- 1Institut de Pharmacologie Moléculaire et Cellulaire CNRS UMR 7275, Valbonne, France
- 2Laboratoire International Associé (LIA) CNRS NEOGENEX, Valbonne, France
- 3Université de Nice, Valbonne, France
The trophic peptide hormone adrenocorticotropic (ACTH) stimulates steroid hormone biosynthesis evoking both a rapid, acute response and a long-term, chronic response, via the activation of cAMP/protein kinase A (PKA) signaling. The acute response is initiated by the mobilization of cholesterol from lipid stores and its delivery to the inner mitochondrial membrane, a process that is mediated by the steroidogenic acute regulatory protein. The chronic response results in the increased coordinated transcription of genes encoding steroidogenic enzymes. ACTH binding to its cognate receptor, melanocortin 2 receptor (MC2R), stimulates adenylyl cyclase, thus inducing cAMP production, PKA activation, and phosphorylation of specific nuclear factors, which bind to target promoters and facilitate coactivator protein recruitment to direct steroidogenic gene transcription. This review provides a general view of the transcriptional control exerted by the ACTH/cAMP system on the expression of genes encoding for steroidogenic enzymes in the adrenal cortex. Special emphasis will be given to the transcription factors required to mediate ACTH-dependent transcription of steroidogenic genes.
Introduction
The adrenal gland is a key component of the hypothalamus–pituitary–adrenal (HPA) axis, thus playing a crucial role in the adaptation of organism to stressors. The adrenocorticotropic hormone (ACTH) belongs to this regulatory circuitry, being one of the most potent physiological modulators of adrenal cortex steroidogenesis and trophicity (1, 2). It exerts its role through the binding to the G protein-coupled receptor (GPCR) melanocortin 2 receptor (MC2R), which activates adenylyl cyclase cascade leading to cAMP production and subsequent activation of cAMP-dependent protein kinase A (PKA). PKA is the main kinase responsible for the phosphorylation of specific transcription factors, which in turn regulate free cholesterol availability and activate steroidogenic enzyme expression (3, 4). Among those transcription factors, steroidogenic factor 1 (SF-1), cAMP response element-binding protein (CREB), CRE modulator (CREM), CCAAT/enhancer-binding proteins (C/EBPs), and activator protein 1 (AP-1) have been extensively described for their implication in regulating the expression of the genes encoding for steroidogenic acute regulatory (StAR) protein and steroidogenic enzymes (5–8). Any perturbation of this ACTH/cAMP/PKA-dependent cascade may cause alteration in adrenocortical cell proliferation and dysregulation of steroidogenesis as occur in various human diseases. The aim of the present review is to provide an overview of the ACTH/cAMP-dependent transcriptional regulation of the steroidogenic process in the adrenal cortex.
Adrenal Steroidogenesis
Steroid hormones are implicated in the regulation of a plethora of developmental and physiological processes from fetal life to adulthood. Cholesterol is the precursor of all those hormones that hence share a closely related structure based on the cyclopentanophenanthrene 4-ring hydrocarbon nucleus. Cholesterol can be synthesized de novo from acetate in the adrenal (9). However, the main adrenal source of cholesterol is plasma low-density lipoproteins (LDLs) provided by dietary cholesterol (10). The sterol response element-binding proteins (SREBPs) are a family of transcription factors implicated in the regulation of genes participating in the biosynthesis of cholesterol and fatty acids (11). They are the main regulators of intracellular cholesterol metabolism. ACTH promotes the activation of 3-hydroxy-3-methylglutaryl coenzyme A reductase, the rate-limiting enzyme in cholesterol synthesis. It also stimulates the uptake of LDL cholesterol esters, which, once taken up by receptor-mediated endocytosis can be converted to free cholesterol for steroid hormone biosynthesis (12) or directly stored. Moreover, ACTH stimulates hormone-sensitive lipase (HSL) and inhibits acyl-coenzyme A (CoA):cholesterol acyltransferase (ACAT), thereby increasing the free cholesterol pool available for steroid hormone biosynthesis.
The initial step of steroidogenesis occurs in the mitochondria. Whereas the movement of cholesterol from the outer mitochondrial membrane (OMM) to the inner mitochondrial membrane (IMM) is known to be promoted by StAR (see below), the molecular mechanism underlying cholesterol transport and loading into the OMM is still under investigation (13). The enzymes that participate in steroid biosynthesis are either cytochrome P450s (CYPs) or hydroxysteroid dehydrogenases (HSDs).
Cytochrome P450s are a group of oxidative enzymes. In the human genome, genes for 57 CYPs enzymes have been described. Seven of them are called “type 1” enzymes. They are targeted to mitochondria and receive electrons from NADPH via a flavoprotein (ferrodoxin reductase) and a small iron-sulfur protein (ferredoxin). “Type 2” enzymes are localized in the endoplasmic reticulum and received electrons form NADPH via a single 2-flavin protein called P450 oxidoreductase (14). Six P450 enzymes participate in steroidogenesis. The mitochondrial P450 side chain cleavage (SCC, encoded by the CYP11A1 gene) catalyzes breakage of the bond between positions 20 and 22 in the cholesterol side chain (20, 22 desmolase). P450c11β (11β-hydroxylase, encoded by the CYP11B1 gene) and P450c11AS (aldosterone synthase, encoded by the CYP11B2 gene) catalyze 11β-hydroxylase, 18-hydroxylase, and 18-methyl oxidase activities. At the level of the endoplasmic reticulum, we distinguish: P450c17 (encoded by the CYP17A1 gene) that catalyzes both 17α-hydroxylase and 17,20-lyase activities; P450c21 (encoded by the CYP21A1 gene) that catalyzes 21-hydroxylation in the synthesis of both glucocorticoids and mineralocorticoids; and P450-Aro (encoded by the CYP19A1 gene) that catalyzes the aromatization of androgens to estrogens.
The HSDs use nicotinamide adenine dinucleotides or their phosphate forms (NADH/NAD+ or NADPH/NADP+) as cofactors to either reduce or oxidize a steroid through a hydride transfer mechanism (15). Differently from steroidogenic reactions catalyzed by P450 enzymes, which are mediated by a single form of P450, each reaction catalyzed by HSDs involves at least two, often different isozymes. On the basis of their structures, those enzymes are divided into: short-chain dehydrogenase/reductase (SDR) family, which include 11β-HSDs 1, 2, and 17β-HSDs 1, 2, and 3 and aldo-keto reductase (AKR) family, which include 17β-HSD5 (15, 16). Under a physiological point of view, it is preferable to classify the HSDs as dehydrogenases or reductases. The former use NAD+ as cofactors to oxidize hydroxysteroids to ketosteroids, whereas the latter mainly use NADPH to reduce ketosteroids to hydroxysteroids. Those enzymes act in vitro typically bidirectionally on the basis of the pH and cofactor concentration, while in intact cells they work mainly in one direction, with the direction established by the cofactors available (15, 16).
The pattern of steroid hormones secreted by each adrenal zone is determined by the enzymes expressed in each zone (17). Specifically, adrenal zona glomerulosa expresses angiotensin II receptors and P450c11AS, whereas it does not express P450c17. Indeed, zona glomerulosa produces aldosterone under the regulation of the renin–angiotensin system. In contrast, at the levels of zona fasciculata, angiotensin II receptors, and P450c11AS are not detected, whereas the ACTH receptor MC2R and P450c11β are expressed (18). The zona fasciculata also expresses P450c17, which catalyzes 17α-hydroxylation, exhibiting only little 17,20-lyase activity. Indeed, zona fasciculata secretes the two glucocorticoids, cortisol and corticosterone, under the influence of ACTH, but very little dehydroepiandrosterone (DHEA). Interestingly, patients displaying mutations in P450c17 are not able to produce cortisol, but show increased corticosterone production (19), like in rodents, which normally do not express P450c17 in their adrenals. Regarding the zona reticularis, it also expresses MC2R and large amounts of P450c17 and cytochrome b5, thus displaying 17,20-lyase activity with subsequent DHEA production, the most part of which is sulfated to DHEAS by SULT2A1. In general, small amounts of DHEA are converted to androstenedione, and very little amounts of androstenedione are converted to testosterone, likely through AKR1C3/17βHSD5. In contrast, zona reticularis expresses very little P450c21 and P450c11β (thus producing only a minimal amount of cortisol) and relatively little 3βHSD2.
Acute Response to ACTH: The Steroidogenic Acute Regulatory Protein
Steroidogenic cells are able to store very little amounts of steroids, which imply a rapid synthesis of new steroids in response to a sudden demand. ACTH exerts its role in promoting steroidogenic cell growth and stimulating steroidogenesis at three distinct levels. We first distinguish a long-term exposure to ACTH. It takes weeks or months to stimulate adrenal growth and it is mediated by ACTH-dependent production of cAMP, which in turn triggers IGF-II (20), fibroblast growth factor (FGF) (21), and epidermal growth factor (EGF) (22) synthesis. The concerted action of those growth factors is to promote adrenal cellular hypertrophy and hyperplasia. Second, ACTH can act over days through cAMP to stimulate the transcription of genes, which encode for different steroidogenic enzymes (see below). Third, ACTH can mediate an acute response, which occurs within minutes and is inhibited by protein synthesis inhibitors (like puromycin or cycloheximide). This ACTH-mediated acute response is accompanied by a rapid stimulation of the StAR gene transcription in steroidogenic cells of the adrenal cortex, testis, and ovary (5, 23) and by the phosphorylation of Ser195 in the existent pool of StAR (24). Those events promote cholesterol flow from the OMM to the IMM, where cholesterol is converted to pregnenolone in the first and rate limiting step of steroid hormone biosynthesis. The first to show that ACTH acute steroidogenic response involved the rapid synthesis of a 37-kDa phosphoprotein were Orme-Johnson and coworkers (25, 26). Just a few years later, Stocco and colleagues cloned this protein and they gave it the name of “StAR” (27). StAR is an acutely regulated, cycloheximide-sensitive protein exhibiting a mitochondrial leader sequence by which it is directed to the mitochondria. Once inside the mitochondria, StAR is cleaved to a 30-kDa protein. It has been shown that the overexpression of a mouse StAR in the mouse Leydig MA-10 cells increased their basal steroidogenesis (27). Moreover, when expression vectors for both StAR and P450scc enzyme are cotransfected in non-steroidogenic COS-1 cells, the synthesis of pregnenolone is augmented respect to that obtained with P450scc alone (28). The pivotal role of StAR in the regulation of steroidogenesis was strengthened after the identification of mutations causing premature stop codons in the StAR gene of patients affected by the most common form of congenital lipoid adrenal hyperplasia (CAH) (28, 29), a rare disorder of steroid biosynthesis. In this disorder, glucocorticoid, mineralocorticoid, and sex steroids biosynthesis is impaired, which may lead to adrenal failure, severe salt wasting crisis and hyperpigmentation in phenotypical female infants irrespective of genetic sex (29). Moreover, it has been shown that in mice the targeted disruption of the Star gene causes defects in steroidogenesis, with consequent male pseudohermaphroditism and lethality within 1 week after birth (30, 31).
