- 1Department of Physiology, Faculty of Science, Mahidol University, Bangkok, Thailand
- 2Novo Nordisk Foundation Center for Basic Metabolic Research, Faculty of Health and Medical Sciences, University of Copenhagen, Copenhagen, Denmark
Exercise training triggers numerous positive adaptations through the regulation of genes controlling muscle structure and function. Epigenetic modifications, including DNA methylation, participate in transcriptional activation by allowing the recruitment of the transcription machinery to gene promoters. Exercise induces dynamic DNA demethylation at gene promoters; however, the contribution of the demethylation precursor hydroxymethylcytosine is unknown. Given the evanescent nature of hydroxymethylcytosine, a muscle contraction model that allows for the collection of samples that are repeatedly stimulated over time is required to determine whether contraction-induced demethylation is preceded by changes in the hydroxymethylcytosine level. Here, we established an acute skeletal muscle contraction model to mimic the effects of acute exercise on gene expression. We used this model to investigate the effect of muscle contraction on DNA demethylation and hydroxymethylation. First, we performed an acute exercise study in healthy humans to identify an exercise-responsive gene that we could study in culture. We identified the nuclear receptor subfamily 4 group A member 3 (Nr4a3) gene with the highest fold-expression increase after acute exercise. We then refined an electrical pulse stimulation (EPS) protocol that could induce expression of the Nr4a3 gene in C2C12 myotubes. Using targeted bisulfite sequencing, we found that in response to EPS, a region of the Nr4a3 promoter is rapidly demethylated at 60 min and re-methylated at 120 min. Of interest, hydroxymethylation of the differentially methylated region of Nr4a3 promoter after EPS was elevated immediately after EPS, with lowest levels reached at 60 min after EPS. In conclusion, we have established a cell culture-based protocol to mimic the acute transcriptional responses to exercise. Furthermore, we provide insight into the mechanism by which the exercise-responsive gene Nr4a3 is demethylated after muscle contraction.
Introduction
Physical exercise remodels skeletal muscle structure and function through the regulation of numerous genes controlling muscle metabolism, structure, and growth (1). At the DNA level, the regulation of gene expression is orchestrated by epigenetic mechanisms that coordinate the recruitment of transcription and cis-regulatory factors to gene promoters (2). DNA methylation and histone acetylation constitute the two major epigenetic modifications implicated in exercise-induced gene expression. Indeed, following an acute exercise bout, histone deacetylases 4 and 5 are exported to the nuclei of the muscle fiber, which allows increased acetylation of histone proteins and subsequent relaxation of chromatin at exercise-responsive loci (3). More recently, we have shown that muscle contraction induces dramatic demethylation at promoter regions of peroxisome proliferator-activated receptor delta (PPARD), pyruvate dehydrogenase lipoamide kinase isozyme 4 (PDK4), and peroxisome proliferator-activated receptor gamma coactivator 1-alpha (PPARGC1A) (4), suggesting that targeted DNA demethylation facilitates the initiation of the transcription machinery at exercise-responsive genes.
Active DNA demethylation occurs in non-dividing cell through a pathway involving the ten-eleven translocation (TET) enzymes, which catalyze several steps of the demethylation process, notably by the generation of hydroxymethyl cytosine, an intermediate for demethylation (5). To date, the effect of muscle contraction on hydroxymethylation at exercise-responsive genes is unknown. The fugacity of hydroxymethylation intermediate in the DNA demethylation process is likely to account for this lack of evidence. To address this question, a cell-based model amenable to the collection of data points at short intervals is required. Several ex vivo or in vitro models exist to study muscle contraction. Cell culture models represent the “in vitro” models of choice for time series investigations. Various cell culture models using electrical stimulation have been proposed (6–13); however, these models mimic long-term contraction and are aiming at inducing changes in protein abundance. Thus, they are not suitable for investigating the acute effect of muscle cell (myotube) contraction on mRNA expression.
In this study, we established an acute skeletal muscle contraction model that partly mimics the effect of exercise on gene expression. We used this model to investigate the effect of muscle contraction on DNA methylation remodeling. We refined an electrical pulse stimulation (EPS) protocol in differentiated C2C12 myotubes that we benchmarked against changes in the expression of nuclear receptor subfamily 4 group A member 3 (Nr4a3), a gene selected based on our observation that it shows the highest fold increase in expression after acute exercise in untrained humans. Using this assay, we provide evidence that the Nr4a3 promoter is rapidly hydroxymethylated prior to demethylation, supporting a role for hydroxymethylation in contraction-induced DNA methylation remodeling in skeletal muscle.
