- 1Combat Protection and Performance Program, DSO National Laboratories, Defence Medical and Environmental Research Institute, Singapore
- 2Department of Physiology, Yong Loo Lin School of Medicine, National University of Singapore, Singapore
- 3Division of Endocrinology, Department of Medicine, Khoo Teck Puat Hospital, Singapore
- 4Institute for Memory Impairments and Neurological Disorders (MIND Institute), University of California Irvine, Irvine, CA, USA
Obesity is a major public health problem in the twenty-first century. Mutations in genes that regulate substrate metabolism, subsequent dysfunction in their protein products, and other factors, such as increased adipose tissue inflammation, are some underlying etiologies of this disease. Increased inflammation in the adipose tissue microenvironment is partly mediated by the presence of cells from the innate and adaptive immune system. A subset of the innate immune population in adipose tissue include macrophages, termed adipose tissue macrophages (ATMs), which are central players in adipose tissue inflammation. Being extremely plastic, their responses to diverse molecular signals in the microenvironment dictate their identity and functional properties, where they become either pro-inflammatory (M1) or anti-inflammatory (M2). Endurance exercise training exerts global anti-inflammatory responses in multiple organs, including skeletal muscle, liver, and adipose tissue. The purpose of this review is to discuss the different mechanisms that drive ATM-mediated inflammation in obesity and present current evidence of how exercise training, specifically endurance exercise training, modulates the polarization of ATMs from an M1 to an M2 anti-inflammatory phenotype.
Introduction
The immune system is instrumental in mediating a number of physiological processes in the mammalian species, including pathogen surveillance, wound repair, and metabolic regulation. Accumulating evidence shows that the immune system interacts with other organ systems, including the adipose tissue. Traditionally, adipose tissue is known for its role in energy homeostasis, especially as a storage depot for lipids. The scientific paradigm of this once-neglected organ shifted, when researchers discovered novel secretory functions of adipose tissue in the mid-1990s. Seminal studies demonstrated that gene and protein products of hormones that regulate satiety, such as leptin, were found to be overexpressed in, and secreted from adipocytes (1, 2).
Adipose tissue was further recognized as an endocrine-like organ, particularly after adipose tissue per se was shown to express and secrete cytokines that can exert effects in distant organs, such as tumor necrosis factor (TNF)-α and interleukin (IL)-6, among other cytokines previously thought to originate only from immune cells (3, 4). Cytokines, hormones, and other protein factors secreted from adipose tissue were subsequently termed “adipokines,” and they can exhibit autocrine, paracrine, and endocrine functions (5). Since then, adipose tissue has also been recognized to be in a chronic inflammatory state in an obese host, wherein immune cells, such as macrophages, were found in greater abundance – 40% of all cells within white adipose tissue (WAT) of obese mice, relative to 10% in lean mice (6). In addition, this increased macrophage density correlated with glucose and insulin resistance (7). In an obese state, more than 90% of all adipose tissue macrophages (ATMs) were found at sites of adipocyte death, where they form a crown-like structure (8) and participate in tissue remodeling, such as scavenging lipids from necrotic adipocytes (9) or inducing vessel growth (10).
Resident macrophages are phenotypically heterogeneous, where two distinct forms of macrophages, M1 and M2 phenotypes, are found within adipose tissue of both obese and lean individuals (or mice), with the extent of each phenotype dependent on local signals from the adipose microenvironment. In general, murine studies have demonstrated that excess adiposity increases the proportion of M1 to M2 macrophages in WAT (8). Lumeng’s group reported that after diet-induced obesity, murine ATMs had high gene expressions of cluster of differentiation (CD)11c+, TNF-α, and inducible nitric oxide synthase (iNOS), which are markers characteristic of M1 macrophages, whereas ATMs from lean mice expressed many genes characteristic of M2 macrophages, including arginase 1 (Arg1), Ym1, and IL-10 (8).
A Caveat on M1/M2 Macrophage Phenotypes
It is important to note that the M1/M2 nomenclature is an oversimplification of the polarized macrophage phenotype, with other subsets, such as M2a that can also be found in adipose tissue, although the proportions would vary based on physiological conditions. For example, the ratio of M2a to M1 murine ATMs in high-fat diet-induced obesity was approximately 1.2:1, but in lean mice it was 4:1 (11). Furthermore, although CD11c+ M1 macrophages accumulate after 8 weeks in adipose tissue of high-fat diet fed mice, these macrophages also presented with increased M2 macrophage gene expression (Arg1, IL-1ra) as well as other genes related to matrix remodeling (12). It is interesting to note that after 12 weeks of the high-fat diet, the ATMs presented with decreased M1 macrophage gene transcripts (e.g., IL-1β) and upregulated M2 macrophage gene transcripts (e.g., Ym1). Moreover, genes involved in oxidative metabolism, such as peroxisome proliferator-activated receptor (PPAR) gamma co-activator 1-alpha (PGC-1α) were found to be upregulated (12). Hence, the polarized state of ATMs is not constant, but in dynamic flux. Studies that have single time-point measurements of ATMs may, thus, miss the dynamic changes in M1 and M2 populations during obesity and exercise intervention. In addition, a recent study by Kratz’s group (13) demonstrated that polarization of ATMs by metabolic substrates, such as palmitate and glucose in vitro induced a distinct form of macrophage, which did not express surface markers coincident with classical activation, but rather, upregulated proteins involved in lipid metabolism, such as CD36, ATP-binding cassette transporter (ABCA)-1 and Perilipin-2, which are associated with M2 macrophages. The data from this group further suggest that the M1 and M2 paradigm is more complex, and in metabolic disease, may need further elucidation of the polarized phenotypes to better distinguish from other disease models.