Several studies have been carried out to understand the mechanism of action of StAR (32), which still remains to be fully elucidated. It was hypothesized that the “mature” 30-kDa intramitochondrial form of StAR was the biologically active portion of the protein, due to its longer half-life respect to the short one of the 37 kDa precursor. However, when the two StAR forms are expressed in the cytoplasm or added to mitochondria in vitro, they are equally active (33). Moreover, while constitutively active when it is localized on the OMM, StAR results to be inactive at the level of the mitochondrial intramembranous space or the matrix (34). That evidence suggested that StAR exerts its action on the OMM, its steroidogenesis-promoting function tightly depending on the residency time on the OMM itself (33, 34). This implies that StAR activity is linked to its localization rather than to its cleavage to the “mature” form. When StAR interacts with the OMM, it undergoes to conformational changes (35, 36) that allow StAR to accept and discharge cholesterol molecules. Interestingly, steroidogenesis-promoting and cholesterol-transfer activities of StAR are distinct. Indeed, StAR-mediated transfer of cholesterol between synthetic membranes in vitro (37) is maintained also by the inactive R182L mutant, which impairs steroidogenesis, causing lipoid CAH (38). Finally, StAR activity on the OMM requires the translocator protein TSPO, also called peripheral benzodiazepine receptor (PBR) (39, 40), which has been identified as a key player in the flow of cholesterol into mitochondria to permit the initiation of steroid hormone synthesis. Moreover, it has been demonstrated that phosphorylated StAR interacts with voltage-dependent anion channel 1 (VDAC1) on the OMM, which in turn promotes processing of the 37-kDa phospho-StAR to the 32-kDa intermediate (41). In the absence of VDAC1, phospho-StAR undergoes degradation by cysteine proteases prior to mitochondrial import and subsequent cleavage to the 30-kDa protein. StAR phosphorylation by PKA requires phosphate carrier protein on the OMM, which seems to interact with StAR before it interacts with VDAC1 (41). Importantly, although StAR is necessary for the ACTH-mediated acute steroidogenic response, steroidogenesis still occurs in the absence of StAR (around 14% of StAR-induced rate) (29, 42). This may account for the steroidogenic capacity of tissues lacking StAR, like placenta and brain.
Chronic Response to ACTH
The transcription of steroidogenic genes depends on the slower, chronic response to ACTH in the adrenal cortex. Indeed, ACTH interaction with specific membrane receptors leads to the activation of coupled G proteins, with subsequent stimulation of membrane-associated adenylyl cyclase catalyzing cAMP formation. cAMP-activated PKA hence phosphorylates multiple transcription factors, whose concerted action and interaction with different cis-regulatory elements direct StAR and steroidogenic gene expression. Furthermore, after transcription factor binding to gene promoters, posttranslational modifications, like phosphorylation/dephosphorylation and coactivator proteins binding, are required to activate gene expression. In the following section, the main transcription factors that direct the transcription of steroidogenic genes in response to ACTH will be described (Table 1; Figure 1).
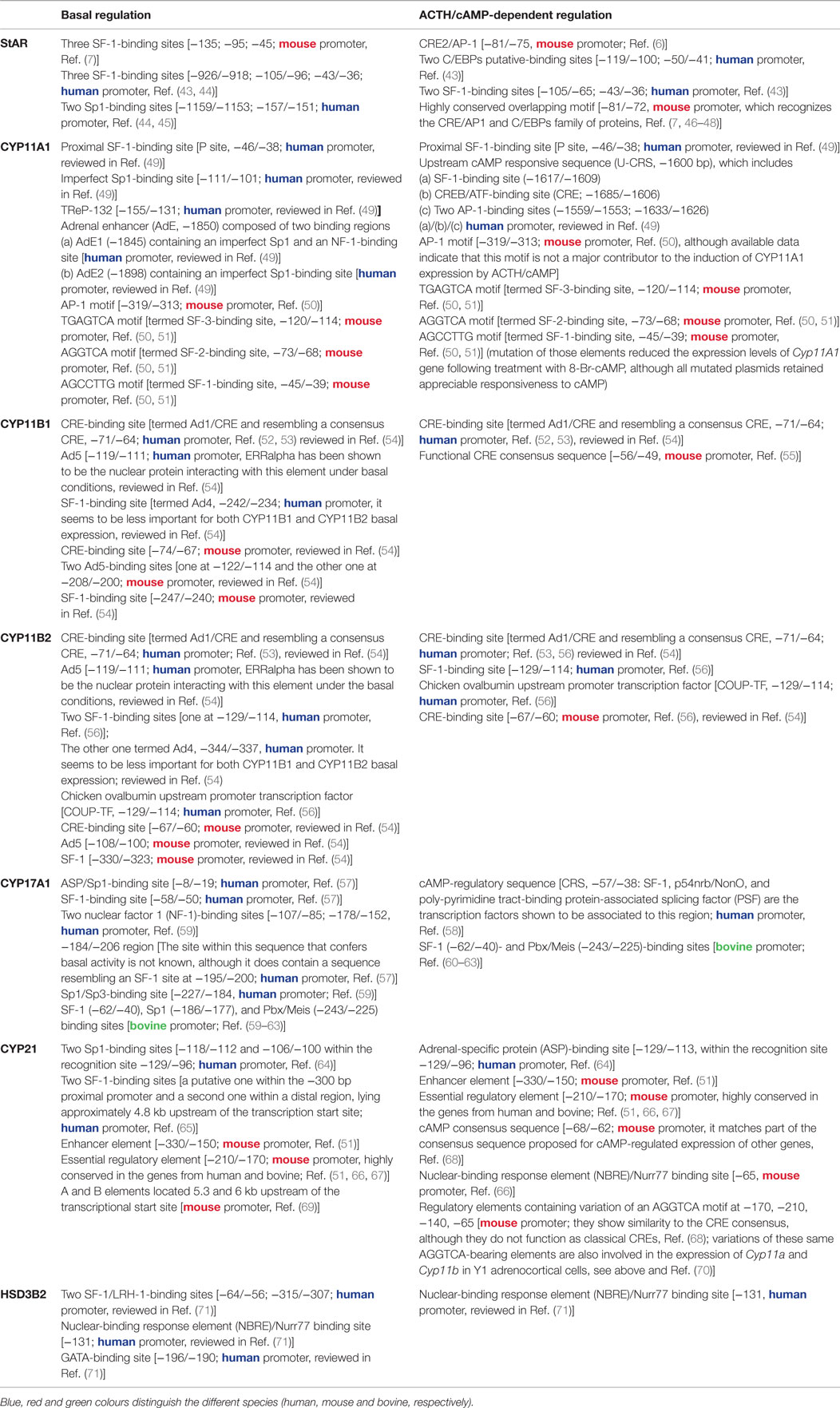
Table 1. Promoter elements implicated in basal and ACTH/cAMP-regulated expression of steroidogenic genes.
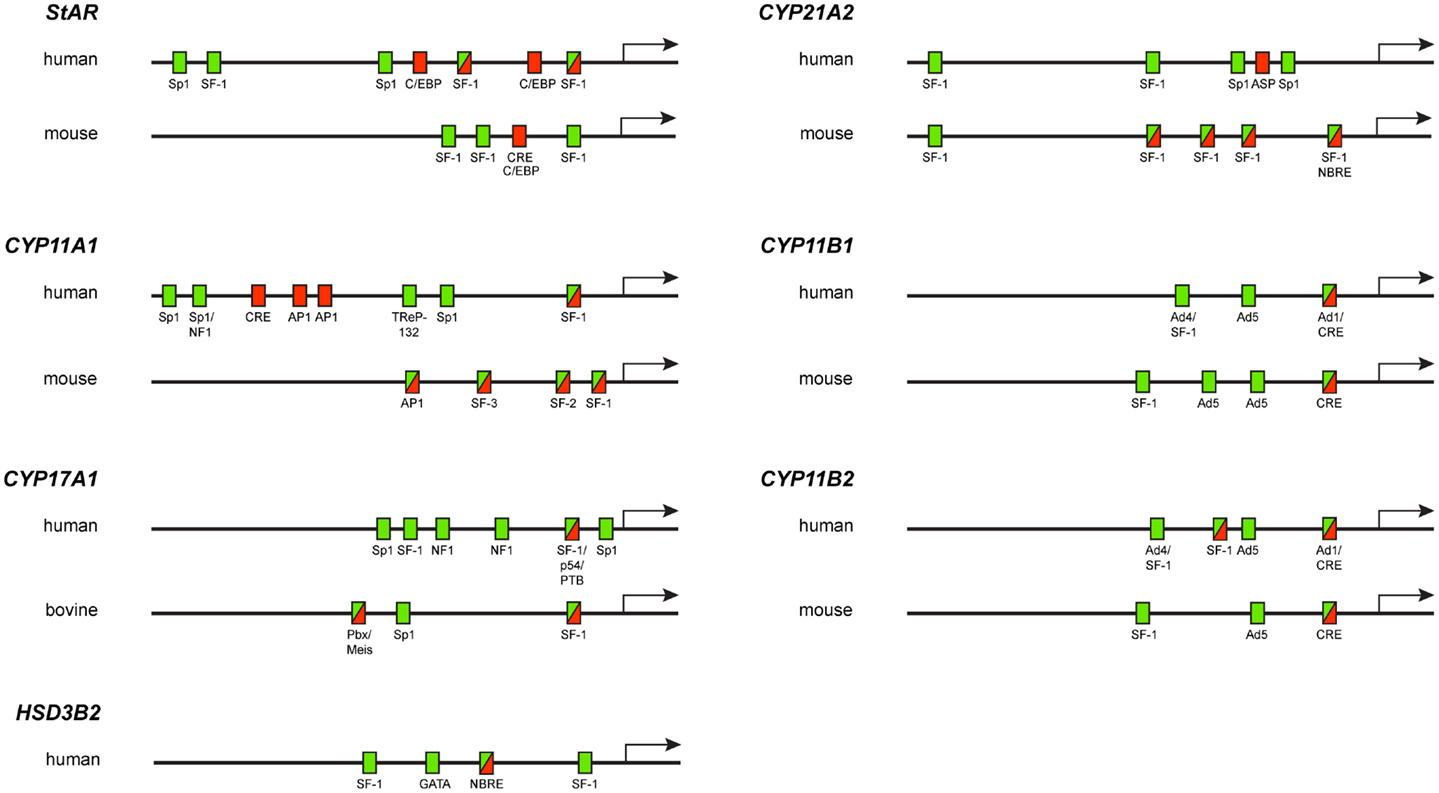
Figure 1. Diagram showing the localization of elements required for basal activity (green rectangles), cAMP-regulated activity (red rectangles) or both basal and cAMP-regulated activities (green–red rectangles) in steroidogenic gene promoters.
Steroidogenic Factor 1
Steroidogenic factor-1 (SF-1; NR5A1) is an orphan member of the nuclear receptor superfamily, which acts as a key regulator of adrenogonadal development and tissue-specific gene expression in steroidogenic cells. Parker and Morohashi groups identified SF-1 by its capacity to bind to and activate transcription from multiple P450 steroidogenic enzyme promoters, which display one or more SF-1-binding sequences in close proximity to the TATA box (72, 73). Furthermore, it has been shown that SF-1 interacts with multiple coactivator and corepressor proteins that function as bridges between transcription factors and the basal transcription system (74–76).
In the human adrenocortical cancer cell line H295R StAR gene transcription is induced by both angiotensin II and cAMP via increased SF-1-binding to a cAMP-responsive region within the first 350 bp upstream of the transcription initiation site (45, 77–79). On the other hand, the mutation of the SF-1 response elements in the mouse promoter of the StAR gene does not impair cAMP-dependent StAR induction in MA-10 Leydig cells or Y1 adrenocortical cells (78).
Adrenocorticotropic hormone/cAMP-dependent transcription of the human CYP11A1 gene, encoding for the mitochondrial enzyme P450scc responsible for cholesterol conversion to pregnenolone, requires the binding of SF-1 at two sites (−40 and −1600 bp) on the gene promoter (80). Remarkably, Omura and Chung laboratories have shown that the upstream promoter elements confer a large portion of CYP11A1 responsiveness to cAMP in Y1 or I-10 Leydig tumor cells and primary adrenal cells of transgenic mice (80, 81). SF-1-dependent activation of the CYP11A1 promoter can be potentiated by cotransfection with c-Jun in steroidogenic JEG3 cells, but not in COS-1 cells (82). Thus, c-Jun and SF-1 act synergistically to activate the transcription of CYP11A1. It has also been shown that in the human adrenal cortex CYP11A1 can be strongly activated by GATA-6 in a SF-1-dependent and DAX1-sensitive fashion (83).
Moreover, it has been shown that SF-1 interacts with the homeodomain-containing transcription factor pituitary homeobox 1 (Ptx/Pitx1) to synergistically promote CYP11B1 gene transcription (84). Interestingly, in the bovine adrenal only one CYP11B1 gene is expressed. Its promoter is characterized by the presence of both a SF-1- and a CRE (cAMP-response element)-like binding site, which are essential for cAMP-driven transcription (85, 86).
