- Department of Engineering and Materials Physics, Institute of Chemical Technology-IndianOil Odisha Campus, Bhubaneswar, India
Recently, metal-halide perovskite nanocrystals (NCs) have shown major development and have attracted substantial interest in a wide range of applications, such as light-emitting diodes (LEDs), solar cells, lasers, and photodetectors due to their attractive properties, such as superior PL emission, a wider range of color tunability, narrow emission spectra, better color purity, low cost, easy solution-processability, and so on. In the past, many color-converting materials, such as III-nitrides, organics, polymers, metal chalcogenides, were investigated for solid-state lighting (SSL) white light-emitting diodes (WLEDs). Still, they suffer from issues such as low stability, low color rendering index (CRI), high correlated color temperature (CCT), low luminous efficiency (LE), and high cost. In this sense, metal-halide perovskite NCs exhibit a better color gamut compared with conventional lighting sources, and production costs are comparatively cheaper. Such materials may offer an upcoming substitute for future color-converting WLEDs. In this review, we discuss the metal halide perovskite NCs and their synthesis protocols. Then we elaborate on the recent progress of halide perovskite NCs as a conversion layer in the application of WLEDs.
Introduction
Solid-state lighting (SSL) is a promising lighting technology where solid-state materials are being used as light sources (Schubert and Kim, 2005; Krames et al., 2007; D'Andrade and Forrest, 2004; Wang et al., 2018a; Dai et al., 2010; Wood and Bulović, 2010). In 1993–1994, Shuji Nakamura first introduced double-heterostructure InGaN/GaN and InGaN/AlGaN as active materials for blue LED chips that were proved to be suitable for commercial applications (Nakamura et al., 1993; Nakamura et al., 1994a; Nakamura et al., 1994b). Since then, major progress has been accomplished in the evolution of III-nitride LEDs (NLEDs), organic LEDs (OLEDs), polymer LEDs, and metal chalcogenide quantum dot LEDs (QDLEDs) (Schubert and Kim, 2005; Krames et al., 2007; D'Andrade and Forrest, 2004; Wang et al., 2018a; Dai et al., 2010; Wood and Bulović, 2010). In recent times, white light-emitting diodes (WLEDs) are very important in our daily life as they are commonly used in homes, streets, malls, TV, and displays. SSL WLEDs are much more efficient than conventional lighting sources, e.g., incandescent bulbs and fluorescence lamps, in terms of energy consumption and lead to tremendous energy saving (Tsao et al., 2010).
The schematic diagram of a LED chip is shown in Figure 1A. Color-converting WLEDs are usually fabricated on a single UV or blue LED chip combined with color conversion layers (see Figures 1B,C) (Schubert and Kim, 2005; Krames et al., 2007; D'Andrade and Forrest, 2004; Wang et al., 2018a; Dai et al., 2010; Wood and Bulović, 2010). The basic operation of a WLED is based on the radiative recombination of holes and electrons produced by the injection of current into the device. The easiest method to fabricate a WLED is to embed yellow-emitting materials onto a 450- to 470-nm blue GaN LED chip. In this LED device configuration, some parts of blue light emitted from the LED chip excite the yellow-emitting materials. The residual blue light combines with the yellow light, which overall generates the white light emission. However, such WLEDs suffer from low CRI and high CCT values due to a lack of red color emission. Also, they suffer from low stability of CCT values under different driving currents (Phillips et al., 2007; Crawford, 2009; Lin et al., 2016; Baekelant et al., 2017). To improve the color quality of the WLEDs, red-green-blue (RGB) color phosphors are settled down on the top of a UV chip. These LEDs demonstrate higher device efficiency, high CRI, better chromatic stability under different driving currents (Schubert and Kim, 2005; Krames et al., 2007; D'Andrade and Forrest, 2004; Wang et al., 2018a; Dai et al., 2010; Wood and Bulović, 2010). However, the instability of CCT also happens in RGB WLEDs due to degradation of different color phosphors or variations of driving current (Phillips et al., 2007; Crawford, 2009; Baekelant et al., 2017).
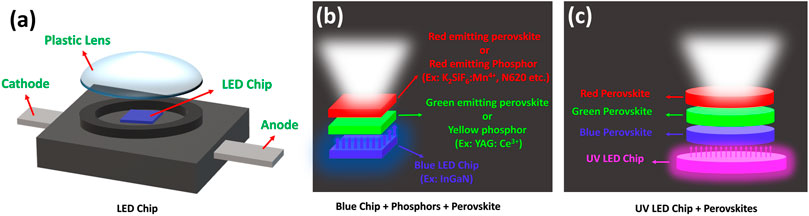
FIGURE 1. Schematic diagrams of (A) a LED chip, (B) WLED with phosphors or perovskite materials integrated onto a blue LED chip, and (C) RGB color-emitting perovskite materials integrated on a UV LED chip.
Next-generation LEDs can be identified as the devices with high efficiency, high color quality, and lower economic and energy costs of manufacturing (Shirasaki et al., 2013; Dou et al., 2020). The III-nitride LEDs have transformed the conventional lighting technologies, but the preparation of these materials relies on high temperature and expensive vacuum-based processing (Mondal et al., 2021). The fabrication of OLEDs is also dependent on vacuum-based sublimation, inappropriate for cost-effective processing on large area (Sasabe and Kido, 2011). The usage of QDLEDs is restricted by large nonradiative recombination processes from the high surface-mediated defect states (Yang et al., 2019). Recently, lead-halide perovskite nanocrystals (NCs) have gathered massive observations toward the fields of LEDs, solar cells, detectors, and lasing due to their tunable wavelength, high photoluminescence quantum yield (QY), narrow emission wavelength, wider color gamut, and better color purity (Kovalenko et al., 2017; Li et al., 2017; Akkerman et al., 2018; Yan et al., 2019; Zhang et al., 2019; Li et al., 2020; Li et al., 2021a). The color gamut of perovskite LEDs exceeds 140% of the National Television System Committee (NTSC) standard, surpassing the commercial OLED and QDLED displays. The high color purity, low turn-on voltage, cost-effectiveness, and low energy consumption of the perovskite NC-based LEDs make them promising candidates for next-generation display technologies. In this review, we execute our key attention toward the properties of metal halide perovskites, the synthesis of perovskite NCs, and their recent progress in WLED applications as a conversion layer.
Characteristics of Metal-Halide Perovskites
Crystal Structure
Metal-halide perovskites have a distinctive crystal structure having the chemical formula in the form of AMX3 [A: organic or inorganic cations (MA = CH3NH3, FA = CH(NH2)2, Cs, Rb); M: divalent metal cations (Pb, Sn); and X: halide anions (Cl, Br, I)] (Veldhuis et al., 2016; Kovalenko et al., 2017; Li et al., 2017; Akkerman et al., 2018; Yan et al., 2019; Li et al., 2020). They can be distinguished into two groups of materials depending on the type of the cations. If the A-site cation involves organic cations (i.e., MA or FA or both), then the perovskite structure belongs to the group of organometallic metal-halide perovskites. On the other hand, if the A-site cation consists of Cs, Rb-cations, then the system is considered all-inorganic metal-halide perovskites. In a three-dimensional (3-D) perovskite unit cell, the A-cations are situated at the eight corners of a [MX6]4- octahedra, the M-cations are located at the body centers, and six numbers of X-anions are placed at the face centers (see Figures 2A,B). The structure of perovskite materials mostly depends on the size of the A-site cation. The larger A-site cations do not fit inside the space and lead to the formation of lower-dimensional perovskite crystal structures. In the case of two-dimensional (2-D) perovskites (A2MX4), the longer cations are located at the A-site positions, and each [MX6]4--octahedron is connected with four neighboring halide anions, which create a 2-D layered network structure as shown in Figure 2C. In the case of one-dimensional (1-D) perovskites (A'AMX5), each octahedron is attached with two opposite corners, which form a series of parallel infinite chains (see Figure 2D). In the case of zero-dimensional (0-D) perovskites (A4MX6), each octahedron is separated by four A-site cations, which behave like an isolated molecule (see Figure 2E).
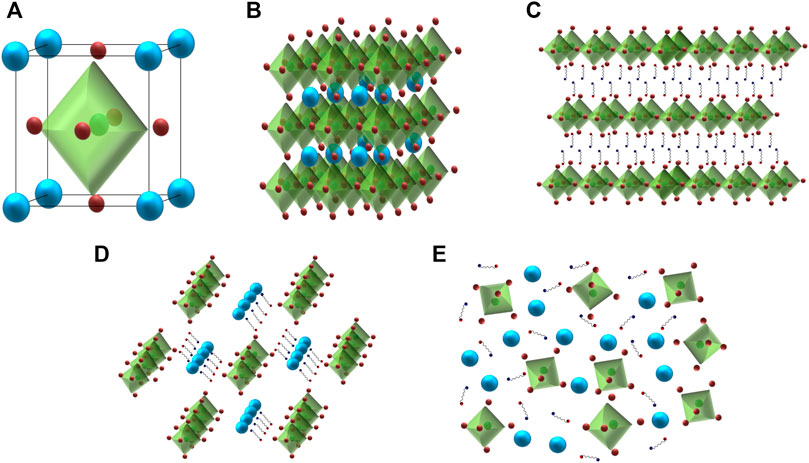
FIGURE 2. Schematic representations of (A) a perovskite unit cell, (B) 3-D, (C) 2-D, (D) 1-D, and (E) 0-D metal halide perovskite structures.
