- Cell Biology Section, Division of Intramural Research, National Institute of Environmental Health Sciences, National Institutes of Health, Research Triangle Park, NC, USA
Retinoic acid-related orphan receptors RORα and RORγ play a regulatory role in lipid/glucose homeostasis and various immune functions, and have been implicated in metabolic syndrome and several inflammatory diseases. RORα-deficient mice are protected against age- and diet-induced obesity, hepatosteatosis, and insulin resistance. The resistance to hepatosteatosis in RORα-deficient mice is related to the reduced expression of several genes regulating lipid synthesis, transport, and storage. Adipose tissue-associated inflammation, which plays a critical role in the development of insulin resistance, is considerably diminished in RORα-deficient mice as indicated by the reduced infiltration of M1 macrophages and decreased expression of many proinflammatory genes. Deficiency in RORγ also protects against diet-induced insulin resistance by a mechanism that appears different from that in RORα deficiency. Recent studies indicated that RORs provide an important link between the circadian clock machinery and its regulation of metabolic genes and metabolic syndrome. As ligand-dependent transcription factors, RORs may provide novel therapeutic targets in the management of obesity and associated metabolic diseases, including hepatosteatosis, adipose tissue-associated inflammation, and insulin resistance.
Introduction
In the past 50 years, the occurrence of obesity has greatly increased worldwide in both adults and children and has become a major health-care concern in many countries. In the United States 30% of the population is considered obese, while more than 66% of adults and almost 17% of children and adolescents are overweight (Browning et al., 2004; Ogden et al., 2012). Obesity is associated with an increased risk of several pathologies, including type 2 diabetes, cardiovascular disease, and non-alcoholic fatty liver disease (NAFLD). Accumulating evidence indicates that networks regulating lipid metabolism and inflammation are highly integrated and play a critical role in the development of these pathologies (Hotamisligil, 2006; Donath and Shoelson, 2011; Ouchi et al., 2011; Glass and Olefsky, 2012). Obesity leads to a systemic state of low-grade inflammation, particularly involving adipose tissue, that is causally involved in the development of insulin resistance and other diseases. Blood levels of free fatty acids (FFA) are elevated in obesity and through their interaction with Toll-like receptor 4 (TLR4) FFA induce proinflammatory pathways in macrophages and other cell types that may promote insulin resistance (Samuel and Shulman, 2012). Recent studies demonstrated that retinoic acid-related orphan receptors (RORs) are among many factors that through their modulation of immune responses and lipid/glucose homeostasis regulate the development of inflammation, metabolic syndrome, and insulin resistance (Jetten, 2009; Solt and Burris, 2012).
RORα and γ Proteins
The RORs alpha, beta, and gamma (RORα–γ or NR1F1–3) constitute a subfamily of nuclear receptors that function as ligand-dependent transcription factors (Jetten, 2004, 2009; Solt and Burris, 2012). RORs exhibit a domain structure typical of nuclear receptors and contain an N-terminal domain, the function of which has not yet been clearly defined, a highly conserved DNA-binding domain (DBD) consisting of two zinc finger motifs, a LBD, and a hinge domain spacing the DBD and LBD. By using different promoters and/or alternative splicing each ROR gene produces several isoforms that vary only in their N-terminal region. Some of these isoforms exhibit a distinct tissue-specific pattern of expression and control different genes and biological processes. RORs regulate transcription by binding as monomers to ROR response elements (RORE), which consist of the core sequence “AGGTCA” preceded by an A/T-rich sequence, in the regulatory region of target genes. The activation function (AF-2), localized at the C-terminus within the LBD of RORs, is involved in the recruitment of co-activators or co-repressors that mediate the transcriptional activation or repression by RORs. Recent studies have identified a number of (ant)agonists that interact with the LBD of ROR and either activate or inhibit ROR transcriptional activity (Kallen et al., 2002; Huh and Littman, 2012; Solt and Burris, 2012). Interaction with agonists induces a conformational change in the LBD that allows release of the co-repressor complex and promotes assembly of a co-activator complex that mediates the transcriptional activation by ROR, while the inverse happens for antagonists. These observations not only indicated that RORs function as ligand-dependent transcription factors, but also suggested that RORs might be potential therapeutic targets to treat disease.
RORs as Regulators of Several Immune Processes
RORα and RORγ are important regulators of several diverse immune functions. RORγ-deficient mice lack lymph nodes and Peyer’s patches indicating that it is essential for lymph node development (Kurebayashi et al., 2000; Sun et al., 2000). Recent studies demonstrated that RORα and the RORγt isoform play a key role in T cell lineage determination (Ivanov et al., 2006; Yang et al., 2008; Jetten, 2009). The RORγt isoform in particular and to a lesser extent RORα, is required for the differentiation of naïve T cells into interleukin 17 (IL-17) producing T helper 17 (Th17) cells. IL-17A expression is directly regulated by RORs through their interaction with ROREs in the Il17 promoter (Yang et al., 2008). Proinflammatory Th17 cells and IL-17 have been implicated in several autoimmune diseases and other inflammatory disorders. Deficiency in RORγt or both RORα/γ receptors has been shown to greatly inhibit the generation of Th17 cells and the development of experimental encephalomyelitis in mice. In addition, mice deficient in RORα or RORγ displayed a diminished susceptibility to allergen-induced lung inflammation and collagen-induced arthritis (Jaradat et al., 2006; Tilley et al., 2007) and polymorphisms in RORα have been associated with increased susceptibility to asthma (Ramasamy et al., 2012). A recent study identified a role for RORα in the generation of natural helper (NH) cells (Halim et al., 2012). RORα-deficient, but not RORγ-deficient, mice lack NH cells. NH cell-deficient mice generated by RORα-deficient bone marrow transplantation exhibited normal Th2 cell responses, but failed to develop acute lung inflammation in response to a protease allergen. These findings might at least in part explain the reduced susceptibility to allergen-induced lung inflammation in RORα-deficient mice (Jaradat et al., 2006).
An increased Th17 response has been reported to correlate with white adipose tissue (WAT)-associated inflammation and the development of insulin resistance in obese mice and patients (Ahmed and Gaffen, 2010; Bertola et al., 2012). Whether inhibition of Th17 differentiation plays a role in the protection RORα- and RORγ-deficient mice against diet-induced insulin resistance needs further study. RORα or RORγ have also been implicated in the regulation of thymopoiesis. Loss of RORγt results in accelerated apoptosis of double-positive thymocytes, while RORα deficiency significantly reduces the generation of single positive thymocytes ( Kurebayashi et al., 2000; Sun et al., 2000; Dzhagalov et al., 2004).
RORα in Diet- and Age-Induced Obesity
Study of Staggerer (RORαsg/sg) mice, a natural mutant strain containing a deletion in the RORα gene that results in loss of RORα expression, indicated that RORα plays a critical role in the control of lipid metabolism and the development of various aspects of metabolic syndrome. These investigations showed that RORαsg/sg mice are protected against age- and diet-induced obesity and the development of several obesity-linked pathologies, including adipose tissue-associated inflammation, hepatosteatosis, and insulin resistance (Kang et al., 2011; Lau et al., 2011). RORαsg/sg mice fed a high fat diet (HFD) gain relatively less weight and exhibit a significantly lower total body fat index compared to wild-type (WT) littermates on a HFD. Similarly, male RORαsg/sg mice were also protected against age-induced obesity. Adipose tissue is the main site of storage of excess energy that is stored in the form of triglycerides in single large lipid droplets. The reduced adiposity in RORαsg/sg mice was largely related to smaller adipocyte size due to diminished deposition of triglycerides.
