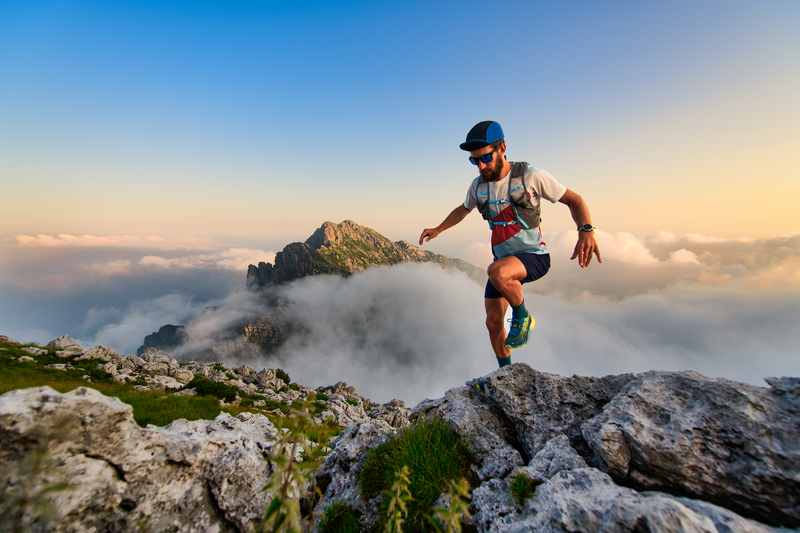
95% of researchers rate our articles as excellent or good
Learn more about the work of our research integrity team to safeguard the quality of each article we publish.
Find out more
REVIEW article
Front. Endocrinol. , 17 September 2013
Sec. Neuroendocrine Science
Volume 4 - 2013 | https://doi.org/10.3389/fendo.2013.00125
This article is part of the Research Topic The regulated secretory pathway in neuroendocrine cells View all 18 articles
The regulated secretory pathway in neuroendocrine cells ends with the release of hormones and neurotransmitters following a rise in cytosolic calcium. This process known as regulated exocytosis involves the assembly of soluble N-ethylmaleimide-sensitive factor attachment protein receptor (SNARE) proteins, the synaptic vesicle VAMP (synaptobrevin), and the plasma membrane proteins syntaxin and SNAP-25. Although there is much evidence suggesting that SNARE proteins play a key role in the fusion machinery, other cellular elements regulating the kinetics, the extent of fusion, and the preparation of vesicle for release have received less attention. Among those factors, lipids have also been proposed to play important functions both at the level of secretory vesicle recruitment and late membrane fusion steps. Here, we will review the latest evidence supporting the concept of the fusogenic activity of lipids, and also discuss how this may be achieved. These possibilities include the recruitment and sequestration of the components of the exocytotic machinery, regulation of protein function, and direct effects on membrane topology.
Release of hormones from neuroendocrine cells occurs through exocytosis, a process in which specialized vesicles (secretory granules) fuse with the plasma membrane in response to extrinsic stimuli leading to elevated cytosolic calcium. Chromaffin cells have proven to be a valuable model for unraveling the molecular mechanisms underlying this fundamental membrane fusion event (1). In these cells, secretory granules are first transported from the Golgi area to the cell periphery where they are then docked at the plasma membrane in two apparent stages: a non-primed (fusion incompetent) and a primed (fusion competent) state. Details of the molecular machinery underlying some of these steps have been described (2). For instance, once tethered to the plasma membrane, docking of granules is mediated by soluble N-ethylmaleimide-sensitive factor (NSF) attachment protein receptors (SNAREs) found on granules (v-SNAREs) and on the plasma membrane (t-SNAREs). Through coiled-coil interactions, these proteins form a stable complex that provides the energy necessary to pull membranes into close proximity rendering them fusion competent (3). The mechanism of membrane fusion per se is however an aspect that continues to be debated. SNAREs are able to drive membrane fusion in vitro (4), but with relatively slow kinetics suggesting that additional factors are required for physiological membrane fusion. Recently, the SNARE accessory protein Munc18 was shown to increase the speed of SNARE-mediated fusion (5). Lipids, the prime constituents of the fusing membranes, are also obvious candidates to accelerate the fusion process. The first lipid firmly demonstrated to be critical for exocytosis was phosphatidylinositol-4,5-bisphosphate [PtdIns(4,5)P2], for review, see Ref. (6), and more recently fatty acids have been suggested to play an important function in neurotransmitter release from synaptic vesicles (7). Growing evidence also support a role for cholesterol and phosphatidic acid (PA) during exocytosis, for review, see Ref. (8). Hence, many observations are in agreement with the notion that lipids contribute at different levels to exocytosis. The issues now arising concern the precise step in the dynamic exocytotic process in which a given lipid actually functions.
