- 1UMR8104, CNRS, Institut Cochin, Université Paris Descartes, Paris, France
- 2U1016, INSERM, Paris, France
O-GlcNAcylation is a reversible post-translational modification that regulates the activities of cytosolic and nuclear proteins according to glucose availability. This modification appears to participate in several hyperglycemia-associated complications. An important feature of metabolic diseases such as diabetes and obesity is the presence of a low-grade chronic inflammation that causes numerous complications. Hyperglycemia associated with the metabolic syndrome is known to promote inflammatory processes through different mechanisms including oxidative stress and abnormally elevated protein O-GlcNAcylation. However, the role of O-GlcNAcylation on inflammation remains contradictory. O-GlcNAcylation associated with hyperglycemia has been shown to increase nuclear factor κB (NFκB) transcriptional activity through different mechanisms. This could contribute in inflammation-associated diabetic complications. However, in other conditions such as acute vascular injury, O-linked N-acetyl glucosamine (O-GlcNAc) also exerts anti-inflammatory effects via inhibition of the NFκB pathway, suggesting a complex regulation of inflammation by O-GlcNAc. Moreover, whereas macrophages and monocytes exposed to high glucose for a long-term period developed a pro-inflammatory phenotype, the impact of O-GlcNAcylation in these cells remains unclear. A future challenge will be to clearly establish the role of O-GlcNAcylation in pro- and anti-inflammatory functions in macrophages.
Introduction
In the last decades, changes in lifestyle, including excessive energy intake and consumption of food enriched in saturated fat, combined with the lack of physical activity, have led to a dramatic increased prevalence of pathologies such as diabetes, obesity, and atherosclerosis. These pathologies are part of the metabolic syndrome, which constitutes one of the major threats to global health.
It is now well established that these metabolic diseases are associated with a low-grade chronic inflammation (1) that causes complications such as nephropathy, neuropathy, retinopathy, and atherosclerosis, and contributes to morbidity and mortality associated with the metabolic syndrome. This low-grade inflammation is characterized by an abnormal cytokine production. Thus, it has been demonstrated that the adipose tissue of obese individuals produce higher levels of the pro-inflammatory cytokine tumor-necrosis factor α (TNFα) and other pro-inflammatory factors such as interleukin (IL) 6 (1). The excessive amount of nutritional lipids might have a role not only in the pathogenesis of obesity-associated insulin resistance but also in the chronic inflammation associated with this condition. Indeed, free fatty acids can activate the lipopolysaccharide (LPS) receptor toll-like receptor (TLR) 4 and induce the production of pro-inflammatory cytokines by macrophages (2). Not only lipids but also high-glucose concentrations are involved in inflammatory processes (3, 4). High glycemic index diets appeared to play a key role in the establishment and persistence of inflammation (5–7). In contrast, a 4 weeks food restriction in obese patients was sufficient to significantly reduce oxidative stress (8).
It is well documented that hyperglycemia associated with the metabolic syndrome promotes abnormally elevated protein O-GlcNAcylation, which participates in the glucotoxicity phenomenon (9). O-GlcNAcylation is a reversible post-translational modification consisting in the addition of N-acetylglucosamine to serine or threonine on cytosolic and nuclear proteins (Figure 1). Only two enzymes, O-GlcNAc transferase (OGT) and O-GlcNAcase (OGA), control the level of O-linked N-acetyl glucosamine (O-GlcNAc) on proteins. OGT uses UDP-GlcNAc, produced through the hexosamine biosynthetic pathway (HBP) to O-GlcNAcylate proteins, whereas OGA removes O-GlcNAc from proteins. Thus, according to glucose availability and its flux through the HBP, O-GlcNAcylation modulates protein functions by regulating their sub-cellular localization, stability, interaction with protein partners, or activity. More than 1000 proteins have now been identified as target of this modification, including transcription factors (10–17) and signaling molecules (9, 18–22) involved in glucose and lipid metabolism, insulin resistance, and inflammation. In addition to glucose, the O-GlcNAc also includes amine and acetyl moieties, and therefore also integrates amino-acids (glutamine) and fatty acid (AcetylCoA) metabolisms, suggesting that the availability of other nutrients may also be sensed by this pathway. Thus, infusion of a lipid emulsion in rats induced a twofold increase in UDP-GlcNAc content in skeletal muscle, associated with insulin resistance. Moreover, fatty acids can directly regulate the expression of glutamine:fructose-6-phosphate amidotransferase (GFAT) (23) and other enzymes of the HBP pathway (24) in muscle and pancreatic β-cell. Therefore, increased nutrients, and particularly increased blood glucose and fatty acids levels associated with excess food intake, obesity, and/or diabetes, are likely to impact numerous cellular processes, including those involved in inflammation, through protein O-GlcNAcylation.
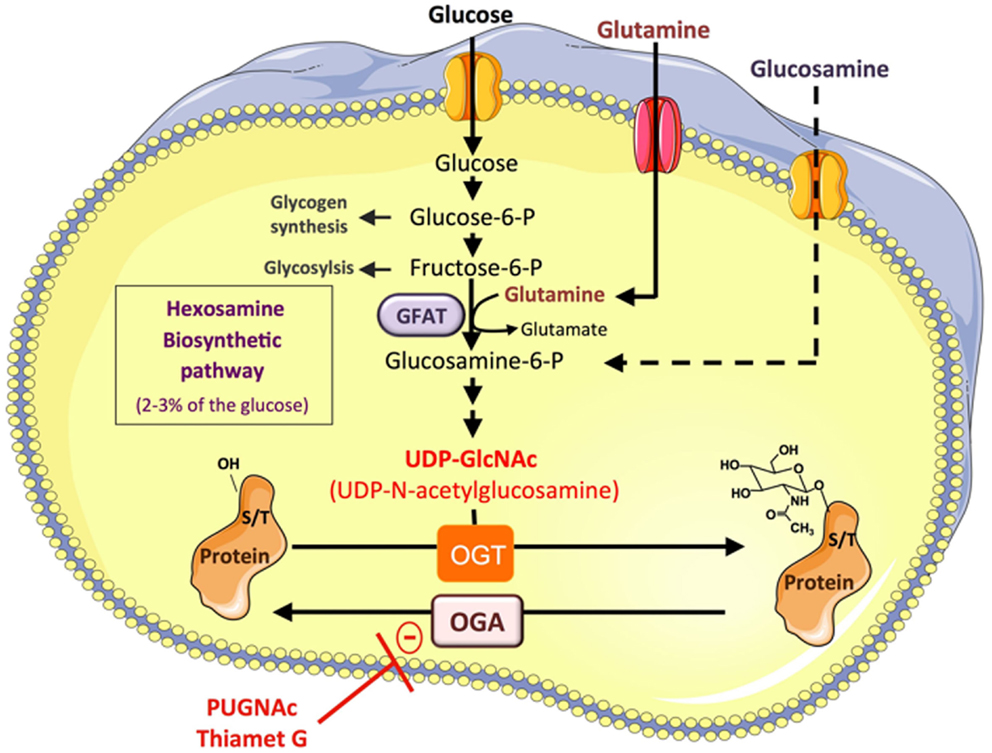
Figure 1. Protein O-GlcNAcylation depends on the flux of glucose through the hexosamine biosynthesis pathway. A small fraction of the glucose entering the cell feeds the hexosamine biosynthetic pathway (HBP) to produce UDP-GlcNAc, the substrate used by O-GlcNAc-transferase (OGT) to add N-acetyl glucosamine on serine or threonine residues of cytosolic or nuclear proteins. This dynamic and reversible post-translational modification controls the activity, the localization, or the stability of proteins according to glucose availability. Glucose enters the HBP as fructose-6-phosphate. The latter is converted to glucosamine-6-phosphate by the glutamine:fructose-6-phosphate amidotransferase (GFAT), the rate limiting enzyme of the pathway. After a subset of reactions, UDP-N-acetylglucosamine (UDP-GlcNAc) is generated and used by OGT to add GlcNAc on serine or threonine residues of target proteins. The O-GlcNAc moiety is removed from O-GlcNAc-modified proteins by the O-GlcNAcase (OGA). Experimentally, O-GlcNAcylation of proteins can be increased by incubating the cells with high concentrations of glucose, or with glucosamine, which bypass the rate limiting step catalyzed by GFAT. Inhibitors of OGA such as O-[2-acetamido-2-deoxy-d-glucopyranosylidene] amino-N-phenylcarbamate (PUGNAc) or (3aR,5R,6S,7R,7aR)-2-(ethylamino)-3a,6,7,7a-tetrahydro-5-(hydroxymethyl)-5H-Pyrano[3,2-d]thiazole-6,7-diol (Thiamet-G) can also be used to increase the O-GlcNAc level on proteins.