RORα, particularly the RORα4 isoform, has been shown to be highly expressed in WAT and to be induced during differentiation of D1 and 3T3-L1 preadipocytes (Austin et al., 1998). Overexpression of RORα in preadipocytes inhibits adipocyte differentiation (Duez et al., 2009; Ohoka et al., 2009). This appears to be mediated through a direct interaction of RORα with CCAAT/enhancer-binding protein β (C/EBPβ) that results in the inhibition of the recruitment of the co-activator CBP and repression of C/EBPβ transcriptional activity. These studies suggest that RORα has a negative regulatory role in adipocyte differentiation. This function, however, does not explain the reduced adiposity observed in RORα-deficient mice.
Obesity is a consequence of an imbalance between energy intake and expenditure (Glass and Olefsky, 2012; Samuel and Shulman, 2012). However, the decrease in diet-induced adiposity in RORαsg/sg mice was found not to be due to reduced food intake or increased fecal lipid excretion. Indirect calorimetric analysis showed that VO2, VCO2, and heat generation were significantly enhanced in RORαsg/sg mice on a HFD (Kang et al., 2011). This suggested that elevated energy expenditure might at least in part be responsible for the reduced weight gain and resistance to hepatosteatosis and insulin insensitivity in RORαsg/sg mice.
RORα and WAT-Associated Inflammation
In addition to functioning as the main site of storage of extra energy in the form of triglycerides derived from food intake, white adipocytes produce a variety of endocrine hormones, including leptin, adiponectin, resistin, and retinol-binding protein 4 (RBP-4) which regulate food intake, lipid metabolism, and inflammation (Hotamisligil, 2006; Guilherme et al., 2008; Glass and Olefsky, 2012). Leptin and adiponectin promote insulin sensitivity, while resistin and RBP4 have the opposite effect and impair insulin sensitivity. It is now well-recognized that obesity is associated with a chronic state of low grade, systemic inflammation and that this is an important contributory factor in the development of insulin resistance (Hotamisligil, 2006; Odegaard and Chawla, 2008; Nishimura et al., 2009; Glass and Olefsky, 2012). Progressive infiltration of various immune cells, including macrophages and CD8+ effector T lymphocytes, in WAT lead to increased release of proinflammatory cyto- and chemokines. In addition to the accumulation of bone marrow-derived macrophages, there is also a shift from anti-inflammatory “alternatively activated” (CD11c-CD206+) M2 macrophages to proinflammatory “classically activated” (CD11c+CD206-) M1 macrophages (Sun et al., 2011; Glass and Olefsky, 2012), which in advanced obesity aggregate into crown-like structures (CLS) surrounding necrotic adipocytes. Recent studies indicated that CD8+ T cells are critical in promoting recruitment of macrophages in WAT in obesity (Weisberg et al., 2003; Odegaard and Chawla, 2008; Nishimura et al., 2009). In addition, a reduction in anti-inflammatory T regulatory (Treg) cells and an increase in proinflammatory Th17 response further stimulate WAT-associated inflammation (Figure 1)
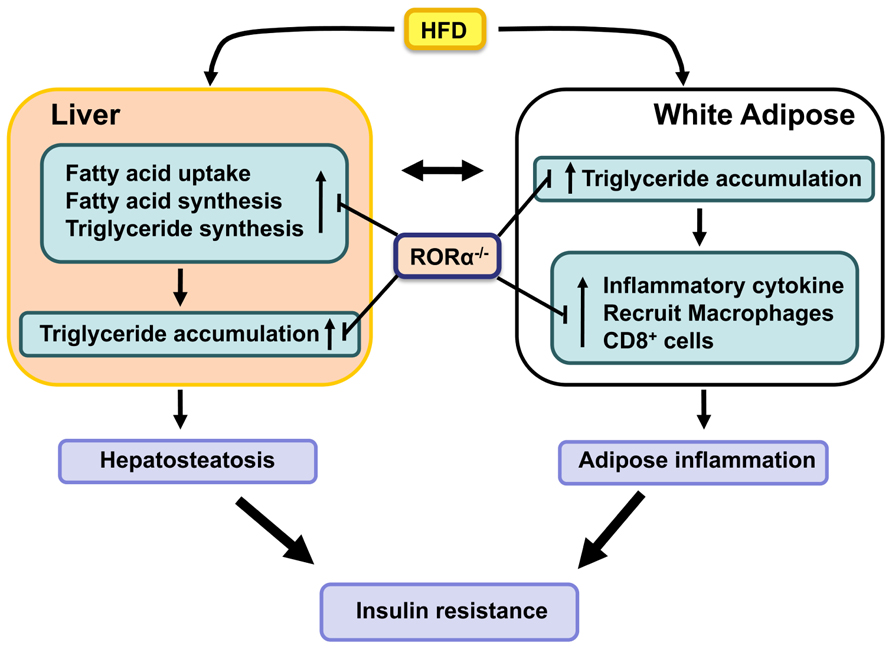
FIGURE 1. RORα functions as a positive regulator of hepatosteatosis, WAT-associated inflammation, and insulin-resistance in diet- and age-induced obesity. Loss of RORα inhibits the hepatic expression of lipogenic genes and suppresses the expression of proinflammatory genes and the infiltration of macrophages in WAT, and as a result protects against these pathologies.
Deficiency of RORα greatly inhibits diet-induced adipose tissue-associated inflammation in mice (Kang et al., 2011; Lau et al., 2011). This is indicated by the greatly reduced infiltration of macrophages and CD8+ T lymphocytes in WAT of RORαsg/sg mice fed a HFD. This was further supported by the significant reduction in the formation of CLS and the expression of several macrophage markers, such as F4/80, Mac-2, macrophage expressed 1 (Mpeg1), and macrophage scavenger receptor 1 (Msr1), in WAT of RORαsg/sg mice. Moreover, the relative percentage of proinflammatory M1 macrophages was significantly diminished in RORαsg/sg WAT. This was supported by flow cytometric analysis and the much lower levels of Cd11c expression. The reduced inflammation in RORαsg/sg WAT is further indicated by gene expression profiling showing a greatly reduced expression of a large number of chemokines, including Ccl2, Ccl8, Ccl3, and Ccl7, the chemokine receptors Ccr3, Ccr5, and Ccr7, the proinflammatory cytokines Tnfα and IL-6, the interleukin 1 receptor antagonist (Il1rn), osteopontin (Opn), CD44, serum amyloid 3 (Saa3), and several TLRs and metalloproteinases in WAT of RORαsg/sg mice compared to their WT counterparts (Kang et al., 2011). The expression of these genes has been reported to be elevated in obesity and many of these genes have been implicated in obesity-induced inflammation in WAT as well as insulin resistance. For example, both the CCL2/CCR2 and CCL3/CCR5 pathways have been reported to promote recruitment of macrophages in adipose tissue (Kanda et al., 2006; Kitade et al., 2012). CD44, a multifunctional cell membrane protein that acts as a receptor for hyaluronan and Opn, has been shown to regulate migration of macrophages and neutrophils (Johnson and Ruffell, 2009). CD44 and Opn null mice are protected against the development of HFD-induced hepatosteatosis, WAT-associated inflammation, and insulin resistance (Nomiyama et al., 2007; Bertola et al., 2009; Kiefer et al., 2011; Kodama et al., 2012). These observations suggest that suppression of several proinflammatory genes and pathways in RORαsg/sg WAT is causally linked to the reduced inflammation (Figure 1). Future studies have to determine what the primary effects are by which RORα regulate the expression of these genes.
