuroanatomy of vasotocin and vasopressin in amphibians and other vertebrates. Comp Biochem Physiol C Pharmacol Toxicol Endocrinol (1998) 119:251–60. doi:10.1016/S0742-8413(98)00014-0
73. Darlison MG, Richter D. Multiple genes for neuropeptides and their receptors: co-evolution and physiology. Trends Neurosci (1999) 22:81–8. doi:10.1016/S0166-2236(98)01333-2
74. Neumann ID, van den Burg EH. Oxytocin and vasopressin release and their receptor-mediated intracellular pathways that determine their behavioral effects. In: Choleris E, Pfaff DW, Kavaliers M, editors. Oxytocin, Vasopressin and Related Peptides in the Regulation of Behavior. Cambridge, UK: Cambridge University Press (2013). p. 27–43.
75. Cho HJ, Acharjee S, Moon MJ, Oh DY, Vaudry H, Kwon HB, et al. Molecular evolution of neuropeptide receptors with regard to maintaining high affinity to their authentic ligands. Gen Comp Endocrinol (2007) 153:98–107. doi:10.1016/j.ygcen.2006.12.013
76. Lim MM, Wang Z, Olazábal DE, Ren X, Terwilliger EF, Young LJ. Enhanced partner preference in a promiscuous species by manipulating the expression of a single gene. Nature (2004) 429:754–7. doi:10.1038/nature02539
77. Insel TR, Wang ZX, Ferris CF. Patterns of brain vasopressin receptor distribution associated with social organization in microtine rodents. J Neurosci (1994) 14:5381–92.
78. Goodson JL, Evans AK, Lindberg L, Allen CD. Neuro-evolutionary patterning of sociality. Proc R Soc B Biol Sci (2005) 272:227–35. doi:10.1098/rspb.2004.2892
79. Choleris E, Pfaff DW, Kavaliers M, editors. Oxytocin, Vasopressin and Related Peptides in the Regulation of Behavior. Cambridge, UK: Cambridge University Press (2013).
81. De Vries GJ, Al-Shamma HA. Sex differences in hormonal responses of vasopressin pathways in the rat brain. J Neurobiol (1990) 21:686–93. doi:10.1002/neu.480210503
82. De Vries GJ, Buijs RM. The origin of the vasopressinergic and oxytocinergic innervation of the rat brain with special reference to the lateral septum. Brain Res (1983) 273:307–17. doi:10.1016/0006-8993(83)90855-7
83. De Vries GJ, Panzica GC. Sexual differentiation of central vasopressin and vasotocin systems in vertebrates: different mechanisms, similar endpoints. Neuroscience (2006) 138:947–55. doi:10.1016/j.neuroscience.2005.07.050
84. Goodson JL, Bass AH. Social behavior functions and related anatomical characteristics of vasotocin/vasopressin systems in vertebrates. Brain Res Rev (2001) 35:246–65. doi:10.1016/S0165-0173(01)00043-1
85. Goodson JL, Thompson RR. Nonapeptide mechanisms of social cognition, behavior and species-specific social systems. Curr Opin Neurobiol (2010) 20:784–94. doi:10.1016/j.conb.2010.08.020
86. Kabelik D, Kelly AM, Goodson JL. Dopaminergic regulation of mate competition aggression and aromatase-Fos colocalization in vasotocin neurons. Neuropharmacology (2010) 58:117–25. doi:10.1016/j.neuropharm.2009.06.009
87. Kelly AM, Goodson JL. Social functions of individual vasopressin–oxytocin cell groups in vertebrates: what do we really know? Front Neuroendocrinol (2014) 35:512–29. doi:10.1016/j.yfrne.2014.04.005
88. Kimura T, Okanoya K, Wada M. Effect of testosterone on the distribution of vasotocin immunoreactivity in the brain of the zebra finch, Taeniopygia guttata castanotis. Life Sci (1999) 65:1663–70. doi:10.1016/S0024-3205(99)00415-4
89. Buckingham J. Understanding the role of vasopressin in the hypothalamo-pituitary adrenocortical axis. In: Laycock JF, editor. Perspectives on Vasopressin. London: Imperial College Press (2009). p. 230–56.