In view of the diverse phenotypes reported in the literature, we would like to recommend a standardized experimental approach to harmonize future research findings in this area. At the International Congress of Immunology in Milan in 2013, a group of macrophage biologists met to discuss the current limitations in the literature pertaining to the activation or polarization of macrophages (14). The major challenge with the macrophage nomenclature stems from the use of terminology, where in addition to M1 and M2, other terms such as “classical” and “alternative,” “regulatory” and subsets of M1 and M2 have been described. Such nomenclatures resulted from disparate experimental approaches, for instance, whether macrophages were activated in vitro by IL-4 or IFN-γ, which gave rise to “alternative” and “classical” macrophage phenotypes, respectively. Alternatively, M1 or M2 classification has also been reported, depending on the growth factors used, for instance, granulocyte macrophage colony-stimulating factor (GM-CSF) or CSF-1 have been used to denote M1 and M2 macrophages cultured in vitro with these growth factors, respectively. Hence, at the Milan congress, the macrophage biologists came up with a framework for studies reporting macrophage phenotypes. The framework essentially calls for investigators to be more precise in (i) describing the source of macrophages (bone marrow, peritoneal, or peripheral macrophages), (ii) specifying the conditions in which macrophages were activated, and (iii) to use a nomenclature consistent with the spectrum of activation states (14).
In this review, we will use the generic nomenclature to denote M1 vs. M2 macrophage phenotypes, with the premise that an M1 phenotype is pro-inflammatory in that these macrophages produce cytokines, such as TNF-α, IL-6, and IL-12, whereas a M2 phenotype is anti-inflammatory, and these M2 macrophages produce large amounts of anti-inflammatory cytokines, such as IL-10 and IL-1 decoy receptor (8). In the next few sections, we will discuss how the excess adiposity contributes to molecular changes in the adipose tissue, leading to the activation and polarization of ATMs, and how regular exercise may reverse the macrophage phenotypes seen in obesity.
Obesity-Related Metabolic Stress, Inflammation, and Macrophage Polarization
Obesity- and exercise-induced macrophage polarization requires an integration of metabolic and immune crosstalk. First, excess adipocyte lipid availability during obesity increases (i) the availability of fatty acids for activation of immune and metabolic mediators of inflammatory response, including toll-like receptor (TLR)-4, nuclear factor-kappa B (NF-κB), IkB kinase (IKK)-β, Jun kinase (JNK)-1, fatty acid binding proteins (FABPs), PPAR(s), (ii) cellular stress [unfolded protein response (UPR) in the endoplasmic reticulum], (iii) mitochondrial reactive oxygen species (ROS) production and mitochondrial dysfunction, and (iv) protein synthesis (mammalian target of rapamycin (mTOR) hyperactivation) (15–17). Each of these factors will be discussed in turn.
Excess Availability of Fatty Acids
In the obese state, increased concentrations of saturated fatty acids activate (i) TLR4 in both adipocytes and macrophages (18), (ii) NF-κB in adipocytes (19), (iii) IKKβ in myeloid cells (20), and (iv) JNK in adipocytes (21), all of which activate downstream inflammatory cytokines and proteins, such as TNF-α, IL-6, and iNOS (16). These pro-inflammatory cytokines and proteins participate in a feedback loop between adipocytes and circulating monocytes, culminating in the M1 polarization of macrophages that infiltrate the adipose tissue.
Although well characterized as a cellular lipid chaperone, ligand-bound FABP4 also demonstrates novel roles in inflammation and in its interaction with nuclear receptors, such as PPARs (15). FABP4−/− macrophages demonstrated impaired IKK and NF-κB activity, concomitant with a reduction in protein expressions of cyclooxygenase (COX)-2 and iNOS, as well as lower LPS-stimulated secretions of monocyte chemotactic protein (MCP)-1, TNF-α, and IL-6 (22). Obesity-associated hyperlipidemia presents excess fatty acids that bind to FABP4 in adipocytes or stromal macrophages in the adipose tissue microenvironment, inducing the secretion of pro-inflammatory cytokines that recruit greater numbers of M1 macrophages. This view is supported by co-culture experiments where deletion of FABP4 in adipocytes resulted in MCP-1 gene expression in macrophage, and deletion of FABP4 in macrophages improved insulin signaling and glucose uptake in adipocytes (23).
Peroxisome proliferator-activated receptors are transcription factors that can be activated by fatty acids, and belong to the nuclear receptor family (24). They are expressed in adipose tissue, skeletal muscle, liver, macrophages, and other organs, although the three isoforms (α, δ, and γ) are tissue specific (25). Both PPARγ and PPARδ expressions are negatively associated with obesity, where they modulate adipogenesis and lipid oxidation, respectively (26). In addition, PPARδ is required for inducing macrophage M2 polarization, as PPARδ−/− macrophages demonstrated a decrease in M2 macrophage profile expression, as gene expressions of IL-13, IL-4, and macrophage galactose N-acetyl-galactosamine were all reduced, compared with wild-type macrophages (27). In addition, myeloid-specific PPARδ−/− mice demonstrated an increase in gene expression of M1 macrophage markers (MCP-1, TNF-α, IL-6) (27). Similarly, PPARγ−/− macrophages are polarized toward the M1 phenotype (28), suggesting that PPARs play a role in mediating macrophage polarization.
Mitochondrial ROS, Cellular Stress, and Aberrant Protein Synthesis
Obesity augments metabolic stress in the organism. At the cellular level, this is demonstrated through mitochondrial dysfunction and mTOR hyperactivation, whereby the integrated cellular signaling of these two pathological conditions can contribute to ER stress (15). Excess lipids in the adipocyte increase the substrate load for inefficient mitochondrial oxidative phosphorylation, leading to generation of ROS that can damage mitochondrial constituents, and perpetuate a cycle of ROS-induced damage and further mitochondrial dysfunction. Adipocyte mitochondrial dysfunction permits further excess cellular lipid build-up, leading to the production of the pro-inflammatory cytokines described earlier and recruitment and polarization of M1 macrophages. Overabundance of lipids in the adipocyte is sensed by mTOR as a high intracellular energy state, leading to hyperactivation of mTOR, which chronically can lead to uncontrolled protein synthesis, increased ER stress, UPR, and JNK activation (15). Lipid overload can also directly mediate the inflammatory response by inducing ER stress, via the initiation of UPR (15). The UPR activates cyclic-AMP-responsive element-binding protein H (CREBH), which induces C-reactive protein (CRP) and serum amyloid P-component (SAP) production, both of which are mediators of the acute phase response that contributes to pro-inflammatory cytokine production.