RORα and Hepatosteatosis
Obesity is associated with increased prevalence of NAFLD, which is characterized by elevated lipid accumulation in hepatocytes (Fabbrini et al., 2010). NAFLD develops when the rate of fatty acid uptake and synthesis and subsequent esterification to triglycerides is greater than the rate of fatty acid oxidation and secretion. Advanced NAFLD progresses into increased inflammation and hepatotoxicity. Several studies showed that compared to WT mice hepatic triglyceride levels are considerably reduced in RORαsg/sg mice fed a HFD or aging male RORαsg/sg mice (Raspe et al., 2001; Lau et al., 2008; Kang et al., 2011). These observations indicated that RORαsg/sg mice are protected against the development of age- and diet-induced hepatosteatosis. Gene expression profiling revealed that the expression of a number of lipogenic genes was significantly reduced in the liver of RORαsg/sg mice fed a HFD. Expression of Srebp-1c and fatty acid synthase (Fas), key regulators for lipogenesis, was reduced in liver of RORαsg/sg mice. In addition, the expression of several genes involved in the main pathway of triglyceride synthesis, including glycerol-3-phosphate acyltransferase (Gpam or Gpat1) and acyl-glycerol-3-phosphate acyltransferase 9 (Agpat9) and Mogat1, which is part of an alternative pathway of triglyceride synthesis, were significantly diminished in RORαsg/sg liver (Kang et al., 2011). The hepatic expression of the cell death-inducing DFFA-like effectors a and c (Cidea and Cidec) and perilipin 2 (Plin2 or Adfp), which play a critical role in the regulation of lipid storage, lipid droplet formation, and lipolysis (Gong et al., 2009; Greenberg et al., 2011), was also suppressed in RORα-deficient mice. RORα has been reported to activate Plin2 transcription directly through interaction with ROREs in the Plin2 promoter (Kang et al., 2011). Recently, the expression of fibroblast growth factor (Fgf21), an important regulator of lipid/glucose metabolism, was found to be directly regulated by RORα in hepatocytes (Wang et al., 2010c). Together these observations suggest that the protection against hepatosteatosis in RORαsg/sg mice is related to reduced expression of many genes involved in promoting lipogenesis and triglyceride storage, some of which are directly regulated by RORα (Figure 1).
RORα and Insulin Resistance
Both adipose-associated inflammation and hepatosteatosis have been linked to the pathogenesis of insulin resistance in obesity (Guilherme et al., 2008; Donath and Shoelson, 2011; Samuel and Shulman, 2012), although a cause-effect relationship not always exists between hepatosteatosis and diabetes (Sun and Lazar, 2013). The phenotypic differences observed between WT and RORαsg/sg mice fed a HFD are consistent with this correlation. RORαsg/sg mice, which are protected against obesity, hepatosteatosis, and WAT-associated inflammation, exhibited a significantly reduced susceptibility to diet-induced insulin resistance and glucose intolerance compared to obese WT mice (Lau et al., 2008; Kang et al., 2011). In humans, two studies have revealed a connection between RORα, obesity, and type 2 diabetes. A rearrangement resulting in disruption of human RORα1 was found to be associated with severe obesity (Klar et al., 2005), while a recent GWAS study showed an association between a single nucleotide polymorphism in RORα (rs7164773) and increased risk for type 2 diabetes in the Mexico Mestizo population (Gamboa-Melendez et al., 2012).
Many inflammatory and lipogenic genes, including Plin2, Il1rn, Opn, CD44, and Cidec, that are down-regulated in RORαsg/sg mice have been reported to also regulate insulin sensitivity. Plin2 null mice displayed reduced hepatic lipid accumulation and improved insulin sensitivity and glucose tolerance in an ob/ob background (Chang et al., 2010). Il1rn, one of the genes most dramatically repressed in WAT of RORαsg/sg mice (Kang et al., 2011), has been reported to be highly up-regulated in WAT of obese humans and to regulate insulin sensitivity (Juge-Aubry et al., 2003; Somm et al., 2006). Similarly, Opn expression was found to be elevated in obesity, while Opn deficiency was shown to inhibit obesity-induced inflammation and insulin resistance (Bertola et al., 2009; Kiefer et al., 2011). Deficiency in CD44, a receptor for Opn, also results in improved insulin sensitivity (Kodama et al., 2012) suggesting a role for the Opn/CD44 pathway in the control of insulin sensitivity. Mice deficient in Cidea or Cidec, which play a role in lipid storage, are protected from diet-induced obesity and display improved insulin sensitivity (Gong et al., 2009). Thus, the down-regulation of several genes, including Plin2, Il1rn, Opn, CD44, and Cidec in RORαsg/sg mice may collaboratively be responsible for the improved insulin sensitivity through their interrelated effects on inflammation, adipogenesis, and lipid homeostasis (Figure 1).
In addition to adipose tissue and liver, the pancreas and the skeletal muscle also play important roles in energy homeostasis and insulin resistance. The pancreatic islets produce a number of hormones, including insulin and glucagon, that are critical in the regulation of lipid and glucose homeostasis (Saltiel and Kahn, 2001; Cryer, 2012). RORα was shown to be selectively expressed in the glucagon-producing alpha cells; however, its role in these cells and its relationship to the phenotype observed in RORα-deficient mice needs yet to be established (Mühlbauer et al., 2013). In skeletal muscle, RORα has been reported to regulate the expression of a number of genes involved in lipid and carbohydrate metabolism (Lau et al., 2011). Ectopic expression of an RORα mutant in skeletal muscle C2C12 cells reduced the expression of the lipogenic genes, sterol regulatory element-binding transcription factor 1 (Srebp1), Fas, and stearoyl-CoA desaturase 1 (Scd1), and genes involved in cholesterol efflux, such as ATP-binding cassette, sub-family A, member 1 (Abca1). Caveolin-3 (Cav3) and carnitine palmitoyltransferase-1 (Cpt1) were found to be directly regulated by RORα. Changes in the expression of these genes may be in part responsible for the modulation of lipid and glucose homeostasis by RORα.
In muscle, insulin stimulates glucose uptake by stimulating the translocation of Glut4 (Slc2a4) to the plasma membrane (Rose and Richter, 2005). This involves phosphorylation of the insulin receptor substrate 1 (IRS1), which leads to the activation of phosphatidylinositol 3-kinase (PI3K) and subsequently AKT, which then promotes Glut4 translocation. Recently, evidence was provided for a role of RORα in PI3K-Akt signaling (Lau et al., 2011). Akt1/2 expression was up-regulated in skeletal muscle of RORαsg/sg mice and this correlated with an increase in the level of insulin-induced Akt phosphorylation, Glut4 expression, and glucose uptake. This stimulation in Akt signaling might at least in part account for the improved insulin sensitivity observed in RORαsg/sg mice.