90. Gillies G, Lowry PJ. Corticotropin-releasing hormone and its vasopressin component. Front Neuroendocrinol (1982) 7:45–75.
91. Shibata M, Fujihara H, Suzuki H, Ozawa H, Kawata M, Dayanithi G, et al. Physiological studies of stress responses in the hypothalamus of vasopressin-enhanced green fluorescent protein transgenic rat. J Neuroendocrinol (2007) 19:285–92. doi:10.1111/j.1365-2826.2007.01532.x
92. Harbuz MS, Jessop DS, Lightman SL, Chowdrey HS. The effects of restraint or hypertonic saline stress on corticotrophin-releasing factor, arginine vasopressin, and proenkephalin A mRNAs in the CFY, Sprague-Dawley and Wistar strains of rat. Brain Res (1994) 667:6–12. doi:10.1016/0006-8993(94)91707-8
93. Xia Y, Krukoff TL. Differential neuronal activation in the hypothalamic paraventricular nucleus and autonomic/neuroendocrine responses to I.C.V. endotoxin. Neuroscience (2003) 121:219–31. doi:10.1016/S0306-4522(03)00290-2
94. Newman SW. The medial extended amygdala in male reproductive behavior: a node in the mammalian social behavior network. Ann N Y Acad Sci (1999) 877:242–57. doi:10.1111/j.1749-6632.1999.tb09271.x
95. Goodson JL. The vertebrate social behavior network: evolutionary themes and variations. Horm Behav (2005) 48:11–22. doi:10.1016/j.yhbeh.2005.02.003
96. Campbell P, Ophir AG, Phelps SM. Central vasopressin and oxytocin receptor distributions in two species of singing mice. J Comp Neurol (2009) 516:321–33. doi:10.1002/cne.22116
97. Dewan AK, Ramey ML, Tricas TC. Arginine vasotocin neuronal phenotypes, telencephalic fiber varicosities, and social behavior in butterflyfishes (Chaetodontidae): potential similarities to birds and mammals. Horm Behav (2011) 59:56–66. doi:10.1016/j.yhbeh.2010.10.002
98. Dewan AK, Maruska KP, Tricas TC. Arginine vasotocin neuronal phenotypes among congeneric territorial and shoaling reef butterflyfishes: species, sex and reproductive season comparisons. J Neuroendocrinol (2008) 20:1382–94. doi:10.1111/j.1365-2826.2008.01798.x
99. Goodson JL, Kabelik D, Schrock SE. Dynamic neuromodulation of aggression by vasotocin: influence of social context and social phenotype in territorial songbirds. Biol Lett (2009) 5:554–6. doi:10.1098/rsbl.2009.0316
100. Landgraf R, Frank E, Aldag JM, Neumann ID, Sharer CA, Ren X, et al. Viral vector-mediated gene transfer of the vole V1a vasopressin receptor in the rat septum: improved social discrimination and active social behaviour. Eur J Neurosci (2003) 18:403–11. doi:10.1046/j.1460-9568.2003.02750.x
101. Oldfield RG, Harris RM, Hendrickson DA, Hofmann HA. Arginine vasotocin and androgen pathways are associated with mating system variation in North American cichlid fishes. Horm Behav (2013) 64:44–52. doi:10.1016/j.yhbeh.2013.04.006
102. Parker KJ, Lee TM. Central vasopressin administration regulates the onset of facultative paternal behavior in Microtus pennsylvanicus (meadow voles). Horm Behav (2001) 39:285–94. doi:10.1006/hbeh.2001.1655