Defect Tolerance and Energy Diagram
Goldschmidt’s tolerance factor is defined as t =
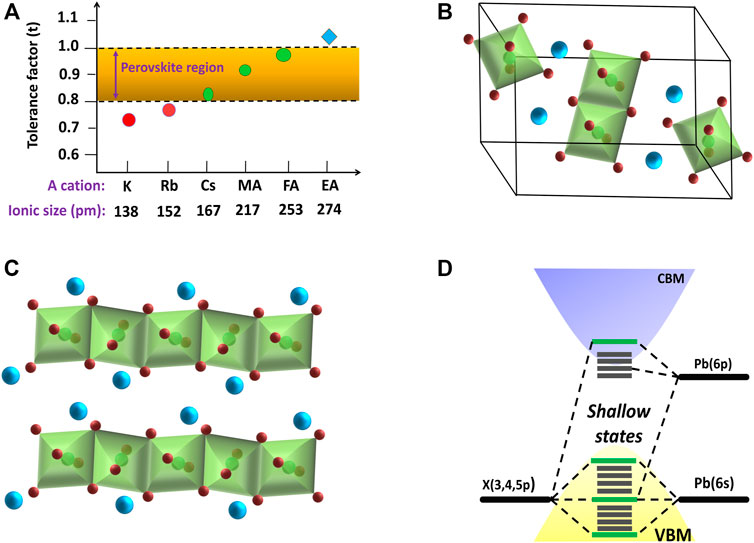
FIGURE 3. (A) Tolerance factor of different cation-based APbI3 perovskites. Schematic diagrams of yellow phase structure of (B) CsPbI3, and (C) FAPbI3. (D) Energy band structure of APbX3 perovskites.
The energy level diagram of lead halide perovskites is dependent on the M-site and X-site ions. From a theoretical point of view, the energy of the emitted photon from perovskite materials can be relatable to the energy difference between the conduction band minimum and the valence band maximum. The valence band for lead halide perovskites is formed by the np orbitals of the X ions and the 6s orbitals of the Pb ions, whereas the conduction band is mainly determined by the Pb-6p orbitals with a minor contribution from the np orbitals of the X ions as shown in Figure 3D (Sum and Mathews, 2014). Henceforth, the absence of bonding–antibonding interaction between these conduction bands and valence bands (VB) results in a high defect tolerance. Thus, the maximum number of defect states lies within the valance or conduction bands instead of in between the bandgap. It has been observed an abrupt change in the bandgap energy with halide substitution in MAPbX3 perovskites, which are 2.97, 2.24, and 1.53 eV for MAPbCl3, MAPbBr3, and MAPbI3, respectively, with slight change in the ionic radii of Cl ions (1.81 Å), Br ions (1.96 Å), and I ions (2.20 Å) (Sum and Mathews, 2014; Correa-Baena et al., 2017). On the other hand, the bandgap energy of the APbI3 perovskites changes very slightly with an exchange in A-site cations [MAPbI3 (1.55 eV) > CsPbI3 (1.5 eV) > FAPbI3 (1.45 eV)], even though the ionic radii of the A-site cation change immensely in the range of 1.8–2.5 Å (Sum and Mathews, 2014; Correa-Baena et al., 2017).
Introduction to NCs
NCs consist of about hundreds to thousands of atoms on the scale of 1–100 nm whose properties are similar to neither the individual atom nor those of the bulk materials (Roduner, 2006; Burda et al., 2005). If the size of NCs is less than the exciton Bohr radius then the energy levels of NCs split to each other due to the quantum size confinement effect and such NCs are defined as quantum dots (QDs). The charge carriers are spatially confined inside the QDs. The photophysical qualities immensely rely on size and shape of the QDs as shown in Figure 4A. A bulk solid contains very few numbers of surface atoms; as a result, broken chemical bonds have a minimal effect on the material properties. However, the smaller the NCs become, the more number of surface atoms combinedly affects the optical qualities of the NCs (Boles et al., 2016; Kazes et al., 2021). Size-dependent emission tunability, high intensity of emission, and narrow emission spectrum allow them to explore considerably in the fields of LEDs, solar cells, lasing, photodetectors, etc. (Shirasaki et al., 2013; Kramer and Sargent, 2014; García de Arquer et al., 2017; Liu et al., 2021).
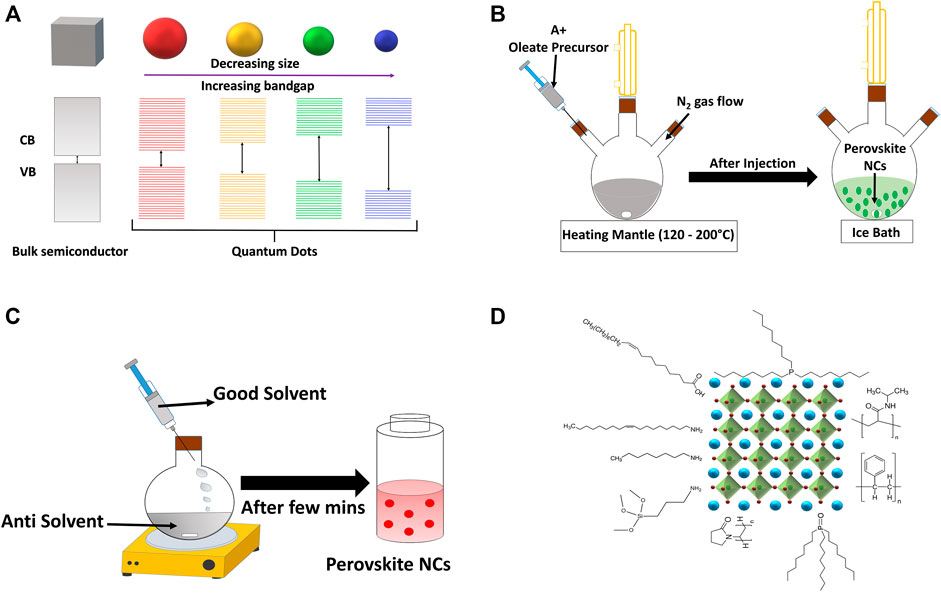
FIGURE 4. (A) Quantum confinement effect in size tunable NCs, schematic representation of colloidal synthesis processes of perovskite NCs by (B) hot injection, (C) LARP, and (D) schematic diagram showing attachment of different ligands to a perovskite NC surface.
Synthesis of Metal Halide Perovskite NCs
Various efforts have been made to develop dependable synthetic strategies for the preparation of high-yielding metal halide perovskite NCs by controlling their shape, restricting their size to nano-range and their optical properties (Kovalenko et al., 2017; Li et al., 2017; Akkerman et al., 2018; Yan et al., 2019; Li et al., 2020). These approaches can be classified either as top-down or as bottom-up methods. The top-down approach defines the fragmentation and structuring of macroscopic solids through a mechanical or chemical approach. At the same time, the bottom-up approach starts with molecules and ions, which proceed via liquid or vapor phase chemical reactions. It is observed that the liquid phase chemical reaction is the best way for synthesizing metal halide perovskite NCs. The two most developed liquid phase synthesis methods of colloidal metal halide perovskite NCs are hot-injection and ligand-assisted reprecipitation (LARP) methods. The hot-injection method requires an inert atmosphere and a high working temperature which makes NC synthesis costly and hence limits its mass production. However, the perovskite NCs can also be synthesized at room temperature via the LARP method that can be employed as the most cost-effective way to grow high-quality perovskite NCs.
Hot Injection Synthesis Strategy
Hot injection synthesis is a strategy where generally the precursor solution is rapidly injected into a hot solution. The hot solution consists of a high boiling solvent containing MX2 halide precursors and ligands (see Figure 4B). (Li et al., 2017; Kovalenko et al., 2017; Akkerman et al., 2018; Yan et al., 2019; Li et al., 2020) This method is generally used for synthesizing NCs that exhibit a narrow size distribution, separating the nucleation and growth stages. Immediately after the injection, a rapid nucleation bursts resulting in the formation of small nuclei. Furthermore, due to the rapid depletion of monomers, the nucleation stage terminates and the nuclei starts growing over time and eventually forming NCs with narrow size distribution. The first report on metal halide perovskite synthesis via the hot-injection method was reported in 2015 by Protesescu et al. for the colloidal synthesis of CsPbX3 NCs (Protesescu et al., 2015). The NCs were synthesized by injecting the Cs-oleate precursor into a hot solution of PbX2 (X = Cl, Br, I) salts at 140–200°C, which were dissolved in octadecene (ODE) along with carboxylic acids and primary amines. The absorption and PL spectra of the resulting NCs are given in Figures 5A,B. The NCs are cubic in shape as shown in Figure 5C. It was observed that monodisperse NCs depend on equal ratios of acids and amines and the size can be adjusted by simply changing the reaction temperature. Mixed-halide perovskite NCs can also be easily synthesized by merely changing chemical proportions of the halide salts (Akkerman et al., 2015; Nedelcu et al., 2015). PL emission of the resulting NCs could be finely adjusted across the entire visible spectrum (410–700 nm) by varying the halide composition and tuning the size of the NCs. Consequently, the hot-injection method was extended to FAPbX3 (X = Br, I) NC systems by replacing Cs-oleate with formamidinium-oleate (Protesescu et al., 2016). In view of this, with passing years, different organic and inorganic metal halide perovskites have been synthesized by this method with control over size and shape of resulting NCs by varying the reaction time, ligands, temperature, and precursor concentrations (Pan et al., 2016; Sun et al., 2016).