RORγ1 and Insulin Sensitivity
The RORγ gene generates two different isoforms, RORγ1 and RORγt (RORγ2), that are expressed in a highly tissue-specific manner (Jetten, 2009). Expression of the RORγ1 isoform is restricted to several peripheral tissues, including liver, adipose tissue, kidney, small intestines, pancreas, and skeletal muscle. Recent studies identified RORγ1 as a negative regulator of adipocyte differentiation and a modulator of obesity-associated insulin resistance (Meissburger et al., 2011; Tinahones et al., 2012). In obese RORγ -/- mice, the number of adipocytes was increased (hyperplasia), while adipocyte size was reduced. Fasting blood insulin levels were shown to be significantly lower in diet-induced obese RORγ-/- mice and in RORγ-/-ob/ob double knockout mice and mice displayed improved insulin sensitivity. In addition, RORα-/- adipocytes were highly insulin sensitive leading to improved control of circulating FFA. These observations are consistent with a recent study showing that, opposed to adipose hypertrophy, obese patients with adipose tissue hyperplasia (many small adipocytes) exhibit better glucose and lipid profiles and might be less susceptible to developing insulin resistance (Hoffstedt et al., 2010) and with data showing that in human patients the level of RORγ1 expression positively correlated with adipocyte size and insulin resistance (Meissburger et al., 2011; Tinahones et al., 2012). Up to now, no association has been established between RORγ polymorphisms and susceptibility to insulin resistance in humans. However, in cattle, a single polynucleotide polymorphism in RORγ has been linked to increased adiposity (Barendse et al., 2007). These observations suggest that the loss or potentially the inhibition of RORγ1 might protect against insulin resistance and type 2 diabetes.
In addition to adipose tissue, regulation of lipid and glucose metabolism in other tissues, including liver, pancreas, and skeletal muscle might be part of the mechanism by which by RORγ modulates insulin sensitivity. In skeletal muscle, RORγ has been reported to regulate the expression of genes associated with lipid and carbohydrate metabolism as well as the production of reactive oxygen species (Raichur et al., 2007). A recent study revealed that RORγ was selectively expressed in insulin-producing pancreatic β cells; however, its role in β cells and how this relates to the modulation of insulin sensitivity by RORγ has yet to be established (Mühlbauer et al., 2013). Further study is required to understand the modulation of lipid/glucose homeostasis and insulin sensitivity by RORγ.
Connection between RORs, Circadian Rhythm, and Metabolic Syndrome
It has been well established that many behavioral and physiological activities display circadian rhythms that are regulated by endogenous clocks (Asher and Schibler, 2011; Bass, 2012; Mohawk et al., 2012). At the molecular level the clockwork consists of an integral network of several interlocking positive and negative transcriptional and translational feedback loops that include the transcriptional regulators brain and muscle ARNT-like 1 (Bmal1), neuronal PAS domain protein 2 (Npas2), circadian locomotor output cycles kaput (Clock), two cryptochrome proteins (Cry1, 2), the nuclear receptors Rev-erbα and -β, E4 promoter-binding protein 4 (E4bp4), and three period proteins (Per1-3).
Accumulating evidence suggests that disruption of circadian rhythm is closely associated with several pathologies, including sleep disorders, cancer and metabolic syndrome (Maury et al., 2010). Recent studies have established a strong link between the circadian clock machinery and the regulation of a number of metabolic pathways (Asher and Schibler, 2011; Bass, 2012). Bmal1, Clock, and Cry1 have been implicated in the regulation of glucose homeostasis and dysfunction in these proteins lead to impaired glucose tolerance (Rudic et al., 2004; Zhang et al., 2010). Hepatic overexpression of Cry1 has been reported to improve insulin-sensitivity in insulin-resistant db/db mice (Zhang et al., 2010). In addition, circadian oscillator components, such as Cry1, have been implicated in the regulation of immune responses (Castanon-Cervantes et al., 2010; Logan and Sarkar, 2012; Narasimamurthy et al., 2012). In Cry1-/-Cry2-/- cells, NF-κB and protein kinase A (PKA) signaling pathways are constitutively activated resulting in elevated levels of circulating TNFα, Il-1β, and Il-6 (Narasimamurthy et al., 2012).
A number of studies demonstrated that RORs play a role in the modulation of circadian behavior and clock gene expression ( Sato et al., 2004; Ueda et al., 2005; Duez and Staels, 2010; Figure 2). Bmal1, Npas2, E4bp4, and Cry1 transcription are directly regulated by RORγ and RORα in several peripheral tissues through their interaction with ROREs in their regulatory regions (Crumbley et al., 2010; Takeda et al., 2011, 2012). RORγ1 appears to be the major ROR isotype modulating the circadian expression of clock genes in peripheral tissues. RORγ1 itself exhibits a strong oscillatory pattern of expression in several peripheral tissues, including kidney, liver, pancreas, and adipose tissue, while RORα exhibits only a weak circadian expression pattern (Mongrain et al., 2008; Takeda et al., 2012; Mühlbauer et al., 2013). The RORγ1 gene is directly regulated by Bmal1/Clock heterodimers which interact with two successive E-boxes in the RORγ1 promoter (Mongrain et al., 2008; Takeda et al., 2012). Recent studies have suggested that RORγ1 and RORα might provide a link between the clock machinery and their regulation of metabolic genes (Takeda et al., 2012; Figure 2). Data demonstrating that the circadian pattern of expression of a number of metabolic genes are regulated by clock proteins and RORs and observations showing that circadian expression of RORγ1 is controlled by the clock machinery suggested that RORs might function as downstream mediators in the mechanism by which clock proteins regulate the circadian expression of metabolic genes (Sato et al., 2004; Akashi and Takumi, 2005; Guillaumond et al., 2005; Ueda et al., 2005; Crumbley et al., 2010; Duez and Staels, 2010; Takeda et al., 2011, 2012). This is supported by observations showing that RORs regulate the circadian pattern of expression of a number of genes involved in the lipid/glucose homeostasis, including Plin2, sulfotransferase Sul1E1, the vasopressin receptor Avpr1a, and citrate synthase (CS), which exhibit roles in lipogenesis, glycogenolysis, and/or cholesterogenesis (Kang et al., 2007; Crumbley et al., 2012; Takeda et al., 2012). Thus, RORs appear to be part of the mechanism that links the circadian clock to its regulation of lipid/glucose homeostasis, inflammation, and insulin resistance (Figure 2).