Adrenocorticotropic hormone/cAMP signaling also regulates the expression of the human CYP11B2 gene in the zona glomerulosa, where it is responsible for mineralocorticoid production via an SF-1-binding site and a CRE (56).
Furthermore, SF-1 is implicated in the transcriptional regulation of CYP17A1, the gene encoding the P450c17 enzyme, which catalyzes both the 17α-hydroxylation of pregnenolone and progesterone (required for cortisol biosynthesis) and the 17,20-lyase reaction of 17α-hydroxylated steroids (leading to androgen production). Studies carried out on H295R cells revealed that CREs are located within the first 63 bp upstream of the transcriptional initiation site and that a second basal transcription element lies between −184 and −206 bp (87). SF-1 forms a complex with p54nrb/NonO and polypyrimidine tract-binding protein-associated splicing factor (PSF), which binds within those first 60-bp upstream of the transcriptional start site, stimulating CYP17 expression in response to ACTH/cAMP signaling (58). As for CYP11A1, GATA-6 promotes the SF-1-dependent transcription of CYP17 in H295R cells (83).
Many studies have revealed that the ACTH-regulated expression of the CYP21A1 gene, coding for the P450c21 enzyme, which has a key role in the production of cortisol and aldosterone, requires the binding of the nuclear receptors SF-1 and Nur77 to its promoter (88–90). Interestingly, SF-1 binds to a distal region that lies approximately 4.8 kb upstream of the CYP21A1 transcription start site driving adrenal-specific expression of the human gene (65).
cAMP Response Element/Binding Protein/CRE Modulator/Activating Transcription Factor
A family of cAMP-responsive nuclear factors mediates transcriptional regulation by ACTH/cAMP signaling pathway. This family is composed by a large number of proteins, which are encoded by the CREB, CREM, and ATF genes. Those proteins recognize and bind the 8-bp 5′-TGACGTCA-3′ palindromic sequence or a minor variation, called the CRE, which lie within 100 nucleotides of the TATA box in the promoters of eukaryotic cAMP responsive genes (91–93). The members of the CREB family are characterized by their DNA-binding leucine zipper (bZIP) domains and generally they interact with each other to mediate cAMP-dependent transcriptional response (91). Interestingly, the sequences of the mouse (27), human (94), and rat StAR promoters, which exhibit an extensive homology, lack a consensus CRE, similarly to the promoters of different steroid hydroxylase genes whose transcription is regulated by ACTH/cAMP signaling (57). It has been shown that the cAMP-responsive region of the StAR gene promoter exhibits a highly conserved motif (50-TGACTGATGA-30 corresponding to 281/272 bp in the mouse promoter) to which different bZIP families of transcription factors, like not only CREB, CREM, and ATF1, but also AP-1 and C/EBPs (see below) bind to drive StAR transcription (7, 46, 47, 95, 96).
cAMP response element-binding protein has been demonstrated to be the principal player in mediating stimulus-transcription coupling in the ACTH/cAMP pathway. However, knockout mouse CREB studies showed that this action can be compensated by other CRE-binding proteins like CREM and ATF-1 (97). This mechanism also seems to work in the regulation of steroiodogenesis, as CREB family members directly induce StAR gene transcription (6, 7, 47). Interestingly, whereas CREB gene products usually function as positive transactivators, CREM can either activate or repress CRE-mediated transcription (98, 99). Alternative splicing of the CREM gene originates multiple isoforms that can act as either activators (τ, τ1, and τ2) or repressors (α, β, and γ) of transcription (100). Identical functional regions have been identified in CREB and CREMτ proteins (99). When overexpressed, either CREB or CREMτ display qualitatively comparable effects toward cAMP-dependent StAR gene transcription in murine adrenal and gonadal cells (6, 7, 101), whereas CREMα and CREMβ have been shown to repress StAR transcription (7). Further, CREM proteins can bind to CREs as homodimers or as heterodimers with CREB/ATF displaying similar functional outcomes to those of CREB (91). Remarkably, it has been shown that CREB and CREM associate with the proximal rather than the distal StAR promoter upon cAMP analog treatment (47, 48). Sugawara and coworkers compared the implication of CREB and CREM in cAMP-mediated StAR gene expression and identified CREM as the main mediator in H295R cells (101). In contrast, another group showed that CREB and ATF-1, but not CREM, mainly bound to the StAR promoter upon ACTH/cAMP stimulation (47). Besides CREB and CREM, also the CRE-binding protein, ATF-1 is a key regulator of StAR gene expression. ATF-1 differs from CREB and CREM as it lacks the glutamine-rich Q1 domain, although this does not affect its ability to work as a transcriptional activator (91, 102). Interestingly, two paralogs of ATF-1, called CRE-binding protein 1(CRE-BP1 or ATF-2) and ATF-a, display alternative exon splicing and bind to CREs, but they are not able to mediate cAMP-responsive transactivations (91).
cAMP response element-binding protein/CREM/ATF are activated by PKA, PKC, and other kinases that phosphorylate them at specific residues within their N-terminus. Indeed, phosphorylation of CREB at Ser133/119 or CREM at Ser117 leads to CREB–CREM interaction with coactivators like CREB-binding protein/p300 (CBP/p300) (see below) with subsequent stimulation of their transcriptional activity (47, 103–105). It has been reported that cAMP analogs increase CREB phosphorylation in a time-dependent manner in steroidogenic cells. This phosphorylation event correlates with the association of both phosphorylated CREB and CBP to the proximal promoter of the StAR gene (47, 48, 106). The phosphorylation of Ser133 is required for CREB activation; however, it is not sufficient for full activation of the protein. Indeed, it has been shown that a short region C-terminal to the PKA phosphorylation site within CREB protein is required for CREB transcriptional activation (91, 107, 108). The crucial role of CREB phosphorylation by PKA has been also observed in vivo where transgenic mice, which express a non-phosphorylatable mutant of CREB (called CREB-M1, Ser133Ala), exhibit somatotroph hypoplasia and dwarfism (109). Moreover, expression of CREB-M1 in adrenal and gonadal cells strongly decreases cAMP-induced StAR gene expression (6, 7, 101).
cAMP response element-binding protein participates together with GATA-6 and AP-1 to the SF-1- and AP-2-dependent CYP11A1 gene transcription in rodent placenta and ovary (110). Interestingly, the ACTH-stimulated transcription of the human CYP11B1 gene depends on the CREB family member ATF-2 (52). Moreover, as reported above, ACTH/cAMP signaling regulates CYP11B2 gene transcription via a CRE and a SF-1-binding site (56). Similarly, CREB binds to a CRE at the level of the mouse Cyp11b1 gene promoter to drive ACTH-dependent transcription (55).
CCAT/Enhancer-Binding Proteins
CCAT/enhancer-binding proteins are a family of transcription factors containing a highly conserved bZIP at the C-terminus that is involved in dimerization and DNA binding. C/EBPs bind with different affinities to a consensus site consisting of a dyad symmetrical repeat (A/GTTGCGC/TAAC/T) (111). At least six members of the family have been cloned and characterized, named from C/EBPα to C/EBPζ. C/EBPα and C/EBPβ are expressed in steroidogenic cells, the expression levels of C/EBPβ being increased in the nucleus by the action of LH and analogs of cAMP (112, 113). The cAMP-inducible domains of C/EBPs, with the exception for C/EBPβ, lack a PKA phosphorylation site, which implies that C/EBPs are able to mediate cAMP-dependent responses by indirect mechanisms. In contrast, C/EBPβ phosphorylation by PKA within its bZIP domain affects its DNA-binding activity (114).
C/EBPβ targets a binding region in the promoter of the mouse Star gene (281/272 bp), which is also bound by CREB/ATF (see above) and Fos/Jun (see below). Further, two putative C/EBP-binding sites have been identified within the human StAR promoter (43). Consequently, an implication for both C/EBPα and C/EBPβ in StAR gene transcription has been reported (43, 115, 116). C/EBPβ phosphorylation on Thr325 increases its association to the proximal StAR promoter, thus inducing StAR transcription (117, 118). Interestingly, it has been shown that GATA-4 and C/EBPβ directly interact in vitro and in vivo and synergistically activate the StAR promoter only in the presence of PKA (95, 117). This suggests that GATA-C/EBP transcriptional cooperation might promote ACTH/cAMP-dependent StAR transcription in all steroidogenic tissues, as this kind of PKA-dependent synergy has been shown for other members of GATA and C/EBP families. Finally, the disruption of either C/EBPα or C/EBPβ impairs normal reproductive development in female mice, with consequent reduced or altered ovulation and corpus luteum formation inability (112, 119).
AP-1 Family of Transcription Factors
The AP-1 family of transcription factors participates in the regulation of cellular responses to multiple stimuli regulating proliferation, transformation, and cell death (120). It recognizes and binds to a DNA sequence known as the AP-1/phorbol 12-O-tetradecanoate 13-acetate responsive element [AP-1/TRE; TGA(C/G)TCA]. AP-1 is composed by a mixture of homo- and hetero-dimers formed between Jun (c-Jun, JunB, and JunD) and Fos (c-Fos, FosB, Fra1, and Fra2) family members (121–123). Fos members heterodimerize with Jun proteins and with specific members of the CREB/ATF family, but they are not able to form homodimers, whereas Jun members function as homodimers or heterodimers among themselves or with members of the Fos and CREB/ATF families (124). Both Jun and Fos family proteins belong to the bZIP group of DNA-binding transcription factors and their dimerization is necessary for specific and high affinity binding to the palindromic DNA sequence TGAC/GTCA (see above) (125). Studies carried out on the mouse Star promoter identified a highly conserved element (TGACTGA, −81/−75 bp), which shows homology with the AP-1/TRE sequence and overlaps also with the CRE2 sequence (6). Interestingly, it has been shown that Fos and Jun bind to this element, called CRE2/AP-1, thus regulating Star gene transcription (46, 96, 126). Moreover, two additional putative AP-1-binding sites have been identified within the rat Star promoter. c-Fos reduces basal, cAMP-, and c-Jun-mediated rat Star gene transcription in Y-1 adrenocortical cells (127). Indeed, a functional comparison between Fos and Jun revealed that c-Jun is the most powerful AP-1 family member for Star gene transactivation. Accordingly to this, it has been shown that only c-Jun, but not other AP-1 members, plays a pivotal role in the regulation of PKC-mediated Star transcription and steroidogenesis in Leydig and adrenal cells (96, 128).
Not only Protein kinase A but also PKC phosphorylate several Ser and Thr residues on c-Jun and c-Fos. In particular, the treatment with a cAMP analog or a growth factor increases the phosphorylation of c-Jun Ser63 and c-Fos Thr325. Those phosphorylation events are associated to StAR gene transcription and steroidogenesis in mouse Leydig cells (47, 96, 126, 129). Interestingly, ACTH/cAMP-dependent c-Jun and c-Fos phosphorylation increases the association between p-c-Jun/p-c-Fos and the CBP/p300 cofactors (see below), with consequent recruitment of CBP/p300 to the StAR promoter (47, 96, 126). The phosphorylation of c-Fos and c-Jun can alter their capacity to interact with other transcription factors, affecting their dimerization and DNA-binding specificity (124). This explains why the crosstalk between CREB and c-Fos/c-Jun can be associated with both gain and loss of function on the same cis-element in the context of a fine regulation of the transregulatory elements which participate in StAR gene transcription (126).
Sp Family of Transcription Factors
The Sp family of transcription factors is characterized by the presence of three conserved Cys2His2-type zinc fingers at their C-terminus that form the sequence-specific DNA-binding domain (130). They can bind and exert their action through GC/GT-rich promoter elements to regulate the expression of multiple target genes (130, 131). Sp1 is the most well characterized member of the family and it exhibits similar structure and a high homology with Sp3 at the level of its DNA-binding domains, both being able to enhance ore repress promoter activity. However, although Sp1 and Sp3 recognize the same consensus-binding sites, it has been reported that both their DNA-binding properties and regulatory functions differ and depend on the promoter context and cellular background (130, 132, 133).