Materials and Methods
Acute Exercise Study
Ten healthy Danish male volunteers, aged between 19 and 25 (22.6 ± 1.6) years old, were recruited to participate in the study. The participants were sedentary and had a body mass index between 19.5 and 28.5 (23.1 ± 2.8, more clinical characteristics can be found in Table S1 in Supplementary Material). All participants reported to the laboratory and completed a peak pulmonary oxygen uptake test under fasting conditions to determine their VO2 max. This test consisted of an incremental exercise bout to volitional fatigue on an electromagnetically braked cycle ergometer (Monark Ergomedic 839E) during which pulmonary gas exchange was measured breath-by-breath with a gas analyzing system (Oxycon Pro, Jaeger). The participants then performed an intense (80% VO2 max) 15-min exercise bout and skeletal muscle biopsies were collected under fasting conditions at rest (basal) and 4 h after cessation of exercise. The aerobic (rather than resistance) exercise was chosen since the literature reporting EPS model describe genes that respond to aerobic exercise (6, 12). The 4-h time point was chosen based on previous observations from our group and others, which peaked that mRNA expression is seen 3–5 h after exercise (1, 4). Biopsies were obtained from the Vastus lateralis muscle using the Bergström needle technique (14), and they were immediately snap-frozen in liquid nitrogen and stored at −80°C for further analysis.
This study was carried out in accordance with the recommendations of the Ethic Committee from the Capital Region of Denmark with written informed consent from all subjects. All subjects gave written informed consent in accordance with the Declaration of Helsinki. The protocol was approved by the Ethic Committee from the Capital Region of Denmark (reference H-1-2013-064).
Total RNA Purification
Total RNA was purified from the skeletal muscle biopsy samples using an AllPrep DNA/RNA/miRNA Universal Kit (Qiagen) following manufacturer’s instructions. The quality of recovered RNA was assessed by using the Agilent RNA 6000 Nano Kit (Agilent Technologies). RNA concentration was determined by spectrophotometry using a NanoDrop 2000. After purification, RNA samples were stored at −80°C until further analysis.
RNA Sequencing
Libraries were prepared after ribosomal RNA depletion with the TruSeq Stranded Total Sample Prep Kit with Ribo-Zero Gold (Illumina). Sequencing was done on a HiSeq 2500, single-read, 100 bp read length per lane. Reads were trimmed for adapters and low quality flanking ends using Trim Galore v0.3.7 and Cutadapt v1.4.2 (15). Pre-processed reads were mapped to the human reference genome (hg19) with tophat2 using the “--b2-very-sensitive” setting (16, 17), and gene coverages were computed with featureCounts (18) using the Gencode v19 annotation. Genes with a coverage larger than 2.5 RPKM in at least five samples were tested for differential expression with edgeR using the glmQLFit/glmQLFTest modeling framework (19).
Cell Culture
Mouse skeletal muscle cell line C2C12 (ATCC CRL1772) were seeded into six-well plates at a density of 105 cells/well, cultured in growth medium containing Dulbecco’s modified Eagle’s medium (25 mM glucose) supplemented with 10% fetal bovine serum (FBS), and 1% penicillin–streptomycin at 37°C, 5% CO2. After reaching ~90% confluence, differentiation was induced by switching 10% FBS to 2% horse serum. Differentiation medium was changed every day until fully differentiated myotubes were visualized under a light microscope (5–6 days).
Electrical Pulse Stimulation
A six-well plate, containing differentiated C2C12 myotubes was connected to C-dish electrode (IonOptix). The electrical current was discharged from a carbon electrode that was immersed in 8 ml differentiation medium added 24 h before the EPS. EPS was applied by C-pace cell culture stimulator (C-pace EP, IonOptix). EPS-induced muscle contraction was validated using light microscopy. Voltage, frequency, pulse duration, and stimulation time were optimized to induce reproducible increases in mRNA expression of exercise-responsive genes (Figure 1). Cells were harvested immediately after EPS at 0, 30, 60, 120, 180, and 240 min.
Gene Expression Analysis
Total RNA was isolated from cells with TRIzol (Invitrogen) or AllPrep DNA/RNA/miRNA universal kit (Qiagen) according to the manufacturer’s instructions. RNA was converted to cDNA by iScript cDNA synthesis kit (Bio-Rad). Gene expression was determined by quantitative real-time PCR (qPCR) using Brilliant III Ultra-Fast SYBR Green Master mix (Agilent Technologies). Sequences of qPCR primers used for Ppargc1a, Ppard, Cyclin D1 (Ccnd1), and Nr4a3 genes are provided (Table S2 in Supplementary Material). mRNA expression was quantified by normalizing Ct values to cDNA amounts.
DNA Isolation
Genomic DNA was isolated from the muscle cell cultures using an AllPrep DNA/RNA/miRNA Universal kit (Qiagen) according to the manufacturer’s instructions.
DNA Methylation Analysis
Genomic DNA was bisulfite-converted using EZ DNA Methylation-Lightning Kit (Zymo Research) according to the manufacturer’s instructions. Bisulfite primers were designed to span the promoter region of Ppard, Ccnd1, and Nr4a3 genes within −3,000 to +2,000 bp relative to transcriptional start site (TSS) (Table S2 in Supplementary Material) using MethPrimer program (20). All bisulfite primers were mixed at equimolar ratio in a multiplexed PCR reaction using 200 ng of DNA as starting material and the HotStarTaq Plus DNA Polymerase kit (Qiagen). The PCR program was run at 95°C for 5 min, 35 cycles of 94°C (1 min) 60°C (1 min) 72°C (1 min), followed by 72°C for 10 min. PCR products were purified using MinElute PCR purification Kit (Qiagen). DNA libraries were prepared using NEBNext Ultra DNA Library Prep Kit for Illumina (New England Biolabs) and sequenced on a Miseq instrument (Illumina) with 151 cycles paired-end run. After sequencing, reads were trimmed for adapters and low quality flanking ends using Trim Galore v0.3.7 and Cutadapt v1.4.2 (15). Pre-processed reads were mapped to the mm10 reference genome and DNA methylation levels quantified by Bismark v0.14.4 and Bowtie2 (21, 22).