Lipid overload can indirectly simulate the pro-inflammation state, as the ER responds to both mitochondrial dysfunction and mTOR hyperactivation and integrates their respective signals to generate ROS (15) and activate the JNK- (29) and NF-κB-mediated (30) inflammation pathways. Thus, the ER, mTOR, and mitochondria can both directly and indirectly induce M1 macrophage activation via production of pro-inflammatory cytokines directly, and through inter-organelle crosstalk as described.
In addition to lipid overload, glucose intolerance and insulin resistance are associated with obesity, which may be partly explained by reduced serum concentrations of adiponectin (31), an important adipokine that promotes glucose uptake, insulin sensitivity, and β-oxidation in peripheral tissues. Adiponectin is an anti-inflammatory and M2 macrophage polarizing molecule, as it attenuates TLR4-mediated NF-κB activation (32) and upregulates IL-10 production in macrophages (33). In addition, adenoviral delivery of adiponectin in wild-type mice increased Arg1 expression in peritoneal macrophages, whereas peritoneal macrophages isolated from adiponectin−/− mice showed increased M1 macrophage polarization (34). Thus, adiponectin deficiency in obesity could alter macrophage polarization fates to favor M1 activation.
Effects of Exercise on Metabolic Stress, Inflammation, and Macrophage Polarization
Attenuation of Metabolic Stress
Exercise training increases adipocyte-specific gene and protein expression of AMPK and PGC-1α (35), which in turn enhances β-oxidation and mitochondrial biogenesis, allowing for greater lipid oxidation per mitochondrion. Improved mitochondrial β-oxidation reduces oxidative stress and mitochondrial dysfunction, thus limiting pro-inflammatory cytokine production and reducing signals for ER stress-mediated inflammation. Improved function and reduced stress in both organelles as direct or indirect consequences of exercise training can, thus, attenuate macrophage M1 polarization or recruitment via reduced stress signaling. Improved exercise-induced lipid oxidation attenuates the need to transport excess fatty acids. For instance, FABP4 concentrations in circulation were reduced with aerobic training in obese women (36). This attenuation in serum FABP4 may be mediated by improved AMPK signaling, since metformin, an AMPK agonist, reduced macrophage Forkhead box O1 (FOXO1)-mediated transcription of FABP4 protein expression (37).
Peroxisome proliferator-activated receptors also respond favorably to exercise training in that protein expression of PPARδ increased by 53% in adipose tissue of exercise-trained rats fed a high-fat diet, compared with sedentary rats on the same diet (38). Similar outcomes in PPARγ were attained with (i) exercise-trained rats, with increased DNA-binding activity in adipocytes (39) and in (ii) exercise-trained humans, with increased gene expression in adipocytes (35). Such outcomes translate to improved adipocyte lipogenesis and oxidation, reducing free fatty acids in the adipose tissue microenvironment for PPARδ/γ-mediated M1 macrophage activation.
Exercise training improves glucose and insulin sensitivity, mediated partly by improved AMPK/insulin receptor substrate (IRS)-1/phosphoinositol-3 kinase (PI3K) signaling. Such exercise-induced improvements are associated with increased gene (40–42) and protein (43) expression of adiponectin and gene expression of adiponectin receptor (40) in adipose tissues of rats and humans.
Reduction of Inflammation and Modulating Phenotypes of Macrophages
Physical inactivity has been associated with several chronic metabolic and inflammatory diseases, such as type 2 diabetes mellitus (T2D) (44–46). Furthermore, a sedentary lifestyle is accompanied by the accumulation of visceral fat, which predisposes adipose tissue to infiltration by pro-inflammatory immune cells, increases adipokine secretion and the development of a low-grade, systemic inflammatory state (47). Low-grade systemic inflammation is associated with the pathology of several diseases, including neurodegenerative diseases and insulin resistance. Chronic moderate exercise, in contrast, has been shown to exert anti-inflammatory effects and, therefore, protects against chronic inflammation-associated diseases (44–46, 48). This protective effect of regular exercise may be mediated through both the reduction of visceral fat mass and the induction of an anti-inflammatory environment with each bout of exercise (48, 49).
Several possible mechanisms have been described regarding the beneficial anti-inflammatory effects of regular physical activity, including: (i) reduction in visceral fat mass (with a subsequent decreased production and release of pro-inflammatory adipokines), (ii) reduction in the expression of TLRs on monocyte and macrophages (50), and (iii) induction of several anti-inflammatory molecules from leukocytes and skeletal muscle (51, 52). In addition, the inhibition of monocyte/macrophage infiltration into adipose tissue and the phenotypic switching of macrophages within adipose tissue (52, 53) have been proposed recently. The last two mechanisms are of great importance, since obesity is accompanied by ATMs infiltration into adipose tissue, and induces a phenotypic switch in ATM polarization from an anti-inflammatory M2 phenotype to a pro-inflammatory M1 phenotype and, hence, contributing to insulin resistance (27, 54–56).
The anti-inflammatory function of exercise might prevent chronic inflammatory diseases through the induction of phenotypic switching from M1 to M2 macrophages, as well as inhibit macrophage infiltration into adipose tissue. There are few studies investigating the role of exercise training on macrophage phenotype switching in adipose tissues. In an earlier study by Kawanishi and colleagues (53), these investigators showed, for the first time, that treadmill running (16 weeks) significantly decreased CD11c (M1 macrophage-specific marker) mRNA expression and increased CD163 (M2 macrophage marker) mRNA expression in adipose tissues of obese mice. In a recent study, these authors showed that exercise training decreased TNF-α mRNA and CD11c levels in the adipose tissues of high-fat-diet obese mice (57).