Regulatory elements for Sp1 and Sp3 have been identified within the human TSPO proximal promoter (134). They have been demonstrated to be strong positive elements for the promoter activity, although differences have been detected in the mechanism by which TSPO is regulated in non-steroidogenic versus steroidogenic cells (134). Sp1 is also involved in the cAMP-dependent transcription of the CYP11A1 gene in human (135), bovine (136, 137), and porcine (138) adrenals. Furthermore, a cooperation between SF-1, Sp1, and CBP has been reported to drive cAMP-dependent CYP11A1 transcription in bovine adrenal (136). Sp1 and Sp3, together with the nuclear fator-1C (NF-1C), bind to the second basal element of the CYP17 gene promoter. This event is crucial for optimal basal transcription (59). Sp1 can also form a complex with GATA-4 or GATA-6 to regulate the expression of CYP17 in the adrenal gland (139). Finally, Sp1 and adrenal-specific protein (ASP) bind to the CYP21 gene promoter to regulate its cAMP-dependent transcription (140). This synergistic action seems to be required for maximal CYP21 induction (140).
DAX-1
DAX-1/NR0B1 encodes an unusual member of the nuclear hormone receptor family of transcription factors. Its mutations cause adrenal hypoplasia congenita (AHC) associated with hypogonadotropic hypogonadism (HHG) (141, 142). DAX-1 expression pattern, mostly restricted to steroidogenic tissues, suggested that it may have a role in the regulation of steroidogenesis. Indeed, in adrenocortical cells, DAX-1 works as a global negative regulator of basal and cAMP-regulated transcription of steroidogenic genes, both through direct binding to gene promoters and interaction with SF-1 and other transcription factors [(143–145); reviewed in Ref. (146)]. In addition, consistently with DAX-1 negative action on steroidogenesis, activation of the PKA pathway by ACTH in adrenocortical cells (147) and FSH in Sertoli cells (148) downregulates Dax-1 expression.
Role of Coregulators
Transcriptional coregulators are crucially implicated in nuclear receptor-mediated transcriptional activation (149–151) and transactivation by other factors, exerting their roles in multiples processes, like histone modification (152–154), chromatin remodeling (155), post-translational modification of transactivation complex members (156, 157), and ordered recruitment of basal transcriptional machinery (158, 159).
The role of coactivators and corepressors in the transcriptional regulation of steroid hydroxylase genes and StAR has been shown by several studies (74, 76). CBP and its functional homolog p300 are transcriptional coactivators that contain multiple functional domains and display intrinsic histone acetyltransferase activity (151, 160), by which they increase transcription factor accessibility to nucleosomal DNA. Classically, ACTH/cAMP signaling triggers the phosphorylation of specific transcription factors, which in turn can bind and recruit CBP/p300 (105, 160–162). As already reported (see above), when phosphorylated at Ser133 CREB is able to interact with CBP (104, 105). Interestingly, CBP/p300 contain PKA consensus sites, the phosphorylation of which is involved in regulating their functions. CBP/p300 play a key role in the transcriptional regulation of the StAR gene. Different studies have shown that cAMP-dependent phosphorylation of CREB at Ser133, c-Jun at Ser63, and c-Fos at Thr325 promotes the association and recruitment of CBP/p300 to the proximal StAR promoter (47, 48, 95, 126). cAMP-stimulated phosphorylation of C/EBPβ at Thr325 also increases C/EBPβ association to the proximal StAR promoter (117). Other factors like SF-1 and GATA-4 (see above), that are bound to the proximal StAR promoter, once phosphorylated in response to ACTH might enhance CBP/p300 recruitment to the promoter. This correlates with the CBP/p300-dependent increased effects of C/EBPβ and GATA-4 on StAR expression (95). Further, when overexpressed, CBP/p300 potentiated CREB, Fos/Jun, C/EBPβ, and GATA-4 transcriptional activity on the StAR gene (95, 126). This event is attenuated by the adenovirus E1A oncoprotein, which acts impairing CBP/p300 histone acetyltransferase activity and/or their interaction with other transcription factors or with the basal transcription machinery.
As already reported, coactivators and corepressors also play a key role in the transcriptional regulation of steroid hydroxylase genes. We report here some examples. Coexpression of CBP/p300 with the zinc finger protein TReP-132 has an additive effect on human CYP11A1 gene promoter activity (163). Similarly, it has been shown that the coactivators CBP/p300, steroid receptor coactivator (SRC)-1, and transcriptional intermediary factor-2 (TIF-2) enhance SF-1-mediated bovine CYP17 transcription, whereas the corepressors nuclear receptor corepressor (N-CoR) and the silencing mediator of retinoic acid and thyroid hormone receptor (SMRT) increase the repressive activity of chicken ovalbumin upstream-transcription factor 1(COUP-TF-1) (164).
Finally, Sewer and coworkers have shown that a corepressor protein called mSin3A inhibits human CYP17 gene transcription by the recruitment of a histone deacetylase to the SF-1/NonO/PSF complex that binds to the CYP17 promoter (58). They have also described that coregulator exchange and sphingosine-sensitive cooperativity of SF-1, general control non-derepressed 5 (GCN5), p54, and p160 coactivators regulate cAMP-dependent CYP17 transcription rate in H295R cells (165). The same group has shown that ACTH/cAMP signaling pathway promotes acid ceramidase (ASAH1) gene transcription via the binding of CREB to multiple region of the ASAH1 promoter. This event triggers the recruitment of CBP/p300 with a related increase in the trimethylation of Lys4 on histone H3 (H3K4) on the ASAH1 promoter in H295R cells (166).
Role of Phosphatase Activity on ACTH/cAMP-Dependent Steroidogenic Gene Transcription
As already discussed, the ACTH-dependent increase in intracellular cAMP levels leads to the activation of PKA, which, in turn, phosphorylates specific nuclear factors to drive steroidogenic gene transcription. Remarkably, in the adrenal cortex ACTH regulation of steroidogenesis depends not only on PKA-mediated Ser/Thr phosphorylation, but also on the activity of protein tyrosine phosphatases (PTPs), which have been implicated in StAR expression and steroidogenesis (167–172). Indeed, the phospho-dephosphorylation of intermediate proteins is considered as a key event in the regulation of steroid biosynthesis. In 1999, Paz and coworkers showed that in vivo treatment with ACTH leads to an increase in total PTPs activity in adrenal zona fasciculata. The stimulation was characterized by a rapid onset (5 min), reached a peak after 15 min of ACTH administration (around twofold) and returned to basal levels after 30 min (168). They showed that the increase in PTPs correlated with a decrease in phosphotyrosine proteins (168). Moreover, the PTPs inhibitors pervanadate (PV) and phenylarsine oxide (PAO) inhibited ACTH- and 8Br-cAMP (a permeant analog of adenosine 3′,5′-phosphate)-dependent steroidogenesis in a dose-dependent fashion, whereas they did not affect steroid production supported by a cell-permeant analog of cholesterol (168). Those studies clearly indicated that PTPs activity has a key role in ACTH/cAMP signaling pathway, acting downstream of PKA activation and upstream of cholesterol transport across the mitochondrial membrane. The same group evaluated steroid production and StAR protein levels in Y1 cells upon PTP inhibition. They reported that PAO reduced ACTH-dependent stimulated steroidogenesis in those cells in a concentration-dependent manner and abrogated StAR protein induction (169). Those effects have been reproduced by a second PTPs inhibitor, benzyl phosphonic acid, which has a different mechanism of action (169). Altogether, those results show that PKA-mediated PTP activation in the steroidogenic system exerts the functional role of mediating StAR protein induction (169). The ACTH/cAMP/PKA signaling pathway stimulates also the release of arachidonic acid (AA) in adrenal and Leydig cells by the concerted action of two enzymes: an acylCoA-thioesterase (Acot2) and an acyl-CoA-synthetase (ACS4) (170, 173). Several reports have shown that AA and its metabolites play a key role in the hormonal control of steroidogenesis by regulating both the expression and function of StAR protein (174, 175). The ACTH/PKA system has been shown to control this pathway upregulating the ACS4 protein levels in adrenal and Leydig cells (175). Two PTP inhibitors both abrogate the ACTH/PKA-dependent ACS4 induction and reduced the effects of cAMP on steroidogenesis and StAR protein levels (175). Interestingly, exogenous AA is able to overcome this PTP-dependent inhibitory effect on StAR protein expression and steroidogenesis (176, 177). Furthermore, Sewer and Waterman have shown that PTP activity is essential for cAMP-dependent transcription of the human CYP17 gene in H295R cells (178). They also investigated whether the inhibition of PTP activity can impair cAMP-dependent mRNA expression of other steroidogenic genes in the adrenal cortex. They have reported that CYP11A1, CYP11B1/2, and CYP21A1 also require PTPs for cAMP-dependent mRNA expression, as the inhibition of both serine/threonine and tyrosine phosphatase activities negatively affected this event (178). Those evidences led those authors to propose a model whereby PKA phosphorylates and activates a dual-specificity phosphatase (DSP), which is able to mediate ACTH/cAMP/PKA-dependent transcription of steroidogenic genes (178, 179). The specific DSP has been identified as mitogen-activated protein phosphatase 1 (MKP-1), whose levels are increased by ACTH and cAMP in H295R cells (180). Moreover, the evidence showing that MKP1 overexpression promotes the transcriptional activity of a human CYP17 promoter-reporter construct and its silencing decreases cAMP-stimulated CYP17 gene expression, suggest a role for MKP-1 in cAMP-dependent CYP17 transcriptional activation (180).
Summary and Conclusion
The studies outlined here have given an important contribution to the understanding of the impact of ACTH on the regulation of steroidogenic gene expression in the adrenal cortex. Given the pivotal role played by ACTH/cAMP signaling in the acute and chronic regulation of steroid secretion and the implication of those hormones in diverse arrays of fundamental physiological processes, an in-depth investigation is needed to completely elucidate the ACTH-dependent transcriptional regulatory mechanisms that control steroid secretion. Indeed, some aspects addressed in this review still represent a challenge for future studies, which might provide the scientific community with a broader picture of the complex signaling pathways and the intricate transcriptional circuitries that coordinately ensure optimal hormonal output.
Author Contributions
All authors listed, have made substantial, direct, and intellectual contribution to the work, and approved it for publication.
Conflict of Interest Statement
The authors declare that the research was conducted in the absence of any commercial or financial relationships that could be construed as a potential conflict of interest.