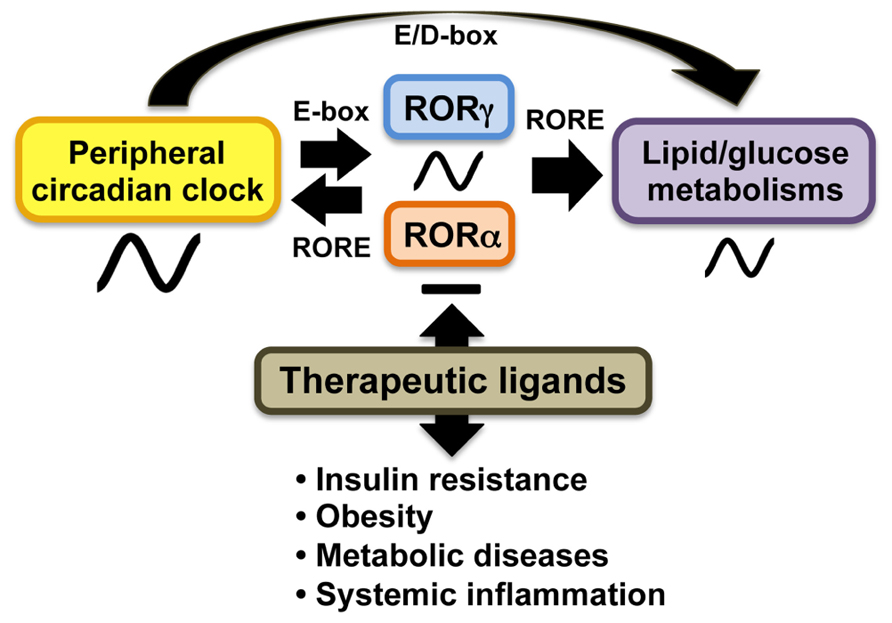
FIGURE 2. In peripheral tissues, RORs function as intermediate regulators providing a link between clock proteins and their regulation of lipid and glucose metabolism. The oscillatory pattern of expression of RORγ is regulated by circadian clock proteins Bmal1 and Clock or Npas2 through E-box, whereas RORα does not exhibit a strong oscillatory pattern of expression. In turn, RORγ activates expression of several clock genes through ROREs together with RORα. Both RORα and RORγ regulate the circadian pattern of expression of target genes involved in lipid/glucose metabolism. ROR (ant)agonists may modulate the amplitude of peripheral clock oscillation of these metabolic outputs and might be useful in the treatment of insulin resistance, obesity, and tissue inflammation.
RORs as Therapeutic Targets for Metabolic Syndrome and Insulin Resistance
X-ray crystallography studies of the LBD of RORα identified the presence of cholesterol in the ligand-binding pocket of RORα (Kallen et al., 2002). Subsequent studies identified cholesterol sulfate, 7-dehydrocholesterol, and 25-hydroxycholesterol as RORα agonists (Kallen et al., 2004). All-trans retinoic acid and the synthetic retinoid, ALRT 1550 were reported to bind and function as antagonists for RORβ and RORγ, but not RORα (Stehlin-Gaon et al., 2003). Recently, ursolic acid and several oxygenated sterols, including 7α-hydroxycholesterol (7α-OHC), 7β-hydroxycholesterol, 7-ketocholesterol, and 24S-hydroxycholesterol, were shown to function as inverse agonists to both RORα and RORγ (Wang et al., 2010a; Xu et al., 2011), while 20α-hydroxycholesterol and 22R-hydroxycholesterol acted as agonists (Jin et al., 2010). The LXR agonist T0901317 and several other synthetic derivatives, including SR1001, were identified as RORα and RORγ inverse agonists. Digoxin and several derivatives were identified as specific inhibitors for RORγ transcriptional activity (Fujita-Sato et al., 2011; Huh et al., 2011). The ROR (inverse) antagonists have been reported to repress the expression of ROR target genes and the activation of their promoter regulatory region by inhibiting the recruitment of co-activators. Moreover, ROR antagonists have been shown to inhibit Th17 cell differentiation and IL-17 production both in vitro and in vivo and to suppress the development of experimental autoimmune encephalomyelitis (Huh et al., 2011; Jetten, 2011; Solt et al., 2011). Therefore, antagonists for RORγ might be potential drugs for pharmacological intervention in the treatment and suppression of several autoimmune diseases, including multiple sclerosis, collagen-induced arthritis, rheumatoid arthritis, and asthma (Solt et al., 2010; Huh and Littman, 2012). Because of their role in regulating various features of metabolic syndrome, RORα and γ antagonists might also have beneficial effects in the management of obesity and insulin resistance.
Summary
The study of ROR-deficient mice has clearly demonstrated that RORα and RORγ are important in several physiological processes, including the regulation of several immune responses, lipid/glucose homeostasis, and circadian rhythm. These studies revealed that loss of RORα protects against the development of diet- and age-induced obesity, hepatosteatosis, glucose intolerance, and insulin resistance, while loss of RORγ protects against insulin resistance. These protective effects have been linked to suppression of the expression of multiple proinflammatory and metabolic genes. RORs regulate expression of some of these genes directly by binding ROREs in their regulatory region and in certain cases involves changes in their circadian pattern of expression. Although much progress has been made, what event or which ROR target genes are the primary driving force by which RORs influences WAT-associated inflammation, hepatosteatosis, and insulin resistance needs further study. With the increasing evidence for an interrelationship between the controls of lipid/glucose metabolism, inflammation and circadian rhythm, RORs might functions as intermediaries between the controls. With the discovery of ROR antagonists, RORs may provide a novel therapeutic target in the management of various aspect of metabolic syndrome.
Conflict of Interest Statement
The authors declare that the research was conducted in the absence of any commercial or financial relationships that could be construed as a potential conflict of interest.
Acknowledgments
The authors would like to thank Drs Kristin Lichti-Kaiser and Gary ZeRuth for their comments on the manuscript. This research was supported by the Intramural Research Program of the National Institute of Environmental Health Sciences, the National Institutes of Health [Z01-ES-101586].
References
Ahmed, M., and Gaffen, S. L. (2010). IL-17 in obesity and adipogenesis. Cytokine Growth Factor Rev. 21, 449–453.
Akashi, M., and Takumi, T. (2005). The orphan nuclear receptor RORα regulates circadian transcription of the mammalian core-clock Bmal1. Nat. Struct. Mol. Biol. 12, 441–448.
Asher, G., and Schibler, U. (2011). Crosstalk between components of circadian and metabolic cycles in mammals. Cell Metab. 13, 125–137.
Austin, S., Medvedev, A., Yan, Z. H., Adachi, H., Hirose, T., and Jetten, A. M. (1998). Induction of the nuclear orphan receptor RORγ during adipocyte differentiation of D1 and 3T3-L1 cells. Cell Growth Differ. 9, 267–276.
Barendse, W., Bunch, R. J., Kijas, J. W., and Thomas, M. B. (2007). The effect of genetic variation of the retinoic acid receptor-related orphan receptor C gene on fatness in cattle. Genetics 175, 843–853.
Bertola, A., Ciucci, T., Rousseau, D., Bourlier, V., Duffaut, C., Bonnafous, S., et al. (2012). Identification of adipose tissue dendritic cells correlated with obesity-associated insulin-resistance and inducing Th17 responses in mice and patients. Diabetes 61, 2238–2247.
Bertola, A., Deveaux, V., Bonnafous, S., Rousseau, D., Anty, R., Wakkach, A., et al. (2009). Elevated expression of osteopontin may be related to adipose tissue macrophage accumulation and liver steatosis in morbid obesity. Diabetes 58, 125–133.