Similar observations were also reported in other pre-clinical studies (58, 59). First, Oliveira et al. (58) reported that two single bouts of swim exercise of 3 h each and separated by 45 min of rest, induced a M1-to-M2 phenotype switch in WAT and stromal vascular fraction (SVF) of rats on a high-fat diet, as evidenced by the increased protein expression of macrophage galactose-type C-type lectin1 (MGL1), a M2 macrophage marker. By contrast, protein expression of TNF-α and iNOS were downregulated in the SVF. Likewise, chronic treadmill running (up to 12 weeks) resulted in the attenuation of CD11c in the adipose tissue of mice on high-fat diets, although surprisingly, the gene expression of two M2-macrophage markers, Arg1 and CD206, was increased in sedentary mice on the high-fat diet, and decreased in chronically trained mice, also on the high-fat diet (59) The authors suggest that the improvements in inflammatory profiles in these mice may involve an attenuation of both M1 and M2 macrophages in adipose tissue.
In humans, only a single study (60) to date had investigated outcomes involving macrophage polarization after exercise training. In this randomized controlled trial that spanned 12 weeks, overweight (BMI: 25–30 kg/m2, body fat >25%), young men were stratified into one of four experimental groups: (i) endurance training group, (ii) dietary control group, (iii) endurance training and increased diet without weight loss group, or (iv) control group. Protein expression of CD68, a pan-macrophage marker, was not significantly different among the four conditions; however, CD163, a M2 macrophage marker was increased in subcutaneous adipose tissue of both exercise groups, but not in either the dietary control group or the control group.
Given the paucity of studies that investigated exercise and macrophage phenotype switching, it is clear that more research is needed to determine the proximal signaling pathways that guide M1 vs. M2 macrophage modification in adipose tissues by exercise. It is possible that exercise may indirectly regulate macrophage phenotype through the enhancement of blood-derived or contracting muscles-derived M2-type marker production. We and others have clearly shown that exercise strongly induced the expression and release of IL-10, Arginase-1, CD163, and IL-6 from human leukocytes and skeletal muscles (49–51).
The mechanism by which exercise induces macrophages polarization toward an M2 phenotype is likely to be related to the induction of PPARγ and its co-factors (PGC-1α/β). PPARγ and PGC-1a are known for their important roles in the regulation of efficient energy utilization and oxidative phosphorylation, both of which are reduced in obesity and insulin resistance (61, 62). In particular, PPARγ plays an important role in controlling adipose tissue inflammation and insulin resistance through the activation and infiltration of alternatively activated (M2) macrophages (63, 64). A previous study by Odegaard and colleagues (28) showed that PPARγ deficiency in macrophages impairs M2 macrophage activation and predisposes the animals to development of diet-induced obesity, insulin resistance, and glucose intolerance. Further evidence for the role of PPAR-γ in macrophage activation stems from studies by Stienstra et al. (64) who showed a repolarization of adipose tissues macrophages to an M2 phenotype following treatment of mice with PPARγ agonist. The authors speculated that M2 macrophages might play a role in PPARγ-dependent expansion and remodeling of adipose tissue.
The PPARγ hypothesis becomes more convincing, given that participation in exercise programs activates PPARγ and PPARγ-mediated signaling events in adipose tissue and monocytes/macrophages (35, 65–68), and that, exercise-induced activation of M2 macrophages is mediated via PPARγ and its co-factors (PGC-1α/β) (69). The beneficial role of exercise-induced PPARγ in serum lipid profiles (increased HDL-cholesterol and decreased total cholesterol, LDL-cholesterol, and triglycerides) has also been reported previously (67, 70). Thus, exercise-triggered adipocyte- and/or monocyte/macrophage-specific PPARγ activation may constitute an additional rationale for prescribing exercise in obesity and type 2 diabetes. Our working hypothesis of how exercise modulates macrophage polarization is summarized in Figure 1.
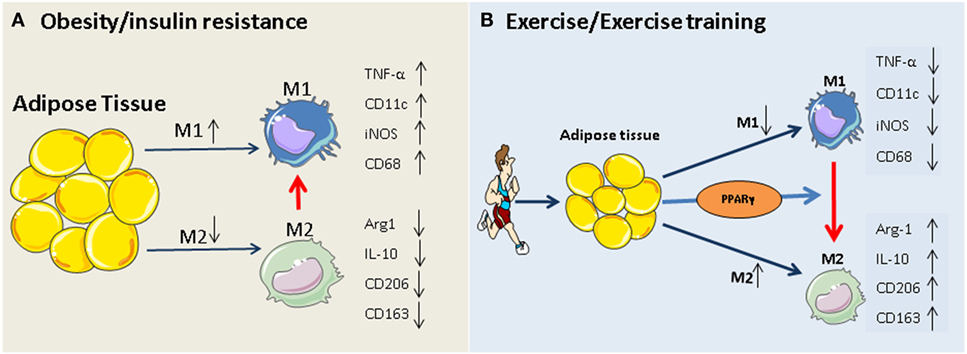
Figure 1. Potential mechanism of exercise-induced anti-inflammatory function in adipose tissues. (A) Obesity and insulin resistance induces a phenotypic switch in adipose tissue macrophage polarization from M2 type to M1 type macrophages. This is accompanied by the induction of M1-specific markers (such as TNF-α and iNOS) and reduction of M2 markers (IL-10, arginase-1). (B) Regular exercise may induce a switch from an M1 to an M2 macrophage phenotype. This, in turn, may contribute to a reduction in the release of pro-inflammatory cytokines (such as IL-6 and TNF) and an increase in the release of anti-inflammatory cytokines (such as IL-10, arginase-1, and adiponectin) from adipose tissue. The exercise-induced phenotypic switch in macrophage type might be mediated by activation of PPARγ.