O-GlcNAcylation, Diabetic Complications, and Inflammatory Processes
A number of experimental data have suggested the involvement of the HBP in pathological manifestations of the metabolic syndrome, such as diabetic associated-kidney disease. Indeed, one-third of diabetic patients will develop diabetic nephropathy, a chronic microvascular complication leading to a progressive decline in renal function, decreased glomerular filtration rate and proteinuria. Clinical trials have demonstrated that high glucose is central to the pathogenesis of diabetic nephropathy (25), and the beneficial effect of glycemia correction on renal complications has been demonstrated (26). Mesangial cells are smooth muscle-like pericytes that surround the filtration capillaries within glomerulus (27). In these cells, glucose flux, through the HBP pathway, regulates the expression of pro-fibrotic factors such as transforming growth factor β1 (TGFβ1) and plasminogen activator inhibitor 1 (PAI-1), and extracellular matrix components (28, 29), at least in part via the O-GlcNAcylation of transcription factors such as Sp1 (11, 30). In mesangial cells, the HBP pathway also regulates the expression of pro-inflammatory factors such as vascular cell adhesion molecule-1 (VCAM-1), IL6, and TNFα, through the nuclear factor κB (NFκB) pathway (31). Abnormal activation of the NFκB pathway is certainly a major contributor in inflammation-associated diabetic complications. In vascular smooth muscle cells, high-glucose conditions resulted in NFκB activation (32). Peripheral blood mononuclear cells isolated from patients with diabetic nephropathy showed an increased activation of NFκB that could be corrected by anti-oxidant treatment (33, 34). Glucose oxidative stress is obviously central to glucotoxicity in diabetic conditions (35), and a link between hyperglycemia, oxidative stress, and O-GlcNAcylation has been proposed, reinforcing the potential involvement of O-GlcNAcylation in inflammation (36, 37). Therefore, exploring the potential regulation of NFκB activity by O-GlcNAcylation in different settings is of paramount importance.
O-GlcNAcylation and the NFκB Pathway
The transcription factor NFκB is involved in a large number of cell functions including apoptosis, cell survival, and differentiation, and is critical to immune response and inflammation. NFκB family comprises five proteins, p65 (RelA), RelB, c-Rel, p105/p50 (NFκB1), and p100/52 (NFκB2) that associate to form distinct homo and hetero-dimeric complexes (38–40). In non-stimulated cells, NFκB is inactive and is retained in the cytoplasm by the inhibitor of κB (IκB) (Figure 2). Upon stimulation by pro-inflammatory cytokines, LPS, or growth factors, IκB is phosphorylated by the IκB kinase (IKK). This phosphorylation leads to IκB ubiquitination and proteosomal degradation. Free NFκB can then translocate into the nucleus to activate its target genes (38–40).
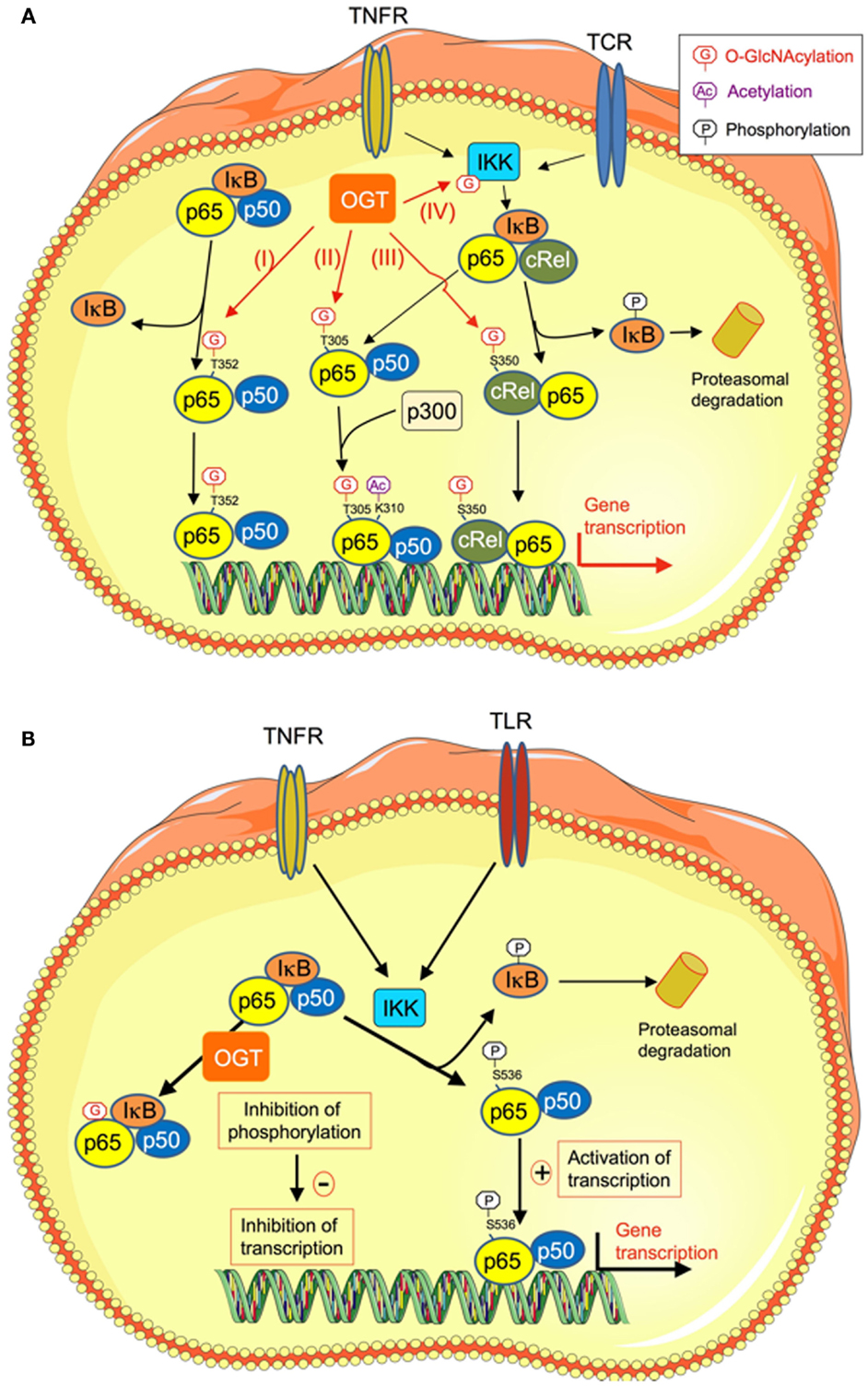
Figure 2. O-GlcNAcylation regulates NFκB transcriptional activity through different mechanisms. (A) O-GlcNAcylation stimulates NFκB transcriptional activity. High-glucose conditions are known to promote inflammatory processes through different mechanisms, including increased O-GlcNAcylation of NFκB. Several mechanisms have been described that could account for increased transcriptional activity of this factor upon O-GlcNAcylation. (I) O-GlcNAcylation of p65/RelA on T352 decreases its affinity for IκB, resulting in increased in its nuclear localization and transcription of its target genes (41). (II) O-GlcNAcylation of T305 on RelA promotes NFκB transcriptional activity by potentiating its p300-dependent acetylation on K310 (42). (III) O-GlcNAcylation of c-Rel on S350. This modification increases c-Rel DNA binding and transcriptional activity. (IV) O-GlcNAcylation of the β-subunit of IKK on Ser733 stimulates its activity, resulting in increased phosphorylation and degradation of IκB, and thereby increased NFκB activity. (B) O-GlcNAcylation inhibits NFκB transcriptional activity. O-GlcNAcylation-inducing treatments appear to have anti-inflammatory and vaso-protective effects during acute vascular injury. In rat aortic smooth muscle cells, O-GlcNAcylation of NFκB specifically inhibits its phosphorylation on Ser 536, while leaving other phosphorylation sites unaffected. This results in increased NFκB binding to IkB, inhibition of TNFα-induced NFκB DNA binding, and reduction of expression of genes coding for inflammatory mediators (TNFR, TNFα receptor; TCR, T-cell receptor; IKK, Iκ kinase).