Browning, J. D., Szczepaniak, L. S., Dobbins, R., Nuremberg, P., Horton, J. D., Cohen, J. C., et al. (2004). Prevalence of hepatic steatosis in an urban population in the United States: impact of ethnicity. Hepatology 40, 1387–1395.
Castanon-Cervantes, O., Wu, M., Ehlen, J. C., Paul, K., Gamble, K. L., Johnson, R. L., et al. (2010). Dysregulation of inflammatory responses by chronic circadian disruption. J. Immunol. 185, 5796–5805.
Chang, B. H., Li, L., Saha, P., and Chan, L. (2010). Absence of adipose differentiation related protein upregulates hepatic VLDL secretion, relieves hepatosteatosis, and improves whole body insulin resistance in leptin-deficient mice. J. Lipid Res. 51, 2132–2142.
Crumbley, C., Wang, Y., Banerjee, S., and Burris, T. P. (2012). Regulation of expression of citrate synthase by the retinoic acid receptor-related orphan receptor α (RORα). PLoS ONE 7:e33804. doi: 10.1371/journal.pone.0033804
Crumbley, C., Wang, Y., Kojetin, D. J., and Burris, T. P. (2010). Characterization of the core mammalian clock component, NPAS2, as a REV-ERBα/RORα target gene. J. Biol. Chem. 285, 35386–35392.
Cryer, P. E. (2012). Minireview: glucagon in the pathogenesis of hypoglycemia and hyperglycemia in diabetes. Endocrinology 153, 1039–1048.
Donath, M. Y., and Shoelson, S. E. (2011). Type 2 diabetes as an inflammatory disease. Nat. Rev. Immunol. 11, 98–107.
Duez, H., Duhem, C., Laitinen, S., Patole, P. S., Abdelkarim, M., Bois-Joyeux, B., et al. (2009). Inhibition of adipocyte differentiation by RORα. FEBS Lett. 583, 2031–2036.
Duez, H., and Staels, B. (2010). Nuclear receptors linking circadian rhythms and cardiometabolic control. Arterioscler. Thromb. Vasc. Biol. 30, 1529–1534.
Dzhagalov, I., Giguere, V., and He, Y. W. (2004). Lymphocyte development and function in the absence of retinoic acid-related orphan receptor α. J. Immunol. 173, 2952–2959.
Fabbrini, E., Sullivan, S., and Klein, S. (2010). Obesity and nonalcoholic fatty liver disease: biochemical, metabolic, and clinical implications. Hepatology 51, 679–689.
Fujita-Sato, S., Ito, S., Isobe, T., Ohyama, T., Wakabayashi, K., Morishita, K., et al. (2011). Structural basis of digoxin that antagonizes RORγt receptor activity and suppresses Th17 cell differentiation and interleukin (IL)-17 production. J. Biol. Chem. 286, 31409–31417.
Gamboa-Melendez, M. A., Huerta-Chagoya, A., Moreno-Macias, H., Vazquez-Cardenas, P., Ordonez-Sanchez, M. L., Rodriguez-Guillen, R., et al. (2012). Contribution of common genetic variation to the risk of type 2 diabetes in the mexican mestizo population. Diabetes 61, 3314–3321.
Glass, C. K., and Olefsky, J. M. (2012). Inflammation and lipid signaling in the etiology of insulin resistance. Cell Metab. 15, 635–645.
Gong, J., Sun, Z., and Li, P. (2009). CIDE proteins and metabolic disorders. Curr. Opin. Lipidol. 20, 121–126.
Greenberg, A. S., Coleman, R. A., Kraemer, F. B., McManaman, J. L., Obin, M. S., Puri, V., et al. (2011). The role of lipid droplets in metabolic disease in rodents and humans. J. Clin. Invest. 121, 2102–2110.
Guilherme, A., Virbasius, J. V., Puri, V., and Czech, M. P. (2008). Adipocyte dysfunctions linking obesity to insulin resistance and type 2 diabetes. Nat. Rev. Mol. Cell Biol. 9, 367–377.
Guillaumond, F., Dardente, H., Giguere, V., and Cermakian, N. (2005). Differential control of Bmal1 circadian transcription by REV-ERB and ROR nuclear receptors. J. Biol. Rhythms 20, 391–403.
Halim, T. Y., MacLaren, A., Romanish, M. T., Gold, M. J., McNagny, K. M., and Takei, F. (2012). Retinoic-acid-receptor-related orphan nuclear receptor α is required for natural helper cell development and allergic inflammation. Immunity 37, 463–474.
Hoffstedt, J., Arner, E., Wahrenberg, H., Andersson, D. P., Qvisth, V., Lofgren, P., et al. (2010). Regional impact of adipose tissue morphology on the metabolic profile in morbid obesity. Diabetologia 53, 2496–2503.
Huh, J. R., Leung, M. W., Huang, P., Ryan, D. A., Krout, M. R., Malapaka, R. R., et al. (2011). Digoxin and its derivatives suppress TH17 cell differentiation by antagonizing RORγ activity. Nature 472, 486–490.
Huh, J. R., and Littman, D. R. (2012). Small molecule inhibitors of RORγt: targeting Th17 cells and other applications. Eur. J. Immunol. 42, 2232–2237.
Ivanov, I. I., McKenzie, B. S., Zhou, L., Tadokoro, C. E., Lepelley, A., Lafaille, J. J., et al. (2006). The orphan nuclear receptor RORγ directs the differentiation program of proinflammatory IL-17+ T helper cells. Cell 126, 1121–1133.
Jaradat, M., Stapleton, C., Tilley, S. L., Dixon, D., Erikson, C. J., McCaskill, J. G., et al. (2006). Modulatory role for retinoid-related orphan receptor α in allergen-induced lung inflammation. Am. J. Respir. Crit. Care Med. 174, 1299–1309.
Jetten, A. M. (2004). Recent advances in the mechanisms of action and physiological functions of the retinoid-related orphan receptors (RORs). Curr. Drug Targets Inflamm. Allergy 3, 395–412.
Jetten, A. M. (2009). Retinoid-related orphan receptors (RORs): critical roles in development, immunity, circadian rhythm, and cellular metabolism. Nucl. Recept. Signal. 7, e003.
Jin, L., Martynowski, D., Zheng, S., Wada, T., Xie, W., and Li, Y. (2010). Structural basis for hydroxycholesterols as natural ligands of orphan nuclear receptor RORγ. Mol. Endocrinol. 24, 923–929.
Johnson, P., and Ruffell, B. (2009). CD44 and its role in inflammation and inflammatory diseases. Inflamm. Allergy Drug Targets 8, 208–220.
Juge-Aubry, C. E., Somm, E., Giusti, V., Pernin, A., Chicheportiche, R., Verdumo, C., et al. (2003). Adipose tissue is a major source of interleukin-1 receptor antagonist: upregulation in obesity and inflammation. Diabetes 52, 1104–1110.
Kallen, J., Schlaeppi, J. M., Bitsch, F., Delhon, I., and Fournier, B. (2004). Crystal structure of the human RORα ligand binding domain in complex with cholesterol sulfate at 2.2 A. J. Biol. Chem. 279, 14033–14038.
