- Department of Biological Sciences, North Carolina State University, Raleigh, NC, USA
Leptin is a pleiotropic hormone that plays a critical role in regulating appetite, energy metabolism, growth, stress, and immune function across vertebrate groups. In mammals, it has been classically described as an adipostat, relaying information regarding energy status to the brain. While retaining poor sequence conservation with mammalian leptins, teleostean leptins elicit a number of similar regulatory properties, although current evidence suggests that it does not function as an adipostat in this group of vertebrates. Teleostean leptin also exhibits functionally divergent properties, however, possibly playing a role in glucoregulation similar to what is observed in lizards. Further, leptin has been recently implicated as a mediator of immune function and the endocrine stress response in teleosts. Here, we provide a review of leptin physiology in vertebrates, with a particular focus on its actions and regulatory properties in the context of stress and the regulation of energy homeostasis.
Introduction
Leptin is a class I helical cytokine encoded by the obese gene (ob) that has typically been characterized as an adipostat, circulating in proportion to the quantity of white adipose tissue and relaying information regarding the energy status of the animal to the central nervous system (1, 2). In mammals, leptin is pleiotropic, regulating a multitude of physiological processes including appetite, lipid metabolism, growth, reproduction, stress, and immune function [reviewed in Ref. (3)]. The function of leptin has been less extensively studied in non-mammalian vertebrates; however, there is growing evidence in teleosts that leptin may play a greater role as a glucoregulatory hormone than an adipostat in this group of vertebrates. Studies on the interactions between leptin and the stress axis as well as the immune system, however, suggest that some of the actions of leptin may be conserved between fish and mammals despite the low sequence conservation between these two groups. Here, we provide an overview of what is known about the role of leptin in regulating energy homeostasis and the stress response in teleost fishes and compare this to the known effects of leptin in mammals and other vertebrate groups.
Leptin Characterization, Distribution, and Signaling
Orthology in Vertebrates
Leptin was first cloned in the mouse by Zhang et al. (1) and has since been identified in all extant vertebrate groups examined to date. Following the discovery of leptin in the mouse, orthologs were identified in several other mammalian species (4); however, attempts to isolate a putative leptin sequence in non-mammalian vertebrates were largely unsuccessful. It was not until 2005, over a decade after its discovery in mammals, that a leptin homolog was cloned in a non-mammalian species, the Japanese pufferfish [Takifugu rubripes (5)]. This delay was due to the low amino acid identity (often less than 30%) between vertebrate leptin sequences (6) (Figure 1). The deduced primary structure of the pufferfish leptin (pLep) shared only 13.2% identity with human leptin; however, three-dimensional modeling suggested a strong conservation of tertiary structure with mammalian leptins, as pLep also possesses four α-helices (5). Further, the amino acid sequence of pLep contained two cysteine residues to form the disulfide bridge between α-helices C and D, a highly conserved element of vertebrate leptins (5).
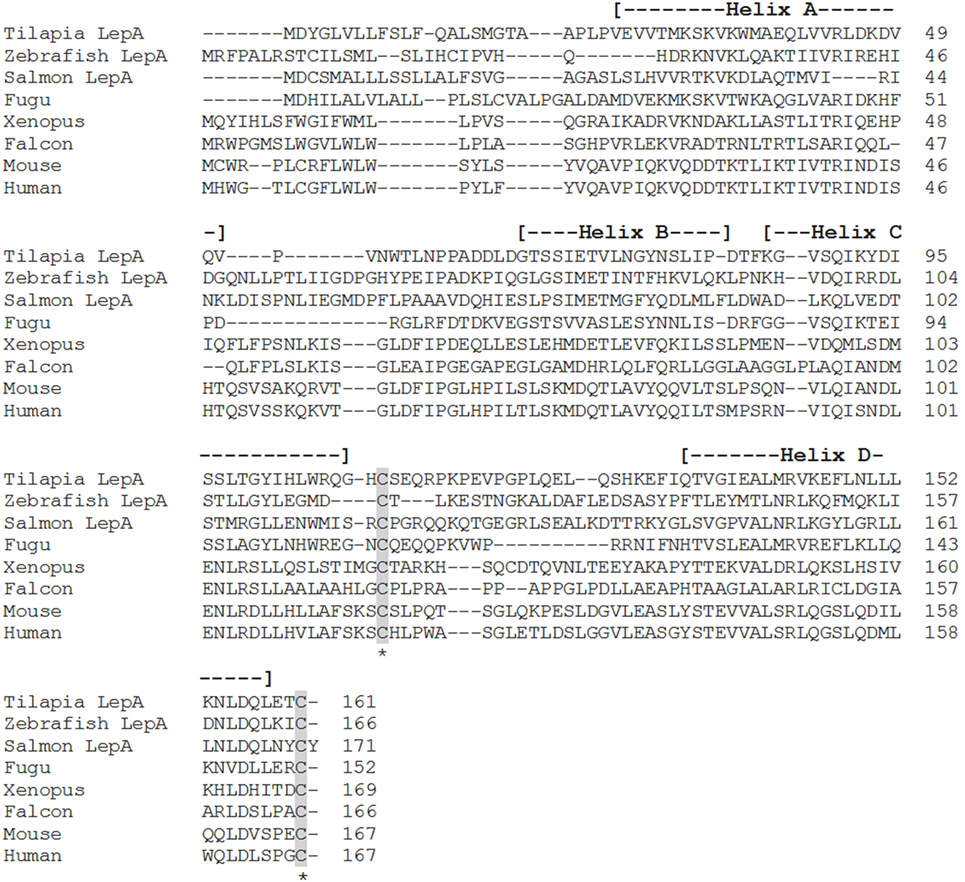
Figure 1. Alignment of teleost leptin A (LepA) with the leptin homologs from other vertebrate classes. Accession numbers: tilapia LepA, AHL37667.1; zebrafish LepA, NP_001025357.2; salmon LepA, ACZ02412.1; fugu, NP_001027897.1; Xenopus, NP_001089183.1; falcon, NP_001298279.1; mouse, NP_032519.1; human, NP_000221.1. Shaded areas represent the conserved cysteine residues required for the formation of the disulfide bridge. The four alpha-helices are indicated by dashed lines within the parentheses.
Shortly after the identification of pLep, a leptin homolog was cloned in an amphibian, Xenopus laevis, that shared 35 and 13% amino acid identity with human and pLeps, respectively (7) (Figure 1). Putative leptin sequences have also been identified in the tiger salamander [Ambystoma tigrinum (8)] and in the green Anole lizard [Anolis carolinensis (9)], both of which show low amino acid identity to human leptin. In teleosts, leptin orthologs have now been characterized in striped bass [Morone saxatilis (10)], common carp [Cyprinus carpio (11)], rainbow trout [Oncorhynchus mykiss (12)], zebrafish [Danio rerio (13)], Atlantic salmon [Salmo salar (14)], orange-spotted grouper [Epinephelus coioides (15)], Japanese medaka [Oryzias latipes (13, 16)], yellow catfish [Pelteobagrus fulvidraco (17)], Nile tilapia [Oreochromis niloticus (18)], Jian carp [C. carpio var. Jian (19)], Arctic charr [Salvelinus alpinus (20)], grass carp [Ctenopharyngodon idella (21)], silver carp [Hypophthalmichthys molitrix (21)], chub mackerel [Scomber japonicus (22)], mandarin fish [Siniperca chuatsi (23)], and white-clouds mountain minnow [Tanichthys albonubes (24)]. These teleost leptins all have low sequence conservation with mammals, varying from 13 to 25% amino acid identity (Figures 1 and 2); however, each one is composed of two exons separated by a short intron, contains the cysteine residues required for formation of the disulfide bridge, and is predicted to have retained the four-helix tertiary structure characteristic of mammalian leptins. Even within the teleost lineage, there is often low amino acid identity between species (<50%), unless the species are closely related, such as within the salmonids or cyprinids.
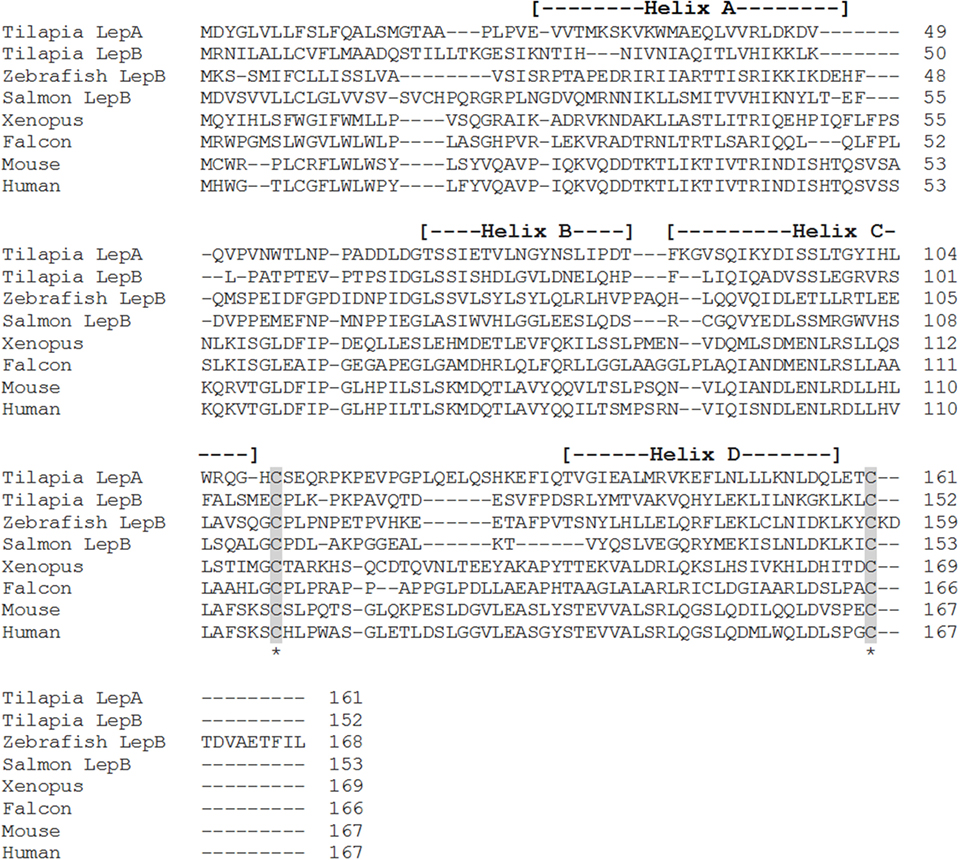
Figure 2. Alignment of teleost leptin B (LepB) with the leptin homologs from other vertebrate classes, tilapia leptin A (LepA), has been included for comparison. Accession numbers: tilapia LepA, AHL37667.1; tilapia LepB, AHL37668.1; salmon LepB, NP_001266063.1; zebrafish LepB, NP_001025357.2; Xenopus, NP_001089183.1; falcon, NP_001298279.1; mouse, NP_032519.1; human, NP_000221.1. Shaded areas represent the conserved cysteine residues required for the formation of the disulfide bridge. The four alpha-helices are indicated by dashed lines within the parentheses.