Cholesterol and sphingolipids cluster into discrete microdomains in cellular membranes to form lipid ordered domains. This clustering is triggered by secretagogues at the level of exocytotic sites in chromaffin cells (9). Although cholesterol depletion by methyl-β-cyclodextrin provided the initial evidence for a positive role of cholesterol in exocytosis (10), these experiments are subjected to caution because of the brought structural role of cholesterol in most cellular functions. More compelling evidence supporting a direct role for cholesterol relies on both biochemical and high-resolution imaging observations indicating that SNAREs concentrate in cholesterol-dependent clusters (11). Additionally, a cholesterol-sequestering agent, the polyene antibiotic filipin, that is supposed to have less dramatic effects on membrane structures than methyl-β-cyclodextrin, induces a dose-dependent inhibition of catecholamine secretion and the release from the plasma membrane of annexin A2 which participates in the formation and/or stabilization of GM1-PtdIns(4,5)P2 enriched domains required for granule recruitment (9, 12). Altogether these findings support the notion that cholesterol is involved in the spatial definition of exocytotic sites.
In the plasma membrane, phosphatidylserine (PS) mostly resides in the inner cytoplasmic leaflet. In non-apoptotic cells, several biological functions are accompanied by a disruption of this phospholipid asymmetry resulting in the externalization of PS on the outer leaflet of the plasma membrane. This is the case for calcium-regulated exocytosis in neuroendocrine chromaffin and PC12 cells (13, 14). However the functional importance of PS scrambling for secretion is still under debate and the precise kinetics of this translocation is not established. An interesting possibility lies in the fact that PS contributes substantially to the negative charge of the inner leaflet of the plasma membrane. Consequently, PS scrambling at exocytotic sites could modify the protein/lipid interactions occurring during either the course of exocytosis or the early phases of endocytosis, as suggested recently (15).
Phosphoinositides are a class of phospholipids characterized by an inositol head group that can be phosphorylated on the three, four, and five positions to generate seven distinct species key in cell signaling and trafficking. Much of the work carried out on exocytosis has focused on the role played by PtdIns(4,5)P2. Indeed a number of pioneer studies indicated that PtdIns(4,5)P2 positively modulates secretion in neuroendocrine cells (16–18). Using patch clamp experiments on intact chromaffin cells and in parallel analyzing images of plasma membrane lawns, it was subsequently shown that over-expression of the kinase that generates PtdIns(4,5)P2 causes an increase in the plasmalemmal PtdIns(4,5)P2 level and secretion, whereas over-expression of a membrane-tagged PtdIns(4,5)P2 phosphatase eliminates plasmalemmal PtdIns(4,5)P2 and inhibits secretion (19). Thus, the balance between the generation and degradation rates of the plasmalemmal PtdIns(4,5)P2 directly regulates the extent of exocytosis from chromaffin cell. Using the phosphatidylinositol 3-kinase inhibitor LY294002, a correlation between the level of the plasma membrane PtdIns(4,5)P2 and the size of the primed vesicle pool was found (19, 20). Wen et al. (21) further demonstrated that selective inhibition of phosphatidylinositol 3-kinase delta isoform was responsible for this effect. Importantly, such an inhibition promotes a transient rise in PtdIns(4,5)P2 that was sufficient to mobilize secretory vesicles to the plasma membrane via activation of the small GTPase Cdc42 and actin polymerization. More recently, a functional link between PtdIns(4,5)P2 signaling and secretory vesicle dynamics through de novo remodeling of the actin cytoskeleton was also described (22). These observations are consistent with a function of PtdIns(4,5)P2 as an acute regulator of secretion. PtdIns(4,5)P2 seems to lie in a key position controlling the size and refilling rate of the primed vesicle pools, but not the fusion rate constants per se. In line with this model, we recently reported that the HIV PtdIns(4,5)P2-binding protein Tat is able to penetrate neuroendocrine cells and accumulate at the plasma membrane through its binding to PtdIns(4,5)P2. By sequestering plasma membrane PtdIns(4,5)P2, Tat alters neurosecretion, reducing the number of exocytotic events without significantly affecting kinetic parameters (fusion pore opening, dilatation, and closure) of individual events (23).
Other phosphoinositides seem to act as signaling or recruitment factors to prime secretory vesicles for exocytosis. For instance, experiments carried out on permeabilized chromaffin cells reveal that PtdIns(3)P located on a subpopulation of chromaffin granules positively regulates secretion (21, 24). Extending these observations, PIKfyve kinase that produces PtdIns(3,5)P2 from PtdIns(3)P on secretory granules was shown to negatively affect exocytosis (25). Hence, these studies highlight a complex regulation of neuroexocytosis by phosphoinositides, with PtdIns(4,5)P2 and PtdIns(3)P being essential factors promoting ATP-dependent priming in neurosecretory cells. It is intriguing that PtdIns(3,5)P2 displays an opposite effect, but reveals how fine-tuning of exocytosis by phosphoinositides could potentially control the number of vesicles undergoing priming in response to a stimulation.