Nuclear factor κB activation has been implicated in the metabolic syndrome and in diabetes pathogenesis (43–46). Because NFκB is mainly regulated by post-transcriptional modifications (with an important role of phosphorylation and acetylation), and because high glucose is known to activate NFκB and stimulate its target genes, different studies focused on the potential role of O-GlcNAc on NFκB activation.
O-GlcNAcylation as a Positive Regulator of NFκB Activity
In the first study addressing this question, mesanglial cells treated with glucosamine or high-glucose exhibited an increased nuclear protein binding to NFκB consensus sequences in an electromobilty shift assay, correlated with O-GlcNAcylation of p65 (31). This observation suggested that NFκB O-GlcNAcylation could play a part in inflammatory processes. However, in that first study, the O-GlcNAc modification sites on NFκB had not been identified and the mechanism by which O-GlcNAc modification led to NFκB activation remained unclear (31).
It now clearly appears that different mechanisms, acting at various cellular levels, are involved in the effects of O-GlcNAcylation on activation of NFκB signaling. First, O-GlcNAcylation can regulate the interaction between NFκB and its inhibitor IκB. In porcine vascular smooth muscle cells, it has been demonstrated that down-regulation of O-GlcNAcylation mediated by OGA over-expression inhibits hyperglycemia-induced NFκB activation. In contrast, an increase in O-GlcNAcylation mediated by OGT over-expression increases NFκB activity (41). These effects were due to an increase in O-GlcNAcylation of RelA on T352 that decreases its affinity for IκB, leading to an increased nuclear translocation of RelA [Figure 2A (I)]. This could contribute to the sustained activation of NFκB that is associated with diabetes (41). Another study indicated that O-GlcNAcylation increases NFκB transcriptional activity by promoting its acetylation (42). Indeed, chromatin immunoprecipitation assays demonstrated that, upon induction with TNFα, OGT localizes to NFκB-regulated promoters. OGT siRNA experiments showed that OGT protein was required for NFκB-dependent transcription. The mechanism involved was the attachment of O-GlcNAc moiety to T305 on RelA that promoted NFκB transcription by potentiating p300-dependent acetylation on K310 [Figure 2A (II)] (42).
The O-GlcNAcylation of NFκB also appears to play an important role in the immunity and the production of pro-inflammatory cytokines by T lymphocytes. Golks et al. first showed that OGT was necessary for activation of T lymphocytes by the T-cell receptor (TCR), inducing O-GlcNAcylation of p65 and stimulation of NFκB-dependent transcription (47). More recently, it was reported that in these cells, the c-Rel subunit of NFκB was modified by O-GlcNAcylation on Ser 350 [Figure 2A (III)]. This modification increased c-Rel transcriptional activity and was necessary for c-Rel mediated expression of IL2, IFNG, and CSF2 in response to TCR activation (48). Importantly c-Rel O-GlcNAcylation was not required for TNFα- or TCR-induced expression of other NFκB target genes, such as NFKBIA (which encodes IκBα) and TNFAIP3 (which encodes A20), indicating a gene specific requirement of c-Rel O-GlcNAcylation (48). These results suggest that during chronic hyperglycemia, an increase in c-Rel O-GlcNAcylation could contribute to type-1 diabetes progression by enhancing the production of Th1 pro-inflammatory cytokines, leading to pancreatic β cells destruction (48, 49). Finally, O-GlcNAcylation of IKK [Figure 2A (IV)] has also been demonstrated, resulting in an increase in its kinase activity, leading to subsequent increase in phosphorylation, and degradation of IκB and stimulation of NFκB activity in cancer cells (50). Whether this mechanism is also operative in the context of hyperglycemia-induced inflammation remains to be evaluated.
O-GlcNAcylation as a Negative Regulator of NFκB Activity
Whereas O-GlcNAcylation is generally found associated with an increased in NFκB activity in diabetic conditions, in some situations, O-GlcNAc appears, however, to reduce its pro-inflammatory activity (51–53). Thus, in a rat model of trauma-hemorrhage followed by fluid resuscitation, increased O-GlcNAcylation induced by glucosamine or PUGNAc significantly improved cardiac function and peripheral organ perfusion, and decreased the circulating levels of pro-inflammatory cytokines TNFα and IL6 (51, 52). These authors observed that increased O-GlcNAcylation reduces IκB phosphorylation and NFκB signaling in cardiac tissue from trauma-hemorrhage treated rats. Moreover, O-GlcNAcylation-inducing treatments appear to have anti-inflammatory and vaso-protective effects during acute vascular injury (54, 55). Indeed, Xing et al. showed that in rat aortic smooth muscle cells, O-GlcNAcylation of p65 NFκB upon PUGNAc or glucosamine treatment was accompanied by a reduction in TNFα-induced phosphorylation on serine 536, resulting in increased association of NFκB with IκB, decreased NFκB activity and inhibition of the production of pro-inflammatory mediators (Figure 2B) (53).
It therefore appears that, depending on the cellular context and type of insult (chronic hyperglycemia versus acute vascular injury), O-GlcNAcylation may have different effects on the NFκB pathway, resulting in either pro- or anti-inflammatory outcomes.
O-GlcNAcylation and Macrophage Activity
Monocytes and macrophages play central roles in acute and chronic inflammatory processes. As mentioned previously, insulin resistance, obesity, and diabetes are associated with recruitment of pro-inflammatory monocytes/macrophages in different organs, including adipose tissue, liver, pancreas, as well as blood vessels wall (56–62). Numerous studies have shown that macrophages/monocytes submitted to long-term exposure to high-glucose concentrations developed a pro-inflammatory phenotype. Indeed, in human monocytic cells THP1, high glucose (15 mmol/L) for 72 h increased gene expression of the pro-inflammatory factors monocyte chemotactic protein 1 (MCP1), IL1β, and TNFα. Of interest, in this study, the NFκB activation played an important role in the high glucose-induced MCP1 transcription (63). In THP1 cells, exposure to high glucose also increased the RNA and protein levels of TLR2 and TLR4, which play key roles in innate immune response and inflammation. TLR2 and TLR4 activate MyD88 dependant signaling and induce NFκB transactivation, leading to the production of pro-inflammatory cytokines. These up-regulations of TLR2 and TLR4 under high-glucose condition seemed at least in part mediated by protein kinase C (PKC) (64). In RAW 264.7, a murine macrophages cell line, high-glucose alone did not induce inflammatory mediator expression but increased inducible nitric oxide synthase (iNOS) expression and nitric oxide (NO) production in response to LPS. This effect appeared to be mediated by NFκB activation (65). High-glucose also increased IL1β secretion from LPS activated macrophages, a risk factor in diabetes that contributes to pancreatic β-cell damage (66). This effect appeared to involve activation of ERK1/2, JNK1/2, and PKCα and δ in macrophages cultured in high-glucose conditions (65).