Paralogs in Teleosts
In certain teleost species, two leptin paralogs have been identified. This is a common feature of teleostean class I cytokines, resulting from the genome duplication that occurred in the teleost lineage (6, 25). Zebrafish (D. rerio), Japanese medaka (O. latipes), orange-spotted grouper (E. coioides), Nile tilapia (O. niloticus), Mozambique tilapia (Oreochromis mossambicus), chub mackerel (S. japonicus), mandarin fish (S. chuatsi), and white-clouds mountain minnow (T. albonubes) have all been shown to possess two separate leptin proteins, leptin A (LepA) and leptin B (LepB) (13, 15, 18, 19, 22–24, 26) (Figures 1 and 2). The amino acid identity between LepA and LepB within each species is low, ranging from 18 to 30%, and phylogenetic analysis shows that the two genes form separate branches (18, 19, 24, 27). Due to the additional genome duplications that occurred within the salmonid and cyprinid lineages, a number of species including the common carp (C. carpio), Atlantic salmon (S. salar), goldfish (Carassius auratus), rainbow trout (O. mykiss), and Jian carp (C. carpio var Jian) possess up to four leptin paralogs, two LepA genes, and either one or two LepB genes (11, 14, 19, 28, 29). The two LepA sequences and the two LepB sequences in these species share higher amino acid identities than is seen between the A and B forms (ranging from 71 to 83%); thus, the nomenclature typically used is leptin A1 and A2 and leptin B1 and B2 (13, 14, 28, 29).
Tissue Distribution in Teleosts
Unlike in mammals where leptin is produced predominantly in adipose tissue, teleost leptins often have the highest mRNA expression levels in liver, with most species having low or non-existent leptin expression in adipose tissue. Other sites of expression in teleosts are the brain, gonads, muscle, and kidney; however, this can vary widely between species (10–24, 26). In some instances, the tissue distribution between paralogs within a single species differs, and it has been suggested that lepa is more prominent in the liver, while lepb is predominantly expressed in the gonads, thus indicating divergent roles of the two paralogs (13, 29). However, studies on LepB are limited, and this differential expression pattern is not consistent across species, with most showing substantial overlap in the tissue expression patterns for the two forms. Regardless, lepa appears to be the predominantly expressed form in most species examined (15, 16, 24) showing 10–100 times greater tissue mRNA copy number than lepb and hence likely reflecting the major source of circulating leptin (26).
Receptor and Signaling Pathways
The leptin receptor (LepR) is part of the glycoprotein 130 family of cytokine receptors, which utilize gp130 as a signal transducer to activate signaling pathways within the cell, typically the Janus kinase/signal transducers and activators of transcription (JAK/STAT) pathway (30, 31). Signaling via this pathway has been observed in the pituitary of both mammals and frogs, suggesting conservation of this signaling mechanism for leptin across vertebrate groups (30–32). Although sharing low identity with mammalian receptors (<30%), teleost LepRs show genomic synteny with the human receptor and possess the functionally important JAK- and STAT-binding domains that are largely conserved within vertebrates (14–18, 33–35). In teleosts, lepr mRNA is ubiquitously expressed, with higher levels typically being observed in the pituitary, hypothalamus, and gonads, suggesting that these are prominent sites of leptin action (14–18, 28, 33–35). Indeed, leptin regulates glucose sensing in the hypothalamus and hindbrain of rainbow trout (O. mykiss) both in vitro and in vivo (36, 37). These actions were attenuated when leptin was administered in combination with either a phosphoinositide-3-kinase or JAK2 inhibitor, indicating involvement of these pathways in leptin signaling (36, 37). Further evidence for leptin signaling via the JAK/STAT pathway comes from the increase in Akt and STAT3 phosphorylation observed in trout hypothalamic cells following incubation with leptin (38). The lipid regulatory activity of heterologous leptin on hepatocytes and ovarian follicular cells of yellow catfish (P. fulvidraco) is attenuated by JAK/STAT inhibitors, reiterating a role for this pathway in leptin signaling (39). Leptin has also been shown to act on the pituitary of tilapia (O. mossambicus) to stimulate prolactin (PRL) release through activation of the extracellular signal-related kinase (ERK) pathway (40) and on the liver of the hybrid striped bass [Morone chrysops x Morone saxatilis (41)] and Mozambique tilapia [O. mossambicus (42)] to regulate growth hormone (GH) receptors and insulin-like growth factors (IGFs), although the signaling pathways have yet to be determined. Albeit studies assessing the function of leptin in teleosts are limited, existing data suggest that the sites of leptin action and the signaling pathways responsible for eliciting its effects may be conserved with that of other vertebrate systems. Further investigations are required to elucidate the full complement of intracellular pathways mediating leptin action(s).
Leptin Energy Homeostatic Actions
Feeding
Leptin is renowned for its role in regulating food intake and body mass (43). Secreted primarily from adipose tissue in mammals, leptin serves as a lipostatic signal and conveys critical information regarding metabolic state to the brain (44, 45). As lipid stores accumulate and circulating leptin rises, the hormone enhances energy expenditure and reduces food intake by stimulating anorexic proopiomelanocortin/cocaine and amphetamine-related transcript neurons and inhibiting orexigenic neuropeptide Y/agouti-related protein neurons (46–50). Leptin-deficient pathologies are typically accompanied by hyperphagia and obesity [reviewed in Ref. (45, 49, 51)]. The anorexigenic properties of leptin have been well characterized in the context of leptin deficiency through experimental administration to obese, leptin-deficient ob/ob mice, as well as leptin-deficient humans, resulting in the reduction of food intake and body mass (52, 53).
In some fishes, leptin demonstrates a marked postprandial elevation [(54, 55); reviewed in Ref. (56, 57)] in accordance with the mammalian paradigm. Further, the administration of leptin via injection has been shown to reduce food intake in goldfish [C. auratus (58, 59)], rainbow trout [O. mykiss (12, 36)], grass carp [C. Idella (21)], Atlantic salmon [S. salar (60)], and striped bass [M. saxatilis (10)]. Properties similar to that of leptin-related pathologies initially observed in the db/db mouse have also been reported in a LepR-deficient medaka [O. latipes (61)]. This mutant line showed consistently elevated hypothalamic activity of orexigenic neuropeptides, suppression of anorexigenic neuropeptides, and increased food intake, suggesting a similar regulatory role for leptin in appetite suppression in fishes. While the anorexigenic properties of leptin would also suggest potentially concurrent lipostatic properties as seen in mammals, no changes in adiposity were observed in leptin receptor-deficient strains of zebrafish (62), and other species exhibit inconsistent correlations between fat deposition and leptin expression, e.g., during fasting leptin rises in fish as adiposity declines, while it declines with fasting and lipid stores in mammals (38, 42, 63–65). Nonetheless, the anorexigenic properties of leptin appear well conserved among vertebrates.
Metabolism
Leptin regulates energy availability in mammals by mobilizing lipid stores (66) and stimulating the oxidation of fatty acids (67). It also induces hypoglycemia by enhancing glucose uptake into peripheral tissues (68) and elevates metabolic rate in muscle and liver (69). Studies on the metabolic actions of leptin in other vertebrate classes are limited (Table 1) leading to difficulties in elucidating whether leptin evolved primarily as a lipolytic agent or if its basal metabolic functions are more glucoregulatory in nature. Teleosts appeared relatively early in the vertebrate lineage, and thus, understanding the role of leptin in regulating metabolic pathways in these fish could provide valuable insights into the evolution of energy homeostasis in vertebrates. The existing data in teleosts are equivocal, with lipolytic actions being reported in response to leptin treatment in some species, while in others, leptin instead stimulates glycogen depletion and increases plasma glucose (Table 2).
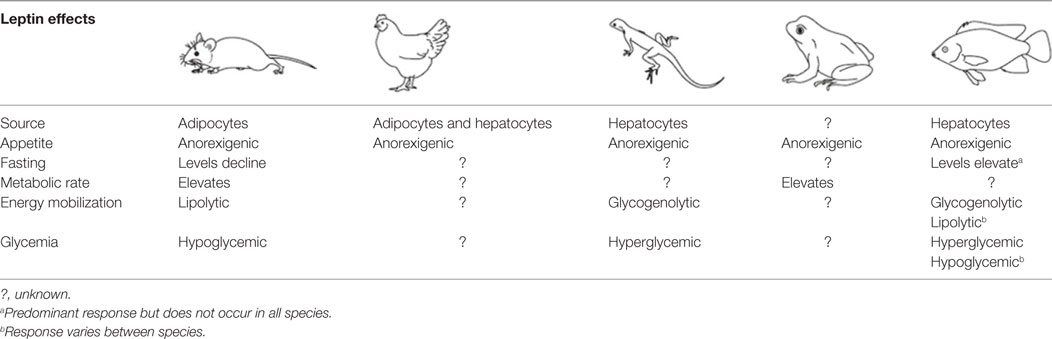
Table 1. Comparison of the source of leptin, response to fasting, and effects on appetite, energy metabolism, glycemia, and metabolic rate in the different vertebrate classes based on current knowledge.
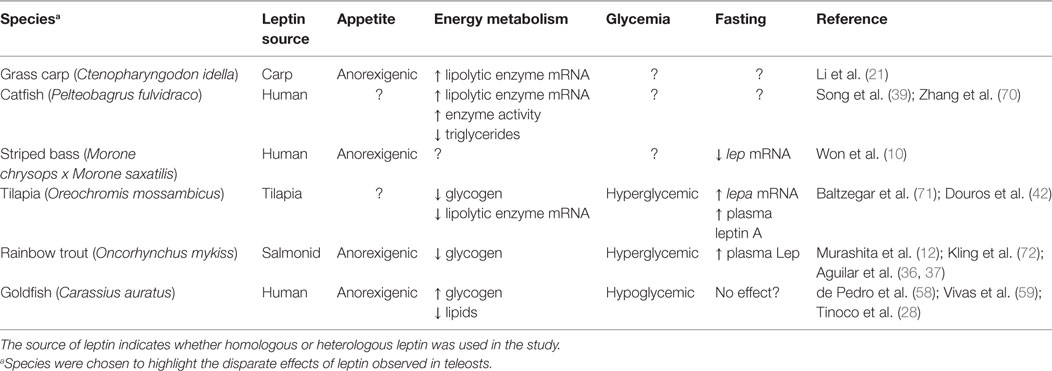
Table 2. Different effects of leptin on appetite, energy metabolism, and glycemia as well as the response to fasting in various teleost species.