The local formation of PA is a recurring theme in intracellular membrane traffic and its involvement in regulated exocytosis has been suggested in various models, including neuroendocrine cells (14, 26). The development of molecular tools has enabled the identification of phospholipase D1 (PLD1) as the key enzyme responsible for PA synthesis during exocytosis (14, 27). Capacitance recordings from chromaffin cells silenced for PLD1 suggest that PLD1 controls the number of fusion competent secretory granules at the plasma membrane without affecting earlier recruitment or docking steps, leading to the idea that PA acts directly in membrane fusion (28). In agreement with this concept, a molecular sensor for PA revealed local PA accumulation at the plasma membrane near morphologically docked granules at sites of active exocytosis (28).
Various other lipids are suspected to take part in regulated exocytosis. Although most of them have been implicated based on in vitro membrane fusion assays, some have also been studied in neuroendocrine cells. For instance, diacylglycerol (DAG) increases stimulus-coupled secretion by recruiting vesicles to the immediately releasable pool through the regulation of the vesicle priming protein Munc13-1 (29). Furthermore by activating protein kinase C, DAG may modulate the phosphorylation level of various proteins contributing or regulating the exocytotic machinery, including SNAP-25 and Munc18 (30, 31). Modulating PS levels also directly affects the rate of exocytosis in PC12 cells. Although it is likely that long term provision of high level of PS (incubation with 100 μM for 48 h or over-expression of PS synthase) may affect numerous key cellular functions altogether, it was shown that PS influences exocytosis by enhancing fusion pore opening and slowing fusion pore dilatation in PC12 cells (32). The kinetic changes in exocytosis resulting from elevated PS levels were shown to be unlikely the reflection of an indirect action of PS on Ca2+ channels, or vesicle size or number, or the levels of some of the major exocytosis proteins, but may be the consequence of synaptotagmin binding to PS (32). Finally, arachidonic acid produced from different phospholipids by phospholipase A2 and from DAG by DAG-lipase potentiates exocytosis from chromaffin cells (33, 34).
Within membranes, the ability of microdomains to sequester specific proteins and exclude others makes them ideally suited to spatially organize cellular pathways. Cholesterol-enriched microdomains seem to concentrate components of the exocytotic/fusion machinery. For instance, numerous studies of the distribution of SNARE proteins in various cell types suggest that SNAREs partially associate with detergent resistant, cholesterol-enriched microdomains (11). Palmitoylation appears to be the major targeting signal in these microdomains, as in the case of SNAP-25, although it is likely that other elements contribute to the enrichment of constituents of the exocytotic machinery within these cholesterol microdomains. However despite intense research there is still little known about what lipid or protein molecules are actually present at sites of exocytosis.
Up to 20 proteins potentially involved in regulated exocytosis have been reported to bind PtdIns(4,5)P2 (35). These include synaptotagmin (36), syntaxin (37), Ca2+-dependent activator protein for secretion (CAPS) (38), and members of the PLD signaling pathway (16, 39, 40). Using immunogold labeling of plasma membrane sheets combined with spatial point pattern analysis, we recently observed that PtdIns(4,5)P2 microdomains co-localize with SNARE clusters and docked secretory granules (12). Translocation of the PtdIns(4,5)P2-binding protein annexin A2 to the plasma membrane following cell stimulation is a hallmark of chromaffin cell exocytosis (41). Annexin A2 plays an essential role in calcium-regulated exocytosis by promoting PtdIns(4,5)P2 and cholesterol-enriched domains containing SNAREs in the vicinity of docked granules (9, 12). Altogether these observation raise the notion that functional exocytotic sites defined by specific lipids such as cholesterol, GM1, and PtdIns(4,5)P2 are able to recruit and sequester components to build a machine that drives fast and efficient membrane fusion (Figure 1).
Figure 1. Model highlighting the importance of lipids for membrane fusion. Exocytotic sites defined as cholesterol, GM1, PtdIns(4,5)P2, and PA enriched microdomains recruit constituents of the docking/fusion machinery and create membrane curvature of the inner leaflet of the plasma membrane prior to promote membrane merging. PtdIns(4,5)P2 and PA may also regulate SNARE complex assembly and structure.
Molecular details of how PtdIns(4,5)P2 forms a platform for vesicle recruitment have recently been proposed (42). In this study, synaptotagmin-1 was shown to interact independently of Ca2+ with the polybasic linker region of syntaxin-1A already associated with PtdIns(4,5)P2 at the plasma membrane. When then triggered by Ca2+, this interaction allows the Ca2+-binding sites of synaptotagmin-1 to bind PS in the vesicle membrane, thereby bridging the vesicle membrane close enough for SNARE assembly and subsequent membrane fusion. Interestingly, this polybasic juxtamembrane domain of syntaxin-1A which binds PtdIns(4,5)P2 has also been shown to tightly bind both PA (37) and PtdIns(3,4,5)P3 (43). Like PtdIns(4,5)P2, these anionic lipids can probably recruit syntaxin-1A, and it is tempting to propose that the recruitment of syntaxin isoforms may depend on the type of lipid present. Furthermore, the fact that these lipids can be quickly converted into different forms using kinases, lipases, and phosphatases, such a protein-recruiting mechanism offers a supplementary level of control to adapt the exocytotic machinery to the physiological demands put on the cell. Finally, using super-resolution optical techniques and fluorescence lifetime imaging microscopy, it was shown that distinct t-SNARE intermediate states on the plasma membrane can be patterned by the underlying lipid environment (44). Undoubtedly these high-resolution imaging techniques will be useful to determine how lipids contribute to the organization of the exocytotic platform.