In vivo hyperglycemia also affects the inflammatory profile of macrophages. An increased pro-inflammatory profile was observed in peritoneal macrophages from mice two weeks after diabetes induction with alloxan or streptozocin (67, 68). However, peritoneal macrophages from mice with 4 months streptozotocin-induced diabetes displayed complex modification of the pro-inflammatory profile, with increased NO production but decreased TNFα and IL6 in response to LPS stimulation (69). Another study showed impaired inflammatory response to multiple TLR ligands in alveolar macrophages from 2 weeks streptozotocin-induced diabetic mice (70). Therefore, in vivo hyperglycemia may have complex effects on macrophages functions, depending on their tissue of origin and on the duration of the diabetes.
High-glucose concentrations may affect macrophages functions through numerous mechanisms, including oxidative stress, activation of PKC, and/or MAP kinases, advanced glycation end products, as well as protein O-GlcNAcylation. Only a few studies evaluated the role of O-GlcNAcylation in macrophages functions, and contradictory results were obtained.
In the human monocyte THP1 cell line, high-glucose concentrations and PUGNAc increased the expression and the secretion of macrophage inflammatory protein MIP1α and β through OGT dependent epigenetic mechanisms (71).
On the other hand, glucosamine exerted neuroprotective effects via suppression of post-ischemic microglia inflammation in rat brain after ischemia/reperfusion injury (72). Accordingly, in cultured mouse BV2 microglial cells and RAW264.7 macrophages, Hwang et al. observed that glucosamine suppressed LPS-induced up regulation of pro-inflammatory molecules by inhibiting NFκB activation by LPS. Glucosamine, which bypass the rate limiting step of the HBP, is often used to increase O-GlcNAcylation in cells. Unexpectedly, in this study, glucosamine induced a decrease in NFκB O-GlcNAcylation. This counter-intuitive result was explained by an inhibitory effect of glucosamine on an LPS-induced interaction between OGT and NFκB (72). More recently, the same group obtained similar results with cRel in BV2 microglial cells, showing glucosamine inhibition of LPS-induced cRel-OGT interaction, associated with decreased O-GlcNAcylation of c-Rel and subsequent inhibition of its transcriptional activity (73). However, the mechanism by which glucosamine may interfere with the LPS pathway and affect OGT-NFκB interaction was not elucidated. For instance, the specific effect of increasing O-GlcNAcylation levels using PUGNAc or Thiamet-G was not evaluated in theses studies. Glucosamine, by increasing UDP-GlcNAc in the cell, may also affect complex glycosylations of proteins. Thus, it is possible that glucosamine effects were mediated by modification of N-linked glycosylation of receptors and/or secreted proteins, as suggested previously in a study using macrophage cell lines (74). Moreover, depending on the experimental setting, glucosamine may also induce ATP depletion (75) or promote oxidative stress (76). Therefore, glycosylation-independent effects might also play a role in the paradoxical effect of glucosamine on NFκB O-GlcNAcylation state. Further confusion was provided by an additional study by Hwang et al. (77), which showed that over-expression of OGT unexpectedly reduced the transcriptional activity of NFκB both in the absence and presence of glucosamine, resulting in inhibition of LPS-mediated expression of the NFκB target gene iNOS.
Innate immune signaling initiated by interaction of pathogen ligands with TLRs induces iNOS expression, and, subsequently, the production of NO, which not only plays a role as a bactericidal agent but also act as an intracellular mediator. Indeed, S-nitrosylation of cysteine thiols regulates protein activities in NO-generating cells. Complex interactions between NO signaling and O-GlcNAcylation pathway have been suggested. Thus, in RAW264.7 cells and in mice peritoneal macrophages, Ryu et al. observed that LPS treatment induces increased global S-Nitrosylation of proteins, concomitant with a paradoxical denitrosylation of S-nitrosylated OGT (78). Denitrosylation of OGT was associated with an increase in its catalytic activity, suggesting a potential mechanism for LPS-induced O-GlcNAcylation of p65 and subsequent production of pro-inflammatory cytokines (78). On the other hand, in N9 microglia cells, Zheng et al. observed that LPS induced a (modest) reduction in global O-GlcNAcylation of proteins, associated with a reduction in OGT protein level (79). Clearly, additional work will be needed in order to untangle the complex relationships between OGT and p65 and their potential regulation by LPS, glucosamine, and S-nitrosylation signaling pathways, and to firmly establish their relative role in pro- and anti-inflammatory functions in macrophages.
Conclusion
Whereas the implication of hyperglycemia in metabolic syndrome-associated inflammation is now well established, the involvement of O-GlcNAcylation appears complex, with both pro- and anti-inflammatory effects associated with this modification, depending on the type and duration (acute versus chronic) of the insult (80). In agreement with a dual effect of O-GlcNAc on inflammation, O-GlcNAcylation of NFκB, through an array of different mechanisms, can have both positive and negative effects on its activity depending on pathophysiological models and cell types (31, 41, 42, 47, 48, 51, 52, 81).
Recent data suggested that O-GlcNAcylation in the immune system may participate in the pathogenesis of both type-1 and type-2 diabetes (48, 49). Interestingly, O-GlcNAcylation was discovered 30 years ago in immune cells (82), and dynamic changes in O-GlcNAc levels upon lymphocyte activation were detected as early as the beginning of the nineties (83). However, only a limited amount of studies have investigated the function and regulation of this modification in immune cells, and very few works concern macrophages biology. This is indeed an emerging field, with many deficiencies in the existing knowledge. Several important points should be addressed in the future. Thus, the role of OGT and O-GlcNAcylation on macrophage functions (phagocytosis, ROS production in the phagosome, cytokine expression and secretion, M1 versus M2 polarization, etc.) should be thoroughly investigated. Ideally, these studies should be performed using primary cultured macrophages rather than in cell lines. In addition, the consequences of in vivo chronic hyperglycemia on protein O-GlcNAcylation in macrophages should also be evaluated. In this context, the development of macrophages specific OGT or OGA knock-out mice should provide important clues on the role of this modification in hyperglycemia-induced inflammation. Therefore, a large continent in the O-GlcNAc world remains to be explored.
Conflict of Interest Statement
The authors declare that the research was conducted in the absence of any commercial or financial relationships that could be construed as a potential conflict of interest.
Acknowledgments
Léa Baudoin holds a Ph.D. fellowship from the CORDDIM-Ile de France. Our work is performed within the Département Hospitalo-Universitaire (DHU) AUToimmune and HORmonal diseaseS and is supported by a grant from the Société Francophone du Diabète-Antadir (2013).