Leptin actions appear to agree with the classic mammalian paradigm in grass carp (C. Idella), wherein it induces a decrease in the hepatic stearoyl-coA desaturase-1 mRNA, an enzyme involved in the synthesis of fatty acids, while simultaneously increasing the mRNA level of hormone-sensitive lipase (hsl) (21). Fatty acid levels were not measured in these studies; however, an overall effect on lipid regulation cannot be ascertained. Nonetheless, human leptin increases activity and mRNA levels of lipolytic enzymes in catfish (P. fulvidraco) hepatocytes and ovarian follicular cells, which paralleled a decrease in overall lipid content, suggesting a lipolytic action of the hormone (39, 70). Further, human leptin increased the mRNA levels of various lipolytic genes, decreased the levels of lipogenic genes, and decreased overall triglyceride content in hepatocytes of the goby [Synechogobius hasta (73)]. In contrast, the mRNA levels of hormone-sensitive lipase, as well as lipoprotein lipase (lpl), decreased in the liver of Mozambique tilapia (O. mossambicus) in response to homologous hormone treatment (71). The latter study also observed a decrease in hepatic glycogen content and corresponding increase in plasma glucose (71), suggesting that leptin has hyperglycemic actions in teleosts and thus may represent a functional divergence from mammalian leptins. This corroborates an earlier study in rainbow trout (O. mykiss) in which central administration of leptin also increased plasma glucose while concurrently reducing the glycogen content of the liver (36). Similar effects were observed in lizards, with leptin decreasing hepatic glycogen content and increasing plasma glucose levels (74). Disparate results have been reported in goldfish, however, with human leptin increasing muscle and liver glycogen while depleting liver lipids and lowering plasma glucose, similar to what is observed in mammals (58). The different actions of leptin reported in teleosts could be a function of differences in life history strategies or from using mammalian vs. homologous leptins. Baltzegar et al. (71) reported similar glucoregulatory effects for both recombinant human leptin and tilapia LepA. However, distinct actions on regulation of hepatic hsl and lpl were observed between the two, with tilapia LepA reducing and human leptin having little effect on the lipases, suggesting that the use of homologous hormone may be essential for determining species-specific effects.
Further glucoregulatory roles for leptin have been demonstrated in the brain of rainbow trout and tilapia. Aguilar et al. (36) demonstrated increases in the glucose and glycogen contents of the trout (O. mykiss) hypothalamus and hindbrain in response to an intracerebroventricular injection of human leptin, which were paralleled by increases in glut2 mRNA and glycogen synthase activity. Leptin also induced a significant increase in glucokinase activity in the brain (36), suggesting that one of the functions of leptin may be to stimulate glucose uptake and metabolism. In the pituitary rostral pars distalis of the tilapia (O. mossambicus), homologous leptin induced an increase in the activity of phosphofructokinase, a rate-limiting glycolytic enzyme, and this was correlated with an increase in lactate secretion or overall glycolytic output (75). Although typically believed to be a lipolytic agent, leptin has also been implicated in glucose metabolism in mammals, having been shown to stimulate glycolysis and gluconeogenesis and inhibit glycogenolysis [reviewed in Ref. (76)]. These data suggest that one of the basal functions of leptin may be to regulate glucose uptake and catabolism (e.g., glycolysis) in vertebrates; however, the source of glucose may vary as the hormone can elicit catabolic effects on either lipid or glycogen stores. One explanation for this could be the evolution of endothermy [see Ref. (77) for review of energetics between endothermy and ectothermy]. Mammals exhibit higher metabolic rates that, if fueled by fatty acids and/or glucose that has been synthesized de novo, would allow glycogen stores to be conserved in the event the animal is in need of a rapid source of energy. Hence, leptin may promote gluconeogenesis, but not glycogenolysis. Whether leptin alters gluconeogenic pathways in fish remains to be determined.
Leptin Integration with the Classical Endocrine Stress Axis
Endocrine Stress Response
It is apparent that leptin is a catabolic hormone in vertebrates that enhances energy mobilization and suppresses appetite, two processes often linked to stress responses. Hence, the hormone may be integral to the endocrine stress response. Stress impacts virtually all aspects of vertebrate physiology including immunity, reproduction, hydromineral balance, and energy homeostasis (78–80). The adrenergic (humoral and neuronal) and hypothalamic–pituitary–adrenal [interrenal in fish; HPA/hypothalamic-pituitary interrenal (HPI)] axes are central components of the vertebrate stress response and ultimately aid in restoration of homeostasis when disrupted. In all vertebrates, including teleost fishes, acute and chronic stress events are mediated through the sympathetic adrenergic and HPA/HPI axes, two primary components of the endocrine stress response. The two axes release catecholamines (epinephrine/norepinephrine) and glucocorticoids (cortisol/corticosterone), respectively, to allow for the mobilization of energy stores (79, 81, 82).
Upon the perception of a stressor, sympathetic nerve fibers release acetylcholine onto chromaffin cells within the adrenal medulla (mammals) or interrenal tissue (teleosts) to stimulate the secretion of catecholamines and allow for the rapid mobilization of energy stores from peripheral tissues (81, 83–85). Simultaneously, the hypothalamus releases corticotropin-releasing factor (CRF), which stimulates the release of adrenocorticotropic hormone (ACTH) from the pituitary. ACTH then triggers the production and release of glucocorticoids from the adrenal cortex (mammals) or interrenal cells of the head kidney (teleosts) (79, 80, 85). These glucocorticoids then elicit a myriad of metabolic effects such as inducing lipid and protein catabolism and stimulating gluconeogenesis to increase plasma glucose levels (79, 86). In a classic negative feedback pathway, the increase in circulating cortisol then inhibits further release of CRF and ACTH, attenuating the stress response.
Catecholamines and Leptin
Epinephrine is thought to be the primary hormone of the humoral adrenergic system in most fishes (80, 81). As part of the “fight or flight” response, catecholamines exert numerous actions that include rapid mobilization of glucose and free fatty acids through enhanced glycogenolysis and lipolysis, respectively, as well as regulation of respiration and blood flow (79, 81, 87). Leptin is also critical for regulating energy expenditure in vertebrates and responds to various stressors (see Leptin Responses to Stress in Vertebrates), yet little is known about how the hormone interacts with components of the endocrine stress axis, particularly in non-mammalian vertebrates (27). To date, the majority of studies examining the relationship between leptin and the stress axis have been performed in mammals (27, 51). However, studies in lizards [Podarcis sicula (74)] and teleosts have indicated that leptin may act as a key metabolic regulator during stress in all vertebrates through mobilization of energy stores (Figure 3).
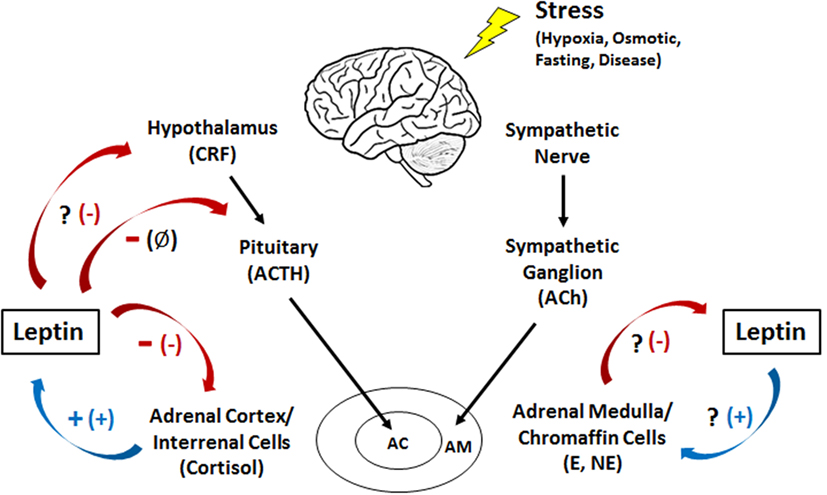
Figure 3. Interactions between leptin and the humoral adrenergic and hypothalamic–pituitary–adrenal/interrenal axes in teleosts and mammals. +, stimulation; -, inhibition; Ø, no effect; ?, an unknown relationship. The mammalian response is represented by the symbol in parentheses. CRF, corticotropin-releasing factor; ACTH, adrenocorticotropic hormone; Ach, acetylcholine; E, epinephrine; NE, norepinephrine; AC, adrenal cortex; AM, adrenal medulla.
Leptin has been shown to stimulate the release of catecholamines in both porcine (88) and bovine (89) adrenal medullary cells. In addition, leptin increased mRNA levels of tyrosine hydroxylase, the rate-limiting enzyme in catecholamine production (88). This suggests a synergistic relationship between leptin and catecholamines wherein leptin mobilizes energy from lipids while simultaneously stimulating the release of catecholamines to mobilize glucose during periods of stress (90). Interestingly, other studies utilizing human chromaffin cells have shown no significant change in catecholamine release with leptin treatment (91). The contradictory responses observed between human and other mammalian models could possibly be due to differences in methodology (isolated cells vs. whole adrenal tissue), the leptin concentrations used, or simply species differences (88). The regulation of catecholamines by leptin in fishes has not been well characterized. In goldfish (C. auratus), chronic leptin treatment resulted in no significant changes in hypothalamic catecholamines (58); however the effects of leptin on circulating catecholamines are yet to be examined.
While leptin exerts a stimulatory effect on catecholamine release in mammals, epinephrine has been shown to directly inhibit leptin secretion (92–95). In addition, increases in intracellular cAMP in medullary cells, one of the second messengers involved in adrenergic signaling, downregulate leptin mRNA (96). Leptin increases intracellular cAMP in addition to stimulating catecholamine release (88), both of which could act in a negative feedback loop to inhibit further leptin release. One theory behind this inhibition is that it is not advantageous for catecholamines to stimulate leptin during acute stress as obtaining energy from lipolysis is too slow for a “fight or flight” response; however, it may play a role in mediating the response to chronic stress (27). The regulation of leptin by catecholamines in fishes and other ectotherms is still unclear. However, both leptin and epinephrine exhibit glycogenolytic and/or lipolytic actions and have been shown to increase during times of stress in fishes (71, 79).
Glucocorticoids and Leptin
The human ob promoter region possesses glucocorticoid response elements, suggesting that cortisol may elicit some of their actions by inducing leptin transcription (97, 98). Indeed, glucocorticoids elicited a stimulatory effect on leptin synthesis and secretion in rats (99), humans (100), and cultured human adipocytes (101) (Figure 3). In addition, the synthetic glucocorticoid dexamethasone increased mRNA levels and stimulated leptin secretion in rat adipocytes (96, 102). Similar results have been observed in teleosts, with cortisol increasing hepatic leptin mRNA levels in rainbow trout (O. mykiss) both in vivo and in vitro (103). In addition, when trout hepatocytes were treated simultaneously with cortisol and RU486, a glucocorticoid receptor antagonist, the increase in leptin mRNA was attenuated (103). Whether a similar response occurs with leptin secretion remains unknown. It has been speculated that since cortisol release is slower than that of catecholamines, the prolonged stressors that elicit cortisol actions would also benefit from the catabolic effects of leptin on lipids and/or carbohydrates reported in fishes, particularly in the liver where leptin is produced and may act locally (26, 27, 39, 71, 73, 104).