PtdIns(4,5)P2 directly binds to a large subset of proteins from the exocytotic machinery. In vitro experiments revealed that at physiological concentrations PtdIns(4,5)P2 regulates exocytosis by recruiting priming factors such as CAPS, which facilitate SNARE-dependent liposome fusion (45). PtdIns(4,5)P2 is also a necessary cofactor for PLD1 activity and PA synthesis during exocytosis (39). Finally, PtdIns(4,5)P2 controls actin polymerization by modulating the activity and targeting of actin regulatory proteins. Indeed the activity of the actin-binding proteins scinderin and gelsolin, two F-actin severing proteins that are constituents of the exocytotic machinery, is regulated by PtdIns(4,5)P2 (46). A transient increase in PIP2 levels is sufficient to promote the mobilization and recruitment of secretory vesicles to the plasma membrane (22). PIP2 therefore links exocytosis and the actin cytoskeleton by coordinating the actin-based delivery of secretory vesicles to the exocytotic sites.
Diacylglycerol production through hydrolysis of PtdIns(4,5)P2 by phospholipase C is mandatory for exocytosis (47). DAG is essential in the priming of exocytosis, owing to the activation of protein kinase C and Munc13, which then modulate the function of syntaxin-1A (29). It is worth to mention that up to 10 different DAG-kinases may also produce DAG from various subcellular pools of PA during exocytosis. Finally DAG is further hydrolyzed by DAG lipases to liberate fatty acids and monoacylglycerols. This pathway is essential for exocytosis as inhibition of DAG lipase blocks exocytosis (48).
Several constituents and regulators of the exocytotic machinery have also been shown to bind to PA, including small GTPases, NSF, and syntaxin-1A (49). PA directly activates these proteins, but evidence that this activation directly contributes to exocytosis remains scarce. PA is also an essential cofactor of phosphatidylinositol-4-phosphate 5-kinase, which produces PtdIns(4,5)P2, suggesting a possible positive feedback loop in the synthesis of PA and PtdIns(4,5)P2 (50). Although no direct evidence in neuroendocrine systems have shown that PA directly regulates the assembly or the function of the minimal fusion machinery, in vitro reconstituted fusion assays with purified yeast vacuolar SNAREs do so. Indeed experiments performed in a complex reconstituted system including the SNARE chaperones Sec17p/Sec18p, the multifunctional HOPS complex including a subunit of the Sec1-Munc18 family and vacuolar lipids suggest that PA is equally essential for SNARE complex assembly and for fusion. PA has been proposed to facilitate functional interactions among SNAREs and SNARE chaperones (Figure 1). Interestingly, in this system, PA could not be replaced by either lipids with small headgroups, such as DAG or acidic lipids, like PS or PI (51).
Arachidonic acid has been described to directly promote the assembly of syntaxin-3 with SNAP-25 and the formation of the ternary SNARE complex (52). Interestingly, omega-3 and omega-6 fatty acids, which play important roles in human health, have be shown to recapitulate this in vitro effect of arachidonic acid on SNARE complex formation, suggesting that syntaxins may represent crucial targets of polyunsaturated lipids (52). In other words, polyunsaturated lipids may physiologically regulate SNARE complex assembly and thus exocytosis. Along this same line, sphingosine a releasable backbone of sphingolipids, activates vesicular synaptobrevin facilitating the assembly of SNARE complexes required for membrane fusion (53). It is however important to note that the effects of arachidonic acid and sphingosine observed in these studies are all achieved near or at the CMC value for these lipids, treatments that may also lead to membrane disorganization like detergent action.
The most widely accepted model for membrane fusion, the stalk pore model proposes that the merging of cis contacting monolayers gives rise to a negatively curved lipid structure called a stalk. The structure of this stalk depends on the composition of the cis monolayers (the outer leaflet of the vesicle and the inner leaflet of the plasma membrane). This model implies that cone-shaped lipids such as cholesterol, DAG, or PA, which have intrinsic negative curvatures, in the cis leaflets of contacting bilayers would enhance membrane fusion (54). Vice versa inverted cone-shaped lipids (such as PS, gangliosides, or lysophospholipids) should prevent membrane fusion in the cis leaflets, but promote fusion when present in the outer leaflets (54). Interestingly, GM1 was found enriched in the outer leaflet of the plasma membrane at the sites of exocytosis in stimulated chromaffin cells (9). These GM1 domains may induce positive membrane curvature in the outer leaflet (55), thereby promoting fusion (Figure 1). Reconstituted fusion assays and direct addition of lipids on cultured cells validate the concept that PA, DAG, and cholesterol might promote membrane fusion by changing the spontaneous curvature of membranes [reviewed in (8)]. At physiological concentrations, PtdIns(4,5)P2 inhibits SNARE-dependent liposome fusion (45), most likely due to its intrinsic positive curvature-promoting properties. However, PtdIns(4,5)P2 has been described to be converted from an inverted cone-shaped structure to a cone-shaped form in the presence of calcium (56). Thus, in stimulated cells, a local accumulation of PA and PtdIns(4,5)P2 at granule docking sites where GM1 is in the outer leaflets may well have a synergistic effect on membrane curvature and promote fusion (Figure 1). In an alternate mode of changing membrane topology, synaptotagmin has been proposed to facilitate membrane fusion by phase separating PS, a process that is expected to locally buckle bilayers and disorder lipids due to the curvature tendencies of PS (57). It is worth to mention that most of lipid mentioned in this review, also have the ability to flip from one leaflet to another. How this flipping is regulated and how it affects curvature remains an unsolved issue. However, it is likely that the ability of these lipids to interact with the fusion machinery largely controls these flipping properties.