Summary
The beneficial effects of exercise, such as improved substrate utilization at rest, result in attenuation of metabolic stress in adipocytes, in part by upregulation of PPARγ-associated signaling. Improved metabolic efficiency in adipocytes may reduce the secretion of pro-inflammatory cytokines and attenuate the recruitment of monocytes, or restore the M2-polarization of macrophages (Figure 2). It is still unclear when the M2-to-M1 macrophage phenotype switch occurs during the onset of obesity, given that during both phenotypes are present in the obese adipose tissue, albeit at different time-points and in different proportions. It is also unclear how exercise training can result in a sustained form of one or both phenotypes in adipose tissue.
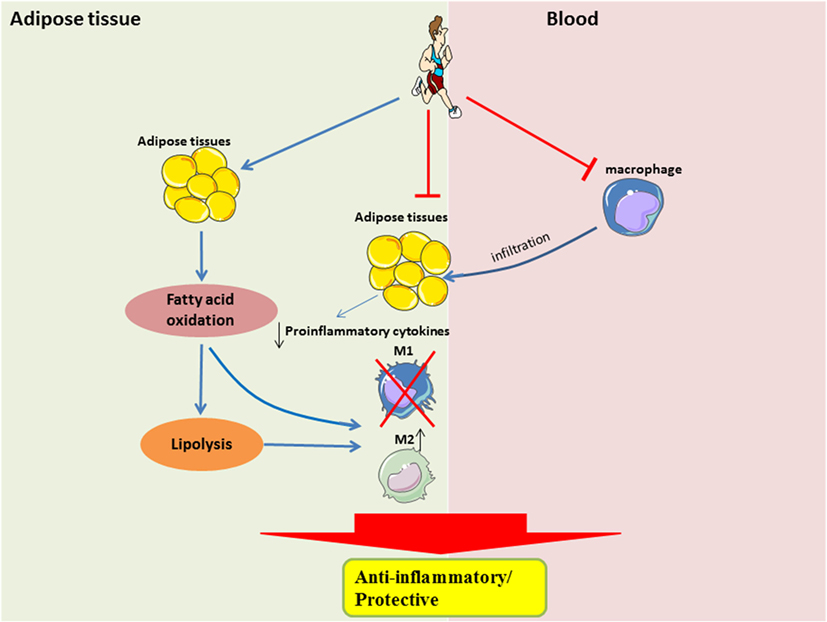
Figure 2. Systemic and local role of exercise training in modulating substrate utilization and macrophage polarization in adipose tissue. In the local adipose tissue microenvironment, regular exercise improves lipolysis and fatty acid oxidation, which is perhaps required for the activation of M2 macrophages. Furthermore, with less metabolic stress, there would be a downregulation of pro-inflammatory signaling. A shift in pro-inflammatory signaling would also result in a reduced recruitment of circulating monocytes, which may also attenuate the polarization of M1 macrophages.
What remains unknown, in addition, is how other forms of exercise, namely resistance exercise, or non-load bearing exercise, such as swimming, may mediate the signaling pathways differently from that of the more typical endurance exercise. More studies are needed to investigate the underlying mechanisms that drive macrophage polarization in response to exercise and also delineate the M1-to-M2 phenotype shift after exercise training based on the consensus guidelines described in the Milan congress (14).
Author Contributions
JG conceived, drafted, and prepared the manuscript. AA prepared the figures, and drafted part of the manuscript. KG critically appraised the manuscript and drafted part of the manuscript. All authors give permission for this manuscript to be published.
Conflict of Interest Statement
The authors declare that the research was conducted in the absence of any commercial or financial relationships that could be construed as a potential conflict of interest.
Acknowledgments
We gratefully acknowledge Dr. Eric Chun Seng Chan for insightful feedback during the preparation of this manuscript.
Funding
This research did not received any specific grant from any funding agency in the public, commercial, or not-for-profit sectors.
References
1. Lönnqvist F, Arner P, Nordfors L, Schalling M. Overexpression of the obese (ob) gene in adipose tissue of human obese subjects. Nat Med (1995) 19:950–3. doi: 10.1038/nm0995-950
2. Klein S, Coppack SW, Mohamed-Ali V, Landt M. Adipose tissue leptin production and plasma leptin kinetics in humans. Diabetes (1996) 45:984–7. doi:10.2337/diabetes.45.7.984
3. Hotamisligil GS, Shargill NS, Spiegelman BM. Adipose expression of tumor necrosis factor-alpha: direct role in obesity-related insulin resistance. Science (1993) 259:87–91. doi:10.1126/science.7678183
4. Ahima RS, Flier JS. Adipose tissue as an endocrine organ. Trends Endocrinol Metab (2000) 11:327–32. doi:10.1016/S1043-2760(00)00301-5
5. Trayhurn P, Wood IS. Adipokines: inflammation and the pleiotropic role of white adipose tissue. Br J Nutr (2004) 92:347–55. doi:10.1079/BJN20041213
6. Weisberg SP, McCann D, Desai M, Rosenbaum M, Leibel RL, Ferrante AW Jr. Obesity is associated with macrophage accumulation in adipose tissue. J Clin Invest (2003) 112:1796–808. doi:10.1172/JCI200319246
7. Kanda H, Tateya S, Tamori Y, Kotani K, Hiasa K, Kitazawa R, et al. MCP-1 contributes to macrophage infiltration into adipose tissue, insulin resistance, and hepatic steatosis in obesity. J Clin Invest (2006) 116:1494–505. doi:10.1172/JCI26498
8. Lumeng CN, Bodzin JL, Saltiel AR. Obesity induces a phenotypic switch in adipose tissue macrophage polarization. J Clin Invest (2007) 117:175–84. doi:10.1172/JCI29881
9. Cinti S, Mitchell G, Barbatelli G, Murano I, Ceresi E, Faloia E, et al. Adipocyte death defines macrophage localization and function in adipose tissue of obese mice and humans. J Lipid Res (2005) 46:2347–55. doi:10.1194/jlr.M500294-JLR200
10. Phipps KD, Gebremeskel S, Gillis J, Hong P, Johnston B, Bezuhly M. Alternatively activated M2 macrophages improve autologous fat graft survival in a mouse model through induction of angiogenesis. Plast Reconstr Surg (2015) 135:140–9. doi:10.1097/PRS.0000000000000793