Kallen, J. A., Schlaeppi, J. M., Bitsch, F., Geisse, S., Geiser, M., Delhon, I., et al. (2002). X-ray structure of the hRORα LBD at 1.63 A: structural and functional data that cholesterol or a cholesterol derivative is the natural ligand of RORα. Structure 10, 1697–1707.
Kanda, H., Tateya, S., Tamori, Y., Kotani, K., Hiasa, K., Kitazawa, R., et al. (2006). MCP-1 contributes to macrophage infiltration into adipose tissue, insulin resistance, and hepatic steatosis in obesity. J. Clin. Invest. 116, 1494–1505.
Kang, H. S., Angers, M., Beak, J. Y., Wu, X., Gimble, J. M., Wada, T., et al. (2007). Gene expression profiling reveals a regulatory role for RORα and RORγ in phase I and phase II metabolism. Physiol. Genomics 31, 281–294.
Kang, H. S., Okamoto, K., Takeda, Y., Beak, J. Y., Gerrish, K., Bortner, C. D., et al. (2011). Transcriptional profiling reveals a role for RORα in regulating gene expression in obesity-associated inflammation and hepatic steatosis. Physiol. Genomics 43, 818–828.
Kiefer, F. W., Neschen, S., Pfau, B., Legerer, B., Neuhofer, A., Kahle, M., et al. (2011). Osteopontin deficiency protects against obesity-induced hepatic steatosis and attenuates glucose production in mice. Diabetologia 54, 2132–2142.
Kitade, H., Sawamoto, K., Nagashimada, M., Inoue, H., Yamamoto, Y., Sai, Y., et al. (2012). CCR5 plays a critical role in obesity-induced adipose tissue inflammation and insulin resistance by regulating both macrophage recruitment and M1/M2 status. Diabetes 61, 1680–1690.
Klar, J., Asling, B., Carlsson, B., Ulvsback, M., Dellsen, A., Strom, C., et al. (2005). RAR-related orphan receptor A isoform 1 (RORα1) is disrupted by a balanced translocation t(4;15)(q22.3;q21.3) associated with severe obesity. Eur. J. Hum. Genet. 13, 928–934.
Kodama, K., Horikoshi, M., Toda, K., Yamada, S., Hara, K., Irie, J., et al. (2012). Expression-based genome-wide association study links the receptor CD44 in adipose tissue with type 2 diabetes. Proc. Natl. Acad. Sci. U.S.A. 109, 7049–7054.
Kurebayashi, S., Ueda, E., Sakaue, M., Patel, D. D., Medvedev, A., Zhang, F., et al. (2000). Retinoid-related orphan receptor γ (RORγ) is essential for lymphoid organogenesis and controls apoptosis during thymopoiesis. Proc. Natl. Acad. Sci. U.S.A. 97, 10132–10137.
Lau, P., Fitzsimmons, R. L., Pearen, M. A., Watt, M. J., and Muscat, G. E. (2011). Homozygous staggerer (sg/sg) mice display improved insulin sensitivity and enhanced glucose uptake in skeletal muscle. Diabetologia 54, 1169–1180.
Lau, P., Fitzsimmons, R. L., Raichur, S., Wang, S. C., Lechtken, A., and Muscat, G. E. (2008). The orphan nuclear receptor, RORα, regulates gene expression that controls lipid metabolism: staggerer (SG/SG) mice are resistant to diet-induced obesity. J. Biol. Chem. 283, 18411–18421.
Logan, R. W., and Sarkar, D. K. (2012). Circadian nature of immune function. Mol. Cell. Endocrinol. 349, 82–90.
Maury, E., Ramsey, K. M., and Bass, J. (2010). Circadian rhythms and metabolic syndrome: from experimental genetics to human disease. Circ. Res. 106, 447–462.
Meissburger, B., Ukropec, J., Roeder, E., Beaton, N., Geiger, M., Teupser, D., et al. (2011). Adipogenesis and insulin sensitivity in obesity are regulated by retinoid-related orphan receptor γ. EMBO Mol. Med. 3, 637–651.
Mohawk, J. A., Green, C. B., and Takahashi, J. S. (2012). Central and peripheral circadian clocks in mammals. Annu. Rev. Neurosci. 35, 445–462.
Mongrain, V., Ruan, X., Dardente, H., Fortier, E. E., and Cermakian, N. (2008). Clock-dependent and independent transcriptional control of the two isoforms from the mouse RORγ gene. Genes Cells 13, 1197–1210.
Mühlbauer, E., Bazwinsky-Wutschke, I., Wolgast, S., Labucay, K., and Peschke, E. (2013). Differential and day-time dependent expression of nuclear receptors RORα, RORβ, RORγ and RXRα in the rodent pancreas and islet. Mol. Cell. Endocrinol. 365, 129–138.
Narasimamurthy, R., Hatori, M., Nayak, S. K., Liu, F., Panda, S., and Verma, I. M. (2012). Circadian clock protein cryptochrome regulates the expression of proinflammatory cytokines. Proc. Natl. Acad. Sci. U.S.A. 109, 12662–12667.
Nishimura, S., Manabe, I., Nagasaki, M., Eto, K., Yamashita, H., Ohsugi, M., et al. (2009). CD8+ effector T cells contribute to macrophage recruitment and adipose tissue inflammation in obesity. Nat. Med. 15, 914–920.
Nomiyama, T., Perez-Tilve, D., Ogawa, D., Gizard, F., Zhao, Y., Heywood, E. B., et al. (2007). Osteopontin mediates obesity-induced adipose tissue macrophage infiltration and insulin resistance in mice. J. Clin. Invest. 117, 2877–2888.
Odegaard, J. I., and Chawla, A. (2008). Mechanisms of macrophage activation in obesity-induced insulin resistance. Nat. Clin. Pract. Endocrinol. Metab. 4, 619–626.
Ogden, C. L., Carroll, M. D., Kit, B. K., and Flegal, K. M. (2012). Prevalence of obesity and trends in body mass index among US children and adolescents, 1999–2010. JAMA 307, 483–490.
Ohoka, N., Kato, S., Takahashi, Y., Hayashi, H., and Sato, R. (2009). The orphan nuclear receptor RORα restrains adipocyte differentiation through a reduction of C/EBPβ activity and perilipin gene expression. Mol. Endocrinol. 23, 759–771.
Ouchi, N., Parker, J. L., Lugus, J. J., and Walsh, K. (2011). Adipokines in inflammation and metabolic disease. Nat. Rev. Immunol. 11, 85–97.
Raichur, S., Lau, P., Staels, B., and Muscat, G. E. (2007). Retinoid-related orphan receptor γ regulates several genes that control metabolism in skeletal muscle cells: links to modulation of reactive oxygen species production. J. Mol. Endocrinol. 39, 29–44.
Ramasamy, A., Kuokkanen, M., Vedantam, S., Gajdos, Z. K., Couto Alves, A., Lyon, H. N., et al. (2012). Genome-wide association studies of asthma in population-based cohorts confirm known and suggested loci and identify an additional association near HLA. PLoS ONE 7:e44008. doi: 10.1371/journal.pone.0044008
Raspe, E., Duez, H., Gervois, P., Fievet, C., Fruchart, J. C., Besnard, S., et al. (2001). Transcriptional regulation of apolipoprotein C-III gene expression by the orphan nuclear receptor RORα. J. Biol. Chem. 276, 2865–2871.