As genuine components of the exocytotic machinery, lipids have several advantages over proteins for this task: lipids can directly change the intrinsic fusion properties of membranes, recruit, and/or activate a large number of different proteins to create a local environment in which exocytosis takes place (Figure 1). As illustrated in this review, a given lipid can play multiple functions, acting either individually or successively or even simultaneously in concert with other lipids. At the same time, the rapid enzymatic production and degradation of lipids at exocytotic sites allows the cell to remain flexible: by changing the lipid levels, physiological function can be modified within seconds or minutes without the need for protein synthesis or degradation. Over the last decade, in vitro reconstituted membrane fusion combined with precise methods to quantify specific lipid species and improved molecular and pharmacological tools to manipulate cellular levels of a given lipid, have lead to a better understanding of the capacities of lipids to promote exocytosis at different steps of the process. For different kinds of vesicles in different cell types, it is likely that the local lipid environment may differentially regulate fusion pore formation, enlargement, and duration, which may in part explain the great variety of fusion kinetics observed in vivo. Finally, lipids could also contribute to the tight coupling between exocytosis and the early stages of membrane retrieval and endocytosis as highlighted in a review of this issue (Houy et al., submitted). Undoubtedly, the next challenge will be to follow individual lipid dynamics at the speed of pore formation and expansion.
The authors declare that the research was conducted in the absence of any commercial or financial relationships that could be construed as a potential conflict of interest.
We wish to thank Dr. Nancy Grant for critical reading of the manuscript. This work is supported by Agence Nationale pour la Recherche (ANR-09-BLAN-0264-01 grant) to Nicolas Vitale.
1. Bader MF, Doussau F, Chasserot-Golaz S, Vitale N, Gasman S. Coupling actin and membrane dynamics during calcium-regulated exocytosis: a role for Rho and ARF GTPases. Biochim Biophys Acta (2004) 1742:37–49. doi:10.1016/j.bbamcr.2004.09.028
2. Jahn R, Fasshauer D. Molecular machines governing exocytosis of synaptic vesicles. Nature (2012) 490:201–7. doi:10.1038/nature11320
3. Sutton RB, Fasshauer D, Jahn R, Brunger AT. Crystal structure of a SNARE complex involved in synaptic exocytosis at 2.4 A resolution. Nature (1998) 395:347–53. doi:10.1038/26412
4. Weber T, Zemelman BV, McNew JA, Westermann B, Gmachl M, Parlati F, et al. SNAREpins: minimal machinery for membrane fusion. Cell (1998) 92:759–72. doi:10.1016/S0092-8674(00)81404-X
5. Ma C, Su L, Seven AB, Xu Y, Rizo J. Reconstitution of the vital functions of Munc18 and Munc13 in neurotransmitter release. Science (2013) 339:421–5. doi:10.1126/science.1230473
6. Martin TF. Role of PI(4,5)P(2) in vesicle exocytosis and membrane fusion. Subcell Biochem (2012) 59:111–30. doi:10.1007/978-94-007-3015-1_4
7. Darios F, Connell E, Davletov B. Phospholipases and fatty acid signalling in exocytosis. J Physiol (2007) 585:699–704. doi:10.1113/jphysiol.2007.136812
8. Chasserot-Golaz S, Coorssen JR, Meunier FA, Vitale N. Lipid dynamics in exocytosis. Cell Mol Neurobiol (2010) 30:1335–42. doi:10.1007/s10571-010-9577-x
9. Chasserot-Golaz S, Vitale N, Umbrecht-Jenck E, Knight D, Gerke V, Bader MF. Annexin 2 promotes the formation of lipid microdomains required for calcium-regulated exocytosis of dense-core vesicles. Mol Biol Cell (2005) 16:1108–19. doi:10.1091/mbc.E04-07-0627
10. Chamberlain LH, Burgoyne RD, Gould GW. SNARE proteins are highly enriched in lipid rafts in PC12 cells: implications for the spatial control of exocytosis. Proc Natl Acad Sci U S A (2001) 98:5619–24. doi:10.1073/pnas.091502398
11. Lang T. SNARE proteins and “membrane rafts”. J Physiol (2007) 585:693–8. doi:10.1113/jphysiol.2007.134346