References
1. Simpson ER, Waterman MR. Regulation of the synthesis of steroidogenic enzymes in adrenal cortical cells by ACTH. Annu Rev Physiol (1988) 50:427–40. doi:10.1146/annurev.ph.50.030188.002235
2. Markowska A, Rebuffat P, Rocco S, Gottardo G, Mazzocchi G, Nussdorfer GG. Evidence that an extrahypothalamic pituitary corticotropin releasing hormone (CRH)/adrenocorticotropin (ACTH) system controls adrenal growth and secretion in rats. Cell Tissue Res (1993) 272:439–45. doi:10.1007/BF00318550
3. Miller WL, Bose HS. Early steps in steroidogenesis: intracellular cholesterol trafficking. J Lipid Res (2011) 52:2111–35. doi:10.1194/jlr.R016675
4. Sewer MB, Waterman MR. ACTH modulation of transcription factors responsible for steroid hydroxylase gene expression in the adrenal cortex. Microsc Res Tech (2003) 61:300–7. doi:10.1002/jemt.10339
5. Stocco DM. Tracking the role of a StAR in the sky of the new millennium. Mol Endocrinol (2001) 15:1245–54. doi:10.1210/mend.15.8.0697
6. Manna PR, Dyson MT, Eubank DW, Clark BJ, Lalli E, Sassone-Corsi P, et al. Regulation of steroidogenesis and the steroidogenic acute regulatory protein by a member of the cAMP response-element binding protein family. Mol Endocrinol (2002) 16:184–99. doi:10.1210/mend.16.1.0759
7. Manna PR, Eubank DW, Lalli E, Sassone-Corsi P, Stocco DM. Transcriptional regulation of the mouse steroidogenic acute regulatory protein gene by the cAMP response-element binding protein and steroidogenic factor 1. J Mol Endocrinol (2003) 30:381–97. doi:10.1677/jme.0.0300381
8. Manna PR, Dyson MT, Stocco DM. Regulation of the steroidogenic acute regulatory protein gene expression: present and future perspectives. Mol Hum Reprod (2009) 15:321–33. doi:10.1093/molehr/gap025
9. Mason JI, Rainey WE. Steroidogenesis in the human fetal adrenal: a role for cholesterol synthesized de novo. J Clin Endocrinol Metab (1987) 64:140–7. doi:10.1210/jcem-64-1-140
10. Gwynne JT, Strauss JF 3rd. The role of lipoproteins in steroidogenesis and cholesterol metabolism in steroidogenic glands. Endocr Rev (1982) 3:299–329. doi:10.1210/edrv-3-3-299
11. Horton JD, Goldstein JL, Brown MS. SREBPs: activators of the complete program of cholesterol and fatty acid synthesis in the liver. J Clin Invest (2002) 109:1125–31. doi:10.1172/JCI15593
12. Brown MS, Kovanen PT, Goldstein JL. Receptor-mediated uptake of lipoprotein-cholesterol and its utilization for steroid synthesis in the adrenal cortex. Recent Prog Horm Res (1979) 35:215–57. doi:10.1126/science.6261329
13. Chang TY, Chang CC, Ohgami N, Yamauchi Y. Cholesterol sensing, trafficking and esterification. Annu Rev Cell Dev Biol (2006) 22:129–57. doi:10.1146/annurev.cellbio.22.010305.104656
14. Miller WL. Regulation of steroidogenesis by electron transfer. Endocrinology (2005) 146:2544–50. doi:10.1210/en.2005-0096
15. Agarwal AK, Auchus RJ. Cellular redox state regulates hydroxysteroid dehydrogenase activity and intracellular hormone potency. Endocrinology (2005) 146:2531–8. doi:10.1210/en.2005-0061
16. Penning TM. Molecular endocrinology of hydroxysteroid dehydrogenases. Endocr Rev (1997) 18:281–305. doi:10.1210/edrv.18.3.0302
17. Auchus RJ, Rainey WE. Adrenarche – physiology, biochemistry and human disease. Clin Endocrinol (Oxf) (2004) 60:288–96. doi:10.1046/j.1365-2265.2003.01858
18. Mulatero P, Curnow KM, Aupetit-Faisant B, Foekling M, Gomez-Sanchez C, Veglio F, et al. Recombinant CYP11B genes encode enzymes that can catalyze conversion of 11-deoxycortisol to cortisol, 18-hydroxycortisol, and 18-oxocortisol. J Clin Endocrinol Metab (1998) 83:3996–4001. doi:10.1210/jcem.83.11.5237
19. Kater CE, Biglieri EG. Disorders of steroid 17α-hydroxylase deficiency. Endocrinol Metab Clin North Am (1994) 23:341–57.
20. Voutilainen R, Miller WL. Coordinate tropic hormone regulation of mRNAs for insulin-like growth factor II and the cholesterol side-chain cleavage enzyme, P450scc, in human steroidogenic tissues. Proc Natl Acad Sci U S A (1987) 84:1590–4. doi:10.1073/pnas.84.6.1590
21. Mesiano S, Mellon SH, Gospodarowicz D, Di Blasio AM, Jaffe RB. Basic fibroblast growth factor expression is regulated by corticotropin in the human fetal adrenal: a model for adrenal growth regulation. Proc Natl Acad Sci U S A (1991) 88:5428–32. doi:10.1073/pnas.88.12.5428
22. Coulter CL, Read LC, Carr BR, Tarantal AF, Barry S, Styne DM. A role for epidermal growth factor in the morphological and functional maturation of the adrenal gland in the fetal rhesus monkey in vivo. J Clin Endocrinol Metab (1996) 81:1254–60. doi:10.1210/jcem.81.3.8772608
23. Clark BJ, Soo SC, Caron KM, Ikeda Y, Parker KL, Stocco DM. Hormonal and developmental regulation of the steroidogenic acute regulatory (StAR) protein. Mol Endocrinol (1995) 9:1346–55. doi:10.1210/mend.9.10.8544843
24. Arakane F, King SR, Du Y, Kallen CB, Walsh LP, Watari H, et al. Phosphorylation of steroidogenic acute regulatory protein (StAR) modulates its steroidogenic activity. J Biol Chem (1997) 272:32656–62. doi:10.1074/jbc.272.51.32656
25. Pon LA, Hartigan JA, Orme-Johnson NR. Acute ACTH regulation of adrenal corticosteroid biosynthesis: rapid accumulation of a phosphoprotein. J Biol Chem (1986) 261:13309–16.
26. Epstein LF, Orme-Johnson NR. Regulation of steroid hormone biosynthesis: identification of precursors of a phosphoprotein targeted to the mitochondrion in stimulated rat adrenal cortex cells. J Biol Chem (1991) 266:19739–45.
27. Clark BJ, Wells J, King SR, Stocco DM. The purification, cloning and expression of a novel luteinizing hormone-induced mitochondrial protein in MA-10 mouse Leydig tumor cells. Characterization of the steroidogenic acute regulatory protein (StAR). J Biol Chem (1994) 269:28314–22.
28. Lin D, Sugawara T, Strauss JF III, Clark BJ, Stocco DM, Saenger P, et al. Role of steroidogenic acute regulatory protein in adrenal and gonadal steroidogenesis. Science (1995) 267:1828–31. doi:10.1126/science.7892608
29. Bose HS, Sugawara T, Strauss JF III, Miller WL; International Congenital Lipoid Adrenal Hyperplasia Consortium. The pathophysiology and genetics of congenital lipoid adrenal hyperplasia. N Engl J Med (1996) 335:1870–8. doi:10.1056/NEJM199612193352503
30. Caron KM, Soo SC, Wetsel WC, Stocco DM, Clark BJ, Parker KL. Targeted disruption of the mouse gene encoding steroidogenic acute regulatory protein provides insights into congenital lipoid adrenal hyperplasia. Proc Natl Acad Sci U S A (1997) 94:11540–5. doi:10.1073/pnas.94.21.11540
31. Hasegawa T, Zhao L, Caron KM, Majdic G, Suzuki T, Shizawa S, et al. Developmental roles of the steroidogenic acute regulatory protein (StAR) as revealed by StAR knockout mice. Mol Endocrinol (2000) 14:1462–71. doi:10.1210/mend.14.9.0515
32. Miller WL. Steroidogenic acute regulatory protein (StAR), a novel mitochondrial cholesterol transporter. Biochim Biophys Acta (2007) 1771:663–76. doi:10.1016/j.bbalip.2007.02.012
33. Arakane F, Sugawara T, Nishino H, Liu Z, Holt JA, Pain D, et al. Steroidogenic acute regulatory protein (StAR) retains activity in the absence of its mitochondrial targeting sequence: implications for the mechanism of StAR action. Proc Natl Acad Sci U S A (1996) 93:13731–6. doi:10.1073/pnas.93.24.13731
34. Bose HS, Lingappa VR, Miller WL. Rapid regulation of steroidogenesis by mitochondrial protein import. Nature (2002) 417:87–91. doi:10.1038/417087a
35. Bose HS, Whittal RM, Baldwin MA, Miller WL. The active form of the steroidogenic acute regulatory protein, StAR, appears to be a molten globule. Proc Natl Acad Sci U S A (1999) 96:7250–5. doi:10.1073/pnas.96.13.7250
36. Baker BY, Yaworsky DC, Miller WL. A pH-dependent molten globule transition is required for activity of the steroidogenic acute regulatory protein, StAR. J Biol Chem (2005) 280:41753–60. doi:10.1074/jbc.M510241200
37. Tuckey RC, Headlam MJ, Bose HS, Miller WL. Transfer of cholesterol between phospholipid vesicles mediated by the steroidogenic acute regulatory protein (StAR). J Biol Chem (2002) 277:47123–8. doi:10.1074/jbc.M206965200
38. Baker BY, Epand RF, Epand RM, Miller WL. Cholesterol binding does not predict activity of the steroidogenic acute regulatory protein, StAR. J Biol Chem (2007) 282:10223–32. doi:10.1074/jbc.M611221200
39. Papadopoulos V. Peripheral-type benzodiazepine/diazepam binding inhibitor receptor: biological role in steroidogenic cell function. Endocr Rev (1993) 14:222–40. doi:10.1210/edrv-14-2-222
40. Hauet T, Yao ZX, Bose HS, Wall CT, Han Z, Li W, et al. Peripheral-type benzodiazepine receptor-mediated action of steroidogenic acute regulatory protein on cholesterol entry into Leydig cell mitochondria. Mol Endocrinol (2005) 19:540–54. doi:10.1210/me.2004-0307
41. Bose M, Whittal RM, Miller WL, Bose HS. Steroidogenic activity of StAR requires contact with mitochondrial VDAC1 and PCP. J Biol Chem (2008) 283:8837–45. doi:10.1074/jbc.M709221200
42. Miller WL, Strauss JF 3rd. Molecular pathology and mechanism of action of the steroidogenic acute regulatory protein, StAR. J Steroid Biochem Mol Biol (1999) 69:131–41. doi:10.1016/S0960-0760(98)00153-8
43. Christenson LK, Johnson PF, McAllister JM, Strauss JF III. CCAAT/enhancer-binding proteins regulate expression of the human steroidogenic acute regulatory protein (StAR) gene. J Biol Chem (1999) 274:26591–8. doi:10.1074/jbc.274.37.26591
44. Sugawara T, Saito M, Fujimoto S. Sp1 and SF-1 interact and cooperate in the regulation of human steroidogenic acute regulatory protein gene expression. Endocrinology (2000) 141:2895–903. doi:10.1210/endo.141.8.7602
45. Sugawara T, Holt JA, Kiriakidou M, Strauss JR III. Steroidogenic factor 1-dependent promoter activity of the human steroidogenic acute regulatory protein (StAR) gene. Biochemistry (1996) 35:9052–9. doi:10.1021/bi960057r
46. Manna PR, Eubank DW, Stocco DM. Assessment of the role of activator protein-1 on transcription of the mouse steroidogenic acute regulatory protein gene. Mol Endocrinol (2004) 18:558–73. doi:10.1210/me.2003-0223
47. Clem BF, Hudson EA, Clark BJ. Cyclic adenosine 3′,5′-monophosphate (cAMP) enhances cAMP-responsive element binding (CREB) protein phosphorylation and phospho-CREB interaction with the mouse steroidogenic acute regulatory protein gene promoter. Endocrinology (2005) 146:1348–56. doi:10.1210/en.2004-0761
48. Hiroi H, Christenson LK, Chang L, Sammel MD, Berger SL, Strauss JF III. Temporal and spatial changes in transcription factor binding and histone modifications at the steroidogenic acute regulatory protein (StAR) locus associated with StAR transcription. Mol Endocrinol (2004) 18:791–806. doi:10.1210/me.2003-0305
49. Shih MC, Chiu YN, Hu MC, Guo IC, Chung BC. Regulation of steroid production: analysis of Cyp11a1 promoter. Mol Cell Endocrinol (2011) 10:80–4. doi:10.1016/j.mce.2010.12.017
50. Rice DA, Kirkman MS, Aitken LD, Mouw AR, Schimmer BP, Parker KL. Analysis of the promoter region of the gene encoding mouse cholesterol side-chain cleavage enzyme. J Biol Chem (1990) 15:11713–20.
51. Handler JD, Schimmer BP, Flynn TR, Szyf M, Seidman JG, Parker KL. An enhancer element and a functional cyclic AMP-dependent protein kinase are required for expression of adrenocortical 21-hydroxylase. J Biol Chem (1988) 263:13068–73.