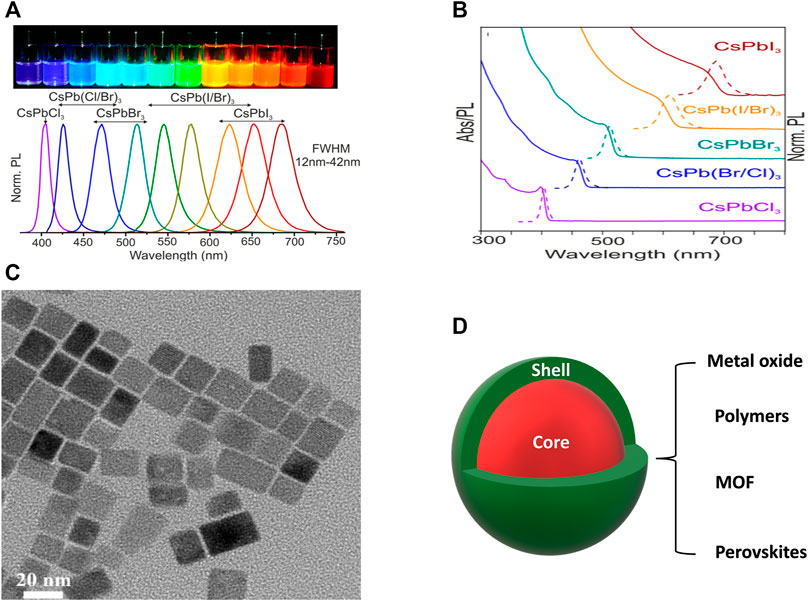
FIGURE 5. (A) PL spectra of different halide perovskite NCs (Inset: photograph of the corresponding NCs in solution form under a UV lamp). (B) Absorption, PL spectra, and (C) TEM image of all inorganic CsPbX3 perovskite NCs. (D) Schematic diagram of a core@shell NC structure. (A,B) are reproduced with permission from Protesescu et al. (2015). Copyright 2015, American Chemical Society. (C) is reproduced with permission from Chen et al. (2021a). Copyright 2021, Elsevier B.V. 3.1.2.
LARP Synthesis Method
LARP is merely a simple process compared with the hot-injection method, which consists of dissolving the respective ions in a good solvent up to a certain equilibrium concentration and then transferring the solution into a state of nonequilibrium, i.e., supersaturation (see Figure 4C) (Kovalenko et al., 2017; Li et al., 2017; Akkerman et al., 2018; Yan et al., 2019; Li et al., 2020). The supersaturated state can be raised by varying the temperature, i.e., cooling down the solution, by evaporating the solvent, or by adding a miscible cosolvent in which the solubility of the ions is low. Under these conditions, spontaneous precipitation and re-crystallization reactions occur until the system reaches an equilibrium state. If this procedure is implemented along with ligands, then this process is referred to as LARP method. The desired organic or inorganic salts (MAX, CsX, or PbX2) are dissolved in suitable polar solvents, i.e., dimethylformamide (DMF) or dimethylsulfoxide (DMSO), and then the precursor solution is injected in poor solvents such as toluene, hexane, or octane, along with suitable ligands or any other capping reagents. When the precursor solution is injected into the poor solvent, the mixture results in a supersaturation instantly, inducing the growth and nucleation of perovskite NCs. It is to be noted that the LARP procedure can be implemented under normal atmospheric conditions by using simple chemical equipment and can be easily multiplied, which allows production of metal halide perovskite NCs on a large scale. Also, these NCs are well distributed and possess a narrow size distribution. The first report on the LARP synthesis of organic–inorganic MAPbBr3 NCs was presented by Schmidt et al.; the perovskite precursor salts were mixed in DMF and injected dropwise in toluene. It formed moderately luminescent green NCs with sizes 6 nm (Schmidt et al., 2014). Later, various color-tunable and highly luminescent perovskite NCs were synthesized via the LARP method (Kovalenko et al., 2017; Li et al., 2017; Akkerman et al., 2018; Yan et al., 2019; Li et al., 2020).
Stability Issues in Metal Halide Perovskites
The instability of metal halide perovskites remains a major issue that needs to be tackled soon because the perovskite structures readily undergo chemical degradation, resulting in optical losses (Park and Seok, 2019; Zhao and Park, 2015; Zhou and Zhao, 2019). They degrade very easily due to their ionic nature. These perovskite crystals suffer from chemical instability against moisture, oxygen, and polar solvents. The attachment of ligands on the surface of NCs is not very strong. They can detach effortlessly, which results in agglomeration of NCs via an oriented attachment process (Baranov et al., 2021; Bhaumik, 2019). This leads to transformation into larger crystals and losses of NC properties in the nanometer regime. Also, mixing different compositions of perovskite NCs leads to an anion-exchange process, resulting in the shifting in the PL emission, deteriorating their initial optical stability. Recently, various research progress has been achieved to improve the NC stability through compositional engineering, development of the 2-D structures interlinked by long-chain organic molecules (see Figure 4D) (Kamat et al., 2020; Ahmed et al., 2021; Kar et al., 2021). An encapsulating shell over the perovskite NCs is a suitable approach to improve the stability from the harsh external atmosphere and enrich the core’s optical properties.
Encapsulation of Perovskite NCs With Different Shelling Materials
The term encapsulation is usually referring to coating of a protective layer around the NCs during or after the synthesis. The aim is to achieve homogenous dispersion of emitting NCs into a single or a matrix of protective shell to reduce agglomeration and quenching of PL. Many approaches have been made by coating the perovskite NC core with some acceptable coating materials such as various metal oxides, metal-halide perovskite, metal organic framework, polymer (see Figure 5D).
Zheng and his coworkers synthesized silica-encapsulated MAPbBr3. They prepared PbBr2, MABr, and APTES precursors mixed in DMF which were injected into toluene. Silica-coated MAPbBr3 NCs were synthesized, followed by the hydrolysis and condensation of tetraethyl orthosilicate (TEOS), forming a silica coating. The PL peak with quantum yield 60.3% was obtained at 523 nm (Zeng et al., 2018). Yang and his coworkers reported an approach in which they used aminopropyl trimethoxy silane (APTMS) in place of aminopropyl triethoxy silane (APTES) to form orthorhombic MAPbBr3@SiO2 (Yang et al., 2018). The orthorhombic phase of NCs was stable after silica encapsulation and was highly luminescent. The PL emission peak was obtained at 527 nm and a QY of 78%. The shape of the NCs was spherical with an average diameter of 2.8 nm. Other than silica, titanium oxide (TiO2) has also been used as an oxide material to encapsulate on the surface of CsPbBr3 NCs (Li et al., 2018). According to the analysis they observed a decrease in PL intensity due to the type-II band alignment of these core@shell NCs than that of normal CsPbBr3 NCs. CsPbBr3@TiO2 NCs had an orthorhombic phase and exhibited PL peak at 520 nm. The NCs were stable for 12 weeks in aqueous media, demonstrating high water stability of these core@shell NCs.
Bhaumik and his coworkers reported a unique approach for synthesizing MAPbBr3 core and octylammonium lead bromide (OA)2PbBr4 shell NCs (Bhaumik et al., 2016). The molar ratios of MABr and OABr were varied to obtain different thicknesses of shell layer around the core. The pure MAPbBr3 NCs showed an absorption peak at 513 nm and a PL peak at 521 nm with a QY of 84%. With an increase in the concentration of OABr, additional peaks were seen at lower wavelength in the absorption spectra. The PL emission peaks were also shifted toward the lower wavelength side, confirming the presence of a layered perovskite structure over the surface of core. The 8:2 M ratio of MABr and OABr gave the best QY of 92% with impressive stability for above 2 months under a normal atmospheric condition. The average size of NCs observed from the TEM images was in the range of 5–12 nm. Novel CsPbBr3@CsPbBrx core@shell NCs were synthesized via the hot-injection method (Wang et al., 2018b). The size of NCs was obtained as 6 nm, while the size of core was 2 nm. The NCs showed an emission peak at 463 nm with QY of 84%, which was much higher than the pure CsPbBr3 NCs (QY∼ 54%). The protective amorphous CsPbBrx shell helps the excited charge carriers to accumulate inside the core and improve the NC luminescence intensity. This shell also protects from direct contact with harmful atmospheric oxygen and water molecules that degrade the perovskite structure. Another report of CsPbX3@Cs4PbX6 core@shell NCs was synthesized via the hot-injection method (Jia et al., 2018). The core NCs were cubic in shape with an edge width of 7.2 nm. However, with Cs4PbBr6 shelling, the width of the core@shell NCs increased. The PL peak was obtained at 516 nm with a QY of 96.2% compared with the QY 84.4% of core NCs.