11. Lumeng CN, DelProposto JB, Westcott DJ, Saltiel AR. Phenotypic switching of adipose tissue macrophages with obesity is generated by spatiotemporal differences in macrophage subtypes. Diabetes (2008) 57:3239–46. doi:10.2337/db08-0872
12. Shaul ME, Bennett G, Strissel KJ, Greenberg AS, Obin MS. Dynamic, M2-like remodeling phenotypes of CD11c+ adipose tissue macrophages during high-fat diet-induced obesity in mice. Diabetes (2010) 59:1171–81. doi:10.2337/db09-1402
13. Kratz M, Coats BR, Hisert KB, Hagman D, Mutskov V, Peris E, et al. Metabolic dysfunction drives a mechanistically distinct proinflammatory phenotype in adipose tissue macrophages. Cell Metab (2014) 20:614–5. doi:10.1016/j.cmet.2014.08.010
14. Murray PJ, Allen JE, Biswas SK, Fisher EA, Gilroy DW, Goerdt S, et al. Macrophage activation and polarization: nomenclature and experimental guidelines. Immunity (2014) 41:14–20. doi:10.1016/j.immuni.2014.06.008
15. Hotamisligil GS, Erbay E. Nutrient sensing and inflammation in metabolic diseases. Nat Rev Immunol (2008) 8:923–34. doi:10.1038/nri2449
16. Odegaard JI, Chawla A. Mechanism of macrophage activation in obesity-induced insulin resistance. Nat Clin Pract Endocrinol Metabol (2008) 4:619–26. doi:10.1038/ncpendmet0976
17. Tilg H, Moschen AR. Adipocytokines: mediators linking adipose tissue, inflammation and immunity. Nat Rev Immunol (2006) 6:772–83. doi:10.1038/nri1937
18. Shi H, Kokoeva MV, Inouye K, Tzameli I, Yin H, Flier JS. TLR4 links innate immunity and fat-induced insulin resistance. J Clin Invest (2006) 116:3015–23. doi:10.1172/JCI28898
19. Ajuwon KM, Spurlock ME. Palmitate activates the NF-kappaB transcription factor and induces IL-6 and TNFalpha expression in 3T3-L1 adipocytes. J Nutr (2005) 135:1841–6.
20. Arkan MC, Hevener AL, Greten FR, Maeda S, Li ZW, Long JM, et al. IKK-beta links inflammation to obesity-induced insulin resistance. Nat Med (2005) 11:191–8. doi:10.1038/nm1185
21. Medzhitov R. Origin and physiological roles of inflammation. Nature (2008) 454:428–35. doi:10.1038/nature07201
22. Makowski L, Britttingham KC, Reynolds JM, Suttles J, Hotamisligil GS. The fatty acid-binding protein, aP2, co-ordinates macrophage cholesterol trafficking and inflammatory activity. Macrophage expression of aP2 impacts peroxisome proliferator-activated receptor gamma and IKappaβ kinase activities. J Biol Chem (2005) 280:12888–95. doi:10.1074/jbc.M413788200
23. Furuhashi M, Fucho R, Gorgun CZ, Tuncman G, Cao H, Hotamisligil GS. Adipocyte/macrophage fatty acid-binding proteins contribute to metabolic deterioration through actions in both macrophages and adipocytes in mice. J Clin Invest (2008) 118:2640–50. doi:10.1172/JCI34750
24. Evans RM, Barish GD, Wang YX. PPARs and the complex journey to obesity. Nat Med (2004) 10:355–61. doi:10.1038/nm1025
25. López-Soriano J, Chiellini C, Maffei M, Grimaldi PA, Argilés JM. Roles of skeletal muscle and peroxisome proliferator-activated receptors in the development and treatment of obesity. Endocr Rev (2006) 27:318–29. doi:10.1210/er.2005-0012
26. Wang YX, Lee CH, Tiep S, Yu RT, Ham J, Kang H, et al. Peroxisome proliferator-activated receptor δ activates fat metabolism to prevent obesity. Cell (2003) 113:159–70. doi:10.1016/S0092-8674(03)00269-1
27. Kang K, Reilly SM, Karabacak V, Gangl MR, Fitzgerald K, Hatano B, et al. Adipocyte-derived Th2 cytokines and myeloid PPARδ regulate macrophage polarization and insulin sensitivity. Cell Metab (2008) 7:485–95. doi:10.1016/j.cmet.2008.04.002
28. Odegaard JI, Ricardo-Gonzalez RR, Goforth MH, Morel CR, Subramanian V, Mukundan L, et al. Macrophage-specific PPARγ controls alternative activation and improves insulin resistance. Nature (2007) 447:1116–20. doi:10.1038/nature05894
29. Urano F, Wang X, Bertolotti A, Zhang Y, Chung P, Harding HP, et al. Coupling of stress in the ER to activation of JNK protein kinases by transmembrane protein kinase IRE1. Science (2000) 287:664–6. doi:10.1126/science.287.5453.664
30. Deng J, Lu PD, Zhang Y, Scheuner D, Kaufman RJ, Sonenberg N, et al. Translational repression mediates activation of nuclear factor κB by phosphorylated translation initiation factor 2. Mol Cell Biol (2004) 24:10161–8. doi:10.1128/MCB.24.23.10161-10168.2004
31. Arita Y, Kihara S, Ouchi N, Takahashi M, Maeda K, Miyagawa J, et al. Paradoxical decrease of an adipose-specific protein, adiponectin in obesity. Biochem Biophys Res Commun (1999) 257:79–83. doi:10.1006/bbrc.1999.0255
32. Kadowaki T, Yamauchi T, Kubota N, Hara K, Ueki K, Tobe K. Adiponectin and adiponectin receptors in insulin resistance, diabetes and the metabolic syndrome. J Clin Invest (2006) 116:1784–92. doi:10.1172/JCI29126