103. Reddon AR, O’Connor CM, Marsh-Rollo SE, Balshine S, Gozdowska M, Kulczykowska E. Brain nonapeptide levels are related to social status and affiliative behaviour in a cooperatively breeding cichlid fish. R Soc Open Sci (2015) 2:140072. doi:10.1098/rsos.140072
104. Wang Z, Ferris CF, Vries GJD. Role of septal vasopressin innervation in paternal behavior in prairie voles (Microtus ochrogaster). Proc Natl Acad Sci U S A (1994) 91:400–4. doi:10.1073/pnas.91.1.400
105. Boer GJ. Development of vasopressin systems and their functions. In: Gash DM, Boer GJ, editors. Vasopressin. Springer US (1987). p. 117–74. Available from: http://link.springer.com/chapter/10.1007/978-1-4615-8129-1_3
106. Eaton JL, Holmqvist B, Glasgow E. Ontogeny of vasotocin-expressing cells in zebrafish: selective requirement for the transcriptional regulators orthopedia and single-minded 1 in the preoptic area. Dev Dyn (2008) 237:995–1005. doi:10.1002/dvdy.21503
107. Swaab DF. Development of the human hypothalamus. Neurochem Res (1995) 20:509–19. doi:10.1007/BF01694533
108. Buijs RM, Velis DN, Swaab DF. Ontogeny of vasopressin and oxytocin in the fetal rat: early vasopressinergic innervation of the fetal brain. Peptides (1980) 1:315–24. doi:10.1016/0196-9781(80)90009-1
109. Arnold-Aldea SA, Sterritt C. Sites of origin and patterns of migration of vasotocin/mesotocin neurons in developing brain of the chick. J Neurobiol (1996) 31:103–16. doi:10.1002/(SICI)1097-4695(199609)31:1<103:AID-NEU9>3.0.CO;2-6
110. Tennyson VM, Nilaver G, Hou-Yu A, Valiquette G, Zimmerman EA. Immunocytochemical study of the development of vasotocin/mesotocin in the hypothalamo-hypophysial system of the chick embryo. Cell Tissue Res (1986) 243:15–31. doi:10.1007/BF00221848
111. Milewski N, Lvell R, Grossmann R, Ellendorff F. Embryonal development of arginine vasotocin/mesotocin gene expression in the chicken brain. J Neuroendocrinol (1989) 1:473–84. doi:10.1111/j.1365-2826.1989.tb00149.x
112. Mühlbauer E, Hamannt D, Xu B, Ivell R, Udovic B, Ellendorff F, et al. Arginine vasotocin gene expression and hormone synthesis during ontogeny of the chicken embryo and the newborn chick. J Neuroendocrinol (1993) 5:281–8. doi:10.1111/j.1365-2826.1993.tb00484.x
113. Szot P, Dorsa DM. Differential timing and sexual dimorphism in the expression of the vasopressin gene in the developing rat brain. Brain Res Dev Brain Res (1993) 73:177–83. doi:10.1016/0165-3806(93)90136-X
114. Jurkevich A, Barth SW, Kuenzel WJ, Köhler A, Grossmann R. Development of sexually dimorphic vasotocinergic system in the bed nucleus of stria terminalis in chickens. J Comp Neurol (1999) 408:46–60. doi:10.1002/(SICI)1096-9861(19990524)408:1<46::AID-CNE4>3.0.CO;2-5
115. Jurkevich A, Grossmann R, Balthazart J, Viglietti-Panzica C. Gender-related changes in the avian vasotocin system during ontogeny. Microsc Res Tech (2001) 55:27–36. doi:10.1002/jemt.1153
116. Fellmann D, Bloch B, Bugnon C, Lenys D. Etude immunocytologique de la maturation des axes neuroglandulaires hypothalamo-neurohypophysaires chez le foetus humain. J Physiol Paris (1979) 75:37–43.