Leptin in turn has an overall inhibitory effect on the HPA axis in mammals (98), inhibiting CRF release from the hypothalamus in mice (105) and suppressing cortisol secretion from adrenal cells (106–108) (Figure 3). In contrast, leptin has no effect on ACTH secretion from the pituitary, suggesting that it regulates glucocorticoid release indirectly via the hypothalamus and directly by acting on the adrenal gland (105). When human adrenocortical cells are incubated with leptin, a dose-dependent decrease in ACTH-stimulated cortisol secretion is observed (91), while in leptin knockout mice (ob/ob), circulating levels of glucocorticoids are 85% higher than basal. Injecting these knockouts with leptin, however, reduced the level of glucocorticoids by 40% (109, 110). These data could potentially suggest a synergism between leptin and cortisol wherein cortisol stimulates the secretion of leptin that, in turn, mobilizes energy stores necessary for coping with a stressor. It has also been suggested that the anorexigenic effects of leptin could counteract the weight gain effects of cortisol in mammals (111). Similar results have been observed in teleosts, suggesting that interactions between leptin and glucocorticoids may be conserved in vertebrates. In the common carp (C. carpio), leptin inhibited ACTH-stimulated cortisol secretion in vivo and caused a dose-dependent decrease in CRF-induced ACTH secretion from the pituitary in vitro (6, 112). No changes in circulating cortisol were observed in leptin-injected goldfish [C. auratus (59)]; however, it is possible that leptin only inhibits glucocorticoid production when the HPI axis has been activated and circulating cortisol levels are elevated. In general, we do know that teleost pituitary glands are responsive to leptin (6, 26, 40, 42), and as such, it has been postulated that leptin may regulate the stress axis at the level of the pituitary (6, 113).
Currently, there are no other studies in fishes examining the relationship between leptin and the hormones of the stress axis, specifically interactions with catecholamines and glucocorticoids. There is a need to address these gaps as understanding these interactions will help to elucidate leptin’s basal function as a putative regulator of the endocrine stress response in these organisms and how these actions may differ from that of the classically described adipostat in mammals.
Leptin Responses to Stress in Vertebrates
Fasting
Catabolic stress associated with fasting typically leads to downregulation of leptin expression in mammals (114). The preponderance of evidence in teleosts, however, points to fasting-induced increases in leptin synthesis and secretion (23, 42, 58, 72, 115, 116); albeit evidence in two species, the hybrid striped bass (M. chrysops x M. saxatilis) and red-bellied piranha (Pygocentrus nattereri) show that production of the hormone may decline with fasting (10, 117). The general increase in leptin during fasting found in most teleosts presents a functional paradox between the role of leptin as an anorexigenic endocrine signal and the drive to increase food intake during fasting. Leptin could aid to limit feeding to avoid the metabolic costs associated with foraging and digestion (118) during periods of low food availability, or perhaps other orexigenic factors such as ghrelin, whose levels are known to increase dramatically with fasting (119), outweigh the anorexigenic properties of leptin in driving food intake when energy status is low. Regardless, the increase in leptin with fasting is likely critical for promoting the catabolism of energy stores to fuel essential cellular processes. The variability of responses in fishes compared to mammals may be attributed to distinct regulation of energy stores, perhaps suggesting that signaling during altered metabolic states may not be reliant solely on leptin, but an integration of lipostatic, glucostatic, and other metabolic and endocrine signals. Further, as a consequence of genome duplication events in teleosts [reviewed in Ref. (120, 121)], some species possess multiple leptin paralogs that may exhibit different functional properties.
Hyperosmotic Stress
Euryhaline fishes can withstand wide fluctuations in environmental salinity. Through active excretion of ions, they can overcome large increases in plasma osmolality (>150 mOsm) during acute seawater challenge (71). The process of seawater acclimation consumes 20–68% of their total metabolic energy demand (122, 123). Elevated leptin stimulates Na+ retention and induces hypertension in rats and may be associated with hypertension induced kidney disease in humans (124). Few studies have investigated the role of leptin in osmoregulation in teleost fishes, despite its regulatory interactions with GH, IGFs, and PRL, hormones known to control salt and water balance (26, 40–42). In the Mozambique tilapia (O. mossambicus), acute seawater transfer induced significant increases in hepatic lepa and lepr mRNA levels (71). The authors propose that leptin may work with cortisol to mobilize energy stores by inducing hepatic glycogenolysis and gluconeogenesis, respectively, thereby allowing the organism to fuel the increased energy demands associated with hyperosmotic stress. The hormone had no direct effect on expression of the gill Na+K+-ATPase pump, so it remains unclear whether the hormone is ionoregulatory in teleosts. Additional studies suggest that leptin may stimulate the release of PRL, an important freshwater osmoregulatory or Na+-retaining hormone in teleosts (26, 40). Collectively, the results suggest that leptin may act to mobilize energy for seawater adaptation and promote GH sensitivity and IGF production to enhance seawater acclimation (41, 42, 71). It may also promote synthesis and secretion of PRL for freshwater adaptation (40).
Hypoxia
Oxygen is a necessary component of energy production in all vertebrates, and thus hypoxia represents a severe and potentially lethal stress. As leptin functions at the intersection of the endocrine stress response and metabolism, it is reasonable to postulate that it is involved in the vertebrate response to hypoxia. Indeed, an increase in the transcription of leptin in humans, observed in response to hypoxia and hypoxia-inducible factor 1 (HIF-1), transactivates the human leptin gene promoter (125, 126). In addition, leptin mRNA levels increase in response to hypoxia in a variety of mammalian cell lines (127–129). Interestingly, Meissner et al. (130) reported that short-term hypoxia in rats had no effect on plasma leptin levels or expression in adipose tissue; however, leptin expression was increased in the liver, kidney, and lungs suggesting a unique metabolic role for leptin under hypoxic stress. Leptin has further been shown to attenuate apoptosis under hypoxic conditions and appears to be necessary for behavioral recovery following acute hypoxia (131, 132). Taken together, the data from mammals point to a crucial role for leptin as a multifaceted mediator of energy homeostasis during hypoxia.
The first report of leptin regulation by hypoxia in fishes came from Chu et al. (133). The authors showed that lepa expression increased after 4 and 10 days of hypoxic exposure in zebrafish (D. rerio) and implicated HIF-1α as a key mediator of this response. In common carp (C. carpio), the expression of lep-a1, lep-a2, and lepr in the liver increases in proportion with the length of hypoxic exposure (113). This study also showed that exposure to hypoxia upregulated expression of lepr mRNA in the pituitary, suggesting potential integration with the HPI axis (113). In addition, transcriptome data for the tilapia (O. mossambicus) shows upregulation of genes responsive to hypoxia in the pituitary following leptin treatment [e.g., chaperone-containing TCP1, chromodomain helicase-binding domain, heat shock protein 90b1, Gene Ontology 0070482/001666 (75)]. Crucian carp (C. carassius) expresses multiple isoforms of the LepR in the gill, and the mRNA levels increased in response to hypoxia in vivo (134). While there are still significant gaps in knowledge with regards to how leptin is acting to augment organism energetics during hypoxia in fishes, it appears that leptin is indeed regulated by hypoxia in much the same way as mammals, increasing in response to the decreased availability of oxygen for ATP production. The emerging role of leptin in stimulating glycolysis among different vertebrates may fit well with its upregulation during hypoxia or normoxia (Warburg effect).
Immune Function and Disease
Immunity is intimately linked to an organism’s metabolism and energy status, and as such, allocating energy to the immune system in states of both health and disease is critical to the overall fitness and survival of an organism (135). Fasting and nutritional deprivation are associated with an increased disease susceptibility, as well as immune system suppression and dysfunction in vertebrates (136–138). Due to its role as a vital neuroendocrine mediator of metabolic state, leptin has been investigated as a regulator of the energetics associated with the innate and adaptive immune responses. In mammals, increases in serum leptin levels occur with inflammation, a response that appears to be modulated by glucocorticoids (139). Further, leptin has been shown to reverse starvation-induced immunosuppression by stimulating the proliferation of pro-inflammatory cytokine-secreting T cells (140). Despite having been extensively studied in mammals, few studies have explored the interplay of leptin and immunity in teleost fishes or other non-mammalian vertebrates.
The correct allocation of energy to the innate immune system, the first line of organism defense and the most important responder in the acute phase of an infection, is critical to host survival. Leptin signaling has been shown to be necessary for innate immunity in mammals (135, 141), increasing chemotaxis and oxidative function and delaying apoptosis in immune cells (142–146). Leptin increases activation and proliferation and induces production of pro-inflammatory cytokines in phagocytes (147). Similar functions have been observed in the adaptive immune response, wherein leptin acts to stimulate B-lymphocytes by inducing cell cycle entry, preventing apoptosis and causing the secretion of pro-inflammatory cytokines (148, 149). In addition, it has been determined that leptin signaling is necessary for normal rates of glucose uptake and glycolysis in activated T-cells (150). These data suggest that, in mammals, leptin may drive immune activation by increasing the oxidative and overall glycolytic capacities of various immune cells.
Very little work has been done to directly connect leptin to the immune system in teleost fishes. Mariano et al. (151) showed that leptin drove ERK and STAT3 phosphorylation in both adherent and non-adherent trout leukocytes. Additional evidence for a role of leptin in regulating immune function in teleosts comes from MacDonald et al. (152) in which rainbow trout (O. mykiss) infected with a pathogenic hemoflagellate exhibited significantly higher mRNA and plasma levels of LepA. The authors determined that leptin was being secreted in response to the hypoxemia associated with the infection to reduce food intake (152). This would serve to prevent the organism from having to allocate energy toward digestive functions while in the diseased state. It is also possible that increases in leptin synthesis and secretion could lead to catabolism of energy stores necessary to meet the energetic demands of fighting the disease. Although limited, the data suggest an integration of leptin with immune function, and future studies should investigate the extent of leptin’s involvement in immunometabolic pathways in teleost fishes.