33. Kumada M, Kihara S, Ouchi N, Kobayashi H, Okamoto Y, Ohashi K, et al. Adiponectin specifically increased tissue inhibitor of metalloproteinase-1 through interleukin-10 expression in human macrophages. Circulation (2004) 109:2046–9. doi:10.1161/01.CIR.0000127953.98131.ED
34. Ohashi K, Parker JL, Ouchi N, Higuchi A, Vita JA, Gokce N, et al. Adiponectin promotes macrophage polarization toward an anti-inflammatory phenotype. J Biol Chem (2010) 285:6153–60. doi:10.1074/jbc.M109.088708
35. Ruschke K, Fishbein L, Dietrich A, Klöting N, Tönjes A, Oberbach A, et al. Gene expression of PPARγ and PGC-1α in human omental and subcutaneous adipose tissues is related to insulin resistance markers and mediates beneficial effects of physical training. Eur J Endocrinol (2010) 162:515–23. doi:10.1530/EJE-09-0767
36. Choi KM, Kim TN, Yoo HJ, Lee KW, Cho GJ, Hwang TG, et al. Effect of exercise training on A-FABP, lipocalin-2 and RBP4 levels in obese women. Clin Endocrinol (2009) 70:569–74. doi:10.1111/j.1365-2265.2008.03374.x
37. Song J, Ren P, Zhang L, Wang XL, Chen L, Shen YH. Metformin reduces lipid accumulation in macrophages by inhibiting FOXO1-mediated transcription of fatty-acid binding protein 4. Biochem Biophys Res Commun (2010) 393:89–94. doi:10.1016/j.bbrc.2010.01.086
38. Yan ZC, Liu DY, Zhang LL, Shen CY, Ma QL, Cao TB, et al. Exercise reduces adipose tissue via cannabinoid receptor type 1 which is regulated by peroxisome proliferator-activated receptor-delta. Biochem Biophys Res Commun (2007) 354:427–33. doi:10.1016/j.bbrc.2006.12.213
39. Petridou A, Tsalouhidou S, Tsalis G, Schulz T, Michna H, Mougios V. Long-term exercise increases the DNA binding activity of peroxisome proliferator-activated receptor y in rat adipose tissue. Metabolism (2007) 56:1029–36. doi:10.1016/j.metabol.2007.03.011
40. Christiansen T, Paulsen SK, Bruun JM, Ploug T, Pedersen SB, Richelsen B. Diet-induced weight loss and exercise alone and in combination enhance the expression of adiponectin receptors in adipose tissue and skeletal muscle, but only diet-induced weight loss enhanced circulating adiponectin. J Clin Endocrinol Metab (2010) 95:911–9. doi:10.1210/jc.2008-2505
41. Miyazaki S, Izawa T, Ogasawara J, Sakurai T, Nomura S, Kizaki T, et al. Effect of exercise training on adipocyte-size-dependent expression of leptin and adiponectin. Life Sci (2010) 86:691–8. doi:10.1016/j.lfs.2010.03.004
42. Zeng Q, Fu L, Takekoshi K, Kawakami Y, Isobe K. Effects of short term exercise on adiponectin and adiponectin receptor levels in rats. J Atheroscler Thromb (2007) 14:261–5. doi:10.5551/jat.E498
43. Yamashita AS, Lira FS, Rosa JC, Paulino EC, Brum PC, Negrão CE, et al. Depot-specific modulation of adipokine levels in rat adipose tissue by diet-induced obesity: the effect of aerobic training and energy restriction. Cytokine (2010) 52:168–74. doi:10.1016/j.cyto.2010.07.006
44. Pedersen BK, Saltin B. Evidence for prescribing exercise as therapy in chronic disease. Scand J Med Sci Sports (2006) 16:5–65. doi:10.1111/j.1600-0838.2006.00520.x
45. Walsh NP, Gleeson M, Shephard RJ, Gleeson M, Woods JA, Bishop NC, et al. Position statement. Part one: immune function and exercise. Exerc Immunol Rev (2011) 17:1–65.
46. Warren TY, Barry V, Hooker SP, Sui X, Church TS, Blair SN. Sedentary behaviors increase risk of cardiovascular disease mortality in men. Med Sci Sports Exerc (2010) 42:879–85. doi:10.1249/MSS.0b013e3181c3aa7e
47. Ouchi N, Parker JL, Lugus JJ, Walsk K. Adipokines in inflammation and metabolic disease. Nat Rev Immunol (2011) 11:85–97. doi:10.1038/nri2921
48. Petersen AM, Pedersen BK. The antiinflammatory effect of exercise. J Appl Physiol (2005) 98:1154–62. doi:10.1152/japplphysiol.00164.2004
49. Mathur N, Pedersen BK. Exercise as a mean to control low-grade inflammation. Mediators Inflamm (2008) 2008:109502. doi:10.1155/2008/109502
50. Flynn MG, McFarlin BK. Toll-like receptor 4: link to the anti-inflammatory effects of exercise? Exerc Sport Sci Rev (2006) 34:176–81. doi:10.1249/01.jes.0000240027.22749.14
51. Abbasi A, Hauth M, Walter M, Hudemann J, Wank V, Niess AM, et al. Exhaustive exercise modifies different gene expression profiles and pathways in LPS-stimulated and un-stimulated whole blood cultures. Brain Behav Immun (2014) 39:130–41. doi:10.1016/j.bbi.2013.10.023
52. Pedersen BK, Febbraio MA. Muscle as an endocrine organ: focus on muscle-derived interleukin-6. Physiol Rev (2008) 88:1379–406. doi:10.1152/physrev.90100.2007
53. Kawanishi N, Yano H, Yokogawa Y, Suzuki K. Exercise training inhibits inflammation in adipose tissue via both suppression of macrophage infiltration and acceleration of phenotypic switching from M1 to M2 macrophages in high-fat diet-induced obese mice. Exerc Immunol Rev (2010) 16:105–18.