117. Voorhuis TAM, De Kloet ER, De Wied D. Ontogenetic and seasonal changes in immunoreactive vasotocin in the canary brain. Brain Res Dev Brain Res (1991) 61:23–31. doi:10.1016/0165-3806(91)90110-5
118. Workman AD, Charvet CJ, Clancy B, Darlington RB, Finlay BL. Modeling transformations of neurodevelopmental sequences across mammalian species. J Neurosci (2013) 33:7368–83. doi:10.1523/JNEUROSCI.5746-12.2013
119. Buijs RM. Intra- and extrahypothalamic vasopressin and oxytocin pathways in the rat. Cell Tissue Res (1978) 192:423–35. doi:10.1007/BF00212323
120. Almazan G, Lefebvre DL, Zingg HH. Ontogeny of hypothalamic vasopressin, oxytocin and somatostatin gene expression. Brain Res Dev Brain Res (1989) 45:69–75. doi:10.1016/0165-3806(89)90008-4
121. Clancy B, Teague-Ross TJ, Nagarajan R. Cross-species analyses of the cortical GABAergic and subplate neural populations. Front Neuroanat (2009) 3:20. doi:10.3389/neuro.05.020.2009
122. Clancy B, Darlington RB, Finlay BL. Translating developmental time across mammalian species. Neuroscience (2001) 105:7–17. doi:10.1016/S0306-4522(01)00171-3
123. Darlington RB, Dunlop SA, Finlay BL. Neural development in metatherian and eutherian mammals: variation and constraint. J Comp Neurol (1999) 411:359–68. doi:10.1002/(SICI)1096-9861(19990830)411:3<359:AID-CNE1>3.0.CO;2-J
124. Kawabe S, Matsuda S, Tsunekawa N, Endo H. Ontogenetic shape change in the chicken brain: implications for paleontology. PLoS One (2015) 10:e0129939. doi:10.1371/journal.pone.0129939
125. Vergara MN, Canto-Soler MV. Rediscovering the chick embryo as a model to study retinal development. Neural Dev (2012) 7:22. doi:10.1186/1749-8104-7-22
126. Ikebuchi M, Nanbu S, Okanoya K, Suzuki R, Bischof H-J. Very early development of nucleus taeniae of the amygdala. Brain Behav Evol (2013) 81:12–26. doi:10.1159/000342785
127. Bischof H-J, Lassek R. The gaping reaction and the development of fear in young zebra finches (Taeniopygia guttata castanotis). Z Für Tierpsychol (1985) 69:55–65. doi:10.1111/j.1439-0310.1985.tb00756.x
128. Burbach JPH, Luckman SM, Murphy D, Gainer H. Gene Regulation in the magnocellular hypothalamo-neurohypophysial system. Physiol Rev (2001) 81:1197–267.
129. Hammock EAD, Law CS, Levitt P. Vasopressin eliminates the expression of familiar odor bias in neonatal female mice through V1aR. Horm Behav (2013) 63:352–60. doi:10.1016/j.yhbeh.2012.12.006
130. Petracca FM, Baskin DG, Diaz J, Dorsa DM. Ontogenetic changes in vasopressin binding site distribution in rat brain: an autoradiographic study. Brain Res (1986) 28:63–8. doi:10.1016/0165-3806(86)90065-9
131. Smith CJW, Poehlmann ML, Li S, Ratnaseelan AM, Bredewold R, Veenema AH. Age and sex differences in oxytocin and vasopressin V1a receptor binding densities in the rat brain: focus on the social decision-making network. Brain Struct Funct (2017) 222:981–1006. doi:10.1007/s00429-016-1260-7
132. Schank JC. Early locomotor and social effects in vasopressin deficient neonatal rats. Behav Brain Res (2009) 197:166–77. doi:10.1016/j.bbr.2008.08.019
133. Boer GJ, Quak J, de Vries MC, Heinsbroek RPW. Mild sustained effects of neonatal vasopressin and oxytocin treatment on brain growth and behavior of the rat. Peptides (1994) 15:229–36. doi:10.1016/0196-9781(94)90007-8
134. Winslow JT, Insel TR. Effects of central vasopressin administration to infant rats. Eur J Pharmacol (1993) 233:101–7. doi:10.1016/0014-2999(93)90354-K
135. Veenema AH, Bredewold R, De Vries GJ. Vasopressin regulates social recognition in juvenile and adult rats of both sexes, but in sex- and age-specific ways. Horm Behav (2012) 61:50–6. doi:10.1016/j.yhbeh.2011.10.002
136. Bredewold R, Smith CJW, Dumais KM, Veenema AH. Sex-specific modulation of juvenile social play behavior by vasopressin and oxytocin depends on social context. Front Behav Neurosci (2014) 8:216. doi:10.3389/fnbeh.2014.00216