Conclusion
In teleost fishes, there is much that remains to be elucidated about the role of leptin in energy homeostasis. Although there is evidence that leptin acts as a glucoregulatory agent in teleosts, there are also reports of leptin having lipolytic actions, particularly in the cyprinid fishes. In mammals, leptin has been implicated in regulating the metabolism of both glucose and lipids, suggesting some conservation of function between the two groups, perhaps sharing roles in promoting glycolysis. However, the increase in leptin levels during fasting presents a functional paradox against its role as an anorexigenic hormone. A further look into the function of leptins in regulating basal metabolism may shed light in this area. As multiple paralogs of leptin have been identified in teleosts, future studies should focus on whether the disparate actions are simply species-specific differences or the result of neofunctionalization between the various leptin paralogs. To date, the studies investigating the involvement of leptin in regulating immunity and the endocrine stress response suggest that such roles may be conserved within vertebrates. However, it is currently unclear by what means metabolic energy stores might be preferentially mobilized by leptin upon exposure to acute and chronic stressors, such as osmotic stress or hypoxia. Further, it remains to be determined how multiple endocrine signals (e.g., catecholamines, glucocorticoids) might integrate with leptin signaling to achieve the appropriate physiological response under such conditions. Studies in teleosts, or other ectotherms, may shed light on potential new functions of leptin that may be well conserved in the vertebrate lineage.
Author Contributions
All individuals contributed to writing and reviewing the final version of the article.
Conflict of Interest Statement
The authors declare that the research was conducted in the absence of any commercial or financial relationships that could be construed as a potential conflict of interest.
Acknowledgments
The authors would like to thank Scott Salger for providing comments on the manuscript.
Funding
Some of the work described herein was supported by grants to RB from the National Science Foundation (IOS-1457040) and the Feed-the-Future AquaFish Innovation Lab of the United States Agency for International Development (EPP-A-00-06-00012-00, accession number 1471). The opinions expressed herein are those of the authors and do not necessarily reflect that of the granting agencies.
References
1. Zhang Y, Proenca R, Maffei M, Barone M, Leopold L, Friedman JM. Positional cloning of the mouse obese gene and its human homologue. Nature (1994) 372:425–31. doi: 10.1038/372425a0
2. Broberger C, De Lecea L, Sutcliffe JG, Hökfelt T. Hypocretin/orexin-and melanin-concentrating hormone-expressing cells form distinct populations in the rodent lateral hypothalamus: relationship to the neuropeptide Y and Agouti gene-related protein systems. J Comp Neurol (1998) 402(4):460–74. doi:10.1002/(SICI)1096-9861(19981228)402:4<460::AID-CNE3>3.3.CO;2-J
3. Londraville RL, Macotela Y, Duff RJ, Easterling MR, Liu Q, Crespi EJ. Comparative endocrinology of leptin: assessing function in a phylogenetic context. Gen Comp Endocrinol (2014) 203:146–57. doi:10.1016/j.ygcen.2014.02.002
4. Doyon C, Drouin G, Trudeau VL, Moon TW. Molecular evolution of leptin. Gen Comp Endocrinol (2001) 124:188–98. doi:10.1006/gcen.2001.7701
5. Kurokawa T, Uji S, Suzuki T. Identification of cDNA coding for a homologue to mammalian leptin from pufferfish, Takifugu rubripes. Peptides (2005) 26:745–50. doi:10.1016/j.peptides.2004.12.017
6. Gorissen M, Flik G. Leptin in teleostean fish, towards the origins of leptin physiology. J Chem Neuroanat (2014) 62:200–6. doi:10.1016/j.jchemneu.2014.06.005
7. Crespi EJ, Denver RJ. Roles of stress hormones in food intake regulation in anuran amphibians throughout the life cycle. Comp Biochem Physiol A Mol Integr Physiol (2005) 142:3–19. doi:10.1016/j.cbpb.2004.12.007
8. Boswell T, Dunn IC, Wilson PW, Joseph N, Burt DW, Sharp PJ. Identification of a non-mammalian leptin-like gene: characterization and expression in the tiger salamander (Ambystoma tigrinum). Gen Comp Endocrinol (2006) 146:157–66. doi:10.1016/j.ygcen.2005.08.001
9. Boorse GC, Libbon JV. Genomic characterization of two leptin genes and a leptin receptor gene in the Green Anole, Anolis carolinensis. Integrative and Comparative Biology. (Vol. 50), Cary, NC: Oxford University Press (2010). p. E207.
10. Won ET, Baltzegar DA, Picha ME, Borski RJ. Cloning and characterization of leptin in a Perciform fish, the striped bass (Morone saxatilis): control of feeding and regulation by nutritional state. Gen Comp Endocrinol (2012) 178:98–107. doi:10.1016/j.ygcen.2012.04.019
11. Huising MO, Geven EJW, Kruiswijk CP, Nabuurs SB, Stolte EH, Spanings FAT, et al. Increased leptin expression in common carp (Cyprinus carpio) after food intake but not after fasting or feeding to satiation. Endocrinology (2006) 147(12):5786–97. doi:10.1210/en.2006-0824
12. Murashita K, Uji S, Yamamoto T, Rønnestad I, Kurokawa T. Production of recombinant leptin and its effects on food intake in rainbow trout (Oncorhynchus mykiss). Comp Biochem Physiol B (2008) 150:377–84. doi:10.1016/j.cbpb.2008.04.007
13. Gorissen M, Bernier NJ, Nabuurs SB, Flik G, Huising MO. Two divergent leptin paralogues in zebrafish (Danio rerio) that originate early in teleostean evolution. J Endocrinol (2009) 201:329–39. doi:10.1677/JOE-09-0034
14. Rønnestad I, Nilsen TO, Murashita K, Angotzi AR, Moen AG, Stefansson SO, et al. Leptin and leptin receptor genes in Atlantic salmon: cloning, phylogeny, tissue distribution and expression correlated to long-term feeding status. Gen Comp Endocrinol (2010) 168:55–70. doi:10.1016/j.ygcen.2010.04.010
15. Zhang H, Chen H, Zhang Y, Li S, Lu D, Zhang H, et al. Molecular cloning, characterization and expression profiles of multiple leptin genes and a leptin receptor gene in orange-spotted grouper (Epinephelus coioides). Gen Comp Endocrinol (2013) 181:295–305. doi:10.1016/j.ygcen.2012.09.008
16. Kurokawa T, Murashita K. Genomic characterization of multiple leptin genes and a leptin receptor gene in the Japanese medaka, Oryzias latipes. Gen Comp Endocrinol (2009) 161:229–37. doi:10.1016/j.ygcen.2009.01.008
17. Gong Y, Luo Z, Zhu Q-L, Zheng J-L, Tan X-Y, Chen Q-L, et al. Characterization and tissue distribution of leptin, leptin receptor and leptin receptor overlapping transcript genes in yellow catfish Pelteobagrus fulvidraco. Gen Comp Endocrinol (2013) 182:1–6. doi:10.1016/j.ygcen.2012.11.006
18. Shpilman M, Hollander-Cohen L, Ventura T, Gertler A, Levavi-Sivan B. Production, gene structure and characterization of two orthologs of leptin and a leptin receptor in tilapia. Gen Comp Endocrinol (2014) 207:74–85. doi:10.1016/j.ygcen.2014.05.006
19. Tang Y, Yu J, Li H, Xu P, Li J, Ren H. Molecular cloning, characterization and expression analysis of multiple leptin genes in Jian carp (Cyprinus carpio var. Jian). Comp Biochem Physiol B (2013) 166:133–40. doi:10.1016/j.cbpb.2013.07.009
20. Frøiland E, Murashita K, Jørgensen EH, Kurokawa T. Leptin and ghrelin in anadramous Arctic charr: cloning and change in expressions during a seasonal feeding cycle. Gen Comp Endocrinol (2010) 165:136–43. doi:10.1016/j.ygcen.2009.06.010
21. Li G-G, Liang X-F, Xie Q, Li G, Yu Y, Lai K. Gene structure, recombinant expression and functional characterization of grass carp leptin. Gen Comp Endocrinol (2010) 166:117–27. doi:10.1016/j.ygcen.2009.10.009
22. Ohga H, Matsumori K, Kodama R, Kitano H, Nagano N, Yamaguchi A, et al. Two leptin genes and a leptin receptor gene of female chub mackerel (Scomber japonicus): molecular cloning, tissue distribution and expression in different obesity indices and pubertal stages. Gen Comp Endocrinol (2015) 222:88–98. doi:10.1016/j.ygcen.2015.06.002
23. Yuan X, Li A, Liang XF, Huang W, Song Y, He S, et al. Leptin expression in mandarin fish Siniperca chuatsi (Basilewsky): regulation by postprandial and short-term fasting treatment. Comp Biochem Physiol A Mol Integr Physiol (2016) 194:8–18. doi:10.1016/j.cbpa.2016.01.014
24. Chen T, Chen S, Ren C, Hu C, Tang D, Yan A. Two isoforms of leptin in the white-clouds mountain minnow (Tanichthys albonubes): differential regulation by estrogen despite similar response to fasting. Gen Comp Endocrinol (2016) 225:174–84. doi:10.1016/j.ygcen.2015.08.002
25. Venkatesh B. Evolution and diversity of fish genomes. Curr Opin Genet Dev (2003) 13:588–92. doi:10.1016/j.gde.2003.09.001
26. Douros JD, Baltzegar DA, Breves JP, Lerner DT, Seale AP, Grau EG, et al. Prolactin is a major inhibitor of hepatic leptin A synthesis and secretion: studies utilizing a homologous leptin A ELISA in the tilapia. Gen Comp Endocrinol (2014) 207:86–93. doi:10.1016/j.ygcen.2014.03.007
27. Copeland DL, Duff RJ, Liu Q, Prokop J, Londraville RL. Leptin in teleost fishes: an argument for comparative study. Front Physiol (2011) 2:26. doi:10.3389/fphys.2011.00026
28. Tinoco AB, Nisembaum LG, Isorna E, Delgado MJ, de Pedro N. Leptins and leptin receptor expression in the goldfish (Carassius auratus): regulation by food intake and fasting/overfeeding conditions. Peptides (2012) 34:329–35. doi:10.1016/j.peptides.2012.02.001
29. Angotzi AR, Stefansson SO, Nilsen TO, Rathore RM, Rønnestad I. Molecular cloning and genomic characterization of novel leptin-like genes in salmonids provide new insight into the evolution of the leptin gene family. Gen Comp Endocrinol (2013) 187:48–59. doi:10.1016/j.ygcen.2013.03.022
30. Tartaglia LA. The leptin receptor. J Biol Chem (1997) 272(10):6093–6. doi:10.1074/jbc.272.10.6093
31. White UA, Stephens JM. The gp130 receptor cytokine family: regulators of adipocyte development and function. Curr Pharm Des (2011) 17(4):340–6. doi:10.2174/138161211795164202