12. Umbrecht-Jenck E, Demais V, Calco V, Bailly Y, Bader MF, Chasserot-Golaz S. S100A10-mediated translocation of annexin-A2 to SNARE proteins in adrenergic chromaffin cells undergoing exocytosis. Traffic (2010) 11:958–71. doi:10.1111/j.1600-0854.2010.01065.x
13. Ceridono M, Ory S, Momboisse F, Chasserot-Golaz S, Houy S, Calco V, et al. Selective recapture of secretory granule components after full collapse exocytosis in neuroendocrine chromaffin cells. Traffic (2011) 12:72–88. doi:10.1111/j.1600-0854.2010.01125.x
14. Vitale N, Caumont AS, Chasserot-Golaz S, Du G, Wu S, Sciorra VA, et al. Phospholipase D1: a key factor for the exocytotic machinery in neuroendocrine cells. EMBO J (2001) 20:2424–34. doi:10.1093/emboj/20.10.2424
15. Ory S, Ceridono M, Momboisse F, Houy S, Chasserot-Golaz S, Heintz D, et al. Phospholipid scramblase-1-induced lipid reorganization regulates compensatory endocytosis in neuroendocrine cells. J Neurosci (2013) 33:3545–56. doi:10.1523/JNEUROSCI.3654-12.2013
16. Aikawa Y, Martin TF. ARF6 regulates a plasma membrane pool of phosphatidylinositol(4,5)bisphosphate required for regulated exocytosis. J Cell Biol (2003) 162:647–59. doi:10.1083/jcb.200212142
17. Eberhard DA, Cooper CL, Low MG, Holz RW. Evidence that the inositol phospholipids are necessary for exocytosis. Loss of inositol phospholipids and inhibition of secretion in permeabilized cells caused by a bacterial phospholipase C and removal of ATP. Biochem J (1990) 268:15–25.
18. Holz RW, Hlubek MD, Sorensen SD, Fisher SK, Balla T, Ozaki S, et al. A pleckstrin homology domain specific for phosphatidylinositol 4, 5-bisphosphate (PtdIns-4,5-P2) and fused to green fluorescent protein identifies plasma membrane PtdIns-4,5-P2 as being important in exocytosis. J Biol Chem (2000) 275:17878–85. doi:10.1074/jbc.M000925200
19. Milosevic I, Sorensen JB, Lang T, Krauss M, Nagy G, Haucke V, et al. Plasmalemmal phosphatidylinositol-4,5-bisphosphate level regulates the releasable vesicle pool size in chromaffin cells. J Neurosci (2005) 25:2557–65. doi:10.1523/JNEUROSCI.3761-04.2005
20. Chasserot-Golaz S, Hubert P, Thierse D, Dirrig S, Vlahos CJ, Aunis D, et al. Possible involvement of phosphatidylinositol 3-kinase in regulated exocytosis: studies in chromaffin cells with inhibitor LY294002. J Neurochem (1998) 70:2347–56. doi:10.1046/j.1471-4159.1998.70062347.x
21. Wen PJ, Osborne SL, Morrow IC, Parton RG, Domin J, Meunier FA. Ca2+-regulated pool of phosphatidylinositol-3-phosphate produced by phosphatidylinositol 3-kinase C2alpha on neurosecretory vesicles. Mol Biol Cell (2008) 19:5593–603. doi:10.1091/mbc.E08-06-0595
22. Wen PJ, Osborne SL, Zanin M, Low PC, Wang HT, Schoenwaelder SM, et al. Phosphatidylinositol(4,5)bisphosphate coordinates actin-mediated mobilization and translocation of secretory vesicles to the plasma membrane of chromaffin cells. Nat Commun (2011) 2:491. doi:10.1038/ncomms1500
23. Tryoen-Toth P, Chasserot-Golaz S, Tu A, Gherib P, Bader MF, Beaumelle B, et al. HIV-1 Tat protein inhibits neurosecretion by binding to phosphatidylinositol 4,5-bisphosphate. J Cell Sci (2013) 126:454–63. doi:10.1242/jcs.111658
24. Meunier FA, Osborne SL, Hammond GR, Cooke FT, Parker PJ, Domin J, et al. Phosphatidylinositol 3-kinase C2alpha is essential for ATP-dependent priming of neurosecretory granule exocytosis. Mol Biol Cell (2005) 210:4841–51. doi:10.1091/mbc.E05-02-0171
25. Osborne SL, Wen PJ, Boucheron C, Nguyen HN, Hayakawa M, Kaizawa H, et al. PIKfyve negatively regulates exocytosis in neurosecretory cells. J Biol Chem (2008) 283:2804–13. doi:10.1074/jbc.M704856200
26. Bader MF, Vitale N. Phospholipase D in calcium-regulated exocytosis: lessons from chromaffin cells. Biochim Biophys Acta (2009) 1791:936–41. doi:10.1016/j.bbalip.2009.02.016
27. Waselle L, Gerona RR, Vitale N, Martin TF, Bader MF, Regazzi R. Role of phosphoinositide signaling in the control of insulin exocytosis. Mol Endocrinol (2005) 19:3097–106. doi:10.1210/me.2004-0530