Besides metal oxides and perovskite shells, MOFs are another suitable shelling material for perovskite NCs forming composites since they have a very high surface area. Kong and his coworkers synthesized CsPbBr3@Zerolite imidazole framework photocatalyst, which then showed enhanced CO2 reduction compared with normal CsPbBr3 (Kong et al., 2018).
Coating NCs with suitable polymers is another way of increasing material stability. For the effective passivation of the NC surface, the preferred polymer can be introduced at the synthesis stage or post synthesis eventually forming a polymer shell or a matrix around the NCs. The selectivity of the polymer is generally based on the final material’s specific advantages, which leads to flexibility, hydrophobicity, or biocompatibility. Raja et al. reported that the poly(styrene-ethylene-butylene-styrene) (SEBS) was mixed with NCs (CsPbBr3), which significantly improved the water stability for nearly 4 months (Raja et al., 2016). Also, the samples showed more than 200 times less Pb atoms than the one that contained unprotected NCs. They also studied the interaction of poly(lauryl methacrylate) (PLMA) with these NCs. The composites were highly stable in an ambient environment and partially soluble in an aqueous medium. Huang and his coworkers reported CsPb(Cl/Br)3 NCs dispersed with ethyl-cellulose, spin-coated onto optical glass (Huang et al., 2019). The stability of the NCs was more than 5 days under environmental conditions. Wang and his coworkers coated MAPbBr3 NCs with various polymers such as polymethyl methacrylate (PMMA), polyvinyl chloride (PVC), cellulose acetate, acetonitrile butadiene styrene (ABS), polycarbonate (PC), and polystyrene (PS) and studied their optical properties under different conditions (Wang et al., 2016). The polymer-coated film was prepared by the swelling and de-swelling technique, which enables polymer dissolution in suitable solvents. During the evaporation process, shrinkage in polymers occurs, forming a strong binding on the NC layer. Then the water stability of the films in the above-discussed polymer matrices was performed. A slight decrease in QY was observed after boiling the polycarbonate and polystyrene-coated films for 30 min. In addition, polycarbonate films can withstand up to 180°C exhibiting a high thermal stability. Zhang et al. synthesized NCs in micro-hemispheres of polystyrene matrix (Zhang et al., 2017). The polymer PVP was added to perovskite precursors to passivate the surface and further the PVP-passivated NC solution was injected into the PS solution. Owing to the interfacial tension, PS molecules aggregate into spherical micelles with NCs trapped inside them. The spherical micelles form micro-hemispheres when the resulting solution is dropped onto a quartz substrate under the effect of gravity.
Key Parameters of WLEDs
The major parameters for the characterization of LED to be considered when developing luminescent materials for WLEDs are CCT, CRI, LE, and CIE 1931 (x,y) color space.
CCT is meant to identify the color temperature of any light source (Wood and Bulović, 2010). Ideally, a pure white light source should have a CCT value between 2500 and 6500 K. Light sources with CCT values above 5000 K represent bluish-white light sources, whereas temperatures below 4000 K signify yellowish light sources (i.e., incandescent lamps). However, it is difficult to represent a complex spectral distribution of a light source with a simple CCT number. Therefore, many other parameters are used to define the quality of any light source. CRI is represented as a quantitative measure of a light source to reveal the colors in comparison with natural or standard light sources (Wood and Bulović, 2010). It is measured on a scale of 0–100, where 100 is known as the illumination of a standard light source where daylight and incandescent bulbs are used as typical reference sources. When the CRI value is low, the colors are somewhat saturated (with pale aspect) or too saturated (with vivid appearance). The CRI value for white light is considered to be 80 (Nardelli et al., 2017).
The CIE maps the color of a light source in terms of hue and saturation (Wood and Bulović, 2010). The boundary of the CIE color diagram can be identified by the different saturated hues perceived by the human eye in wavelength range from 380 to 780 nm. The color purity defines the closeness to the boundary of the CIE diagram. The color gamut can be enabled by a display with a triangle where red, green, and blue (RGB) pixels define the color coordinates of the individual pixels. The triangle of RGB-NCs is larger than the International Telecommunication Union HDTV standard, highlighting the advantage of NC emitters. The CIE color coordinates for WLED must have lied close to the value of (0.33, 0.33) (Wood and Bulović, 2010). LE is defined as a measurement of brightness based on a standardized model of the human eye’s sensitivity, which can be measured by the ratio of luminous flux to input power (Lumens/Watt in SI unit). High LE requires high light extraction from an LED package which should be in between 20 and 60 Lumens/Watt for an efficient WLED. The antisenility of phosphors and epoxy can directly influence the lifespan of WLEDs.
Utilization of Metal Halide Perovskites in WLED Applications
Blue LED + Red/Yellow Phosphor + Metal Halide Perovskite NCs
In WLED applications based on red/yellow phosphor, the generation of white light generally has low color-rendering index as the spectrum has less green/red components, respectively. Henceforth, red and green halide perovskite NCs can improve the color-rendering index and reduce the color temperature values by placing them onto a phosphor-converted WLED.
In the context of this, Xu and his coworkers reported the synthesis of CsPbBr3 NCs via the hot-injection method, where olive oil was used in the place of oleic acid as a surfactant (Xu et al., 2019). QY was achieved up to 93%. The WLED was fabricated by mixing the CsPbBr3 NCs with K2SiF6:Mn4+ phosphors that were blended with silicone resin A and silicone resin B in the ratio of 1:1 and then sintering at 120°C for 30 min. Finally, the mixture was directly coated onto a blue LED chip (453 nm). Under an operating voltage of 3 V, the WLED exhibited a high CRI of 85, an LE of 46 Lumens/Watt, a CIE of (0.34, 0.31), a CCT of 4754 K, and a color gamut of 118% of NTSC standard. Xuan and his coworkers reported the synthesis of CsPbBr3 nano-crystals synthesized via hot injection and then embedded into super-hydrophobic porous organic polymer frameworks (CsPbBr3@SHFW) (Xuan et al., 2019). The composites exhibited a QY of 60% and showed better water stability due to super hydrophobicity. The respective WLED was fabricated by encapsulating a mixture of CsPbBr3@SHFW composites, KSF phosphors, and silicone resin onto a blue InGaN LED (447 nm). It exhibited a high LE of 50 Lumens/Watt at a driving current of 20 mA. The corresponding EL spectrum consisted of three emissions peaks centered at 447 nm, 525 nm, and 630 nm. The WLED showed a CIE value of (0.329, 0.305) and a color gamut of 127% of NTSC standards.
Furthermore, Chen and his coworkers reported the synthesis of CsPbX3 NCs by using peanut oil as a ligand source (Chen et al., 2019). QY of the NCs was obtained as high as 96.9%. First, the green-emitting CsPbBr3 PNCs, red-emitting (Sr, Ca) AlSiN3:Eu2+ phosphors, and epoxy resin were mixed. This mixture was then coated on a GaN blue LED chip to complete the fabrication of a WLED. The WLED exhibited a white light emission which showed a CIE value of (0.4050, 0.3985), a CRI value of 81.1, and a CCT value of 3529 K. Xu and his coworkers prepared CsPbX3 NCs via a hot injection method. They studied the phase transition from monoclinic to cubic CsPbX3 NCs, which helped to understand the growth and synthetic kinetics of these NCs better (Xu et al., 2020). The obtained QY was 99.8%. They fabricated a WLED by combining green-emitting NCs with red-emitting KSF phosphors with silicone resin. The anhydride curing agent in the ratio of (1:2) was coated onto a blue LED (453 nm) and then thermally cured at 120°C for 2 h. The WLED showed the CIE value of (0.389, 0.376) at a driving current of 20 mA with a wide color gamut of 123% compared with NTSC standards. Furthermore, Li et al. synthesized and encapsulated porous Y2O3 nanoparticles (P-Y2O3) on CsPbBrI2 PNCs using a porous-nanoparticle-assisted dispersion strategy (Li et al., 2022). The corresponding WLED device was fabricated by combining the as-prepared CsPbBrI2/Y2O3 composite and YAG: Ce3+ yellow phosphor on an InGaN blue chip (460 nm). The fabricated WLED provided white light emission at a driving current of 5 mA. Two discrete peaks were observed for composite-based LED in the blue and green-to-red regions. The EL spectra of the WLEDs are shown in Figure 6A. As a result, the CIE value of the fabricated WLED was (0.339, 0.354) with a CCT value of 5049 K (see Figure 6C). The LE of the as-prepared WLED was obtained at 61 Lumens/Watt with a CRI of 83. Besides the 3-D perovskites, Fang et al., in 2021, reported a 2-D layered perovskite with high quantum yields and enhanced stability. They synthesized Mn-doped PEA2(Pb/Mn)(Br/I)4 PNCs by anion exchange surface engineering. They achieved a QY of 52%, which was comparable to most of the reported red-emitting phosphors (Fang et al., 2021). They fabricated a WLED by using commercial (Sr, Ba)2SiO4:Eu phosphor and PEA2(Pb/Mn)(Br/I)4. The mixture was then dispersed in PMMA/toluene solution and coated onto a quartz glass. These color converters were finally incorporated onto a blue LED (440 nm) chip. The corresponding WLED had a white light emission (see Figure 6B) with a CIE value obtained at (0.34, 0.33).