32. Cui MY, Hu CK, Pelletier C, Dziuba A, Slupski RH, Li C, et al. Ancient origins and evolutionary conservation of intracellular and neural signaling pathways engaged by the leptin receptor. Endocrinology (2014) 155(11):4202–14. doi:10.1210/en.2014-1301
33. Kurokawa T, Murashita K, Suzuki T, Uji S. Genomic characterization and tissue distribution of leptin receptor and leptin receptor overlapping transcript genes in the pufferfish, Takifugu rubripes. Gen Comp Endocrinol (2008) 158:108–14. doi:10.1016/j.ygcen.2008.06.003
34. Gong N, Björnsson BT. Leptin signaling in the rainbow trout central nervous system is modulated by a truncated leptin receptor isoform. Endocrinology (2014) 155(7):2445–55. doi:10.1210/en.2013-2131
35. Escobar S, Rocha A, Felip A, Carrillo M, Zanuy S, Kah O, et al. Leptin receptor gene in the European sea bass (Dicentrarchus labrax): cloning, phylogeny, tissue distribution and neuroanatomical organization. Gen Comp Endocrinol (2016) 229:100–11. doi:10.1016/j.ygcen.2016.03.017
36. Aguilar AJ, Conde-Sieira M, Polakof S, Miguez JM, Soengas JL. Central leptin treatment modulates brain glucosensing function and peripheral energy metabolism of rainbow trout. Peptides (2010) 31:1044–54. doi:10.1016/j.peptides.2010.02.026
37. Aguilar AJ, Conde-Sieira M, Lopez-Patino M, Miguez JM, Soengas JL. In vitro leptin treatment of rainbow trout hypothalamus and hindbrain affects glucosensing and gene expression of neuropeptides involved in food intake regulation. Peptides (2011) 32:232–40. doi:10.1016/j.peptides.2010.11.007
38. Gong N, Jönsson E, Bjöornsson BT. Acute anorexigenic action of leptin in rainbow trout is mediated by the hypothalamic PI3K pathway. J Mol Endocrinol (2016) 56(3):227–38. doi:10.1530/JME-15-0279
39. Song YF, Wu K, Tan XY, Zhang LH, Zhuo MQ, Pan YX, et al. Effects of recombinant human leptin administration on hepatic lipid metabolism in yellow catfish Pelteobagrus fulvidraco: in vivo and in vitro studies. Gen Comp Endocrinol (2015) 212:92–9. doi:10.1016/j.ygcen.2015.01.022
40. Tipsmark CK, Strom CN, Bailey ST, Borski RJ. Leptin stimulates pituitary prolactin release through an extracellular signal-regulated kinase-dependent pathway. J Endocrinol (2008) 196:275–81. doi:10.1677/JOE-07-0540
41. Won ET, Douros JD, Hurt DA, Borski RJ. Leptin stimulates hepatic growth hormone receptor and insulin-like growth factor gene expression in a teleost fish, the hybrid striped bass. Gen Comp Endocrinol (2016) 229:84–91. doi:10.1016/j.ygcen.2016.02.003
42. Douros JD, Baltzegar DA, Mankiewicz J, Taylor J, Yamaguchi Y, Lerner DT, et al. Control of leptin by metabolic state and its regulatory interactions with pituitary growth hormone and hepatic growth hormone receptors and insulin like growth factors in the tilapia (Oreochromis mossambicus). Gen Comp Endocrinol (2017) 240:227–37. doi:10.1016/j.ygcen.2016.07.017
43. Saladin R, de Vos P, Guerre-Millo M, Leturque A, Girard J, Staels B, et al. Transient increase in obese gene expression after food intake or insulin administration. Nature (1995) 377(6549):527. doi:10.1038/377527a0
44. Bjorbaek C, Kahn BB. Leptin signaling in the central nervous system and the periphery. Recent Prog Horm Res (2004) 59:305–32. doi:10.1210/rp.59.1.305
45. Arora S. Leptin and its metabolic interactions – an update. Diabetes Obes Metab (2008) 10(11):973–93. doi:10.1111/j.1463-1326.2008.00852.x
46. Elias CF, Aschkenasi C, Lee C, Kelly J, Ahima RS, Bjorbaek C, et al. Leptin differentially regulates NPY and POMC neurons projecting to the lateral hypothalamic area. Neuron (1999) 23(4):775–86. doi:10.1016/S0896-6273(01)80035-0
47. Cowley MA, Smart JL, Rubinstein M, Cerdán MG, Diano S, Horvath TL, et al. Leptin activates anorexigenic POMC neurons through a neural network in the arcuate nucleus. Nature (2001) 411(6836):480–4. doi:10.1038/35078085
48. Elmquist JK. Hypothalamic pathways underlying the endocrine, autonomic, and behavioral effects of leptin. Physiol Behav (2001) 74(4):703–8. doi:10.1016/S0031-9384(01)00613-8
49. Ahima RS. Adipose tissue as an endocrine organ. Obesity (2006) 14(S8):242S–9S. doi:10.1038/oby.2006.317
50. Ahima RS, Qi Y, Singhal NS, Jackson MB, Scherer PE. Brain adipocytokine action and metabolic regulation. Diabetes (2006) 55(Suppl 2):S145–54. doi:10.2337/db06-S018
51. Ahima RS, Flier JS. Adipose tissue as an endocrine organ. Trends Endocrinol Metab (2000) 11(8):327–32. doi:10.1016/S1043-2760(00)00301-5
52. Weigle DS, Bukowski TR, Foster DC, Holderman S, Kramer JM, Lasser G, et al. Recombinant ob protein reduces feeding and body weight in the ob/ob mouse. J Clin Invest (1995) 96(4):2065. doi:10.1172/JCI118254
53. Farooqi IS, Matarese G, Lord GM, Keogh JM, Lawrence E, Agwu C, et al. Beneficial effects of leptin on obesity, T cell hyporesponsiveness, and neuroendocrine/metabolic dysfunction of human congenital leptin deficiency. J Clin Invest (2002) 110(8):1093–103. doi:10.1172/JCI0215693
54. Volkoff H, Eykelbosh AJ, Peter RE. Role of leptin in the control of feeding of goldfish Carassius auratus: interactions with cholecystokinin, neuropeptide Y and orexin A, and modulation by fasting. Brain Res (2003) 972(1):90–109. doi:10.1016/S0006-8993(03)02507-1
55. Volkoff H. The role of neuropeptide Y, orexins, cocaine and amphetamine-related transcript, cholecystokinin, amylin and leptin in the regulation of feeding in fish. Comp Biochem Physiol B (2006) 144(3):325–31. doi:10.1016/j.cbpa.2005.10.026
56. Volkoff H, Canosa LF, Unniappan S, Cerda-Reverter JM, Bernier NJ, Kelly SP, et al. Neuropeptides and the control of food intake in fish. Gen Comp Endocrinol (2005) 142(1):3–19. doi:10.1016/j.ygcen.2004.11.001
57. Volkoff H. The neuroendocrine regulation of food intake in fish: a review of current knowledge. Front Neurosci (2016) 10:540. doi:10.3389/fnins.2016.00540
58. de Pedro N, Martinez-Alvarez R, Delgado MJ. Acute and chronic leptin reduces food intake and body weight in goldfish (Carassius auratus). J Endocrinol (2006) 188(3):513–20. doi:10.1677/joe.1.06349
59. Vivas Y, Azpeleta C, Feliciano A, Velarde E, Isorna E, Delgado MJ, et al. Time-dependent effects of leptin on food intake and locomotor activity in goldfish. Peptides (2011) 32(5):989–95. doi:10.1016/j.peptides.2011.01.028
60. Rønnestad I, Søyland MA, Hansen T, Jordal AE, Nilsen TO, Gomes AS, et al. Effects of intraperitoneal administration of leptin on voluntary feed intake, appetite signaling pathways and metabolism in Atlantic salmon, Salmo salar. FASEB J (2016) 30(1):lb644.
61. Chisada SI, Kurokawa T, Murashita K, Rønnestad I, Taniguchi Y, Toyoda A, et al. Leptin receptor-deficient (knockout) medaka, Oryzias latipes, show chronical up-regulated levels of orexigenic neuropeptides, elevated food intake and stage specific effects on growth and fat allocation. Gen Comp Endocrinol (2014) 195:9–20. doi:10.1016/j.ygcen.2013.10.008
62. Michel M, Page-McCaw PS, Chen W, Cone RD. Leptin signaling regulates glucose homeostasis, but not adipostasis, in the zebrafish. Proc Natl Acad Sci U S A (2016) 113(11):3084–9. doi:10.1073/pnas.1513212113
63. Frøiland E, Jobling M, Björnsson BT, Kling P, Ravuri CS, Jørgensen EH. Seasonal appetite regulation in the anadromous Arctic charr: evidence for a role of adiposity in the regulation of appetite but not for leptin in signalling adiposity. Gen Comp Endocrinol (2012) 178(2):330–7. doi:10.1016/j.ygcen.2012.06.017
64. Kling P, Jönsson E, Nilsen TO, Einarsdottir IE, Rønnestad I, Stefansson SO, et al. The role of growth hormone in growth, lipid homeostasis, energy utilization and partitioning in rainbow trout: interactions with leptin, ghrelin and insulin-like growth factor I. Gen Comp Endocrinol (2012) 175(1):153–62. doi:10.1016/j.ygcen.2011.10.014
65. Salmerón C, Johansson M, Angotzi AR, Rønnestad I, Jönsson E, Björnsson BT, et al. Effects of nutritional status on plasma leptin levels and in vitro regulation of adipocyte leptin expression and secretion in rainbow trout. Gen Comp Endocrinol (2015) 210:114–23. doi:10.1016/j.ygcen.2014.10.016
66. Siegrist-Kaiser CA, Pauli V, Juge-Aubry CE, Boss O, Pernin A, Chin WW, et al. Direct effects of leptin on brown and white adipose tissue. J Clin Invest (1997) 100:2858–64. doi:10.1172/JCI119834
67. Minokoshi Y, Kim YB, Peroni OD, Fryer LG, Muller C, Carling D, et al. Leptin stimulates fatty-acid oxidation by activating AMP-activated protein kinase. Nature (2002) 415:339–43. doi:10.1038/415339a
68. Minokoshi Y, Haque MS, Shimazu T. Microinjection of leptin into the ventromedial hypothalamus increases glucose uptake in peripheral tissues in rats. Diabetes (1999) 48(2):287–91. doi:10.2337/diabetes.48.2.287
69. Wang MY, Lee Y, Unger RH. Novel form of lipolysis induced by leptin. J Biol Chem (1999) 274:17541–4. doi:10.1074/jbc.274.25.17541
70. Zhang LH, Tan XY, Wu K, Zhuo MQ, Song YF, Chen QL. Regulation and mechanism of leptin on lipid metabolism in ovarian follicle cells from yellow catfish Pelteobagrus fulvidraco. Gen Comp Endocrinol (2015) 222:116–23. doi:10.1016/j.ygcen.2015.06.008
71. Baltzegar DA, Reading BJ, Douros JD, Borski RJ. Role for leptin in promoting glucose mobilization during acute hyperosmotic stress in teleost fishes. J Endocrinol (2014) 220(1):61–72. doi:10.1530/JOE-13-0292
72. Kling P, Rønnestad I, Stefansson SO, Murashita K, Kurokawa T, Björnsson BT. A homologous salmonid leptin radioimmunoassay indicates elevated plasma leptin levels during fasting of rainbow trout. Gen Comp Endocrinol (2009) 162(3):307–12. doi:10.1016/j.ygcen.2009.04.003
73. Wu K, Tan XY, Xu YH, Shi X, Fan YF, Li DD, et al. JAK family members: molecular cloning, expression profiles and their roles in leptin influencing lipid metabolism in Synechogobius hasta. Comp Biochem Physiol B (2017) 203:122–31. doi:10.1016/j.cbpb.2016.10.004
74. Paolucci M, Buono S, Sciarrillo R, Putti R. Effects of leptin administration on the endocrine pancreas and liver in the lizard Podarcis sicula. J Exp Zool (2006) 305(5):383–95. doi:10.1002/jez.a.284
75. Douros JD. The Function and Regulation of Leptin in Teleost Fish [Doctoral Dissertation]. Raleigh, NC: North Carolina State University (2015).