52. Wang XL, Bassett M, Zhang Y, Yin S, Clyne C, White PC, et al. Transcriptional regulation of human 11beta-hydroxylase (hCYP11B1). Endocrinology (2000) 141:3587–94. doi:10.1210/endo.141.10.7689
53. Bassett MH, Zhang Y, White PC, Rainey WE. Regulation of human CYP11B2 and CYP11B1: comparing the role of the common CRE/Ad1 element. Endocr Res (2000) 26:941–51. doi:10.3109/07435800009048620
54. Cheng LC, Pai TW, Li LA. Regulation of human CYP11B1 and CYP11B2 promoters by transposable elements and conserved cis elements. Steroids (2012) 77:100–9. doi:10.1016/j.steroids.2011.10.010
55. Rice DA, Aitken LD, Vandenbark GR, Mouw AR, Franklin A, Schimmer BP, et al. A cAMP-responsive element regulates expression of the mouse steroid 11 betahydroxylase gene. J Biol Chem (1989) 264:14011–5.
56. Clyne CD, Zhang Y, Slutsker L, Mathis JM, White PC, Rainey WE. Angiotensin II and potassium regulate human CYP11B2 transcription through common cis-elements. Mol Endocrinol (1997) 11:638–49. doi:10.1210/mend.11.5.9920
57. Waterman MR. Biochemical diversity of cAMP-dependent transcription of steroid hydroxylase genes in the adrenal cortex. J Biol Chem (1994) 269:27783–6.
58. Sewer MB, Nguyen V, Huang C, Tucker PW, Kagawa N, Waterman MR. Transcriptional activation of human CYP17 in H295R adrenocortical cells depends on complex formation between p54nrb/NonO, PSF and SF-1, a complex which also participates in repression of transcription. Endocrinology (2002) 143:1280–90. doi:10.1210/endo.143.4.8748
59. Lin CJ, Martens JWM, Miller WL. NF-1C, Sp1, and Sp3 are essential for transcription of the human gene for P450c17 (steroid 17a-hydroxylase/17,20 lyase) in human adrenal NCI-H295A cells. Mol Endocrinol (2001) 15:1277–93. doi:10.1210/mend.15.8.0679
60. Lund J, Ahlgren R, Wu D, Kagimoto M, Simpson ER, Waterman MR. Transcriptional regulation of the bovine CYP17 (P-45017α) gene. J Biol Chem (1990) 265:3304–12.
61. Bakke M, Lund J. Mutually exclusive interactions of two nuclear orphan receptors determine activity of a cyclic adenosine 3′,5′-monophosphate-responsive sequence in the bovine CYP17 gene. Mol Endocrinol (1995) 9:327–9. doi:10.1210/mend.9.3.7776979
62. Kagawa N, Ogo A, Takahashi Y, Iwamatsu A, Waterman M. A cAMP-regulatory sequence (CRS1) of CYP17 is a cellular target for the homeodomain protein Pbx1. J Biol Chem (1994) 269:18716–9.
63. Bischof L, Kagawa N, Moskow JJ, Takahashi Y, Iwamatsu A, Buchberg AM, et al. Members of the Meis and Pbx homeodomain proteins families cooperatively bind a cAMP-responsive sequence (CRS1) from bovine CYP17. J Biol Chem (1998) 273:7941–8. doi:10.1074/jbc.273.14.7941
64. Kagawa N, Waterman MR. Purification and characterization of a transcription factor which appears to regulate cAMP responsiveness of the human CYP21B gene. J Biol Chem (1992) 15:25213–9.
65. Wijesuriya SD, Zhang G, Dardis A, Miller WL. Transcriptional regulatory elements of the human gene for cytochrome P450c21 (steroid 21-hydroxylase) lie within intron 35 of the linked C4B gene. J Biol Chem (1999) 274:38097–106. doi:10.1074/jbc.274.53.38097
66. Wilson TE, Mouw AR, Weaver CA, Milbrandt J, Parker KL. The orphan nuclear receptor NGFI-B regulates expression of the gene encoding steroid 21-hydroxylase. Mol Cell Biol (1993) 13:861–8. doi:10.1128/MCB.13.2.861
67. Parker KL, Schimmer BP, Chaplin DD, Seidman JG. Characterization of a regulatory region of the steroid 21-hydroxylase gene. J Biol Chem (1986) 261:15353–5.
68. Schimmer BP, Parker KL. Promoter elements of the mouse 21-hydroxylase (Cyp-21) gene involved in cell-selective and cAMP-dependent gene expression. J Steroid Biochem Mol Biol (1992) 43:937–50. doi:10.1016/0960-0760(92)90322-A
69. Milstone DS, Shaw SK, Parker KL, Szyf M, Seidman JG. An element regulating adrenal-specific steroid 21-hydroxylase expression is located within the slp gene. J Biol Chem (1992) 267:21924–7.
70. Rice DA, Mouw AR, Bogerd AM, Parker KL. A shared promoter element regulates the expression of three steroidogenic enzymes. Mol Endocrinol (1991) 5:1552–61. doi:10.1210/mend-5-10-1552
71. Udhane S, Kempna P, Hofer G, Mullis PE, Flück CE. Differential regulation of human 3β-hydroxysteroid dehydrogenase type 2 for steroid hormone biosynthesis by starvation and cyclic AMP stimulation: studies in the human adrenal NCI-H295R cell model. PLoS One (2013) 9:e68691. doi:10.1371/journal.pone.0068691
72. Lala DS, Rice DA, Parker KL. Steroidogenic factor I, a key regulator of steroidogenic enzyme expression, is the mouse homolog of fushi tarazu-factor I. Mol Endocrinol (1992) 6:1249–58. doi:10.1210/mend.6.8.1406703
73. Morohashi K, Honda S, Inomata Y, Handa H, Omura T. A common trans-acting factor, Ad4-binding protein, to the promoters of steroidogenic P-450s. J Biol Chem (1992) 25:17913–9.
74. Hammer GD, Krylova I, Zhang Y, Darimont BD, Simpson K, Weigel NL, et al. Phosphorylation of the nuclear receptor SF-1 modulates cofactor recruitment: integration of hormone signalling in reproduction and stress. Mol Cell (1999) 3:521–6. doi:10.1016/S1097-2765(00)80480-3
75. Ito M, Yu RN, Jameson JL. Steroidogenic factor-I contains a carboxy-terminal transcriptional activation domain that interacts with steroid receptor coactivator-1. Mol Endocrinol (1998) 12:290–301. doi:10.1210/mend.12.2.0059
76. Monte D, DeWitte F, Hum DW. Regulation of the human P450scc gene by steroidogenic factor 1 is mediated by CBP/p300. J Biol Chem (1998) 273:4585–91. doi:10.1074/jbc.273.8.4585
77. Clark BJ, Combs R. Angiotensin II and cyclic adenosine 3′,5′-monophosphate induce human steroidogenic acute regulatory protein transcription through a common steroidogenic factor-1 element. Endocrinology (1999) 140:4390–8. doi:10.1210/endo.140.10.7085
78. Caron KM, Ikeda Y, Soo SC, Stocco DM, Parker KL, Clark BJ. Characterization of the promoter region of the mouse gene encoding the steroidogenic acute regulatory protein. Mol Endocrinol (1997) 11:137–47. doi:10.1210/mend.11.2.9880
79. Sandhoff TW, Hales DB, Hales KH, McLean MP. Transcriptional regulation of steroidogenic acute regulatory protein gene by steroidogenic factor 1. Endocrinology (1998) 139:4820–31. doi:10.1210/endo.139.12.6345
80. Hu MC, Hsu NC, Pai CI, Wang CK, Chung B. Functions of the upstream and proximal steroidogenic factor 1 (SF-1)-binding sites in the CYP11A1 promoter in basal transcription and hormonal response. Mol Endocrinol (2001) 15:812–28. doi:10.1210/me.2002-0055
81. Takayama K, Morohashi K, Honda S, Hara N, Omura T. Contribution of Ad4BP, a steroidogenic cell-specific transcription factor, to regulation of the human CYP11A and bovine CYP11B genes through their distal promoters. J Biochem (1994) 116:193–203.
82. Huang Y, Hu M, Hsu N, Wang CL, Chung B. Action of hormone responsive sequence in 2.3 kb promoter of CYP11A1. Mol Cell Endocrinol (2001) 175:205–10. doi:10.1016/S0303-7207(01)00388-4
83. Jimenez P, Saner K, Mayhew B, Rainey WE. GATA-6 is expressed in the human adrenal and regulates transcription of genes required for adrenal androgen biosynthesis. Endocrinology (2003) 144:4285–8. doi:10.1210/en.2003-0472
84. Hiroi N, Kino T, Bassett M, Rainey WE, Phung M, Abu-Asab M, et al. Pituitary homeobox factor 1, a novel transcription factor in the adrenal regulating steroid 11beta-hydroxylase. Horm Metab Res (2003) 35:273–8. doi:10.1055/s-2003-41301
85. Hashimoto T, Morohashi K, Takayama K, Honda S, Wada T, Handa H, et al. Cooperative transcription activation between Ad1, CRE-like element, and other elements in the CYP11B gene promoter. J Biochem (1992) 12:573–5.
86. Morohashi K, Zanger UM, Honda S, Hara M, Waterman MR, Omura T. Activation of CYP11A and CYP11B gene promoters by the steroidogenic cell-specific transcription factor, Ad4BP. Mol Endocrinol (1993) 7:1196–204. doi:10.1210/mend.7.9.8247022
87. Rodriguez H, Hum DW, Staels B, Miller WL. Transcription of the human genes for cytochrome P450scc and P450c17 is regulated differently in human adrenal NCI-H295 cells than in mouse adrenal Y1 cells. J Clin Endocrinol Metab (1997) 82:365–71. doi:10.1210/jcem.82.2.3721
88. Rice DA, Kronenberg MS, Mouw AR, Aitken LD, Franklin A, Schimmer BP, et al. Multiple regulatory elements determine adrenocortical expression of steroid 21-hydroxylase. J Biol Chem (1990) 265:8052–8.
89. Parissenti AM, Parker KL, Schimmer BP. Identification of promoter elements in the mouse 21-hydroxylase (Cyp21) gene that require a functional cyclic adenosine 3′,5′-monophosphate-dependent protein kinase. Mol Endocrinol (1993) 7:283–90. doi:10.1210/mend.7.2.8385740
90. Parker KL, Schimmer BP. Transcriptional regulation of the genes encoding the cytochrome P-450 steroid hydroxylases. Vitam Horm (1995) 51:339–70. doi:10.1016/S0083-6729(08)61044-4
91. Meyer TE, Habener JF. Cyclic adenosine 3′,5′-monophosphate response element binding protein (CREB) and related transcription-activating deoxyribonucleic acid-binding proteins. Endocr Rev (1993) 14:269–90. doi:10.1210/edrv-14-3-269
92. Montminy M. Transcriptional regulation by cyclic AMP. Annu Rev Biochem (1997) 66:807–22. doi:10.1146/annurev.biochem.66.1.807
93. Lalli E, Sassone-Corsi P. Signal transduction and gene regulation: the nuclear response to cAMP. J Biol Chem (1994) 269:17359–62.