54. Aron-Wisnewsky J, Tordjman J, Poitou C, Darakhshan F, Hugol D, Basdevant A, et al. Human adipose tissue macrophages: m1 and m2 cell surface markers in subcutaneous and omental depots and after weight loss. J Clin Endocrinol Metab (2009) 94:4619–23. doi:10.1210/jc.2009-0925
55. Acosta JR, Douagi I, Andersson DP, Bäckdahl J, Rydén M, Arner P, et al. Increased fat cell size: a major phenotype of subcutaneous white adipose tissue in non-obese individuals with type 2 diabetes. Diabetologia (2016) 59:560–70. doi:10.1007/s00125-015-3810-6
56. Behan JW, Ehsanipour EA, Sheng X, Pramanik R, Wang X, Hsieh YT, et al. Activation of adipose tissue macrophages in obese mice does not require lymphocytes. Obesity (2013) 7:1380–8. doi:10.1002/oby.20159
57. Kawanishi N, Mizokami T, Yano H, Suzuki K. Exercise attenuates M1 macrophages and CD8+ T cells in the adipose tissue of obese mice. Med Sci Sports Exerc (2013) 45:1684–93. doi:10.1249/MSS.0b013e31828ff9c6
58. Oliveira AG, Araujo TG, Carvalho BM, Guadagnini D, Rocha GZ, Bagarolli RA, et al. Acute exercise induces a phenotypic switch in adipose tissue macrophage polarization in diet-induced obese rats. Obesity (2013) 21:2545–56. doi:10.1002/oby.20402
59. Linden MA, Pincu Y, Martin SA, Woods JA, Baynard T. Moderate exercise training provides modest protection against adipose tissue inflammatory gene expression in response to high-fat feeding. Physiol Rep (2014) 2:e12071. doi:10.14814/phy2.12071
60. Auerbach P, Nordby P, Bendtsen LQ, Mehlsen JL, Basnet SK, Vestergaard H, et al. Differential effects of endurance training and weight loss on plasma adiponectin multimers and adipose tissue macrophages in younger, moderately overweight men. Am J Physiol Regul Integr Comp Physiol (2013) 305:R490–8. doi:10.1152/ajpregu.00575.2012
61. Hammarstedt A, Jansson PA, Wesslau C, Yang X, Smith U. Reduced expression of PGC-1 and insulin-signaling molecules in adipose tissue is associated with insulin resistance. Biochem Biophys Res Commun (2003) 301:578–82. doi:10.1016/S0006-291X(03)00014-7
62. Semple RK, Crowley VC, Sewter CP, Laudes M, Christodoulides C, Considine RV, et al. Expression of the thermogenic nuclear hormone receptor coactivator PGC-1alpha is reduced in the adipose tissue of morbidly obese subjects. Int J Obes Relat Metab Disord (2004) 28:176–9. doi:10.1038/sj.ijo.0802482
63. Bouhlel MA, Derudas B, Rigamonti E, Dievart R, Brozek J, Haulon S, et al. PPAR activation primes human monocytes into alternative M2 macrophages with anti-inflammatory properties. Cell Metab (2007) 6:137–43. doi:10.1016/j.cmet.2007.06.010
64. Stienstra R, Duval C, Keshtkar S, van der Laak J, Kersten S, Muller M. PPARγ activation promotes infiltration of alternatively activated macrophages into adipose tissue. J Biol Chem (2008) 283:22620–7. doi:10.1074/jbc.M710314200
65. Stanford KI, Middelbeek RJ, Goodyear LJ. Exercise effects on white adipose tissue: beiging and metabolic adaptations. Diabetes (2015) 64:2361–8. doi:10.2337/db15-0227
66. Tanaka G, Kato H, Izawa T. Endurance exercise training induces fat depot-specific differences in basal autophagic activity. Biochem Biophys Res Commun (2015) 466:512–7. doi:10.1016/j.bbrc.2015.09.061
67. Butcher L, Thomas AW, Backx K, Roberts A, Webb R, Morris K. Low-intensity exercise exerts beneficial effects on plasma lipids via PPAR. Med Sci Sports Exerc (2008) 40:1–7. doi:10.1249/MSS.0b013e31816c091d
68. Liu WX, Wang T, Zhou F, Wang Y, Xing JW, Zhang S, et al. Voluntary exercise prevents colonic inflammation in high-fat diet-induced obese mice by up-regulating PPAR-γ activity. Biochem Biophys Res Commun (2015) 459:475–80. doi:10.1016/j.bbrc.2015.02.047
69. Yakeu G, Butcher L, Isa S, Webb R, Roberts AW, Thomas AW, et al. Low-intensity exercise enhances expression of markers of alternative activation in circulating leukocytes: roles of PPARγ and Th2 cytokines. Atherosclerosis (2010) 212:668–73. doi:10.1016/j.atherosclerosis.2010.07.002
Keywords: obesity, exercise, adipose tissue, macrophages, inflammation, polarization
Citation: Goh J, Goh KP and Abbasi A (2016) Exercise and Adipose Tissue Macrophages: New Frontiers in Obesity Research? Front. Endocrinol. 7:65. doi: 10.3389/fendo.2016.00065
Received: 21 April 2016; Accepted: 01 June 2016;
Published: 14 June 2016
Edited by:
Timo Dirk Müller, Institute for Diabetes and Obesity, GermanyReviewed by:
Geanncarlo Lugo-Villarino, Institut de Pharmacologie et de Biologie Structurale, FranceCarol Geralyn Chitko-McKown, U.S. Meat Animal Research Center, USA
Copyright: © 2016 Goh, Goh and Abbasi. This is an open-access article distributed under the terms of the Creative Commons Attribution License (CC BY). The use, distribution or reproduction in other forums is permitted, provided the original author(s) or licensor are credited and that the original publication in this journal is cited, in accordance with accepted academic practice. No use, distribution or reproduction is permitted which does not comply with these terms.
*Correspondence: Jorming Goh, Z2pvcm1pbmdAZHNvLm9yZy5zZw==