DNA Hydroxymethylation Analysis
DNA (1 µg) was sheared into fragments (~200–300 bp) using a Bioruptor Plus sonicator (Diagenode). Hydroxymethylated DNA was captured from 220 ng of fragmented DNA using the hydroxymethylated DNA Immunoprecipitation (hMeDIP) ChIP Kit (Abcam) according to the manufacturer’s instructions. PCR primers were designed to cover a region of Nr4a3 promoter predetermined by DNA methylation analysis in response to EPS (Table S2 in Supplementary Material). DNA hydroxymethylation levels were determined by qPCR using Brilliant III Ultra-Fast SYBR Green Master mix (Agilent Technologies). Hydroxymethylated DNA was quantified by normalizing to total DNA input.
Data Analysis
Data are presented as mean ± SE. Statistical analysis was performed using Graphpad Prism 6 (GraphPad Software). Two-way ANOVA was performed to determine the effect of EPS on gene expression, DNA methylation, and hydroxymethylation. When differences were statistically significant, the difference between control (non-stimulated) and EPS groups at corresponding time points was compared by an unpaired t-test.
Results
Identification of Exercise-Responsive Genes in Humans
We performed RNA sequencing on human skeletal muscle biopsies collected before and 4 h after an acute exercise bout to identify genes showing the highest transcriptional response. Analysis of differential mRNA expression showed 165 differentially expressed genes (out of 6,242 genes detected), where 72 were over-expressed and 93 were under-expressed after acute exercise (Table 1; Table S3 in Supplementary Material). The most upregulated gene was the Nuclear Receptor subfamily 4 group A member 3 (NR4A3) (P = 3.41E−08; false discovery rate = 2.51E − 05), which increased 26-fold after acute exercise. Thus, we selected NR4A3 to benchmark a model of muscle contraction in cultured myotubes.
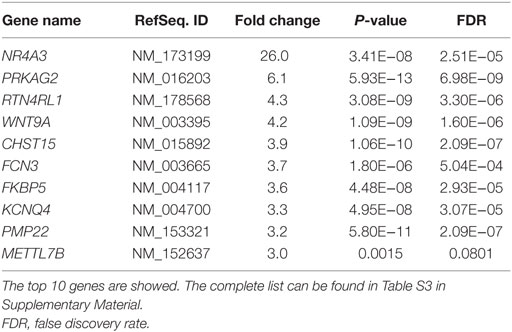
Table 1. Genes showing highest fold upregulation after acute exercise in skeletal muscle from young healthy humans.
EPS Mimics the Effect of Exercise on Nr4a3
Electrical pulse stimulation of cultured myotubes has been described (9, 11) and used to potentiate myotube differentiation (8) and increase the expression of specific genes, notably Ppargc1a (6). Here, we developed a novel EPS protocol to mimic the effects of acute exercise on the expression of Nr4a3. We tested several EPS conditions to stimulate C2C12 myotubes with contraction (Figure 1). While an EPS protocol applying 2 ms, 30 V, and 1 Hz had no effect on Ppargc1a mRNA expression (Figure 2A), it consistently induced the expression of Nr4a3 up to threefold at 60 min (Figure 2B) and also led to changes in mRNA expression of Ppard and Ccnd1 (Figures 2C,D). Thus, we selected this EPS protocol to study the epigenetic response of Nr4a3, Ccnd1, and Ppard after acute contraction.
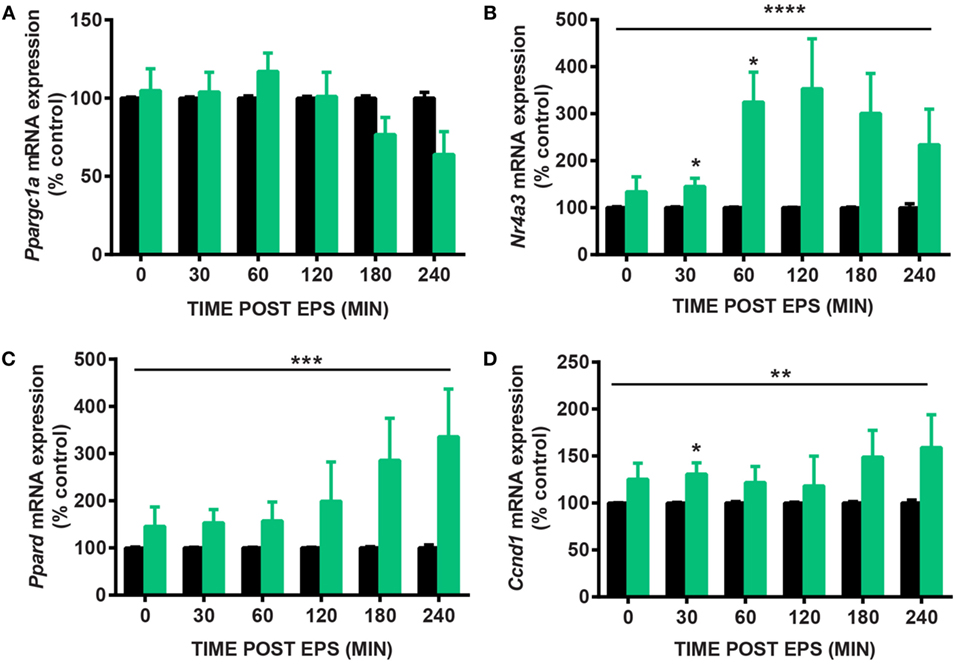
Figure 2. Gene expression changes after electrical pulse stimulation (EPS) contraction. C2C12 myotubes were subjected to EPS at 2 ms, 30 V, and 1 Hz for 30 min and harvested immediately (0), 30, 60, 120, 180, and 240 min after EPS. Quantitative real-time PCR measures of Ppargc1a (A), Nr4a3 (B), Ppard (C), and Ccnd1 (D) are shown for unstimulated cells (CON, black bars) and EPS-stimulated cells (EPS, green bars). For each time point, EPS value is expressed as percentage of control value which is set at 100%. Data are presented for four independent experiments as mean ± SE (*P < 0.05, **P < 0.01, ****P < 0.0001 EPS-stimulated vs. control).