References
1. Hotamisligil GS. Inflammation and metabolic disorders. Nature (2006) 444(7121):860–7. doi:10.1038/nature05485
2. Shi H, Kokoeva MV, Inouye K, Tzameli I, Yin H, Flier JS. TLR4 links innate immunity and fatty acid-induced insulin resistance. J Clin Invest (2006) 116(11):3015–25. doi:10.1172/JCI28898
Pubmed Abstract | Pubmed Full Text | CrossRef Full Text | Google Scholar
3. Esposito K, Nappo F, Marfella R, Giugliano G, Giugliano F, Ciotola M, et al. Inflammatory cytokine concentrations are acutely increased by hyperglycemia in humans: role of oxidative stress. Circulation (2002) 106(16):2067–72. doi:10.1161/01.CIR.0000034509.14906.AE
Pubmed Abstract | Pubmed Full Text | CrossRef Full Text | Google Scholar
4. Mohanty P, Hamouda W, Garg R, Aljada A, Ghanim H, Dandona P. Glucose challenge stimulates reactive oxygen species (ROS) generation by leucocytes. J Clin Endocrinol Metab (2000) 85(8):2970–3. doi:10.1210/jcem.85.8.6854
Pubmed Abstract | Pubmed Full Text | CrossRef Full Text | Google Scholar
5. Liu S, Manson JE, Buring JE, Stampfer MJ, Willett WC, Ridker PM. Relation between a diet with a high glycemic load and plasma concentrations of high-sensitivity C-reactive protein in middle-aged women. Am J Clin Nutr (2002) 75(3):492–8.
6. Dickinson S, Hancock DP, Petocz P, Ceriello A, Brand-Miller J. High-glycemic index carbohydrate increases nuclear factor-kappaB activation in mononuclear cells of young, lean healthy subjects. Am J Clin Nutr (2008) 87(5):1188–93.
7. de Carvalho Vidigal F, Guedes Cocate P, Goncalves Pereira L, de Cassia Goncalves Alfenas R. The role of hyperglycemia in the induction of oxidative stress and inflammatory process. Nutr Hosp (2012) 27(5):1391–8. doi:10.3305/nh.2012.27.5.5917
Pubmed Abstract | Pubmed Full Text | CrossRef Full Text | Google Scholar
8. Dandona P, Mohanty P, Ghanim H, Aljada A, Browne R, Hamouda W, et al. The suppressive effect of dietary restriction and weight loss in the obese on the generation of reactive oxygen species by leukocytes, lipid peroxidation, and protein carbonylation. J Clin Endocrinol Metab (2001) 86(1):355–62. doi:10.1210/jcem.86.1.7150
Pubmed Abstract | Pubmed Full Text | CrossRef Full Text | Google Scholar
9. Issad T, Masson E, Pagesy P. O-GlcNAc modification, insulin signaling and diabetic complications. Diabetes Metab (2010) 36(6 Pt 1):423–35. doi:10.1016/j.diabet.2010.09.001
Pubmed Abstract | Pubmed Full Text | CrossRef Full Text | Google Scholar
10. Gao Y, Miyazaki J, Hart GW. The transcription factor PDX-1 is post-translationally modified by O-linked N-acetylglucosamine and this modification is correlated with its DNA binding activity and insulin secretion in min6 beta-cells. Arch Biochem Biophys (2003) 415(2):155–63. doi:10.1016/S0003-9861(03)00234-0
Pubmed Abstract | Pubmed Full Text | CrossRef Full Text | Google Scholar
11. Goldberg HJ, Whiteside CI, Hart GW, Fantus IG. Posttranslational, reversible O-glycosylation is stimulated by high glucose and mediates plasminogen activator inhibitor-1 gene expression and Sp1 transcriptional activity in glomerular mesangial cells. Endocrinology (2006) 147(1):222–31. doi:10.1210/en.2006-0523
Pubmed Abstract | Pubmed Full Text | CrossRef Full Text | Google Scholar
12. Andrali SS, Qian Q, Ozcan S. Glucose mediates the translocation of NeuroD1 by O-linked glycosylation. J Biol Chem (2007) 282(21):15589–96. doi:10.1074/jbc.M701762200
Pubmed Abstract | Pubmed Full Text | CrossRef Full Text | Google Scholar
13. Kuo M, Zilberfarb V, Gangneux N, Christeff N, Issad T. O-glycosylation of FoxO1 increases its transcriptional activity towards the glucose 6-phosphatase gene. FEBS Lett (2008) 582(5):829–34. doi:10.1016/j.febslet.2008.02.010
Pubmed Abstract | Pubmed Full Text | CrossRef Full Text | Google Scholar
14. Kuo M, Zilberfarb V, Gangneux N, Christeff N, Issad T. O-GlcNAc modification of FoxO1 increases its transcriptional activity: a role in the glucotoxicity phenomenon? Biochimie (2008) 90:679–85. doi:10.1016/j.biochi.2008.03.005
Pubmed Abstract | Pubmed Full Text | CrossRef Full Text | Google Scholar
15. Guinez C, Filhoulaud G, Rayah-Benhamed F, Marmier S, Dubuquoy C, Dentin R, et al. O-GlcNAcylation increases ChREBP protein content and transcriptional activity in the liver. Diabetes (2011) 60(5):1399–413. doi:10.2337/db10-0452
Pubmed Abstract | Pubmed Full Text | CrossRef Full Text | Google Scholar
16. Fardini Y, Masson E, Boudah O, Ben Jouira R, Cosson C, Pierre-Eugene C, et al. O-GlcNAcylation of FoxO1 in pancreatic beta cells promotes Akt inhibition through an IGFBP1-mediated autocrine mechanism. FASEB J (2014) 28(2):1010–21. doi:10.1096/fj.13-238378
Pubmed Abstract | Pubmed Full Text | CrossRef Full Text | Google Scholar
17. Issad T, Kuo M. O-GlcNAc modification of transcription factors, glucose sensing and glucotoxicity. Trends Endocrinol Metab (2008) 19(10):380–9. doi:10.1016/j.tem.2008.09.001
Pubmed Abstract | Pubmed Full Text | CrossRef Full Text | Google Scholar
18. Park SY, Ryu J, Lee W. O-GlcNAc modification on IRS-1 and Akt2 by PUGNAc inhibits their phosphorylation and induces insulin resistance in rat primary adipocytes. Exp Mol Med (2005) 37(3):220–9. doi:10.1038/emm.2005.30
Pubmed Abstract | Pubmed Full Text | CrossRef Full Text | Google Scholar
19. Lima VV, Giachini FR, Carneiro FS, Carneiro ZN, Fortes ZB, Carvalho MH, et al. Increased vascular O-GlcNAcylation augments reactivity to constrictor stimuli – VASOACTIVE PEPTIDE SYMPOSIUM. J Am Soc Hypertens (2008) 2(6):410–7. doi:10.1016/j.jash.2008.06.001
Pubmed Abstract | Pubmed Full Text | CrossRef Full Text | Google Scholar
20. Luo B, Soesanto Y, McClain DA. Protein modification by O-linked GlcNAc reduces angiogenesis by inhibiting Akt activity in endothelial cells. Arterioscler Thromb Vasc Biol (2008) 28(4):651–7. doi:10.1161/ATVBAHA.107.159533
Pubmed Abstract | Pubmed Full Text | CrossRef Full Text | Google Scholar
21. Yang X, Ongusaha PP, Miles PD, Havstad JC, Zhang F, So WV, et al. Phosphoinositide signalling links O-GlcNAc transferase to insulin resistance. Nature (2008) 451(7181):964–9. doi:10.1038/nature06668
Pubmed Abstract | Pubmed Full Text | CrossRef Full Text | Google Scholar
22. Lefebvre T, Dehennaut V, Guinez C, Olivier S, Drougat L, Mir AM, et al. Dysregulation of the nutrient/stress sensor O-GlcNAcylation is involved in the etiology of cardiovascular disorders, type-2 diabetes and Alzheimer’s disease. Biochim Biophys Acta (2009) 1800(2):67–79. doi:10.1016/j.bbagen.2009.08.008
Pubmed Abstract | Pubmed Full Text | CrossRef Full Text | Google Scholar
23. Weigert C, Klopfer K, Kausch C, Brodbeck K, Stumvoll M, Haring HU, et al. Palmitate-induced activation of the hexosamine pathway in human myotubes: increased expression of glutamine:fructose-6-phosphate aminotransferase. Diabetes (2003) 52(3):650–6. doi:10.2337/diabetes.52.3.650
Pubmed Abstract | Pubmed Full Text | CrossRef Full Text | Google Scholar
24. Busch AK, Cordery D, Denyer GS, Biden TJ. Expression profiling of palmitate- and oleate-regulated genes provides novel insights into the effects of chronic lipid exposure on pancreatic beta-cell function. Diabetes (2002) 51(4):977–87. doi:10.2337/diabetes.51.4.977
Pubmed Abstract | Pubmed Full Text | CrossRef Full Text | Google Scholar
25. Schrijvers BF, De Vriese AS. Novel insights in the treatment of diabetic nephropathy. Acta Clin Belg (2007) 62(5):278–90. doi:10.1179/acb.2007.043
Pubmed Abstract | Pubmed Full Text | CrossRef Full Text | Google Scholar
26. Haneda M, Koya D, Isono M, Kikkawa R. Overview of glucose signaling in mesangial cells in diabetic nephropathy. J Am Soc Nephrol (2003) 14(5):1374–82. doi:10.1097/01.ASN.0000064500.89551.76
27. Stockand JD, Sansom SC. Glomerular mesangial cells: electrophysiology and regulation of contraction. Physiol Rev (1998) 78(3):723–44.