Rose, A. J., and Richter, E. A. (2005). Skeletal muscle glucose uptake during exercise: how is it regulated? Physiology (Bethesda) 20, 260–270.
Rudic, R. D., McNamara, P., Curtis, A. M., Boston, R. C., Panda, S., Hogenesch, J. B., et al. (2004). BMAL1 and CLOCK, two essential components of the circadian clock, are involved in glucose homeostasis. PLoS Biol. 2:e377. doi: 10.1371/journal.pbio.0020377
Saltiel, A. R., and Kahn, C. R. (2001). Insulin signalling and the regulation of glucose and lipid metabolism. Nature 414, 799–806.
Samuel, V. T., and Shulman, G. I. (2012). Mechanisms for insulin resistance: common threads and missing links. Cell 148, 852–871.
Sato, T. K., Panda, S., Miraglia, L. J., Reyes, T. M., Rudic, R. D., McNamara, P., et al. (2004). A functional genomics strategy reveals Rora as a component of the mammalian circadian clock. Neuron 43, 527–537.
Solt, L. A., and Burris, T. P. (2012). Action of RORs and their ligands in (patho)physiology. Trends Endocrinol. Metab. 23, 619–627.
Solt, L. A., Griffin, P. R., and Burris, T. P. (2010). Ligand regulation of retinoic acid receptor-related orphan receptors: implications for development of novel therapeutics. Curr. Opin. Lipidol. 21, 204–211.
Solt, L. A., Kumar, N., Nuhant, P., Wang, Y., Lauer, J. L., Liu, J., et al. (2011). Suppression of TH17 differentiation and autoimmunity by a synthetic ROR ligand. Nature 472, 491–494.
Somm, E., Cettour-Rose, P., Asensio, C., Charollais, A., Klein, M., Theander-Carrillo, C., et al. (2006). Interleukin-1 receptor antagonist is upregulated during diet-induced obesity and regulates insulin sensitivity in rodents. Diabetologia 49, 387–393.
Stehlin-Gaon, C., Willmann, D., Zeyer, D., Sanglier, S., Van Dorsselaer, A., Renaud, J. P., et al. (2003). All-trans retinoic acid is a ligand for the orphan nuclear receptor RORβ. Nat. Struct. Biol. 10, 820–825.
Sun, K., Kusminski, C. M., and Scherer, P. E. (2011). Adipose tissue remodeling and obesity. J. Clin. Invest. 121, 2094–2101.
Sun, Z., and Lazar, M. A. (2013). Dissociating fatty liver and diabetes. Trends Endocrinol. Metab. 24, 4–12.
Sun, Z., Unutmaz, D., Zou, Y. R., Sunshine, M. J., Pierani, A., Brenner-Morton, S., et al. (2000). Requirement for RORγ in thymocyte survival and lymphoid organ development. Science 288, 2369–2373.
Takeda, Y., Jothi, R., Birault, V., and Jetten, A. M. (2012). RORγ directly regulates the circadian expression of clock genes and downstream targets in vivo. Nucleic Acids Res. 40, 8519–8539.
Takeda, Y., Kang, H. S., Angers, M., and Jetten, A. M. (2011). Retinoic acid-related orphan receptor γ directly regulates neuronal PAS domain protein 2 transcription in vivo. Nucleic Acids Res. 39, 4769–4782.
Tilley, S. L., Jaradat, M., Stapleton, C., Dixon, D., Hua, X., Erikson, C. J., et al. (2007). Retinoid-related orphan receptor γ controls immunoglobulin production and Th1/Th2 cytokine balance in the adaptive immune response to allergen. J. Immunol. 178, 3208–3218.
Tinahones, F. J., Moreno-Santos, I., Vendrell, J., Chacon, M. R., Garrido-Sanchez, L., Garcia-Fuentes, E., et al. (2012). The retinoic acid receptor-related orphan nuclear receptor γ1 (RORγ1): a novel player determinant of insulin sensitivity in morbid obesity. Obesity 20, 488–497.
Ueda, H. R., Hayashi, S., Chen, W., Sano, M., Machida, M., Shigeyoshi, Y., et al. (2005). System-level identification of transcriptional circuits underlying mammalian circadian clocks. Nat. Genet. 37, 187–192.
Wang, Y., Kumar, N., Crumbley, C., Griffin, P. R., and Burris, T. P. (2010a). A second class of nuclear receptors for oxysterols: regulation of RORα and RORγ activity by 24S-hydroxycholesterol (cerebrosterol). Biochim. Biophys. Acta 1801, 917–923.
Wang, Y., Kumar, N., Solt, L. A., Richardson, T. I., Helvering, L. M., Crumbley, C., et al. (2010b). Modulation of retinoic acid receptor-related orphan receptor α and γ activity by 7-oxygenated sterol ligands. J. Biol. Chem. 285, 5013–5025.
Wang, Y., Solt, L. A., and Burris, T. P. (2010c). Regulation of FGF21 expression and secretion by retinoic acid receptor-related orphan receptor α. J. Biol. Chem. 285, 15668–15673.
Weisberg, S. P., McCann, D., Desai, M., Rosenbaum, M., Leibel, R. L., and Ferrante, A. W. Jr. (2003). Obesity is associated with macrophage accumulation in adipose tissue. J. Clin. Invest. 112, 1796–1808.
Xu, T., Wang, X., Zhong, B., Nurieva, R. I., Ding, S., and Dong, C. (2011). Ursolic acid suppresses interleukin-17 (IL-17) production by selectively antagonizing the function of RORγt protein. J. Biol. Chem. 286, 22707–22710.
Yang, X. O., Pappu, B. P., Nurieva, R., Akimzhanov, A., Kang, H. S., Chung, Y., et al. (2008). T helper 17 lineage differentiation is programmed by orphan nuclear receptors RORα and RORγ. Immunity 28, 29–39.
Keywords: retinoic acid-related orphan receptor, obesity, inflammation, adipose tissue, hepatosteatosis, diabetes, insulin-resistance, circadian rhythm
Citation: Jetten AM, Kang HS and Takeda Y (2013) Retinoic acid-related orphan receptors α and γ: key regulators of lipid/glucose metabolism, inflammation, and insulin sensitivity. Front. Endocrin. 4:1. doi: 10.3389/fendo.2013.00001
Received: 30 November 2012; Paper pending published: 21 December 2012;
Accepted: 05 January 2013; Published online: 25 January 2013.
Edited by:
Tsuguhito Ota, Kanazawa University, JapanReviewed by:
Krzysztof W. Nowak, Pozan University of Life Sciences, PolandVenu Lagishetty, University of South Florida, USA
Copyright: © 2013 Jetten, Kang and Takeda. This is an open-access article distributed under the terms of the Creative Commons Attribution License, which permits use, distribution and reproduction in other forums, provided the original authors and source are credited and subject to any copyright notices concerning any third-party graphics etc.
*Correspondence: Anton M. Jetten, Cell Biology Section, Division of Intramural Research, National Institute of Environmental Health Sciences, National Institutes of Health, 111 T.W. Alexander Drive, Research Triangle Park, NC 27709, USA. e-mail: jetten@niehs.nih.gov