EPS Induces Transient Nr4a3 Promoter Demethylation
We used targeted bisulfite sequencing to investigate the effect of EPS on Nr4a3, Ccnd1, and Ppard methylation. EPS altered the methylation status of several cytosines located in a region encompassing −2,201 to −2,081 bp relative to the TSS of the Nr4a3 gene (Figure 3A). Methylation of this region was inversely associated with Nr4a3 mRNA expression measured 60 min after EPS, at a time point where methylation was decreased (Figures 2B and 3B). Interestingly, Nr4a3 methylation was increased at 180 min, which marks the time point where Nr4a3 mRNA expression started to decrease (Figures 2B and 3B). EPS-induced transient methylation of the region +389 to +566 bp relative the TSS of the Ccnd1 gene at 30 and 60 min post-EPS (Figure 3C), which was associated with elevated mRNA expression at the 30-min time point (Figure 2D), suggesting hypermethylation is secondary to gene expression. Of potential interest, a wave of demethylation on the Ccnd1 promoter was detected at 120 min post-EPS, with mRNA expression tending to increase at the following two time points (Figure 2D). Similarly, methylation level of the Ppard promoter at a region of +706 to +852 bp relative to TSS was decreased at 60 min post-EPS (Figure 3D), which was followed by a progressive increase in Ppard mRNA expression in the following time points (Figure 2C). Our results provide evidence that EPS induces DNA methylation remodeling. Furthermore, we also show that contraction-induced DNA demethylation precedes transcriptional activation.
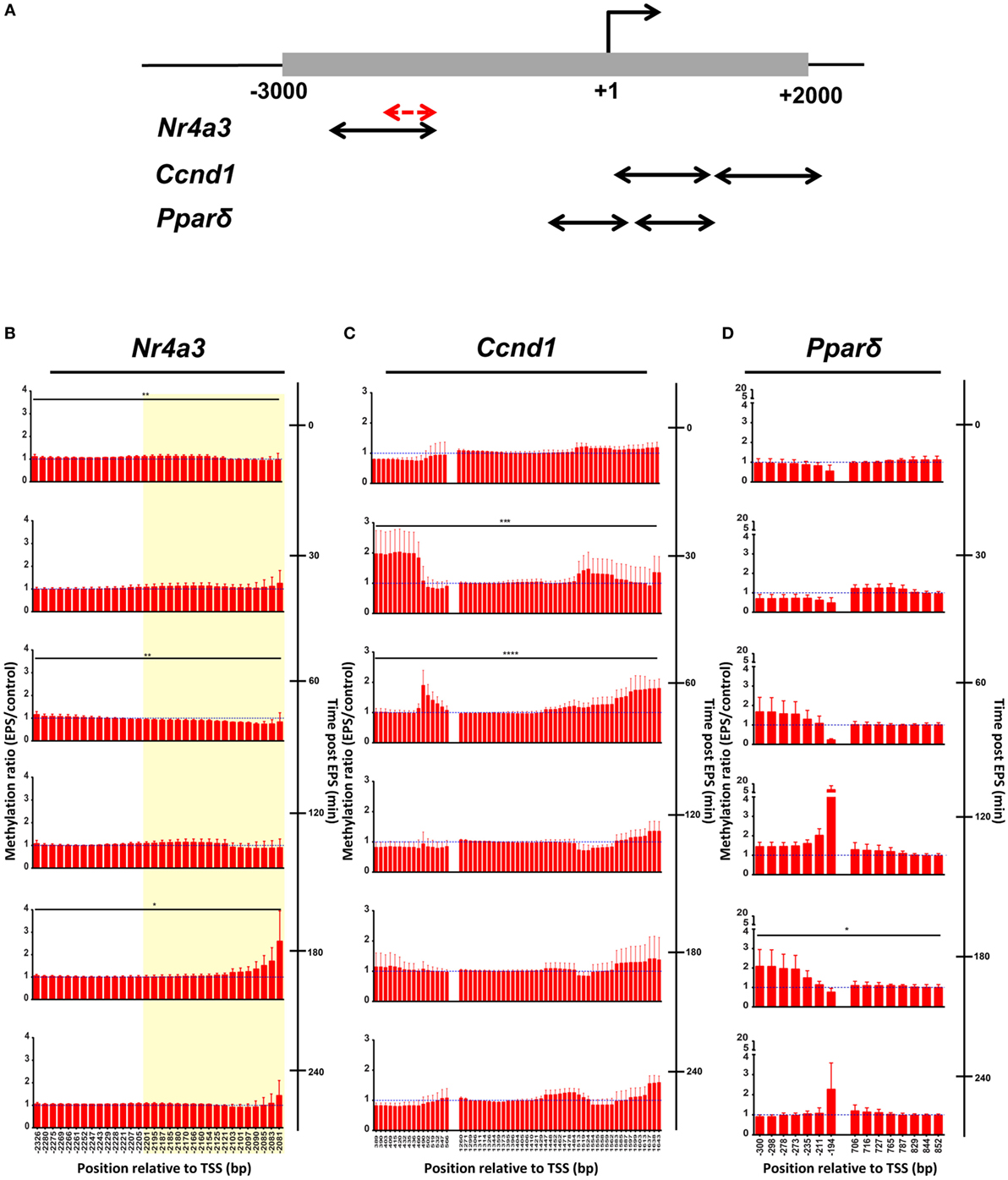
Figure 3. DNA methylation is remodeled by electrical pulse stimulation (EPS) contraction. (A) Schematic view of regions analyzed by bisulfite sequencing. One to two pairs of primers were designed for each gene. All primers were located within −3,000 to +2,000 bp relative to transcriptional start site (black arrows). A fraction of Nr4a3 promoter was further selected for hydroxymethylation analysis in Figure 4 (red arrows) and highlighted in yellow area on the Nr4a3 graph (B). DNA methylation of Nr4a3 (B), Ccnd1 (C), and Ppard (D). Methylation ratios between the unstimulated control cells and EPS-stimulated at various time points are shown. For each time point, ratio of 1 is indicated by a blue, hatched line. Data are presented for three independent experiments as mean ± SE (*P < 0.05, **P < 0.01, ***P < 0.001, ****P < 0.0001 EPS-stimulated vs. control).