94. Sugawara T, Holt JA, Driscoll D, Strauss JF III, Lin D, Miller WL, et al. Human steroidogenic acute regulatory protein: functional activity in COS-1 cells, tissue-specific expression, and mapping of the structural gene to 8p11.2 and a pseudogene to chromosome 13. Proc Natl Acad Sci U S A (1995) 92:4778–82. doi:10.1073/pnas.92.11.4778
95. Silverman E, Yivgi-Ohana N, Sher N, Bell M, Eimerl S, Orly J. Transcriptional activation of the steroidogenic acute regulatory protein (StAR) gene: GATA-4 and CCAAT/enhancer-binding protein beta confer synergistic responsiveness in hormone-treated rat granulosa and HEK293 cell models. Mol Cell Endocrinol (2006) 252:92–101. doi:10.1016/j.mce.2006.03.008
96. Manna PR, Stocco DM. The role of JUN in the regulation of PRKCC-mediated STAR expression and steroidogenesis in mouse Leydig cells. J Mol Endocrinol (2008) 41:329–41. doi:10.1677/JME-08-0077
97. Hummler E, Cole TJ, Blendy JA, Ganss R, Aguzzi A, Schmid W, et al. Targeted mutation of the CREB gene: compensation within the CREB/ATF family of transcription factors. Proc Natl Acad Sci U S A (1994) 91:5647–51. doi:10.1073/pnas.91.12.5647
98. Foulkes NS, Sassone-Corsi P. More is better: activators and repressors from the same gene. Cell (1992) 68:411–4. doi:10.1016/0092-8674(92)90178-F
99. Meyer TE, Habener JF. Cyclic AMP response element binding protein CREB and modulator protein CREM are products of distinct genes. Nucleic Acids Res (1992) 20:6106. doi:10.1093/nar/20.22.6106
100. Walker WH, Habener JF. Role of transcription factors CREB and CREM in cAMP-regulated transcription during spermatogenesis. Trends Endocrinol Metab (1996) 7:133–8. doi:10.1016/1043-2760(96)00035-5
101. Sugawara T, Sakuragi N, Minakami H. CREM confers cAMP responsiveness in human steroidogenic acute regulatory protein expression in NCI-H295R cells rather than SF-1/Ad4BP. J Endocrinol (2006) 191:327–327. doi:10.1677/joe.1.06601
102. Della Fazia MA, Servillo G, Sassone-Corsi P. Cyclic AMP signalling and cellular proliferation: regulation of CREB and CREM. FEBS Lett (1997) 410:22–4. doi:10.1016/S0014-5793(97)00445-6
103. Gonzalez GA, Montminy MR. Cyclic AMP stimulates somatostatin gene transcription by phosphorylation of CREB at serine 133. Cell (1989) 59:675–80. doi:10.1016/0092-8674(89)90013-5
104. Chrivia JC, Kwok RP, Lamb N, Hagiwara M, Montminy MR, Goodman RH. Phosphorylated CREB binds specifically to the nuclear protein CBP. Nature (1993) 365:855–9. doi:10.1038/365855a0
105. Parker D, Ferreri K, Nakajima T, LaMorte VJ, Evans R, Koerber SC, et al. Phosphorylation of CREB at Ser-133 induces complex formation with CREB-binding protein via a direct mechanism. Mol Cell Biol (1996) 16:694–703. doi:10.1128/MCB.16.2.694
106. Hiroi H, Christenson LK, Strauss JF III. Regulation of transcription of the steroidogenic acute regulatory protein (StAR) gene: temporal and spatial changes in transcription factor binding and histone modification. Mol Cell Endocrinol (2004) 215:119–26. doi:10.1016/j.mce.2003.11.014
107. Bonni A, Ginty DD, Dudek H, Greenberg ME. Serine 133-phosphorylated CREB induces transcription via a cooperative mechanism that may confer specificity to neurotrophin signals. Mol Cell Neurosci (1995) 6:168–83. doi:10.1006/mcne.1995.1015
108. Johannessen M, Delghandi MP, Moens U. What turns CREB on? Cell Signal (2004) 16:1211–27. doi:10.1016/j.cellsig.2004.05.001
109. Struthers RS, Vale WW, Arias C, Sawchenko PE, Montminy MR. Somatotroph hypoplasia and dwarfism in transgenic mice expressing a nonphosphorylatable CREB mutant. Nature (1991) 350:622–4. doi:10.1038/350622a0
110. Sher N, Yivgi-Ohana N, Orly J. Transcriptional regulation of the cholesterol side chain cleavage cytochrome P450 gene (CYP11A1) revisited: binding of GATA, cyclic adenosine 3′,5′-monophosphate response element-binding protein and activating protein (AP)-1 proteins to a distal novel cluster of cis-regulatory elements potentiates AP-2 and steroidogenic Factor-1-dependent gene expression in the rodent placenta and ovary. Mol Endocrinol (2007) 21:948–62. doi:10.1210/en.2008-054
111. Osada S, Yamamoto H, Nishihara T, Imagawa M. DNA binding specificity of the CCAAT/enhancer-binding protein transcription factor family. J Biol Chem (1996) 271:3891–6. doi:10.1074/jbc.271.7.3891
112. Piontkewitz Y, Enerback S, Hedin L. Expression of CCAAT enhancer binding protein-alpha (C/EBP alpha) in the rat ovary: implications for follicular development and ovulation. Dev Biol (1996) 179:288–96. doi:10.1006/dbio.1996.0258
113. Nalbant D, Williams SC, Stocco DM, Khan SA. Luteinizing hormone dependent gene regulation in Leydig cells may be mediated by CCAAT/enhancer-binding protein-beta. Endocrinology (1998) 139:272–9. doi:10.1210/endo.139.1.5663
114. Wilson HL, Roesler WJ. CCAAT/enhancer binding proteins: do they possess intrinsic cAMP-inducible activity? Mol Cell Endocrinol (2002) 188:15–20. doi:10.1016/S0303-7207(01)00754-7
115. Reinhart AJ, Williams SC, Clark BJ, Stocco DM. SF-1 (steroidogenic factor-1) and C/EBP beta (CCAAT/enhancer binding protein-b) cooperate to regulate the murine StAR (steroidogenic acute regulatory) promoter. Mol Endocrinol (1999) 13:729–41. doi:10.1210/mend.13.5.0279
116. Silverman E, Eimerl S, Orly J. CCAAT enhancer-binding protein beta and GATA-4 binding regions within the promoter of the steroidogenic acute regulatory protein (StAR) gene are required for transcription in rat ovarian cells. J Biol Chem (1999) 274:17987–96. doi:10.1074/jbc.274.25.17987
117. Tremblay JJ, Hamel F, Viger RS. Protein kinase A-dependent cooperation between GATA and CCAAT/enhancer-binding protein transcription factors regulates steroidogenic acute regulatory protein promoter activity. Endocrinology (2002) 143:3935–45. doi:10.1210/en.2002-220413
118. Hsu CC, Lu CW, Huang BM, Wu MH, Tsai SJ. Cyclic adenosine 3′,5′-monophosphate response element-binding protein and CCAAT/enhancer-binding protein mediate prostaglandin E2-induced steroidogenic acute regulatory protein expression in endometriotic stromal cells. Am J Pathol (2008) 173:433–41. doi:10.2353/ajpath.2008.080199
119. Sterneck E, Tessarollo L, Johnson PF. An essential role for C/EBPbeta in female reproduction. Genes Dev (1997) 11:2153–62. doi:10.1101/gad.11.17.2153
120. Shaulian E, Karin M. AP-1 as a regulator of cell life and death. Nat Cell Biol (2002) 4:131–6. doi:10.1038/ncb0502-e131
121. Sassone-Corsi P, Lamph WW, Kamps M, Verma IM. Fos-associated cellular p39 is related to nuclear transcription factor AP-1. Cell (1988) 54:553–60. doi:10.1016/0092-8674(88)90077-3
122. Curran T, Franza BR Jr. Fos and Jun: the AP-1 connection. Cell (1998) 55:395–7. doi:10.1016/0092-8674(88)90024-4
123. Halazonetis TD, Georgopoulos K, Greenberg ME, Leder P. c-Jun dimerizes with itself and with c-Fos, forming complexes of different DNA binding affinities. Cell (1988) 55:917–24. doi:10.1016/0092-8674(88)90147-X
124. Hai T, Curran T. Cross-family dimerization of transcription factors Fos/Jun and ATF/CREB alters DNA binding specificity. Proc Natl Acad Sci U S A (1991) 1:3720–4. doi:10.1016/0092-8674(88)90147-X
125. Gentz R, Rauscher FJ III, Abate C, Curran T. Parallel association of Fos and Jun leucine zippers juxtaposes DNA binding domains. Science (1989) 243:1695–9. doi:10.1126/science.2494702
126. Manna PR, Stocco DM. Crosstalk of CREB and Fos/Jun on a single cis-element: transcriptional repression of the steroidogenic acute regulatory protein gene. J Mol Endocrinol (2007) 39:261–77. doi:10.1677/JME-07-0065
127. Shea-Eaton W, Sandhoff TW, Lopez D, Hales DB, McLean MP. Transcriptional repression of the rat steroidogenic acute regulatory (StAR) protein gene by the AP-1 family member c-Fos. Mol Cell Endocrinol (2002) 188:161–70. doi:10.1016/S0303-7207(01)00715-88
128. Lehoux JG, Fleury A, Ducharme L. The acute and chronic effects of adrenocorticotropin on the levels of messenger ribonucleic acid and protein of steroidogenic enzymes in rat adrenal in vivo. Endocrinology (1998) 139:3913–22. doi:10.1210/endo.139.9.6196
129. Manna PR, Chandrala SP, Jo Y, Stocco DM. cAMP-independent signaling regulates steroidogenesis in mouse Leydig cells in the absence of StAR phosphorylation. J Mol Endocrinol (2006) 37:81–95. doi:10.1677/jme.1.02065
130. Suske G. The Sp-family of transcription factors. Gene (1999) 238:291–300. doi:10.1016/S0378-1119(99)00357-1
131. Kadonaga JT, Jones KA, Tjian R. Promoter-specific activation of RNA polymerase II transcription by Sp1. Trends Biochem Sci (1986) 11:20–3. doi:10.1016/0968-0004(86)90226-4
132. Majello B, De Luca P, Suske G, Lania L. Differential transcriptional regulation of c-myc promoter through the same DNA binding sites targeted by Sp1-like proteins. Oncogene (1995) 10:1841–8.
133. Yu B, Datta PK, Bagchi S. Stability of the Sp3-DNA complex is promoter-specific: Sp3 efficiently competes with Sp1 for binding to promoters containing multiple Sp-sites. Nucleic Acids Res (2003) 102:5368–76. doi:10.1093/nar/gkg706
134. Giatzakis C, Papadopoulos V. Differential utilization of the promoter of peripheral-type benzodiazepine receptor by steroidogenic versus nonsteroidogenic cell lines and the role of Sp1 and Sp3 in the regulation of basal activity. Endocrinology (2004) 145:1113–23. doi:10.1210/en.2003-1330
135. Chou SJ, Lai KN, Chung BC. Characterization of the upstream sequence of the human CYP11A1 gene for cell type-specific expression. J Biol Chem (1996) 271:22125–9. doi:10.1074/jbc.271.36.22125
136. Ahlgren R, Suske G, Waterman MR, Lund J. Role of Sp1 in cAMP-dependent transcriptional regulation of the bovine CYP11A gene. J Biol Chem (1999) 274:19422–8. doi:10.1074/jbc.274.27.19422
137. Liu Z, Simpson ER. Molecular mechanism for cooperation between Sp1 and steroidogenic factor-1 (SF-1) to regulate bovine CYP11A gene expression. Mol Cell Endocrinol (1999) 153:183–96. doi:10.1016/S0303-7207(99)00036-2
138. Urban RJ, Bodenburg Y, Kurosky A, Wood TG, Gasic S. Polypyrimidine tract-binding protein-associated splicing factor is a negative regulator of transcriptional activity of the porcine P450scc insulin-like growth factor response element. Mol Endocrinol (2000) 14:774–82. doi:10.1210/mend.14.6.0485
139. Fluck CE, Miller WL. GATA-4 and GATA-6 modulate tissue-specific transcription of the human gene for P450c17 by direct interaction with Sp1. Mol Endocrinol (2004) 18:1144–57. doi:10.1210/me.2003-0342
140. Zanger UM, Kagawa N, Lund J, Waterman MR. Distinct biochemical mechanisms for cAMP-dependent transcription of CYP17 and CYP21. FASEB J (1992) 6:719–23.