137. Veenema AH, Bredewold R, De Vries GJ. Sex-specific modulation of juvenile social play by vasopressin. Psychoneuroendocrinology (2013) 38:2554–61. doi:10.1016/j.psyneuen.2013.06.002
138. Bales KL, Carter CS. Sex differences and developmental effects of oxytocin on aggression and social behavior in prairie voles (Microtus ochrogaster). Horm Behav (2003) 44:178–84. doi:10.1016/S0018-506X(03)00154-5
139. Bales KL, Plotsky PM, Young LJ, Lim MM, Grotte N, Ferrer E, et al. Neonatal oxytocin manipulations have long-lasting, sexually dimorphic effects on vasopressin receptors. Neuroscience (2007) 144:38–45. doi:10.1016/j.neuroscience.2006.09.009
140. Stribley JM, Carter CS. Developmental exposure to vasopressin increases aggression in adult prairie voles. Proc Natl Acad Sci U S A (1999) 96:12601–4. doi:10.1073/pnas.96.22.12601
141. Yamamoto Y, Cushing B, Kramer K, Epperson P, Hoffman G, Carter C. Neonatal manipulations of oxytocin alter expression of oxytocin and vasopressin immunoreactive cells in the paraventricular nucleus of the hypothalamus in a gender-specific manner. Neuroscience (2004) 125:947–55. doi:10.1016/j.neuroscience.2004.02.028
142. Katz PS, Lillvis JL. Reconciling the deep homology of neuromodulation with the evolution of behavior. Curr Opin Neurobiol (2014) 29:39–47. doi:10.1016/j.conb.2014.05.002
143. Goodson JL. Nonapeptides and the evolutionary patterning of sociality. In: Neumann ID, Landgraf R, editors. Progress in Brain Research Advances in Vasopressin and Oxytocin – From Genes to Behaviour to Disease. Elsevier (2008). p. 3–15. Available from: http://www.sciencedirect.com/science/article/pii/S0079612308004019
144. Goodson JL, Kelly AM, Kingsbury MA. Evolving nonapeptide mechanisms of gregariousness and social diversity in birds. Horm Behav (2012) 61(3):239–50. doi:10.1016/j.yhbeh.2012.01.005
145. Lim MM, Young LJ. Vasopressin-dependent neural circuits underlying pair bond formation in the monogamous prairie vole. Neuroscience (2004) 125:35–45. doi:10.1016/j.neuroscience.2003.12.008
146. Okhovat M, Berrio A, Wallace G, Ophir AG, Phelps SM. Sexual fidelity trade-offs promote regulatory variation in the prairie vole brain. Science (2015) 350:1371–4. doi:10.1126/science.aac5791
147. Rose JD, Moore FL. Behavioral neuroendocrinology of vasotocin and vasopressin and the sensorimotor processing hypothesis. Front Neuroendocrinol (2002) 23:317–41. doi:10.1016/S0091-3022(02)00004-3
148. Moore FL, Zoeller RT. Endocrine control of amphibian sexual behavior: evidence for a neurohormone-androgen interaction. Horm Behav (1979) 13:207–13. doi:10.1016/0018-506X(79)90038-2
149. Boyd SK, Moore FL. Gonadectomy reduces the concentrations of putative receptors for arginine vasotocin in the brain of an amphibian. Brain Res (1991) 541:193–7. doi:10.1016/0006-8993(91)91018-V
150. Lowry CA, Richardson CF, Zoeller TR, Miller LJ, Muske LE, Moore FL. Neuroanatomical distribution of vasotocin in a urodele amphibian (Taricha granulosa) revealed by immunohistochemical and in situ hybridization techniques. J Comp Neurol (1997) 385:43–70. doi:10.1002/(SICI)1096-9861(19970818)385:1<43:AID-CNE3>3.0.CO;2-C
151. Thompson RR, Moore FL. Vasotocin stimulates appetitive responses to the visual and pheromonal stimuli used by male roughskin newts during courtship. Horm Behav (2000) 38:75–85. doi:10.1006/hbeh.2000.1610
152. Leung CH, Goode CT, Young LJ, Maney DL. Neural distribution of nonapeptide binding sites in two species of songbird. J Comp Neurol (2009) 513:197–208. doi:10.1002/cne.21947
153. Voorhuis TAM, De Kloet ER. Immunoreactive vasotocin in the zebra finch brain (Taeniopygia guttata). Brain Res Dev Brain Res (1992) 69:1–10. doi:10.1016/0165-3806(92)90116-E
154. Leung CH, Abebe DF, Earp SE, Goode CT, Grozhik AV, Mididoddi P, et al. Neural distribution of vasotocin receptor mRNA in two species of songbird. Endocrinology (2011) 152:4865–81. doi:10.1210/en.2011-1394