EPS Rapidly Increases Hydroxymethylation of the Nr4a3 Promoter
Given that EPS induced robust change in mRNA expression and demethylation of the Nr4a3 gene, we investigated the amount of the demethylation precursor hydroxymethylcytosine in the region −2,201 to −2,081 bp relative to the TSS of Nr4a3. We used hydroxymethylcytosine capture followed by quantitative PCR to gain further insight into the potential mechanisms by which EPS-induced myotube contraction induces Nr4a3 demethylation. We detected a threefold increase in hydroxymethylation levels of Nr4a3 immediately after EPS-induced contraction (0 min time point, Figure 4). This increase was transient, with the hydroxymethylation level restored to the baseline level by 30 min and further decreased at the 60 and 120 min time points (Figure 4). As the methylation level corresponding to this region was decreased at 60 and 120 min (Figure 3A), our results strongly suggest that contraction-induced Nr4a3 promoter demethylation occurs through intermediate hydroxymethylation.
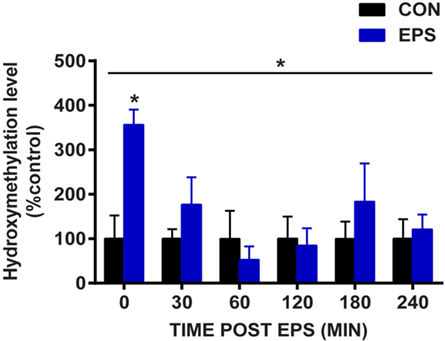
Figure 4. Electrical pulse stimulation (EPS) increases hydroxymethylcytosine levels in Nr4a3 promoter. DNA from unstimulated cells (CON, black bars) or subjected to EPS (EPS, blue bars) was analyzed for hydroxymethylation at a specific region of Nr4a3 (schematized in Figure 3A, red arrows) using hydroxymethylcytosine capture followed quantitative real-time PCR. For each time point, EPS value is expressed as percentage of control value which is set at 100%. Data for three independent experiments are presented as mean ± SE (*P < 0.05 EPS-stimulated vs. control).
Discussion
We identified NR4A3 as an exercise-responsive gene showing the highest fold change in mRNA expression using RNASeq of skeletal muscle biopsies collected from healthy untrained men after acute exercise. The Nr4a3 gene has previously been associated with enhanced metabolic function and endurance capacity (23). Consistent with our RNASeq data, NR4A3 has previously been reported to show the highest fold change in mRNA level in response to acute exercise (24, 25). In addition, Nr4a3 expression is increased in mouse skeletal muscle immediately after exercise or in response to two different types of exercise in combination with electrical stimulation (26). Based on these data, we established an EPS-based model of muscle contraction to mimic the effect of muscle contraction on the Nr4a3 gene.