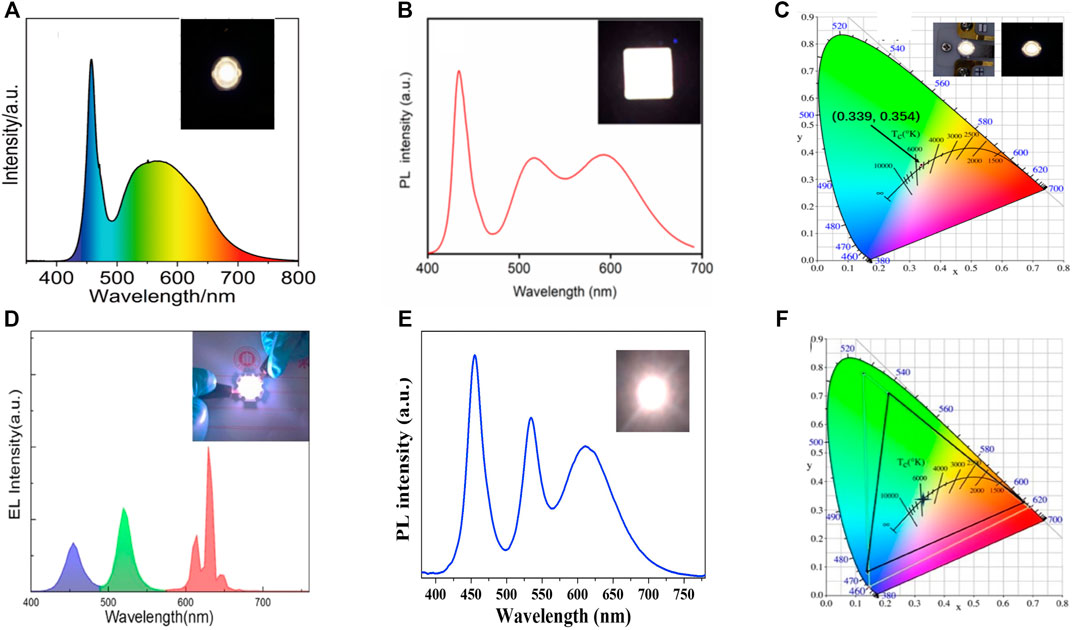
FIGURE 6. (A) EL spectrum of CsPbBrI2/Y2O3 composite-based WLED. (B) EL spectra of WLED based on (Sr,Ba)2SiO4:Eu phosphor and PEA2(Pb/Mn)(Br/I)4. (Inset of a and b is the photograph of WLED under the external bias.) (C) CIE coordinates of CsPbBrI2/Y2O3 composite-based WLED. (D) EL spectrum based on Zn-doped CsPbBr3 NCs, KSF phosphors, and a blue LED chip (the inset photograph shows the WLED under working condition). (E) EL spectra of WLED fabricated by depositing the mixture of CsPbBr3-AlSt3 composites and nitrate phosphors. (F) The color coordinate and the color gamut of as-fabricated WLED based on Zn-doped CsPbBr3 NCs, KSF phosphors, and a blue LED chip. (A,C) are reproduced with permission from Li et al. (2022). Copyright 2021, Elsevier Ltd. (B) It is reproduced with permission from Fang et al. (2021). Copyright 2021, Elsevier B.V. (D,F) These are reproduced with permission from Chen et al. (2021a). Copyright 2021, Elsevier B.V. (E) It is reproduced with permission from Shu et al. (2021). Copyright 2021, Elsevier B.V.
Later, Shu et al. incorporated CsPbX3 PNCs in an aluminum stearate (AlSt3) matrix via the co-precipitation approach (Shu et al., 2021). AlSt3 used as a hydrophobic shell to protect CsPbBr3 PNCs from the environment. These shells passivate the PNC surface via the coordination bonding formed between St-Cs and St-Pb and bonding between the Al3+ and Br−ions. They fabricated a WLED combining CsPbBr3@AlSt3 composites on a N620 phosphors. Epoxy was deposited on a blue-emitting InGaN chip. As a result, the device obtained bright white light with a CRI (Ra) of 90, a CCT (Tc) of 4929 K, and a CIE of (0.34, 0.32) (see Figure 6E). The crafted WLED possessed excellent Ra compared with commercially used WLEDs with low Ra (<80). Furthermore, there was no significant change in Ra and Tc after continuous operation of 24 h. Renjie Chen et al. synthesized CsPbBr3 NCs and doped them with zinc ions to improve their optical properties and stability due to the enhancement in the formation of energy and surface passivation (Chen et al., 2021a). The doped NCs were synthesized via the hot-injection method achieving a QY of 91.3%. The respective WLED was fabricated by combining the Zn-doped CsPbBr3 NCs and K2SiF6:Mn6+ phosphors onto a blue LED chip exhibiting LE of 36 Lumens/Watt (see Figure 6D) with a CIE value obtained at (0.327, 0.336), as shown in Figure 6F. A CCT value of 5760 K and a wide color gamut of 137% of the NTSC standard were obtained.
Li and his coworkers reported the room-temperature synthesis of silica-coated di-dodecyl dimethylammonium bromide (DDAB)-capped CsPbBr3 NCs (Li et al., 2021b). The DDAB-coated CsPbBr3 encapsulated by SiO2 NC composites exhibited an improved QY and a higher stability in ethanol and heat than that of pure CsPbBr3 and DDAB-capped CsPbBr3 (see Figure 7A). As a result, the WLED was fabricated by depositing green DDAB-capped CsPbBr3 encapsulated by SiO2 NC composites and red InAgZnS NCs as a color-conversion layer on a blue LED for warm white WLEDs. Figures 8A–C show the PL spectra of WLEDs based on DDAB-capped CsPbBr3, DDAB-capped CsPbBr3 encapsulated by SiO2 NCs, and pure CsPbBr3 at a voltage of 2.6 V, respectively. The PL intensities of DDAB-capped CsPbBr3 and DDAB-capped CsPbBr3 encapsulated by SiO2 were more than the pure CsPbBr3, for which there was a shift in color coordinates toward green, resulting in a better CRI of 86, a CIE value of (0. 41, 0.38) of DDAB-CsPbBr3/SiO2 composites. The CCT value of 3209 K and a high-power efficiency of 63.4 Lumens/Watt at a driving voltage of 3 V for DDAB-CsPbBr3/SiO2 composites, while those based on DDAB-CsPbBr3 showed a low CRI of 73.
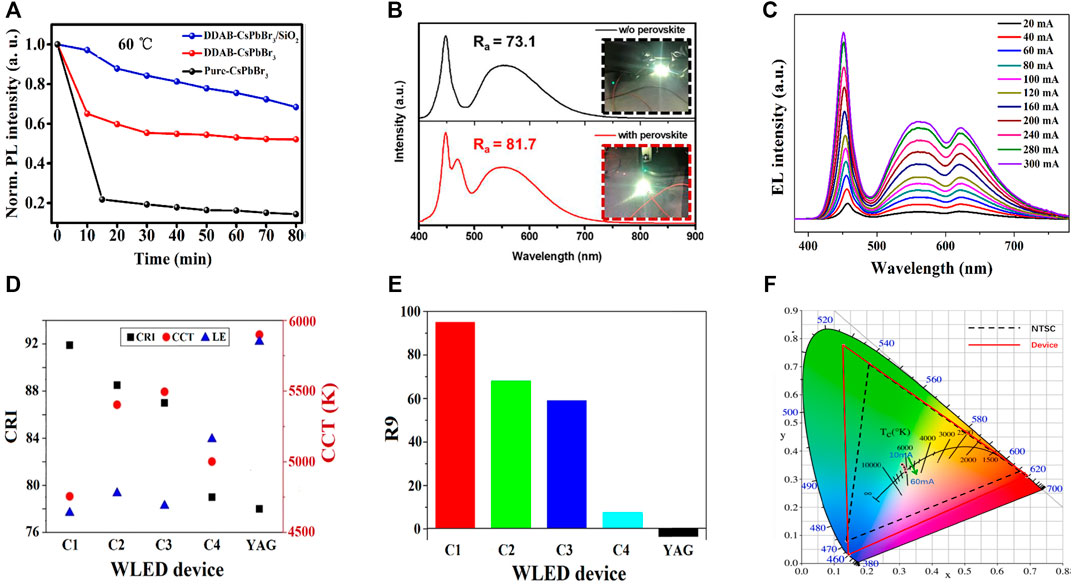
FIGURE 7. (A) PL intensities of pure CsPbBr3 QD, DDAB-CsPbBr3 QD, and DDAB-CsPbBr3/SiO2 QD composites under a continuous heating at 60°C as a function of heating time. (B) Emission spectra of WLEDs based on PEABr and TmMA-modified (MA)x(DMA)PbBr3+x. (Inset: photographs of corresponding working WLEDs). (C) CRI, CCT, and LE of WLEDs C1 to C4 and YAG:Ce. (D) EL spectra of C1-based WLED operated at working currents of 20–300 mA. (E) R9 of WLEDs C1 to C4 and YAG:Ce. (F) Color gamut of the white LED based on PQD solid powders and red K2SiF6:Mn4+ phosphor with a blue LED. (A) It is reproduced with permission from Li X. et al. (2021). Copyright 2021, Elsevier B.V. (B) It is reproduced with permission from Shen et al. (2021). Copyright 2021, Elsevier B.V. (C–E) These are reproduced with permission from Tang et al. (2022). Copyright 2021, Elsevier B.V. (F) It is reproduced with permission from Chen et al. (2021b). Copyright 2021, Elsevier Ltd.