28. Kolm-Litty V, Sauer U, Nerlich A, Lehmann R, Schleicher ED. High glucose-induced transforming growth factor beta1 production is mediated by the hexosamine pathway in porcine glomerular mesangial cells. J Clin Invest (1998) 101(1):160–9. doi:10.1172/JCI119875
Pubmed Abstract | Pubmed Full Text | CrossRef Full Text | Google Scholar
29. Weigert C, Friess U, Brodbeck K, Haring HU, Schleicher ED. Glutamine:fructose-6-phosphate aminotransferase enzyme activity is necessary for the induction of TGF-beta1 and fibronectin expression in mesangial cells. Diabetologia (2003) 46(6):852–5. doi:10.1007/s00125-003-1122-8
Pubmed Abstract | Pubmed Full Text | CrossRef Full Text | Google Scholar
30. Goldberg HJ, Whiteside CI, Fantus IG. The hexosamine pathway regulates the plasminogen activator inhibitor-1 gene promoter and Sp1 transcriptional activation through protein kinase C-beta I and -delta. J Biol Chem (2002) 277(37):33833–41. doi:10.1074/jbc.M112331200
Pubmed Abstract | Pubmed Full Text | CrossRef Full Text | Google Scholar
31. James LR, Tang D, Ingram A, Ly H, Thai K, Cai L, et al. Flux through the hexosamine pathway is a determinant of nuclear factor kappaB-dependent promoter activation. Diabetes (2002) 51(4):1146–56. doi:10.2337/diabetes.51.4.1146
Pubmed Abstract | Pubmed Full Text | CrossRef Full Text | Google Scholar
32. Yerneni KK, Bai W, Khan BV, Medford RM, Natarajan R. Hyperglycemia-induced activation of nuclear transcription factor kappaB in vascular smooth muscle cells. Diabetes (1999) 48(4):855–64. doi:10.2337/diabetes.48.4.855
Pubmed Abstract | Pubmed Full Text | CrossRef Full Text | Google Scholar
33. Hofmann MA, Schiekofer S, Kanitz M, Klevesath MS, Joswig M, Lee V, et al. Insufficient glycemic control increases nuclear factor-kappa B binding activity in peripheral blood mononuclear cells isolated from patients with type 1 diabetes. Diabetes Care (1998) 21(8):1310–6. doi:10.2337/diacare.21.8.1310
Pubmed Abstract | Pubmed Full Text | CrossRef Full Text | Google Scholar
34. Hofmann MA, Schiekofer S, Isermann B, Kanitz M, Henkels M, Joswig M, et al. Peripheral blood mononuclear cells isolated from patients with diabetic nephropathy show increased activation of the oxidative-stress sensitive transcription factor NF-kappaB. Diabetologia (1999) 42(2):222–32. doi:10.1007/s001250051142
35. Brownlee M. Biochemistry and molecular cell biology of diabetic complications. Nature (2001) 414(6865):813–20. doi:10.1038/414813a
36. Du XL, Edelstein D, Rossetti L, Fantus IG, Goldberg H, Ziyadeh F, et al. Hyperglycemia-induced mitochondrial superoxide overproduction activates the hexosamine pathway and induces plasminogen activator inhibitor-1 expression by increasing Sp1 glycosylation. Proc Natl Acad Sci U S A (2000) 97(22):12222–6. doi:10.1073/pnas.97.22.12222
Pubmed Abstract | Pubmed Full Text | CrossRef Full Text | Google Scholar
37. Brownlee M. The pathobiology of diabetic complications: a unifying mechanism. Diabetes (2005) 54(6):1615–25. doi:10.2337/diabetes.54.6.1615
38. Sen R, Baltimore D. Inducibility of kappa immunoglobulin enhancer-binding protein Nf-kappa B by a posttranslational mechanism. Cell (1986) 47(6):921–8. doi:10.1016/0092-8674(86)90807-X
Pubmed Abstract | Pubmed Full Text | CrossRef Full Text | Google Scholar
39. Sen R, Baltimore D. Multiple nuclear factors interact with the immunoglobulin enhancer sequences. Cell (1986) 46(5):705–16. doi:10.1016/0092-8674(86)90346-6
Pubmed Abstract | Pubmed Full Text | CrossRef Full Text | Google Scholar
40. Oeckinghaus A, Ghosh S. The NF-kappaB family of transcription factors and its regulation. Cold Spring Harb Perspect Biol (2009) 1(4):a000034. doi:10.1101/cshperspect.a000034
Pubmed Abstract | Pubmed Full Text | CrossRef Full Text | Google Scholar
41. Yang WH, Park SY, Nam HW, Kim do H, Kang JG, Kang ES, et al. NFkappaB activation is associated with its O-GlcNAcylation state under hyperglycemic conditions. Proc Natl Acad Sci U S A (2008) 105(45):17345–50. doi:10.1073/pnas.0806198105
Pubmed Abstract | Pubmed Full Text | CrossRef Full Text | Google Scholar
42. Allison DF, Wamsley JJ, Kumar M, Li D, Gray LG, Hart GW, et al. Modification of RelA by O-linked N-acetylglucosamine links glucose metabolism to NF-kappaB acetylation and transcription. Proc Natl Acad Sci U S A (2012) 109(42):16888–93. doi:10.1073/pnas.1208468109
Pubmed Abstract | Pubmed Full Text | CrossRef Full Text | Google Scholar
43. Yuan M, Konstantopoulos N, Lee J, Hansen L, Li ZW, Karin M, et al. Reversal of obesity- and diet-induced insulin resistance with salicylates or targeted disruption of Ikkbeta. Science (2001) 293(5535):1673–7. doi:10.1126/science.1061620
Pubmed Abstract | Pubmed Full Text | CrossRef Full Text | Google Scholar
44. Cai D, Yuan M, Frantz DF, Melendez PA, Hansen L, Lee J, et al. Local and systemic insulin resistance resulting from hepatic activation of IKK-beta and NF-kappaB. Nat Med (2005) 11(2):183–90. doi:10.1038/nm1166
Pubmed Abstract | Pubmed Full Text | CrossRef Full Text | Google Scholar
45. Itani SI, Ruderman NB, Schmieder F, Boden G. Lipid-induced insulin resistance in human muscle is associated with changes in diacylglycerol, protein kinase C, and IkappaB-alpha. Diabetes (2002) 51(7):2005–11. doi:10.2337/diabetes.51.7.2005
Pubmed Abstract | Pubmed Full Text | CrossRef Full Text | Google Scholar
46. Dandona P, Aljada A, Chaudhuri A, Mohanty P, Garg R. Metabolic syndrome: a comprehensive perspective based on interactions between obesity, diabetes, and inflammation. Circulation (2005) 111(11):1448–54. doi:10.1161/01.CIR.0000158483.13093.9D
47. Golks A, Tran TT, Goetschy JF, Guerini D. Requirement for O-linked N-acetylglucosaminyltransferase in lymphocytes activation. EMBO J (2007) 26(20):4368–79. doi:10.1038/sj.emboj.7601845
Pubmed Abstract | Pubmed Full Text | CrossRef Full Text | Google Scholar