Using an EPS protocol mimicking the effect of exercise in cultured muscle cells, we performed time course experiments and quantified gene expression of exercise-responsive genes. EPS altered mRNA level of Ccnd1 and Ppard, two genes respectively involved in muscle growth and metabolism and previously shown to be induced by muscle contraction (27, 28). In contrast, our EPS protocol failed to induce Ppargc1a mRNA expression although several studies including one from our group have reported that Ppargc1a expression is increased after a single bout of exercise (4, 25, 28, 29). The lack of response for Ppargc1a gene in our hands is likely due to the nature of the contraction protocol, as previous reports show Ppargc1a gene expression is induced by slow-type tetanic contraction (30). Conversely, an EPS protocol of 1 ms, 14 V, and 50 Hz at 1-s trains/1-s pauses on C2C12 induced Ppargc1a gene expression after 24 h, but not in cells stimulated for less than 90 min (6). Likewise, EPS contraction at 2 ms, 14 V, and 5 Hz of primary human myotubes induced PPARGC1A gene expression after 24 h, but failed to elicit changes after 2, 4, or 8 h of stimulation (12). Collectively, these data suggest that our EPS model can mimic some, but not all the features of muscle contraction in vivo. As exercise triggers several distinct intracellular events including calcium release or variations of the redox state that coordinate gene expression, the lack of gene expression response after EPS may be due to a lower magnitude in one particular intracellular signal or the absence of specific signals targeting a specific class of genes.
Exercise is associated with remodeling of the DNA methylation profile of several genes involved in glucose and lipid metabolism in skeletal muscle (4). Using our EPS protocol in cultured myotubes, we confirm that contraction alters DNA methylation status at promoter regions of specific genes. Depending on the type of assay used to detect DNA methylation, a loss in DNA methylation can be caused by a true demethylation or, by an additional modification on the methylated cytosine that escape detection. For example, DNA methylation capture assays relying on antibodies targeting 5-methylcytosine (MeDIP) would fail to distinguish between demethylation and hydroxylation of methylated cytosine. Bisulfite sequencing relies on the property of sodium bisulfite to convert unmodified cytosine to uracil and therefore, unlike MeDIP, can be used to detect true cytosine demethylation. Thus, in this study, we used bisulfite sequencing as a direct measure of DNA methylation. Our results provide evidence that EPS-stimulated myotube contraction induces active demethylation on the promoter of Nr4a3, Ccnd1, and Ppard. Using hydroxymethylation capture, we reveal that hydroxymethylcytosine levels are elevated immediately after EPS, with lowest levels observed 60 min thereafter, corresponding to a time point where demethylation has peaked. Active demethylation occurs through two distinct steps: the first step involves oxidative modifications of methylated cytosines to hydroxymethylcytosines by the TET enzymes and the second step corresponds directly to demethylation, where modified cytosines are replaced by cytosines by the base-excision repair machinery (31). Although we did not establish a direct relationship between methylated cytosine hydroxylation and DNA demethylation, our results support the notion that EPS contraction activates a TET enzyme and this leads to a hydroxymethylation-based demethylation of exercise-responsive genes.
Skeletal muscle contraction generates intracellular metabolites that may participate in demethylation of gene promoters (2). This hypothesis is supported by the recent observation that the intermediate metabolite of the Krebs cycle, alpha-ketoglutarate (aKG), a catalytic activator of the TET enzymes (31), is implicated in brown adipocyte differentiation through demethylation of the master regulator of brown adipocyte differentiation Prdm16 (32). In addition, activation of the AMP kinase by the pharmacological agent AICAR increases levels of aKG and this is associated with demethylation of Prdm16 (32). Thus, AMP kinase activation and fuel utilization during active muscle contraction could change the intracellular availability of aKG, which could in turn regulate TET-dependant DNA demethylation of exercise-responsive genes. Other intracellular metabolites could also participate in contraction-induced demethylation. For instance, intracellular availability of the antioxidant Vitamin C, an allosteric activator of TET enzymes (33), is likely to be influenced by contraction-induced changes in the redox state and may thereby modulate TET enzyme activity. Dietary interventions and metabolomic studies are warranted to assess the potential role of metabolite changes during muscle contraction and the effect of these metabolites on the epigenetic remodeling of exercise-responsive genes.
In conclusion, we have established an EPS model to further explore DNA demethylation after muscle contraction. We identified acute increase in hydroxymethylation levels followed by demethylation of the Nr4a3 promoter and proposed a role of DNA demethylation in the control of gene expression after exercise. Understanding how exercise orchestrates gene expression changes may provide therapeutic entry points for a plethora of age-related and metabolic diseases.
Data Availability
Bisulfite-Seq and RNA-Seq data are deposited in the NCBI Gene Expression Omnibus database with the accession number GSE87749.
Author Contributions
RB was the guarantor of this work and, as such, had full access to all the data in the study, and took responsibility for the integrity of the data and the accuracy of the data analysis. RB and PP contributed to the study design; PP and OF contributed to data acquisition; PP, CG, OF, and RB contributed to data analysis. All the authors contributed to data interpretation and manuscript drafting and approved the final version of the manuscript.
Conflict of Interest Statement
The authors declare that the research was conducted in the absence of any commercial or financial relationships that could be construed as a potential conflict of interest.