141. Zanaria E, Muscatelli F, Bardoni B, Strom TM, Guioli S, Guo W, et al. An unusual member of the nuclear hormone receptor superfamily responsible for X-linked adrenal hypoplasia congenita. Nature (1994) 372:635–41. doi:10.1038/372635a0
142. Muscatelli F, Strom TM, Walker AP, Zanaria E, Récan D, Meindl A, et al. Mutations in the DAX-1 gene give rise to both X-linked adrenal hypoplasia congenita and hypogonadotropic hypogonadism. Nature (1994) 372:672–6. doi:10.1038/372672a0
143. Zazopoulos E, Lalli E, Stocco DM, Sassone-Corsi P. DNA binding and transcriptional repression by DAX-1 blocks steroidogenesis. Nature (1997) 390:311–5. doi:10.1038/36899
144. Ito M, Yu R, Jameson JL. DAX-1 inhibits SF-1-mediated transactivation via a carboxy-terminal domain that is deleted in adrenal hypoplasia congenita. Mol Cell Biol (1997) 17:1476–83. doi:10.1128/MCB.17.3.1476
145. Lalli E, Melner MH, Stocco DM, Sassone-Corsi P. DAX-1 blocks steroid production at multiple levels. Endocrinology (1998) 139:4237–43. doi:10.1210/en.139.10.4237
146. Lalli E. Role of orphan nuclear receptor DAX-1 in development, physiology and disease. Adv Biol (2014). doi:10.1155/2014/582749
147. Ragazzon B, Lefrançois-Martinez AM, Val P, Sahut-Barnola I, Tournaire C, Chambon C, et al. Adrenocorticotropin-dependent changes in SF-1/DAX-1 ratio influence steroidogenic genes expression in a novel model of glucocorticoid-producing adrenocortical cell lines derived from targeted tumorigenesis. Endocrinology (2006) 147:1805–18. doi:10.1210/en.2005-1279
148. Tamai KT, Monaco L, Alastalo TP, Lalli E, Parvinen M, Sassone-Corsi P. Hormonal and developmental regulation of DAX-1 expression in Sertoli cells. Mol Endocrinol (1996) 10:1561–9. doi:10.1210/me.10.12.1561
149. McKenna NJ, O’Malley BW. Nuclear receptor coregulators. Pure Appl Chem (2003) 75:1665–9. doi:10.1351/pac200375111665
150. McKenna NJ, Xu J, Nawaz Z, Tsai SY, Tsai M-J, O’Malley BW. Nuclear receptor coactivators: multiple enzymes, multiple complexes, multiple functions. J Steroid Biochem Mol Biol (1999) 69:3–12. doi:10.1016/S0960-0760(98)00144-7
151. Lonard DM, O’Malley BW. Expanding functional diversity of the coactivators. Trends Biochem Sci (2005) 30:126–32. doi:10.1016/j.tibs.2005.01.001
152. Spencer TE, Jenster G, Burcin MM, Allis CD, Zhou J, Mizzen CA, et al. Steroid receptor coactivator-1 is a histone acetyltransferase. Nature (1997) 389:194–8. doi:10.1038/38304
153. Chen H, Lin RJ, Schiltz RL, Chakravarti D, Nash A, Nagy L, et al. Nuclear receptor coactivator ACTR is a novel histone acetyltransferase and forms a multimeric activation complex with P/CAF and CBP/p300. Cell (1997) 90:569–80. doi:10.1016/S0092-8674(00)80516-4
154. Vo N, Goodman RH. CREB-binding protein and p300 in transcriptional regulation. J Biol Chem (2001) 276:13505–8. doi:10.1074/jbc.R000025200
155. Chen J, Kinyamu K, Archer TK. Changes in attitude, changes in latitude: nuclear receptors remodeling chromatin to regulate transcription. Mol Endocrinol (2006) 20:1–13. doi:10.1210/me.2005-0192
156. Chen H, Lin R, Xie W, Wilpitz D, Evans R. Regulation of hormone-induced histone hyperacetylation and gene activation via acetylation of an acetylase. Cell (1999) 98:675–86. doi:10.1016/S0092-8674(00)80054-9
157. Chen WY, Juan LJ, Chung BC. SF-1 (nuclear receptor 5A1) activity is activated by cyclic AMP via p300-mediated recruitment to active foci, acetylation, and increased DNA binding. Mol Cell Biol (2005) 25:10442–53. doi:10.1128/MCB.25.23.10442-10453.2005
158. An W, Kim J, Roeder RG. Ordered cooperative functions of PRMT1, p300, and CARM1 in transcriptional activation by p53. Cell (2004) 117:735–48. doi:10.1016/j.cell.2004.05.009
159. Perissi V, Rosenfeld MG. Controlling nuclear receptors: the circular logic of cofactor cycles. Nat Rev Mol Cell Biol (2005) 6:542–54. doi:10.1038/nrm1682
160. Bannister AJ, Kouzarides T. The CBP co-activator is a histone acetyltransferase. Nature (1996) 384:641–3. doi:10.1038/384641a0
161. Fronsdal K, Engedal N, Slagsvold T, Saatcioglu F. CREB binding protein is a coactivator for the androgen receptor and mediates cross-talk with AP-1. J Biol Chem (1998) 273:31853–9. doi:10.1074/jbc.273.48.31853
162. Kovacs KA, Steinmann M, Magistretti PJ, Halfon O, Cardinaux JR. CCAAT/enhancer-binding protein family members recruit the coactivator CREBbinding protein and trigger its phosphorylation. J Biol Chem (2003) 278:36959–65. doi:10.1074/jbc.M303147200
163. Gizard F, Lavallee B, DeWitte F, Hum DW. A novel zinc finger protein TReP-132 interacts with CBP/p300 to regulate human CYP11A1 gene expression. J Biol Chem (2001) 276:33881–92. doi:10.1074/jbc.M100113200
164. Shibata H, Ando T, Kurihara I, Suzuki T, Lund J, Morohashi K, et al. Functional role of COUP-TF1, SF-1, and nuclear receptor coregulators in the steroidogenesis of adrenocortical adenomas. In: Nawata H, editor. Molecular Steroidogenesis. Okamoto MIY. Tokyo: J Universal Academy Press (2000). p. 345–8.
165. Dammer EB, Leon A, Sewer MB. Coregulator exchange and sphingosine-sensitive cooperativity of steroidogenic factor-1, general control nonderepressed 5, p54, and p160 coactivators regulate cyclic adenosine 3′,5′-monophosphate-dependent cytochrome P450c17 transcription rate. Mol Endocrinol (2007) 21:415–38. doi:10.1210/me.2006-0361
166. Lucki N, Sewer M. The cAMP-responsive element binding protein (CREB) regulates the expression of acid ceramidase (ASAH1) in H295R human adrenocortical cells. Biochim Biophys Acta (2009) 1791:706–13. doi:10.1016/j.bbalip.2009.03.005
167. Sayed SB, Jones PM, Persaud SJ, Whitehouse BJ. Effects of phosphoprotein phosphatase inhibitors on steroidogenesis and StAR protein expression in Y1 cells. Endocr Res (1998) 24:413–4. doi:10.3109/07435809809032624
168. Paz C, Cornejo MacIel F, Mendez C, Podesta EJ. Corticotropin increases protein tyrosine phosphatase activity by a cAMP-dependent mechanism in rat adrenal gland. Eur J Biochem (1999) 265:911–8. doi:10.1046/j.1432-1327.1999.00759.x
169. Poderoso C, Cornejo Maciel F, Gorostizaga A, Bey P, Paz C, Podestá EJ. The obligatory action of protein tyrosine phosphatases in ACTH-stimulated steroidogenesis is exerted at the level of StAR protein. Endocr Res (2002) 28:413–7. doi:10.1081/ERC-120016816
170. Castillo F, Cano F, Maloberti P, Castilla R, Neuman I, Poderoso C, et al. Tyrosine phosphates act on steroidogenesis through the activation of arachidonic acid release. Endocr Res (2004) 30:623–7. doi:10.1081/ERC-200043795
171. Gorostizaga A, Cornejo Maciel F, Brion L, Maloberti P, Podestá EJ, Paz C. Tyrosine phosphatases in steroidogenic cells: regulation and function. Mol Cell Endocrinol (2007) 26(5–266):131–7. doi:10.1016/j.mce.2006.12.009
172. Cooke M, Mele P, Maloberti P, Duarte A, Poderoso C, Orlando U, et al. Tyrosine phosphatases as key regulators of StAR induction and cholesterol transport: SHP2 as a potential tyrosine phosphatase involved in steroid synthesis. Mol Cell Endocrinol (2011) 336:63–9. doi:10.1016/j.mce.2010.11.030
173. Maloberti P, Castilla R, Castillo F, Cornejo Maciel F, Mendez CF, Paz C, et al. Silencing the expression of mitochondrial acyl-CoA thioesterase I and acyl-CoA synthetase 4 inhibits hormone-induced steroidogenesis. FEBS J (2005) 272:1804–14. doi:10.1111/j.1742-4658.2005.04616.x
174. Mele PG, Dada LA, Paz C, Cymeryng CB, Cornejo Maciel MF, Neuman MI, et al. Site of action of proteinases in the activation of steroidogenesis in rat adrenal gland. Biochim Biophys Acta (1996) 29:260–8. doi:10.1016/0167-4889(95)00177-8
175. Wang XJ, Dyson MT, Jo Y, Eubank DW, Stocco DM. Involvement of 5-lipoxygenase metabolites of arachidonic acid in cyclic AMP-stimulated steroidogenesis and steroidogenic acute regulatory protein gene expression. J Steroid Biochem Mol Biol (2003) 85:159–66. doi:10.1016/S0960-0760(03)00189-4
176. Cornejo Maciel F, Maloberti P, Neuman I, Cano F, Castilla R, Castillo F, et al. An arachidonic acid-preferring acyl-CoA synthetase is a hormone-dependent and obligatory protein in the signal transduction pathway of steroidogenic hormones. J Mol Endocrinol (2005) 3:655–66. doi:10.1677/jme.1.01691
177. Cano F, Poderoso C, Cornejo Maciel F, Castilla R, Maloberti P, Castillo F, et al. Protein tyrosine phosphatases regulate arachidonic acid release, StAR induction and steroidogenesis acting on a hormone-dependent arachidonic acid-preferring acyl-CoA synthetase. J Steroid Biochem Mol Biol (2006) 99:197–202. doi:10.1016/j.jsbmb.2006.01.003
178. Sewer MB, Waterman MR. Adrenocorticotropin/cyclic adenosine 3′,5′-monophosphate-mediated transcription of the human CYP17 gene in the adrenal cortex is dependent on phosphatase activity. Endocrinology (2002) 143:1769–77. doi:10.1210/endo.143.5.8820
179. Sewer MB, Waterman MR. cAMP-dependent transcription of steroidogenic genes in the human adrenal cortex requires a dual-specificity phosphatase in addition to protein kinase A. J Mol Endocrinol (2002) 29:163–74. doi:10.1677/jme.0.0290163
Keywords: adrenal cortex, steroidogenesis, cAMP, transcription factors, gene regulation
Citation: Ruggiero C and Lalli E (2016) Impact of ACTH Signaling on Transcriptional Regulation of Steroidogenic Genes. Front. Endocrinol. 7:24. doi: 10.3389/fendo.2016.00024
Received: 23 February 2016; Accepted: 14 March 2016;
Published: 29 March 2016
Edited by:
Antoine Martinez, Centre National de la Recherche Scientifique, FranceReviewed by:
Mario Perello, National Scientific and Technical Research Council, ArgentinaKazuhiro Takahashi, Tohoku University Graduate School of Medicine, Japan
Copyright: © 2016 Ruggiero and Lalli. This is an open-access article distributed under the terms of the Creative Commons Attribution License (CC BY). The use, distribution or reproduction in other forums is permitted, provided the original author(s) or licensor are credited and that the original publication in this journal is cited, in accordance with accepted academic practice. No use, distribution or reproduction is permitted which does not comply with these terms.
*Correspondence: Carmen Ruggiero, Y3J1Z2dpZXJvQGlwbWMuY25ycy5mcg==;
Enzo Lalli, bmluaW5vQGlwbWMuY25ycy5mcg==