28. Zeniou-Meyer M, Zabari N, Ashery U, Chasserot-Golaz S, Haeberle AM, Demais V, et al. Phospholipase D1 production of phosphatidic acid at the plasma membrane promotes exocytosis of large dense-core granules at a late stage. J Biol Chem (2007) 282:21746–57. doi:10.1074/jbc.M702968200
29. Bauer CS, Woolley RJ, Teschemacher AG, Seward EP. Potentiation of exocytosis by phospholipase C-coupled G-protein-coupled receptors requires the priming protein Munc13-1. J Neurosci (2007) 27:212–9. doi:10.1523/JNEUROSCI.4201-06.2007
30. Barclay JW, Craig TJ, Fisher RJ, Ciufo LF, Evans GJ, Morgan A, et al. Phosphorylation of Munc18 by protein kinase C regulates the kinetics of exocytosis. J Biol Chem (2003) 278:10538–45. doi:10.1074/jbc.M211114200
31. Yang Y, Craig TJ, Chen X, Ciufo LF, Takahashi M, Morgan A, et al. Phosphomimetic mutation of Ser-187 of SNAP-25 increases both syntaxin binding and highly Ca2+-sensitive exocytosis. J Gen Physiol (2007) 129:233–44. doi:10.1085/jgp.200609685
32. Zhang Z, Hui E, Chapman ER, Jackson MB. Phosphatidylserine regulation of Ca2+-triggered exocytosis and fusion pores in PC12 cells. Mol Biol Cell (2009) 20:5086–95. doi:10.1091/mbc.E09-08-0691
33. Latham CF, Osborne SL, Cryle MJ, Meunier FA. Arachidonic acid potentiates exocytosis and allows neuronal SNARE complex to interact with Munc18a. J Neurochem (2007) 100:1543–54.
34. Vitale N. Synthesis of fusogenic lipids through activation of phospholipase D1 by GTPases and the kinase RSK2 is required for calcium-regulated exocytosis in neuroendocrine cells. Biochem Soc Trans (2010) 38:167–71. doi:10.1042/BST0380167
35. Koch M, Holt M. Coupling exo- and endocytosis: an essential role for PIP(2) at the synapse. Biochim Biophys Acta (2012) 1821:1114–32. doi:10.1016/j.bbalip.2012.02.008
36. Schiavo G, Gu QM, Prestwich GD, Sollner TH, Rothman JE. Calcium-dependent switching of the specificity of phosphoinositide binding to synaptotagmin. Proc Natl Acad Sci U S A (1996) 93:13327–32. doi:10.1073/pnas.93.23.13327
37. Lam AD, Tryoen-Toth P, Tsai B, Vitale N, Stuenkel EL. SNARE-catalyzed fusion events are regulated by syntaxin1A-lipid interactions. Mol Biol Cell (2008) 19:485–97. doi:10.1091/mbc.E07-02-0148
38. Loyet KM, Kowalchyk JA, Chaudhary A, Chen J, Prestwich GD, Martin TF. Specific binding of phosphatidylinositol 4,5-bisphosphate to calcium-dependent activator protein for secretion (CAPS), a potential phosphoinositide effector protein for regulated exocytosis. J Biol Chem (1998) 273:8337–43. doi:10.1074/jbc.273.14.8337
39. Du G, Altshuller YM, Vitale N, Huang P, Chasserot-Golaz S, Morris AJ, et al. Regulation of phospholipase D1 subcellular cycling through coordination of multiple membrane association motifs. J Cell Biol (2003) 162:305–15. doi:10.1083/jcb.200302033
40. Liao H, Ellena J, Liu L, Szabo G, Cafiso D, Castle D. Secretory carrier membrane protein SCAMP2 and phosphatidylinositol 4,5-bisphosphate interactions in the regulation of dense core vesicle exocytosis. Biochemistry (2007) 46:10909–20. doi:10.1021/bi701121j
41. Chasserot-Golaz S, Vitale N, Sagot I, Delouche B, Dirrig S, Pradel LA, et al. Annexin II in exocytosis: catecholamine secretion requires the translocation of p36 to the subplasmalemmal region in chromaffin cells. J Cell Biol (1996) 133:1217–36. doi:10.1083/jcb.133.6.1217
42. Honigmann A, van den Bogaart G, Iraheta E, Risselada HJ, Milovanovic D, Mueller V, et al. Phosphatidylinositol 4,5-bisphosphate clusters act as molecular beacons for vesicle recruitment. Nat Struct Mol Biol (2013) 20:679–86. doi:10.1038/nsmb.2570
43. Khuong TM, Habets RL, Kuenen S, Witkowska A, Kasprowicz J, Swerts J, et al. Synaptic PI(3,4,5)P3 is required for syntaxin1A clustering and neurotransmitter release. Neuron (2013) 77:1097–108. doi:10.1016/j.neuron.2013.01.025
44. Rickman C, Medine CN, Dun AR, Moulton DJ, Mandula O, Halemani ND, et al. t-SNARE protein conformations patterned by the lipid microenvironment. J Biol Chem (2010) 285:13535–41. doi:10.1074/jbc.M109.091058