48. Ramakrishnan P, Clark PM, Mason DE, Peters EC, Hsieh-Wilson LC, Baltimore D. Activation of the transcriptional function of the NF-kappaB protein c-Rel by O-GlcNAc glycosylation. Sci Signal (2013) 6(290):ra75. doi:10.1126/scisignal.2004097
Pubmed Abstract | Pubmed Full Text | CrossRef Full Text | Google Scholar
49. Hart GW. Nutrient regulation of immunity: O-GlcNAcylation regulates stimulus-specific NF-kappaB-dependent transcription. Sci Signal (2013) 6(290):e26. doi:10.1126/scisignal.2004596
Pubmed Abstract | Pubmed Full Text | CrossRef Full Text | Google Scholar
50. Kawauchi K, Araki K, Tobiume K, Tanaka N. Loss of p53 enhances catalytic activity of IKKbeta through O-linked beta-N-acetyl glucosamine modification. Proc Natl Acad Sci U S A (2009) 106(9):3431–6. doi:10.1073/pnas.0813210106
Pubmed Abstract | Pubmed Full Text | CrossRef Full Text | Google Scholar
51. Zou L, Yang S, Hu S, Chaudry IH, Marchase RB, Chatham JC. The protective effects of PUGNAc on cardiac function after trauma-hemorrhage are mediated via increased protein O-GlcNAc levels. Shock (2007) 27(4):402–8. doi:10.1097/01.shk.0000245031.31859.29
Pubmed Abstract | Pubmed Full Text | CrossRef Full Text | Google Scholar
52. Zou L, Yang S, Champattanachai V, Hu S, Chaudry IH, Marchase RB, et al. Glucosamine improves cardiac function following trauma-hemorrhage by increased protein O-GlcNAcylation and attenuation of NF-{kappa}B signaling. Am J Physiol Heart Circ Physiol (2009) 296(2):H515–23. doi:10.1152/ajpheart.01025.2008
Pubmed Abstract | Pubmed Full Text | CrossRef Full Text | Google Scholar
53. Xing D, Gong K, Feng W, Nozell SE, Chen YF, Chatham JC, et al. O-GlcNAc modification of NFkappaB p65 inhibits TNF-alpha-induced inflammatory mediator expression in rat aortic smooth muscle cells. PLoS One (2011) 6(8):e24021. doi:10.1371/journal.pone.0024021
Pubmed Abstract | Pubmed Full Text | CrossRef Full Text | Google Scholar
54. Xing D, Feng W, Not LG, Miller AP, Zhang Y, Chen YF, et al. Increased protein O-GlcNAc modification inhibits inflammatory and neointimal responses to acute endoluminal arterial injury. Am J Physiol Heart Circ Physiol (2008) 295(1):H335–42. doi:10.1152/ajpheart.01259.2007
Pubmed Abstract | Pubmed Full Text | CrossRef Full Text | Google Scholar
55. Hilgers RH, Xing D, Gong K, Chen YF, Chatham JC, Oparil S. Acute O-GlcNAcylation prevents inflammation-induced vascular dysfunction. Am J Physiol Heart Circ Physiol (2012) 303(5):H513–22. doi:10.1152/ajpheart.01175.2011
Pubmed Abstract | Pubmed Full Text | CrossRef Full Text | Google Scholar
56. Xu H, Barnes GT, Yang Q, Tan G, Yang D, Chou CJ, et al. Chronic inflammation in fat plays a crucial role in the development of obesity-related insulin resistance. J Clin Invest (2003) 112(12):1821–30. doi:10.1172/JCI19451
Pubmed Abstract | Pubmed Full Text | CrossRef Full Text | Google Scholar
57. Weisberg SP, McCann D, Desai M, Rosenbaum M, Leibel RL, Ferrante AW Jr. Obesity is associated with macrophage accumulation in adipose tissue. J Clin Invest (2003) 112(12):1796–808. doi:10.1172/JCI19246
Pubmed Abstract | Pubmed Full Text | CrossRef Full Text | Google Scholar
58. Obstfeld AE, Sugaru E, Thearle M, Francisco AM, Gayet C, Ginsberg HN, et al. C-C chemokine receptor 2 (CCR2) regulates the hepatic recruitment of myeloid cells that promote obesity-induced hepatic steatosis. Diabetes (2010) 59(4):916–25. doi:10.2337/db09-1403
Pubmed Abstract | Pubmed Full Text | CrossRef Full Text | Google Scholar
59. Ehses JA, Perren A, Eppler E, Ribaux P, Pospisilik JA, Maor-Cahn R, et al. Increased number of islet-associated macrophages in type 2 diabetes. Diabetes (2007) 56(9):2356–70. doi:10.2337/db06-1650
Pubmed Abstract | Pubmed Full Text | CrossRef Full Text | Google Scholar
60. Eriksson EE, Xie X, Werr J, Thoren P, Lindbom L. Importance of primary capture and L-selectin-dependent secondary capture in leukocyte accumulation in inflammation and atherosclerosis in vivo. J Exp Med (2001) 194(2):205–18. doi:10.1084/jem.194.2.205
Pubmed Abstract | Pubmed Full Text | CrossRef Full Text | Google Scholar
61. Osborn O, Olefsky JM. The cellular and signaling networks linking the immune system and metabolism in disease. Nat Med (2012) 18(3):363–74. doi:10.1038/nm.2627
Pubmed Abstract | Pubmed Full Text | CrossRef Full Text | Google Scholar
62. Stohr R, Federici M. Insulin resistance and atherosclerosis: convergence between metabolic pathways and inflammatory nodes. Biochem J (2013) 454(1):1–11. doi:10.1042/BJ20130121
Pubmed Abstract | Pubmed Full Text | CrossRef Full Text | Google Scholar
63. Shanmugam N, Reddy MA, Guha M, Natarajan R. High glucose-induced expression of proinflammatory cytokine and chemokine genes in monocytic cells. Diabetes (2003) 52(5):1256–64. doi:10.2337/diabetes.52.5.1256
Pubmed Abstract | Pubmed Full Text | CrossRef Full Text | Google Scholar
64. Dasu MR, Devaraj S, Zhao L, Hwang DH, Jialal I. High glucose induces toll-like receptor expression in human monocytes: mechanism of activation. Diabetes (2008) 57(11):3090–8. doi:10.2337/db08-0564
Pubmed Abstract | Pubmed Full Text | CrossRef Full Text | Google Scholar
65. Hua KF, Wang SH, Dong WC, Lin CY, Ho CL, Wu TH. High glucose increases nitric oxide generation in lipopolysaccharide-activated macrophages by enhancing activity of protein kinase C-alpha/delta and NF-kappaB. Inflamm Res (2012) 61(10):1107–16. doi:10.1007/s00011-012-0503-1
Pubmed Abstract | Pubmed Full Text | CrossRef Full Text | Google Scholar
66. Donath MY. Targeting inflammation in the treatment of type 2 diabetes: time to start. Nat Rev Drug Discov (2014) 13(6):465–76. doi:10.1038/nrd4275
Pubmed Abstract | Pubmed Full Text | CrossRef Full Text | Google Scholar
67. Ptak W, Klimek M, Bryniarski K, Ptak M, Majcher P. Macrophage function in alloxan diabetic mice: expression of adhesion molecules, generation of monokines and oxygen and NO radicals. Clin Exp Immunol (1998) 114(1):13–8. doi:10.1046/j.1365-2249.1998.00687.x
Pubmed Abstract | Pubmed Full Text | CrossRef Full Text | Google Scholar