Acknowledgments
The authors would like to acknowledge The Danish National High-Throughput DNA Sequencing Centre, University of Copenhagen, for sequencing services. The authors would like to thank Dr. Ida Donkin for contribution in the human study, M. Emil Andersen for help with bisulfite sequencing, and Dr. Rhianna Laker for help with C2C12 cultures.
Funding
PP received a grant from the Thailand Research Fund (TRF) through the Royal Golden Jubilee (RGJ) PhD Program (PHD/0301/2551). OF received a fellowship from the Danish Diabetes Academy supported by the Novo Nordisk Foundation. The Novo Nordisk Foundation Center for Basic Metabolic Research is an independent Research Center at the University of Copenhagen partially funded by an unrestricted donation from the Novo Nordisk Foundation (www.metabol.ku.dk).
Supplementary Material
The Supplementary Material for this article can be found online at http://journal.frontiersin.org/article/10.3389/fendo.2016.00165/full#supplementary-material.
References
1. Egan B, Zierath JR. Exercise metabolism and the molecular regulation of skeletal muscle adaptation. Cell Metab (2013) 17(2):162–84. doi:10.1016/j.cmet.2012.12.012
2. Rasmussen M, Zierath JR, Barres R. Dynamic epigenetic responses to muscle contraction. Drug Discov Today (2014) 19(7):1010–4. doi:10.1016/j.drudis.2014.03.003
3. McGee SL, Fairlie E, Garnham AP, Hargreaves M. Exercise-induced histone modifications in human skeletal muscle. J Physiol (2009) 587(Pt 24):5951–8. doi:10.1113/jphysiol.2009.181065
4. Barres R, Yan J, Egan B, Treebak JT, Rasmussen M, Fritz T, et al. Acute exercise remodels promoter methylation in human skeletal muscle. Cell Metab (2012) 15(3):405–11. doi:10.1016/j.cmet.2012.01.001
5. Ito S, D’Alessio AC, Taranova OV, Hong K, Sowers LC, Zhang Y. Role of Tet proteins in 5mC to 5hmC conversion, ES-cell self-renewal and inner cell mass specification. Nature (2010) 466(7310):1129–33. doi:10.1038/nature09303
6. Burch N, Arnold AS, Item F, Summermatter S, Brochmann Santana Santos G, Christe M, et al. Electric pulse stimulation of cultured murine muscle cells reproduces gene expression changes of trained mouse muscle. PLoS One (2010) 5(6):e10970. doi:10.1371/journal.pone.0010970
7. Farmawati A, Kitajima Y, Nedachi T, Sato M, Kanzaki M, Nagatomi R. Characterization of contraction-induced IL-6 up-regulation using contractile C2C12 myotubes. Endocr J (2013) 60(2):137–47. doi:10.1507/endocrj.EJ12-0316
8. Lambernd S, Taube A, Schober A, Platzbecker B, Gorgens SW, Schlich R, et al. Contractile activity of human skeletal muscle cells prevents insulin resistance by inhibiting pro-inflammatory signalling pathways. Diabetologia (2012) 55(4):1128–39. doi:10.1007/s00125-012-2454-z
9. Manabe Y, Miyatake S, Takagi M, Nakamura M, Okeda A, Nakano T, et al. Characterization of an acute muscle contraction model using cultured C2C12 myotubes. PLoS One (2012) 7(12):e52592. doi:10.1371/journal.pone.0052592
10. Nedachi T, Fujita H, Kanzaki M. Contractile C2C12 myotube model for studying exercise-inducible responses in skeletal muscle. Am J Physiol Endocrinol Metab (2008) 295(5):E1191–204. doi:10.1152/ajpendo.90280.2008
11. Nikolic N, Bakke SS, Kase ET, Rudberg I, Flo Halle I, Rustan AC, et al. Electrical pulse stimulation of cultured human skeletal muscle cells as an in vitro model of exercise. PLoS One (2012) 7(3):e33203. doi:10.1371/journal.pone.0033203
12. Scheler M, Irmler M, Lehr S, Hartwig S, Staiger H, Al-Hasani H, et al. Cytokine response of primary human myotubes in an in vitro exercise model. Am J Physiol Cell Physiol (2013) 305(8):C877–86. doi:10.1152/ajpcell.00043.2013
13. Whitham M, Chan MH, Pal M, Matthews VB, Prelovsek O, Lunke S, et al. Contraction-induced interleukin-6 gene transcription in skeletal muscle is regulated by c-Jun terminal kinase/activator protein-1. J Biol Chem (2012) 287(14):10771–9. doi:10.1074/jbc.M111.310581
14. Bergstrom J. Percutaneous needle biopsy of skeletal muscle in physiological and clinical research. Scand J Clin Lab Invest (1975) 35(7):609–16. doi:10.3109/00365517509095787
15. Martin M. Cutadapt removes adapter sequences from high-throughput sequencing reads. EMBnet.journal (2011) 17(1):10–2. doi:10.14806/ej.17.1.200
16. Kim D, Pertea G, Trapnell C, Pimentel H, Kelley R, Salzberg SL. TopHat2: accurate alignment of transcriptomes in the presence of insertions, deletions and gene fusions. Genome Biol (2013) 14(4):R36. doi:10.1186/gb-2013-14-4-r36