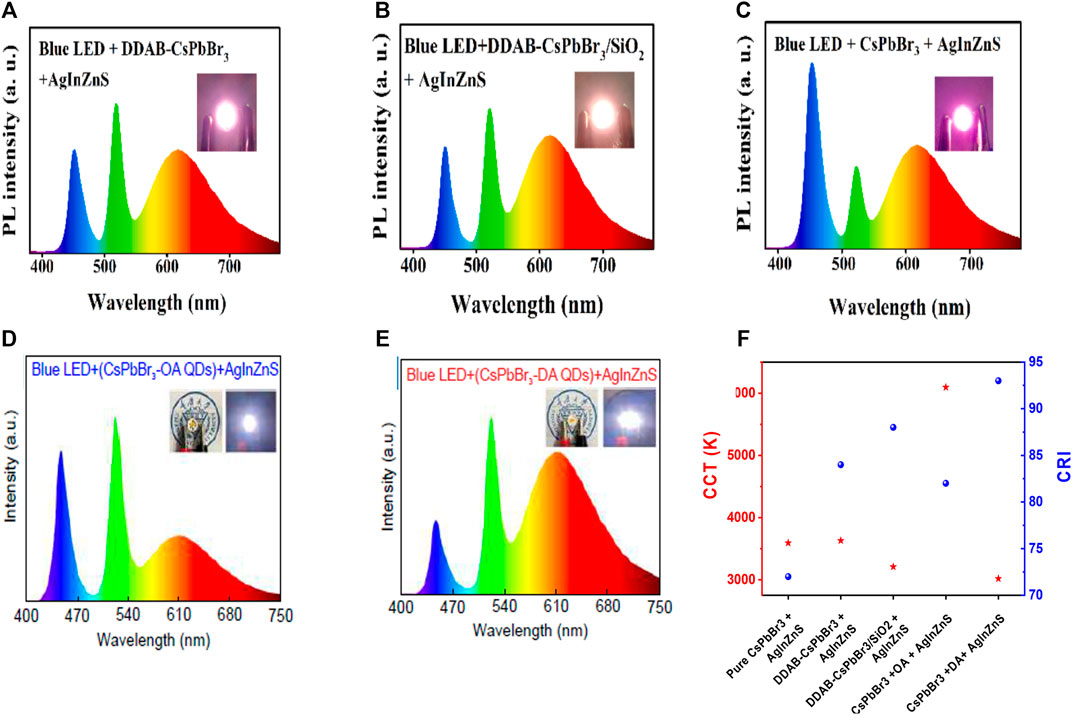
FIGURE 8. EL spectra of WLEDs based on combination of (A) DDAB-CsPbBr3 and AgInZnS QDs, (B) DDAB-CsPbBr3/SiO2 and AgInZnS QDs, and (C) pure CsPbBr3 and AgInZnS QDs. (The inset of a, b, c show photographs of working WLEDs at a driving voltage of 2.6 V.) EL spectrum of WLEDs based on (D) CsPbBr3-OA and AgInZnS QDs, and (E) CsPbBr3-DA and AgInZnS QDs. (Inset shows photographs of the devices without and with driving voltage.) (F) Comparative CCT and CRI of all above-mentioned materials. (A–C) are reproduced with permission from Li X. et al. (2021). Copyright 2021, Elsevier B.V. (D,E) are reproduced with permission from Dongdong et al. (2022). Copyright 2022, OEA.
Yan et al. reported ligand-modified CsPbBr3 NCs where they used 2-hexyldecanoic acid (DA) so as to replace the normal oleic acid (OA) (Li et al., 2021a; Dongdong et al., 2022). Furthermore, two WLEDs were constructed by combining the ligand-modified CsPbBr3 NCs (OA/DA) with AgInZnS NCs on InGaN blue chip. The EL spectra of OA-capped CsPbBr3, and DA-capped CsPbBr3 are shown in Figures 8D,E (the inset images show the cold white and warm white LED of OA and DA-capped CsPbBr3). The DA-capped CsPbBr3 showed the CIE of (0.44, 0.42), a high CRI of 93, and an LE of 64.8 Lumens/Watt. A comparative study of both CRI and CCT values of all the composites from Figures 8A–E is shown in Figure 8F. This comparative study provides a clear understanding between DDAB-capped CsPbBr3 and OA/DA-capped CsPbBr3-fabricated white LED from pure CsPbBr3. It is being noticed that a high CRI value (93) and a lower CCT value (3018 K) were obtained for DA-capped CsPbBr3. Later, Shen et al. reported a WLED based on a cyan-emitting (trimethylsilyl)methylamine (TmMA)-modified (MA)x(DMA)PbBr3+x lower-dimensional perovskite with cyan emission (Shen et al., 2021). They achieved a QY up to ∼87.8%. The WLEDs were fabricated with the commercial YAG: Ce phosphor, CaAlSiN3:Eu2+ red phosphors, and cyan-emitting perovskite applied onto a blue LED InGaN chip. The WLED exhibited white light emission with a CIE value at (0.31, 0.34) with a CCT value of 6677 K. The CRI value was 81.7 of TmMA-modified cyan-emitting perovskite nano-crystals, much higher than the conventional WLEDs, i.e., without the TmMA-modified perovskite material (CRI∼ 73.1) as shown in Figure 7B. The WLED also exhibited an internal quantum efficiency (IQE) of nearly 68.5%.
In addition to the previous reports, Zhao et al. synthesized MAPbBr3 PNCs and encapsulated them with hexagonal boron nitride (h-BN) to form h-BN/MAPbBr3 PNC nanocomposites by the LARP approach (Zhao et al., 2022). Due to the excellent thermal conductivity and the layered structure of the h-BN material, the nanocomposite powder exhibits excellent thermal and moisture stability with a QY of 93.7%. As a result, the fabricated WLEDs with h-BN/MAPbBr3 PNC nanocomposite, a commercial red phosphor, and a blue phosphor possessed CIE value of (0.35, 0.34), a CCT value of 4742 K, and 63.1 Lumens/Watt LE at a driving current of 20 mA. The color gamut of the fabricated WLED was 99.8% of the NTSC standard. The CRI value was found to be 75.
Tang et al. proposed a one-pot anchoring process in which they synthesized MAPbBr0.5I2.5 NCs and formed a composite with YAG:Ce (Tang et al., 2022). They have prepared four kinds of MAPbBr0.5I2.5 PNC@A-YAG composite by changing the amount of A-YAG and without A-YAG named C1, C2, C3, and C4, respectively. A WLED was fabricated by combining InGaN chip and A-YAG combined NC composite. Figure 7C represents the EL intensities of both YAG and PNCs increased with current from 20 to 300 mA, indicating that C1 composite exhibited no saturation. The CRI of 92, CCT of 4755 K, and a high R9 of 95 at an operational current of 20 mA were obtained (see Figure 7D). The CIE coordinates for the WLED were obtained at (0.34, 0.32), and an LE was obtained at 23.5 Lumens/Watt. They also performed the color stability against increasing current and concluded no saturation to the blue light within a wide range of working currents. All the optical properties, including CRI, CCT, and LE of the fabricated WLEDs, were observed. From YAG composite to C1–C4 composites, the CRI value was higher, and CCT was lower. But there was a decrease in LE for enhanced red emission. They also noticed that the R9 value corresponds to the CRI of saturated red, which had vital importance in producing warm white light. It was greatly enhanced from −3.5 for YAG to 95 for C1 composite (see Figure 7E).