155. Nottebohm F, Stokes TM, Leonard CM. Central control of song in the canary, Serinus canarius. J Comp Neurol (1976) 165:457–86. doi:10.1002/cne.901650405
156. Kiss JZ, Voorhuis TA, Van Eekelen JAM, De Kloet ER, De Wied D. Organization of vasotocin-immunoreactive cells and fibers in the canary brain. J Comp Neurol (1987) 263:347–64. doi:10.1002/cne.902630304
157. Panzica GC, Plumari L, García-Ojeda E, Deviche P. Central vasotocin-immunoreactive system in a male passerine bird (Junco hyemalis). J Comp Neurol (1999) 409:105–17. doi:10.1002/(SICI)1096-9861(19990621)409:1<105:AID-CNE8>3.0.CO;2-8
158. Syal S, Finlay BL. Thinking outside the cortex: social motivation in the evolution and development of language. Dev Sci (2011) 14:417–30. doi:10.1111/j.1467-7687.2010.00997.x
159. O’Connell LA, Hofmann HA. The vertebrate mesolimbic reward system and social behavior network: a comparative synthesis. J Comp Neurol (2011) 519:3599–639. doi:10.1002/cne.22735
160. Pedersen PE, Stewart WB, Greer CA, Shepherd GM. Evidence for olfactory function in utero. Science (1983) 221:478–80. doi:10.1126/science.6867725
161. Caspers BA, Krause ET. Odour-based natal nest recognition in the zebra finch (Taeniopygia guttata), a colony-breeding songbird. Biol Lett (2011) 7:184–6. doi:10.1098/rsbl.2010.0775
162. Baran NM, Sklar NC, Adkins-Regan E. Developmental effects of vasotocin and nonapeptide receptors on early social attachment and affiliative behavior in the zebra finch. Horm Behav (2016) 78:20–31. doi:10.1016/j.yhbeh.2015.10.005
163. Baran NM, Tomaszycki ML, Adkins-Regan E. Early life manipulations of the nonapeptide system alter pair maintenance behaviors and neural activity in adult male zebra finches. Front Behav Neurosci (2016) 10:58. doi:10.3389/fnbeh.2016.00058
164. Baran NM, Peck SC, Kim TH, Goldstein MH, Adkins-Regan E. Early life manipulations of vasopressin-family peptides alter vocal learning. Proc R Soc B Biol Sci (2017) 284. doi:10.1098/rspb.2017.1114
165. Finlay BL. Endless minds most beautiful. Dev Sci (2007) 10:30–4. doi:10.1111/j.1467-7687.2007.00560.x
166. Hofmann HA. Early developmental patterning sets the stage for brain evolution. Proc Natl Acad Sci U S A (2010) 107:9919–20. doi:10.1073/pnas.1005137107
Keywords: evo-devo, vasopressin, nonapeptides, sensitive periods, social behavior, vocal learning, developmental psychobiology
Citation: Baran NM (2017) Sensitive Periods, Vasotocin-Family Peptides, and the Evolution and Development of Social Behavior. Front. Endocrinol. 8:189. doi: 10.3389/fendo.2017.00189
Received: 16 April 2017; Accepted: 19 July 2017;
Published: 02 August 2017
Edited by:
Aras Petrulis, Georgia State University, United StatesReviewed by:
Bice Chini, Consiglio Nazionale Delle Ricerche (CNR), ItalyCopyright: © 2017 Baran. This is an open-access article distributed under the terms of the Creative Commons Attribution License (CC BY). The use, distribution or reproduction in other forums is permitted, provided the original author(s) or licensor are credited and that the original publication in this journal is cited, in accordance with accepted academic practice. No use, distribution or reproduction is permitted which does not comply with these terms.
*Correspondence: Nicole M. Baran, bmljb2xlLmJhcmFuQGJpb2xvZ3kuZ2F0ZWNoLmVkdQ==
Disclaimer: All claims expressed in this article are solely those of the authors and do not necessarily represent those of their affiliated organizations, or those of the publisher, the editors and the reviewers. Any product that may be evaluated in this article or claim that may be made by its manufacturer is not guaranteed or endorsed by the publisher.
Research integrity at Frontiers
Learn more about the work of our research integrity team to safeguard the quality of each article we publish.