68. Wen Y, Gu J, Li SL, Reddy MA, Natarajan R, Nadler JL. Elevated glucose and diabetes promote interleukin-12 cytokine gene expression in mouse macrophages. Endocrinology (2006) 147(5):2518–25. doi:10.1210/en.2005-0519
Pubmed Abstract | Pubmed Full Text | CrossRef Full Text | Google Scholar
69. Sun C, Sun L, Ma H, Peng J, Zhen Y, Duan K, et al. The phenotype and functional alterations of macrophages in mice with hyperglycemia for long term. J Cell Physiol (2012) 227(4):1670–9. doi:10.1002/jcp.22891
Pubmed Abstract | Pubmed Full Text | CrossRef Full Text | Google Scholar
70. Yamasawa H, Nakayama M, Bando M, Sugiyama Y. Impaired inflammatory responses to multiple toll-like receptor ligands in alveolar macrophages of streptozotocin-induced diabetic mice. Inflamm Res (2012) 61(5):417–26. doi:10.1007/s00011-011-0426-2
Pubmed Abstract | Pubmed Full Text | CrossRef Full Text | Google Scholar
71. Chikanishi T, Fujiki R, Hashiba W, Sekine H, Yokoyama A, Kato S. Glucose-induced expression of MIP-1 genes requires O-GlcNAc transferase in monocytes. Biochem Biophys Res Commun (2010) 394(4):865–70. doi:10.1016/j.bbrc.2010.02.167
Pubmed Abstract | Pubmed Full Text | CrossRef Full Text | Google Scholar
72. Hwang SY, Shin JH, Hwang JS, Kim SY, Shin JA, Oh ES, et al. Glucosamine exerts a neuroprotective effect via suppression of inflammation in rat brain ischemia/reperfusion injury. Glia (2010) 58(15):1881–92. doi:10.1002/glia.21058
Pubmed Abstract | Pubmed Full Text | CrossRef Full Text | Google Scholar
73. Hwang SY, Hwang JS, Kim SY, Han IO. O-GlcNAcylation and p50/p105 binding of c-Rel are dynamically regulated by LPS and glucosamine in BV2 microglia cells. Br J Pharmacol (2013) 169(7):1551–60. doi:10.1111/bph.12223
Pubmed Abstract | Pubmed Full Text | CrossRef Full Text | Google Scholar
74. Anagnostou SH, Shepherd PR. Glucose induces an autocrine activation of the Wnt/beta-catenin pathway in macrophage cell lines. Biochem J (2008) 416(2):211–8. doi:10.1042/BJ20081426
Pubmed Abstract | Pubmed Full Text | CrossRef Full Text | Google Scholar
75. Hresko RC, Heimberg H, Chi MM, Mueckler M. Glucosamine-induced insulin resistance in 3T3-L1 adipocytes is caused by depletion of intracellular ATP. J Biol Chem (1998) 273(32):20658–68. doi:10.1074/jbc.273.32.20658
Pubmed Abstract | Pubmed Full Text | CrossRef Full Text | Google Scholar
76. Kaneto H, Xu G, Song KH, Suzuma K, Bonner-Weir S, Sharma A, et al. Activation of the hexosamine pathway leads to deterioration of pancreatic beta-cell function through the induction of oxidative stress. J Biol Chem (2001) 276(33):31099–104. doi:10.1074/jbc.M104115200
Pubmed Abstract | Pubmed Full Text | CrossRef Full Text | Google Scholar
77. Hwang SY, Hwang JS, Kim SY, Han IO. O-GlcNAc transferase inhibits LPS-mediated expression of inducible nitric oxide synthase through an increased interaction with mSin3A in RAW264.7 cells. Am J Physiol Cell Physiol (2013) 305(6):C601–8. doi:10.1152/ajpcell.00042.2013
Pubmed Abstract | Pubmed Full Text | CrossRef Full Text | Google Scholar
78. Ryu IH, Do SI. Denitrosylation of S-nitrosylated OGT is triggered in LPS-stimulated innate immune response. Biochem Biophys Res Commun (2011) 408(1):52–7. doi:10.1016/j.bbrc.2011.03.115
Pubmed Abstract | Pubmed Full Text | CrossRef Full Text | Google Scholar
79. Zheng GM, Yu C, Yang Z. Puerarin suppresses production of nitric oxide and inducible nitric oxide synthase in lipopolysaccharide-induced N9 microglial cells through regulating MAPK phosphorylation, O-GlcNAcylation and NF-kappaB translocation. Int J Oncol (2012) 40(5):1610–8. doi:10.3892/ijo.2012.1331
Pubmed Abstract | Pubmed Full Text | CrossRef Full Text | Google Scholar
80. Lima VV, Spitler K, Choi H, Webb RC, Tostes RC. O-GlcNAcylation and oxidation of proteins: is signalling in the cardiovascular system becoming sweeter? Clin Sci (Lond) (2012) 123(8):473–86. doi:10.1042/CS20110638
Pubmed Abstract | Pubmed Full Text | CrossRef Full Text | Google Scholar
81. Ma Z, Vocadlo DJ, Vosseller K. Hyper-O-GlcNAcylation is anti-apoptotic and maintains constitutive NF-kappaB activity in pancreatic cancer cells. J Biol Chem (2013) 288(21):15121–30. doi:10.1074/jbc.M113.470047
Pubmed Abstract | Pubmed Full Text | CrossRef Full Text | Google Scholar
82. Torres CR, Hart GW. Topography and polypeptide distribution of terminal N-acetylglucosamine residues on the surfaces of intact lymphocytes. Evidence for O-linked GlcNAc. J Biol Chem (1984) 259(5):3308–17.
83. Kearse KP, Hart GW. Lymphocyte activation induces rapid changes in nuclear and cytoplasmic glycoproteins. Proc Natl Acad Sci U S A (1991) 88(5):1701–5. doi:10.1073/pnas.88.5.1701
Pubmed Abstract | Pubmed Full Text | CrossRef Full Text | Google Scholar
Keywords: O-GlcNAc glycosylation, diabetes, metabolic syndrome, inflammation, cytokines, macrophages, nitric oxide, NFκB
Citation: Baudoin L and Issad T (2015) O-GlcNAcylation and inflammation: a vast territory to explore. Front. Endocrinol. 5:235. doi: 10.3389/fendo.2014.00235
Received: 05 October 2014; Accepted: 18 December 2014;
Published online: 09 January 2015.
Edited by:
Tony Lefebvre, University Lille 1, FranceReviewed by:
Giovanni Solinas, University of Gothenburg, SwedenJames M. Murphy, Walter and Eliza Hall Institute of Medical Research, Australia
Copyright: © 2015 Baudoin and Issad. This is an open-access article distributed under the terms of the Creative Commons Attribution License (CC BY). The use, distribution or reproduction in other forums is permitted, provided the original author(s) or licensor are credited and that the original publication in this journal is cited, in accordance with accepted academic practice. No use, distribution or reproduction is permitted which does not comply with these terms.
*Correspondence: Tarik Issad, Department of Endocrinology, Metabolism and Diabetes, Institute Cochin, 22 rue Méchain, Paris 75014, France e-mail:dGFyaWsuaXNzYWRAaW5zZXJtLmZy