76. Frühbeck G, Salvador J. Relation between leptin and the regulation of glucose metabolism. Diabetologia (2000) 43:3–12. doi:10.1007/s001250050002
77. van de Pol I, Flix G, Gorissen M. Comparative physiology of energy metabolism: fishing for endocrine signals in the early vertebrate pool. Front Endocrinol (2017) 8:36. doi:10.3389/fendo.2017.00036
78. Chrousos GP, Gold PW. The concepts of stress and stress system disorders, overview of physical and behavioral homeostasis. JAMA (1992) 267:1244–52.
80. Barton BA. Stress in fishes: a diversity of responses with particular reference to changes in circulating corticosteroids. Integr Comp Biol (2002) 42:517–25. doi:10.1093/icb/42.3.517
81. Reid SG, Bernier NJ, Perry SF. The adrenergic stress response in fish: control of catecholamine storage and release. Comp Biochem Physiol C (1998) 120:1–27.
82. Gorissen M, Flik G. The endocrinology of the stress response in fish: an adaptation-physiological view. In: Schrek CB, Tort L, Farrell AP, Brauner CJ, editors. Biology of Stress in Fish: Fish Physiology. (Vol. 35), London: Elsevier (2016). p. 75–111.
83. Nilsson S, Abrahamsson T, Grove DJ. Sympathetic nervous control of adrenaline release from the head kidney of the cod, Gadus morhua. Comp Biochem Physiol C (1976) 55:123–7. doi:10.1016/0306-4492(76)90034-4
84. Mazeaud MM, Mazeaud F, Donaldson EM. Primary and secondary effects of stress in fish: some new data with a general review. Trans Am Fish Soc (1977) 106(3):201–12. doi:10.1577/1548-8659(1977)106<201:PASEOS>2.0.CO;2
85. Axelrod J, Reisine TD. Stress hormones: their interaction and regulation. Science (1984) 224:452–9. doi:10.1126/science.6143403
86. Mommsen TP, Vijayan MM, Moon TW. Cortisol in teleosts: dynamics, mechanisms of action, and metabolic regulation. Rev Fish Biol Fish (1999) 9:211–68. doi:10.1023/A:1008924418720
87. Fabbri E, Capuzzo A, Moon TW. The role of circulating catecholamines in the regulation of fish metabolism: an overview. Comp Biochem Physiol C (1998) 120:177–92.
88. Takekoshi K, Mootoka M, Isobe K, Nomura F, Manmoku T, Ishii K, et al. Leptin directly stimulates catecholamine secretion and synthesis in cultured porcine adrenal medullary chromaffin cells. Biochem Biophys Res Commun (1999) 261:426–31. doi:10.1006/bbrc.1999.1025
89. Utsunomiya K, Yanagihara N, Tachikawa E, Cheah TB, Kajiwara K, Toyohira Y, et al. Stimulation of catecholamine synthesis in cultured bovine adrenal medullary cells by leptin. J Neurochem (2001) 76(3):926–34. doi:10.1046/j.1471-4159.2001.00123.x
90. Trayhurn P, Duncan JS, Hoggard N, Rayner DV. Regulation of leptin production: a dominant role for the sympathetic nervous system? Proc Nutr Soc (1998) 57:413–9. doi:10.1079/PNS19980060
91. Glasow A, Haidan A, Hiblers U, Breidert M, Gillespie J, Scherbaum WA, et al. Expression of ob receptor in normal human adrenals: differential regulation of adrenocortical and adrenomedullary function by leptin. J Clin Endocrinol Metab (1998) 83:4459–66. doi:10.1210/jc.83.12.4459
92. Kosaki A, Yamada K, Kuzuya H. Reduced expression of the leptin gene (ob) by catecholamine through a G(S) protein-coupled pathway in 3T3-L1 adipocytes. Diabetes (1996) 45:1744–9. doi:10.2337/diabetes.45.12.1744
93. Mantzoros CS, Frederich RC, Flier JS, Qu D, Susulic VS, Lowell BB, et al. Activation of β3 adrenergic receptors suppresses leptin expression and mediates a leptin-independent inhibition of food intake in mice. Diabetes (1996) 45(7):909–14. doi:10.2337/diab.45.7.909
94. Fritsche A, Wahl HG, Metzinger E, Renn W, Kellerer M, Haring H, et al. Evidence for inhibition of leptin secretion by catecholamines in man. Exp Clin Endocrinol Diabetes (1998) 106:415–8. doi:10.1055/s-0029-1212008
95. Carulli L, Ferrari S, Bertolini M, Tagliafico E, del Rio G. Regulation of ob gene expression: evidence for epinephrine-induced suppression in human obesity. J Clin Endocrinol Metab (1999) 84(0):3309–12. doi:10.1210/jcem.84.9.6007
96. Slieker LJ, Sloop KW, Surface PL, Kriauciunas A, LaQuier F, Manetta J, et al. Regulation of expression of ob mRNA and protein by glucocorticoids and cAMP. J Biol Chem (1996) 271(10):5301–4. doi:10.1074/jbc.271.10.5301
97. Gong DW, Bi S, Pratleys RE, Weintraub BD. Genomic structure and promoter analysis of the human obese gene. J Biol Chem (1996) 271(8):3971–4. doi:10.1074/jbc.271.8.3971
98. Leal-Cerro A, Soto A, Martínez MA, Dieguez C, Casanueva FF. Influence of cortisol status on leptin secretion. Pituitary (2001) 4:111–6. doi:10.1023/A:1012903330944
99. de Vos P, Saladin R, Auwerx J, Staels B. Induction of ob gene expression by corticosteroids is accompanied by body weight loss and reduced food intake. J Biol Chem (1995) 270(27):15958–61. doi:10.1074/jbc.270.27.15958
100. Newcomer JW, Selke G, Melson A, Gross J, Vogler GP, Dagogo-Jack S. Dose-dependent cortisol-induced increases in plasma leptin concentration in healthy humans. Arch Gen Psychiatry (1998) 55(11):995–1000. doi:10.1001/archpsyc.55.11.995
101. Wabitsch M, Jensen PB, Blum WF, Christoffersen CT, Englaro P, Heinze E, et al. Insulin and cortisol promote leptin production in cultured human fat cells. Diabetes (1996) 45:1435–8. doi:10.2337/diab.45.10.1435
102. Murakami T, Ida M, Shima K. Dexamethasone regulates obese expression in isolated rat adipocytes. Biochem Biophys Res Commun (1995) 214(3):1260–7. doi:10.1006/bbrc.1995.2422
103. Madison BN, Tavakoli S, Kramer S, Bernier NJ. Chronic cortisol and the regulation of food intake and the endocrine growth axis in rainbow trout. J Endocrinol (2015) 226(2):103–19. doi:10.1530/JOE-15-0186
104. Lu RH, Liang XF, Wang M, Zhou Y, Bai XL, He Y. The role of leptin in lipid metabolism in fatty degenerated hepatocytes of the grass carp Ctenopharyngodon idella. Fish Physiol Biochem (2012) 38:1759–74. doi:10.1007/s10695-012-9673-6
105. Heiman ML, Ahima RS, Craft LS, Schoner B, Stephens TW, Flier JS. Leptin inhibition of the hypothalamic-pituitary-adrenal axis in response to stress. Endocrinology (1997) 138:3859–63. doi:10.1210/endo.138.9.5366
106. Bornstein SR, Uhlmann K, Haidan A, Ehrhart-Bornstein M, Scherbaum WA. Evidence for a novel peripheral action of leptin as a metabolic signal to the adrenal gland: leptin inhibits cortisol release directly. Diabetes (1997) 46:1235–8. doi:10.2337/diabetes.46.7.1235
107. Pralong FP, Roduit R, Waeber G, Castillo E, Mosimann F, Thorens B, et al. Leptin inhibits directly glucocorticoid secretion by normal human and rat adrenal gland. Endocrinology (1998) 139(10):4264–8. doi:10.1210/endo.139.10.6254
108. Roubos EW, Dahmen M, Kozicz T, Xu L. Leptin and the hypothalamo-pituitary-adrenal axis. Gen Comp Endocrinol (2012) 177:28–36. doi:10.1016/j.ygcen.2012.01.009
109. Ahima RS, Prabakaran D, Flier JS. Postnatal leptin surge and regulation of circadian rhythm of leptin by feeding. Implications for energy homeostasis and neuroendocrine function. J Clin Invest (1998) 101(5):1020. doi:10.1172/JCI1176
110. Davis SN, Lamos EM, Loper H, Younk LM. Leptin in acute stress. In: Dagogo-Jack S, editor. Leptin. Cham: Springer International Publishing (2015). p. 103–15.