45. James DJ, Khodthong C, Kowalchyk JA, Martin TF. Phosphatidylinositol 4,5-bisphosphate regulates SNARE-dependent membrane fusion. J Cell Biol (2008) 182:355–66. doi:10.1083/jcb.200801056
46. Trifaro JM, Gasman S, Gutierrez LM. Cytoskeletal control of vesicle transport and exocytosis in chromaffin cells. Acta Physiol (Oxf) (2008) 192:165–72. doi:10.1111/j.1748-1716.2007.01808.x
47. Hammond GR, Dove SK, Nicol A, Pinxteren JA, Zicha D, Schiavo G. Elimination of plasma membrane phosphatidylinositol (4,5)-bisphosphate is required for exocytosis from mast cells. J Cell Sci (2006) 119:2084–94. doi:10.1242/jcs.02912
48. Sheu L, Pasyk EA, Ji J, Huang X, Gao X, Varoqueaux F, et al. Regulation of insulin exocytosis by Munc13-1. J Biol Chem (2003) 278:27556–63. doi:10.1074/jbc.M303203200
49. Jang JH, Lee CS, Hwang D, Ryu SH. Understanding of the roles of phospholipase D and phosphatidic acid through their binding partners. Prog Lipid Res (2011) 51:71–81. doi:10.1016/j.plipres.2011.12.003
50. Honda A, Nogami M, Yokozeki T, Yamazaki M, Nakamura H, Watanabe H, et al. Phosphatidylinositol 4-phosphate 5-kinase alpha is a downstream effector of the small G protein ARF6 in membrane ruffle formation. Cell (1999) 99:521–32. doi:10.1016/S0092-8674(00)81540-8
51. Mima J, Wickner W. Complex lipid requirements for SNARE- and SNARE chaperone-dependent membrane fusion. J Biol Chem (2009) 284:27114–22. doi:10.1074/jbc.M109.010223
52. Darios F, Davletov B. Omega-3 and omega-6 fatty acids stimulate cell membrane expansion by acting on syntaxin 3. Nature (2006) 440:813–7. doi:10.1038/nature04598
53. Darios F, Wasser C, Shakirzyanova A, Giniatullin A, Goodman K, Munoz-Bravo JL, et al. Sphingosine facilitates SNARE complex assembly and activates synaptic vesicle exocytosis. Neuron (2009) 62:683–94. doi:10.1016/j.neuron.2009.04.024
54. Chernomordik LV, Kozlov MM. Membrane hemifusion: crossing a chasm in two leaps. Cell (2005) 123:375–82. doi:10.1016/j.cell.2005.10.015
55. Mrowczynska L, Lindqvist C, Iglic A, Hagerstrand H. Spontaneous curvature of ganglioside GM1 – effect of cross-linking. Biochem Biophys Res Commun (2012) 422:776–9. doi:10.1016/j.bbrc.2012.05.083
56. Zimmerberg J, Chernomordik LV. Membrane fusion. Adv Drug Deliv Rev (1999) 38:197–205. doi:10.1016/S0169-409X(99)00029-0
Keywords: cholesterol, phosphatidic acids, phosphoinositides, exocytosis, membrane fusion, chromaffin cell
Citation: Ammar MR, Kassas N, Chasserot-Golaz S, Bader M-F and Vitale N (2013) Lipids in regulated exocytosis: what are they doing? Front. Endocrinol. 4:125. doi: 10.3389/fendo.2013.00125
Received: 18 June 2013; Accepted: 31 August 2013;
Published online: 17 September 2013.
Edited by:
Stephane Gasman, Centre National de la Recherche Scientifique, FranceReviewed by:
Thomas F. J. Martin, University of Wisconsin, USACopyright: © 2013 Ammar, Kassas, Chasserot-Golaz, Bader and Vitale. This is an open-access article distributed under the terms of the Creative Commons Attribution License (CC BY). The use, distribution or reproduction in other forums is permitted, provided the original author(s) or licensor are credited and that the original publication in this journal is cited, in accordance with accepted academic practice. No use, distribution or reproduction is permitted which does not comply with these terms.
*Correspondence: Nicolas Vitale, Institut des Neurosciences Cellulaires et Intégratives (INCI), UPR-3212 Centre National de la Recherche Scientifique, Université de Strasbourg, 5 rue Blaise Pascal, 67084 Strasbourg, France e-mail:dml0YWxlbkBpbmNpLWNucnMudW5pc3RyYS5mcg==
Disclaimer: All claims expressed in this article are solely those of the authors and do not necessarily represent those of their affiliated organizations, or those of the publisher, the editors and the reviewers. Any product that may be evaluated in this article or claim that may be made by its manufacturer is not guaranteed or endorsed by the publisher.
Research integrity at Frontiers
Learn more about the work of our research integrity team to safeguard the quality of each article we publish.