In 2022, Qi et al. reported a unique approach for synthesizing CsPbI3 PNCs in the borosilicate glass by melting-quenching and heat treatment (Qi et al., 2022). The corresponding WLED was fabricated by combining red CsPbI3 PNC@glass and green commercial phosphor (LuAG: Ce) onto a blue LED chip. They adjusted the ratio of LuAG and red CsPbI3 PNC@glass powder to contribute to bright white-light luminescence. The WLED yielded a CRI of 95, a CCT of 5755 K, and an LE of 95 Lumens/Watt under a driving current of 20 mA. With respect to the previous ones, the silica coating upon the NCs was reported by Zhihao Chen et al. They synthesized CsPbX3 by APTMS as ligands at room temperature (Chen et al., 2021b). The CsPbBr3 was synthesized by the hot-injection method with a QY of 69.2%. They fabricated a white LED by combining the green CsPbBr3 NCs and commercial red K2SiF6:Mn4+ phosphor with a blue LED chip (455 nm). The fabricated WLED emitted white light with CIE coordinates obtained at (0.3142, 0.3448) operated at 60 mA as shown in Figure 7F and an LE of 70.47 Lumens/Watt. Moreover, the color gamut of this WLED covered over 129.4% of the NTSC standard, suggesting that the prepared PNC solid powders possess great potential for the wide color gamut display application.
Similarly, Zhang et al. synthesized CsPbBr3 NCs by introducing a double-terminal ligand 4,4′-azobis(4-cyanovalericacid) (CA) replacing oleic acid (OA) ligand at room temperature. They achieved a QY of 72% (Zhang et al., 2021). The white LED device was fabricated by using green-emitting CsPbBr3-CA, commercial red-emitting K2SiF6:Mn4+ mixed with silicone resin B and silicone resin A with the ratio of 1:1 onto a blue LED chip (460 nm) and sintered for 2 h at 120°C in an oven. The device exhibited warm white light emission with CIE coordinates obtained at (0.33, 0.33) with an LE of 18.9 Lumens/Watt and a CCT value of 5569 K under a driving current of 20 mA and 2.6 V. A wide color gamut of more than 126% of NTSC standard was obtained from the WLED. In the meantime, Du et al. synthesized CsPbBr3/CsPb2Br5@PbBr(OH) nano/microspheres through a water-assisted process by varying water content (Du et al., 2021). These microspheres had ultrahigh stability, and by mixing green-emitting PNC@PbBr(OH) powder and K2SiF6:Mn4+ (KSF) red phosphor on a 460-nm blue chip, a WLED were fabricated, which had a high luminous efficacy of 101.27 Lumens/Watt at 10 mA and CIE value obtained at (0.32, 0.33). The summary of the fabricated WLEDs by using perovskite NCs with blue chip and red/green phosphors is shown in Table 1.
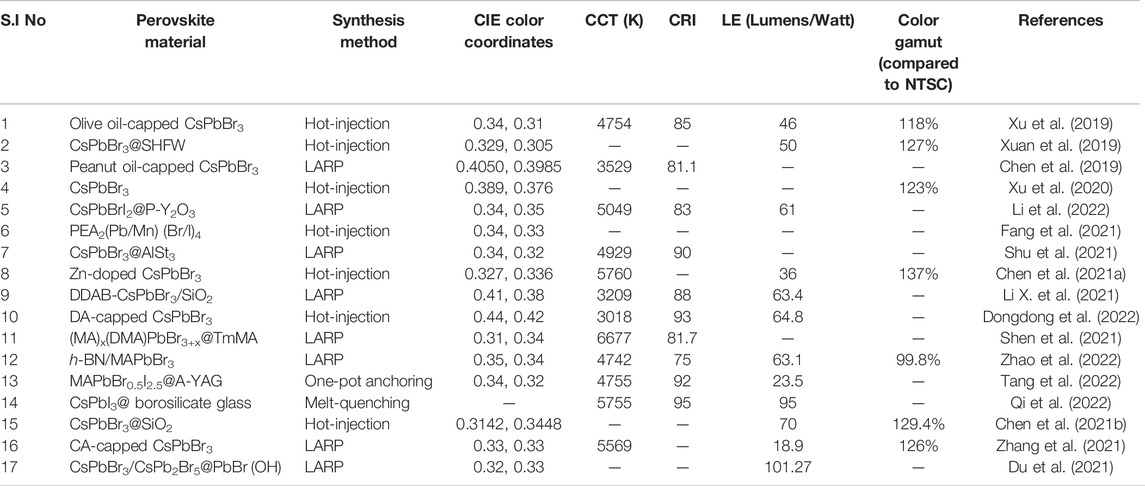
TABLE 1. Chart for different parameters of WLEDs fabricated by embedding perovskite NCs and phosphors upon a blue LED chip.
Blue LED Chip + Metal Halide Perovskite NCs
Apart from integrating red or green perovskite NCs into YAG: Ce3+ based yellow phosphor and KSF phosphor-converted WLEDs, one can also replace these traditional phosphors with perovskites such as green-emitting CsPbBr3 or red-emitting CsPbI3.
Therefore, Gao et al. synthesized CsPbBr3 NCs and assembled them into silica shells to form CsPbBr3@SiO2 nanocomposites with a high QY of 87% (Gao et al., 2021). The introduction of hydrophobic and multi-branched trioctylphosphine oxide (TOPO), suppressed the rate of hydrolysis of tetraethoxysilane (TEOS). As a result, the anion-exchange reaction among different perovskite-NCs was completely inhibited. A WLED was fabricated by combining CsPbBr3@SiO2 NCs with CsPbBr0.6I2.4@SiO2 NCs onto blue GaN chips. He obtained a CIE at (0.32, 0.33) at 5 mA current. They also achieved a high CRI of 90 and an LE of 65 Lumens/Watt. The CCT displayed a tunable range of 5993–6588 K with an injection current from 5 to 40 mA (see Figures 9B,D). To further know about the device stability, Figure 9C showed the EL spectra of this WLED before/after a continuous working period of 10 h at 5 mA. No change was seen for the two EL spectra. Similarly, Cuan and his coworkers used a hierarchically porous metal-organic framework grown in situ on CsPbX3 perovskite NCs (Cuan et al., 2021). Hierarchically porous MOF enables the impregnation of perovskite precursors in one step and allows more facile diffusion of the reactants. By integrating the green-emitting CsPbBr2I@MOF and red-emitting CsPbI3@MOF nanocomposites with a commercial blue GaN LED chip, a WLED was fabricated, which exhibited white light emission with CIE obtained at (0.36, 0.32). The fabricated WLED demonstrated an LE of 21 Lumens/Watt. They also measured the wide color gamut, which showed an NTSC value of 125% and Rec. 2020 of 93% under a driving current of 20 mA.
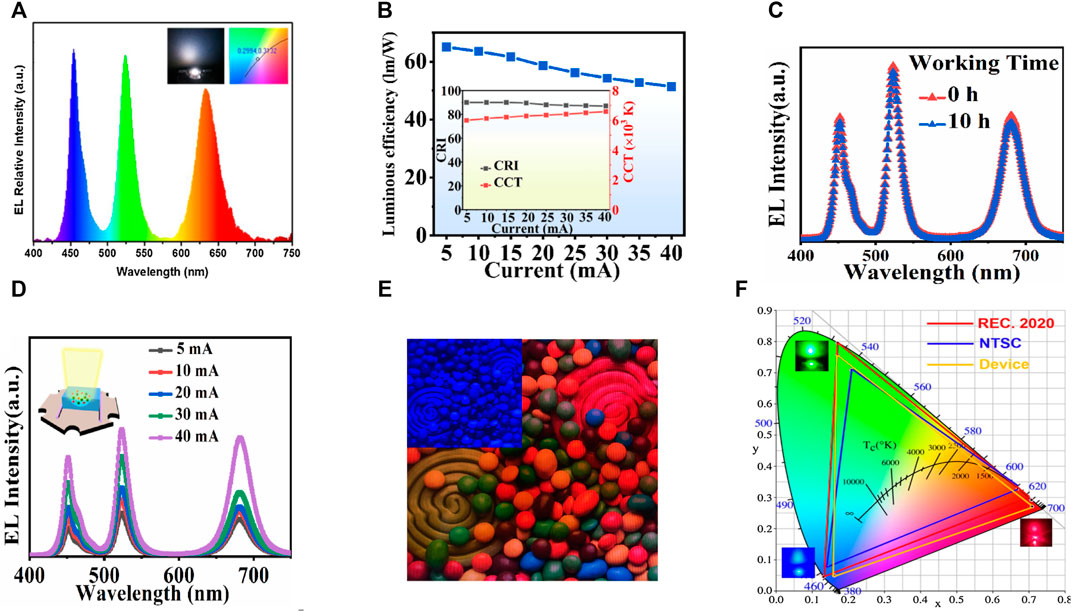
FIGURE 9. (A) EL spectra of WLEDs obtained by covering a green CsPbBr3 PQD glass sheet coated with red CsPb(Br/I)3 PQD glass powder film on a blue InGaN chip (Inset is the image and CIE of WLED). (B) Luminous efficiency of WLED based on CsPbBr3@SiO2 and CsPbBr0.6I2.4@SiO2 NCs as a function of different driving currents. Inset is the CRI and CCT of the WLED under different driving currents). (C) Working stability of the WLED at 5 mA current. (D) EL spectra of the WLED with different driving currents. (Inset is the schematic device architecture of the WLED). (E) Photographs of CsPbX3@POE-based backlit LCDs (inset is LCDs with blue chip). (F) Color gamut of the WLEDs obtained by covering a green CsPbBr3 PQD glass sheet coated with red CsPb(Br/I)3 PQD glasses powder film on a blue InGaN chip in the CIE 1931 color space. (A,F) are reproduced with permission from Yang et al. (2022). Copyright 2021, Elsevier Ltd. (B–D) are reproduced with permission from Gao et al. (2021). Copyright 2020, Elsevier B.V. (E) is reproduced with permission from Mei et al. (2022). Copyright 2022, Elsevier B.V.