17. Liao Y, Smyth GK, Shi W. The Subread aligner: fast, accurate and scalable read mapping by seed-and-vote. Nucleic Acids Res (2013) 41(10):e108. doi:10.1093/nar/gkt214
18. Liao Y, Smyth GK, Shi W. featureCounts: an efficient general purpose program for assigning sequence reads to genomic features. Bioinformatics (2014) 30(7):923–30. doi:10.1093/bioinformatics/btt656
19. Robinson MD, McCarthy DJ, Smyth GK. edgeR: a bioconductor package for differential expression analysis of digital gene expression data. Bioinformatics (2010) 26(1):139–40. doi:10.1093/bioinformatics/btp616
20. Li LC, Dahiya R. MethPrimer: designing primers for methylation PCRs. Bioinformatics (2002) 18(11):1427–31. doi:10.1093/bioinformatics/18.11.1427
21. Krueger F, Andrews SR. Bismark: a flexible aligner and methylation caller for bisulfite-seq applications. Bioinformatics (2011) 27(11):1571–2. doi:10.1093/bioinformatics/btr167
22. Langmead B, Salzberg SL. Fast gapped-read alignment with Bowtie 2. Nat Methods (2012) 9(4):357–9. doi:10.1038/nmeth.1923
23. Pearen MA, Goode JM, Fitzsimmons RL, Eriksson NA, Thomas GP, Cowin GJ, et al. Transgenic muscle-specific Nor-1 expression regulates multiple pathways that effect adiposity, metabolism, and endurance. Mol Endocrinol (2013) 27(11):1897–917. doi:10.1210/me.2013-1205
24. Catoire M, Mensink M, Boekschoten MV, Hangelbroek R, Muller M, Schrauwen P, et al. Pronounced effects of acute endurance exercise on gene expression in resting and exercising human skeletal muscle. PLoS One (2012) 7(11):e51066. doi:10.1371/journal.pone.0051066
25. Mahoney DJ, Parise G, Melov S, Safdar A, Tarnopolsky MA. Analysis of global mRNA expression in human skeletal muscle during recovery from endurance exercise. FASEB J (2005) 19(11):1498–500. doi:10.1096/fj.04-3149fje
26. Kawasaki E, Hokari F, Sasaki M, Sakai A, Koshinaka K, Kawanaka K. Role of local muscle contractile activity in the exercise-induced increase in NR4A receptor mRNA expression. J Appl Physiol (1985) (2009) 106(6):1826–31. doi:10.1152/japplphysiol.90923.2008
27. Haddad F, Adams GR. Aging-sensitive cellular and molecular mechanisms associated with skeletal muscle hypertrophy. J Appl Physiol (1985) (2006) 100(4):1188–203. doi:10.1152/japplphysiol.01227.2005
28. Russell AP, Hesselink MK, Lo SK, Schrauwen P. Regulation of metabolic transcriptional co-activators and transcription factors with acute exercise. FASEB J (2005) 19(8):986–8. doi:10.1096/fj.04-3168fje
29. Lochmann TL, Thomas RR, Bennett JP Jr, Taylor SM. Epigenetic modifications of the PGC-1alpha promoter during exercise induced expression in mice. PLoS One (2015) 10(6):e0129647. doi:10.1371/journal.pone.0129647
30. Tavi P, Westerblad H. The role of in vivo Ca(2)(+) signals acting on Ca(2)(+)-calmodulin-dependent proteins for skeletal muscle plasticity. J Physiol (2011) 589(Pt 21):5021–31. doi:10.1113/jphysiol.2011.212860
31. Wu H, Zhang Y. Reversing DNA methylation: mechanisms, genomics, and biological functions. Cell (2014) 156(1–2):45–68. doi:10.1016/j.cell.2013.12.019
32. Yang Q, Liang X, Sun X, Zhang L, Fu X, Rogers CJ, et al. AMPK/alpha-ketoglutarate axis dynamically mediates DNA demethylation in the Prdm16 promoter and brown adipogenesis. Cell Metab (2016) 156(1–2):45–68. doi:10.1016/j.cmet.2016.08.010
Keywords: skeletal muscle, muscle contraction, gene expression regulation, epigenetics, DNA methylation
Citation: Pattamaprapanont P, Garde C, Fabre O and Barrès R (2016) Muscle Contraction Induces Acute Hydroxymethylation of the Exercise-Responsive Gene Nr4a3. Front. Endocrinol. 7:165. doi: 10.3389/fendo.2016.00165
Received: 13 October 2016; Accepted: 09 December 2016;
Published: 23 December 2016
Edited by:
Tarik Issad, INSERM, FranceReviewed by:
Caroline Cieniewski-Bernard, Lille University of Science and Technology, FranceDonny Michael Camera, Australian Catholic University, Australia
Copyright: © 2016 Pattamaprapanont, Garde, Fabre and Barrès. This is an open-access article distributed under the terms of the Creative Commons Attribution License (CC BY). The use, distribution or reproduction in other forums is permitted, provided the original author(s) or licensor are credited and that the original publication in this journal is cited, in accordance with accepted academic practice. No use, distribution or reproduction is permitted which does not comply with these terms.
*Correspondence: Romain Barrès, YmFycmVzQHN1bmQua3UuZGs=