111. Dagogo-Jack S, Selke G, Melson AK, Newcomer JW. Robust leptin secretory responses to dexamethasone in obese subjects. J Clin Endocrinol Metab (1997) 82:3230–3. doi:10.1210/jcem.82.10.4154
112. Gorissen M, Bernier NJ, Manuel R, De Gelder S, Metz JR, Huising MO, et al. Recombinant human leptin attenuates stress axis activity in common carp (Cyprinus carpio L.). Gen Comp Endocrinol (2012) 178:75–81. doi:10.1016/j.ygcen.2012.04.004
113. Bernier NJ, Gorissen M, Flik G. Differential effects of chronic hypoxia and feed restriction on the expression of leptin and its receptor, food intake regulation and the endocrine stress response in common carp. J Exp Biol (2012) 215(13):2273–82. doi:10.1242/jeb.066183
114. Ahima RS, Prabakaran D, Mantzoros C, Qu D, Lowell B, Maratos-Flier E, et al. Role of leptin in the neuroendocrine response to fasting. Nature (1996) 382(6588):250. doi:10.1038/382250a0
115. Fuentes EN, Kling P, Einarsdottir IE, Alvarez M, Valdés JA, Molina A, et al. Plasma leptin and growth hormone levels in the fine flounder (Paralichthys adspersus) increase gradually during fasting and decline rapidly after refeeding. Gen Comp Endocrinol (2012) 177(1):120–7. doi:10.1016/j.ygcen.2012.02.019
116. Trombley S, Maugars G, Kling P, Björnsson BT, Schmitz M. Effects of long-term restricted feeding on plasma leptin, hepatic leptin expression and leptin receptor expression in juvenile Atlantic salmon (Salmo salar L.). Gen Comp Endocrinol (2012) 175(1):92–9. doi:10.1016/j.ygcen.2011.10.001
117. Volkoff H. Cloning, tissue distribution and effects of fasting on mRNA expression levels of leptin and ghrelin in red-bellied piranha (Pygocentrus nattereri). Gen Comp Endocrinol (2015) 217:20–7. doi:10.1016/j.ygcen.2015.05.004
118. Skalski GT, Picha ME, Gilliam JF, Borski RJ. Variable intake, compensatory growth and increased growth efficiency in fish: models and mechanisms. Ecology (2005) 86(6):1452–62. doi:10.1890/04-0896
119. Picha ME, Strom CN, Riley LG, Walker AA, Won ET, Johnstone WM, et al. Plasma ghrelin and growth hormone regulation in response to metabolic state in hybrid striped bass: effects of feeding, ghrelin and insulin-like growth factor-I on in vivo and in vitro GH secretion. Gen Comp Endocrinol (2009) 161(3):365–72. doi:10.1016/j.ygcen.2009.01.026
120. Hoegg S, Brinkmann H, Taylor JS, Meyer A. Phylogenetic timing of the fish-specific genome duplication correlates with the diversification of teleost fish. J Mol Evol (2004) 59(2):190–203. doi:10.1007/s00239-004-2613-z
121. Brunet FG, Crollius HR, Paris M, Aury JM, Gibert P, Jaillon O, et al. Gene loss and evolutionary rates following whole-genome duplication in teleost fishes. Mol Biol Evol (2006) 23(9):1808–16. doi:10.1093/molbev/msl049
122. Morgan JD, Sakamoto T, Grau EG, Iwama GK. Physiological and respiratory responses of the Mozambique tilapia (Oreochromis mossambicus) to salinity acclimation. Comp Biochem Physiol A Mol Integr Physiol (1997) 117(3):391–8. doi:10.1016/S0300-9629(96)00261-7
123. Boeuf G, Payan P. How should salinity influence fish growth? Comp Biochem Physiol C (2001) 130(4):411–23. doi:10.1016/S1532-0456(01)00268-X
124. Bełtowski J, Wójcicka G, Marciniak A, Jamroz A. Oxidative stress, nitric oxide production, and renal sodium handling in leptin-induced hypertension. Life Sci (2004) 74(24):2987–3000. doi:10.1016/j.lfs.2003.10.029
125. Ambrosini G, Nath AK, Sierra-Honigmann MR, Flores-Riveros F. Transcriptional activation of the human leptin gene in response to hypoxia: involvement of hypoxia-inducible factor 1. J Biol Chem (2002) 277(37):34601–9. doi:10.1074/jbc.M205172200
126. Grosfeld A, André J, Hauguel-de Mouzon S, Berra E, Pouysségur J, Guerre-Millo M. Hypoxia-inducible factor 1 transactivates the human leptin gene promoter. J Biol Chem (2002) 277(45):42953–7. doi:10.1074/jbc.M206775200
127. Grosfeld A, Turban S, André J, Cauzac M, Challier JC, Hauguel-de Mouzon S, et al. Transcriptional effect of hypoxia on placental leptin. FEBS Lett (2001) 502(3):122–6. doi:10.1016/S0014-5793(01)02673-4
128. Bruder ED, Jacobson L, Raff H. Plasma leptin and ghrelin in the neonatal rat: interaction of dexamethasone and hypoxia. J Endocrinol (2005) 185(3):477–84. doi:10.1677/joe.1.06159
129. Trayhurn P. Hypoxia and adipose tissue function and dysfunction in obesity. Physiol Rev (2013) 93(1):1–21. doi:10.1152/physrev.00017.2012
130. Meissner U, Hänisch C, Ostreicher I, Knerr I, Hofbauer KH, Blum WF, et al. Differential regulation of leptin synthesis in rats during short-term hypoxia and short-term carbon monoxide inhalation. Endocrinology (2005) 146(1):215–20. doi:10.1210/en.2004-0782
131. Sherry CL, Kramer JM, York JM, Freund GG. Behavioral recovery from acute hypoxia is reliant on leptin. Brain Behav Immun (2009) 23(2):169–75. doi:10.1016/j.bbi.2008.09.011
132. Shin EJ, Schram K, Zheng XL, Sweeney G. Leptin attenuates hypoxia/reoxygenation-induced activation of the intrinsic pathway of apoptosis in rat H9c2 cells. J Cell Physiol (2009) 221(2):490–7. doi:10.1002/jcp.21883
133. Chu DL, Li VW, Yu RM. Leptin: clue to poor appetite in oxygen-starved fish. Mol Cell Endocrinol (2010) 319(1):143–6. doi:10.1016/j.mce.2010.01.018
134. Cao YB, Xue JL, Wu LY, Jiang W, Hu PN, Zhu J. The detection of 3 leptin receptor isoforms in crucian carp gill and the influence of fasting and hypoxia on their expression. Domest Anim Endocrinol (2011) 41(2):74–80. doi:10.1016/j.domaniend.2011.04.002
135. Kominsky DJ, Campbell EJ, Colgan SP. Metabolic shifts in immunity and inflammation. J Immunol (2010) 184(8):4062–8. doi:10.4049/jimmunol.0903002
136. Cason JA, Britton WM. Effect of short-term feed deprivation on shell quality in laying hens. Poult Sci (1986) 65(3):530–7. doi:10.3382/ps.0650530
137. Chandra RK. 1990 McCollum Award lecture. Nutrition and immunity: lessons from the past and new insights into the future. Am J Clin Nutr (1991) 53(5):1087–101.
138. Shoemaker CA, Klesius PH, Lim C, Yildirim M. Feed deprivation of channel catfish, Ictalurus punctatus (Rafinesque), influences organosomatic indices, chemical composition and susceptibility to Flavobacterium columnare. J Fish Dis (2003) 26(9):553–61. doi:10.1046/j.1365-2761.2003.00489.x
139. Gualillo O, Eiras S, Lago F, Diegues C, Casanueva FF. Elevated serum leptin concentrations induced by experimental acute inflammation. Life Sci (2000) 67(20):2433–41. doi:10.1016/S0024-3205(00)00827-4
140. Lord GM, Matarese G, Howard JK, Baker RJ, Bloom SR, Lechler RI. Leptin modulates the T-cell immune response and reverses starvation-induced immunosuppression. Nature (1998) 394(6696):897–901. doi:10.1038/29795
141. Abella V, Scotece M, Conde J, Pino J, Gonzalez-Gay MA, Gómez-Reino, et al. Leptin in the interplay of inflammation, metabolism and immune system disorders. Nat Rev Rheumatol (2017) 13(2):100–9. doi:10.1038/nrrheum.2016.209
142. Caldefie-Chezet F, Poulin A, Vasson MP. Leptin regulates functional capacities of polymorphonuclear neutrophils. Free Radic Res (2003) 37(8):809–14. doi:10.1080/1071576031000097526
143. Conus S, Bruno A, Simon HU. Leptin is an eosinophil survival factor. J Allergy Clin Immunol (2005) 116(6):1228–34. doi:10.1016/j.jaci.2005.09.003
144. Wong MM, Richard MK, Ng PK, Law SH, Tsang AK, Kong RY. Characterization of a hypoxia-responsive leptin receptor (omLepR(L)) cDNA from the marine medaka (Oryzias melastigma). Mar Pollut Bull (2007) 54(6):797–803. doi:10.1016/j.marpolbul.2007.01.025
145. Kato H, Ueki S, Kamada R, Kihara J, Yamauchi Y, Suzuki T, et al. Leptin has a priming effect on eotaxin-induced human eosinophil chemotaxis. Int Arch Allergy Immunol (2011) 155(4):335–44. doi:10.1159/000321195
146. Suzukawa M, Nagase H, Ogahara I, Han K, Tashimo H, Shibui A, et al. Leptin enhances survival and induces migration, degranulation, and cytokine synthesis of human basophils. J Immunol (2011) 186(9):5254–60. doi:10.4049/jimmunol.1004054
147. Santos-Alvarez J, Goberna R, Sanchez-Margalet V. Human leptin stimulates proliferation and activation of human circulating monocytes. Cell Immunol (1999) 194(1):6–11. doi:10.1006/cimm.1999.1490
148. Lam QL, Wang S, Ko OK, Kincade PW, Lu L. Leptin signaling maintains B-cell homeostasis via induction of Bcl-2 and Cyclin D1. Proc Natl Acad Sci U S A (2010) 107(31):13812–7. doi:10.1073/pnas.1004185107
149. Agrawal S, Gollapudi S, Su H, Gupta S. Leptin activates human B cells to secrete TNF-alpha, IL-6, and IL-10 via JAK2/STAT3 and p38MAPK/ERK1/2 signaling pathway. J Clin Immunol (2011) 31(3):472–8. doi:10.1007/s10875-010-9507-1
150. Saucillo DC, Gerriets VA, Sheng J, Rathmell JC, MacIver NJ. Leptin metabolically licenses T cells for activation to link nutrition and immunity. J Immunol (2014) 192(1):136–44. doi:10.4049/jimmunol.1301158
151. Mariano G, Terrazzano G, Coccia E, Vito P, Varricchio E, Paolucci M. Effects of recombinant trout leptin in superoxide production and NF-kappaB/MAPK phosphorylation in blood leukocytes. Peptides (2013) 48:59–69. doi:10.1016/j.peptides.2013.07.026
Keywords: leptin, energy homeostasis, stress, teleosts, metabolism, cortisol, appetite
Citation: Deck CA, Honeycutt JL, Cheung E, Reynolds HM and Borski RJ (2017) Assessing the Functional Role of Leptin in Energy Homeostasis and the Stress Response in Vertebrates. Front. Endocrinol. 8:63. doi: 10.3389/fendo.2017.00063
Received: 24 February 2017; Accepted: 23 March 2017;
Published: 07 April 2017
Edited by:
Maximilian Michel, University of Michigan, USAReviewed by:
Helene Volkoff, Memorial University of NewfoundlandMarnix Gorissen, Radboud University, Netherlands
Copyright: © 2017 Deck, Honeycutt, Cheung, Reynolds and Borski. This is an open-access article distributed under the terms of the Creative Commons Attribution License (CC BY). The use, distribution or reproduction in other forums is permitted, provided the original author(s) or licensor are credited and that the original publication in this journal is cited, in accordance with accepted academic practice. No use, distribution or reproduction is permitted which does not comply with these terms.
*Correspondence: Russell J. Borski, cnVzc2VsbF9ib3Jza2lAbmNzdS5lZHU=