Next year, Yang et al. synthesized CsPbBr3-embedded borosilicate glass through melt quenching and in situ crystallization using PbO instead of PbBr2 as the lead source (Yang et al., 2022). By adjusting the halogen element, they also synthesized red CsPb(Br/I)3 and blue CsPb(Br/Cl)3 PNC glasses. The WLED was fabricated by combining green CsPbBr3 PNC glass sheet coated with red CsPb(Br/I) PNC glasses powder film on a blue InGaN chip. The WLED had a white light emission (EL spectra are shown in Figure 9A) with CIE at (0.2994, 0.3132) (see Figure 9F) with an LE of 21 Lumens/Watt. The device achieved a wide color gamut of 121.9% NTSC standard and 91.1% Rec. 2020 standard in the CIE 1931 color space. Later, Mei et al. reported the synthesis of green and red CsPbX3 PNCs by encapsulating SiO2 and POE to protect them from moisture and heat stability (Mei et al., 2022). They obtained CsPbBr3 and CsPbBrI2 PNCs by the hot-injection method. Due to the excellent photoelectric properties of POE-encapsulated PNCs, they fabricated a WLED by combining CsPbBr3@POE and CsPbBrI2@POE composite film with a blue chip. The LE of the fabricated WLED was 15.72 Lumens/Watt with a CIE value of (0.3389, 0.3348). Besides this, the WLED showed a wide color gamut with 117% of the NTSC standard. Next, they demonstrated a liquid crystal (LCD) display by combining green-emitting CsPbBr3@ POE and red-emitting CsPbBrI2 @ POE composite films, as shown in Figure 9E. The summary of all the parameters of the fabricated WLEDs by using green- and red-emitting halide perovskites with blue chip is shown in Table 2.

TABLE 2. Chart for different parameters of WLEDs fabricated by embedding green- and red-emitting perovskite NCs upon a blue LED chip.
UV LED Chip + Metal Halide Perovskite NCs
To fabricate WLED by using a UV LED chip needs three main colors, i.e., blue, green, and red to be excited under UV illumination. Here halide perovskites are major candidates to be used as color conversion layers. These materials cover the entire visible spectrum.
In this context, Zheng et al. reported Mn and Cu co-doping at a different molar concentration to obtain CsPbCl3-xBrx NCs through anion exchange by PbBr2 at room temperature (Zheng et al., 2020). In this work, they first synthesized Mn and Cu co-doped CsPbCl3. Then they mixed the NC solution with PbBr2 precursor to obtain Mn and Cu co-doped CsPbCl3-xBrx. The incorporation of Cu ions in CsPbCl3 reduced the defect states, resulting in an enhancement in emission due to Mn doping. These Mn and Cu co-doped CsPbCl3-xBrx NCs show dual blue-orange emission with a QY of 53% and green-emitting NCs, i.e., CsPbBr3@Cs4PbBr6 core@shell with a QY over 90% was used to fabricate a WLED. The emission spectra of WLED fabricated by green CsPbBr3@Cs4PbBr6 core@shell and blue-orange co-doped CsPbCl3-xBrx NCs with the molar ratio of Mn, Pb, and Cu (2/1/2) excited by a 395-nm InGaN UV chip were obtained at 517, 450, and 600 nm, respectively. The improved performance of the WLEDs was controlled by changing the thickness of two emitting layers. The obtained WLED had CRI 85, LE 69%, and CCT 4872 K, and got a CIE value of WLED (0.346, 0.336) operated at a current of 10 mA. Also, they observed that an increase in the intensity of Mn emission improves the CRI of WLEDs. The stability of WLED was also checked with different work times from 0 to 24 h. A significant decrease in emission was observed, which was because WLED was not encapsulated. But it was noted that the emission of dual emitting blue-orange Mn and Cu co-doped CsPbCl3-xBrx NCs decreases comparatively slower than green-emitting undoped CsPbBr3 NCs. These results showed that Mn and Cu co-doped CsPbCl3-xBrx NCs were promising perovskites for fabricating high-quality WLEDs.
In 2021, Chen et al. reported Mn-doped lead halide CsPb(Br/Cl)3 as a promising phosphor material for fabricating WLEDs (Chen et al., 2021c). The obtained perovskites showed dual-color emission with blue emission at 463 nm and red emission at 602 nm. They fabricated two kinds of WLED, including the single-component Mn-doped CsPb(Br/Cl)3 phosphor-based WLED and the dual-component CsPbBr3/Mn-doped CsPb(Br/Cl)3 mixture phosphors. The prototypes were fabricated by coating as-prepared phosphors/silicone gel mixture on UV InGaN LED chips. The EL spectra of single-component WLED exhibited strong orange-red emission and relatively weak blue emission, which had no significant change when the driving current is increased from 30 to 150 mA. The warm light had been obtained at a driving current of 30 mA. But the dual-component WLED contained blue, green, and red PL peaks, which are at 426, 520, and 609 nm, respectively. The CIE obtained was (0.39, 0.38).
Similarly, Naresh et al. reported the blue-green-red emitting CsPbX3 (X = Cl/Br, Br, Br/I) PNC synthesis via the hot-injection method. Later they coated with silica and embedded in poly(methylmethacrylate) (PMMA) matrix (Naresh et al., 2021). The QYs of blue, green, and red-emitting SiO2/PMMA-coated PNCs were 37, 86, and 71%, respectively. Furthermore, the PNC-encapsulated SiO2/PMMA composite films were coupled on the surface of 365 nm UV LED to achieve white light emission. The designed WLED device was operated at 20 mA current. The obtained white light has CIE of (0.349, 0.350) and LE of 39.2%. A CCT of 4825 K and a CRI of 84.7 were achieved. They also performed the stability of fabricated WLED, which showed tremendous resilience up to 24 h at 20 mA driving current (see Figure 10D). There was no spectral variation observed in the EL spectra. The constructed WLED also exhibited EL spectra by increasing the current flow from 20 to 100 mA (see Figure 10E). Additionally, the device produced a wide color gamut of 121.47% of NTSC and 98.56% of Rec. 2020 in CIE 1931 color space.
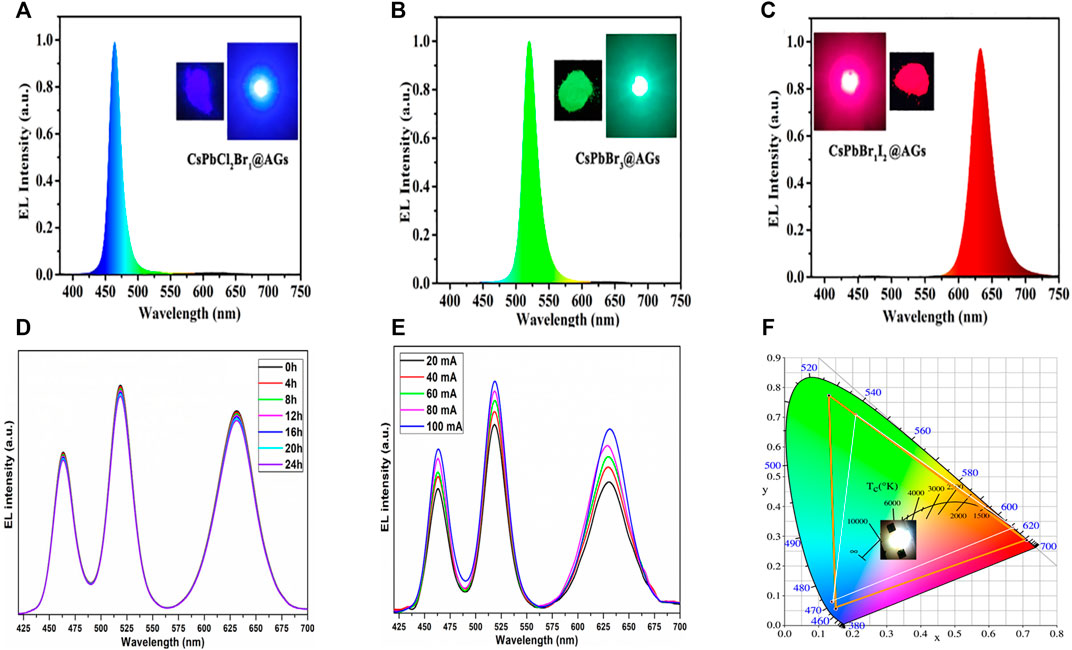
FIGURE 10. EL spectra of LEDs fabricated from (A) CsPbCl2Br1@AG, (B) CsPbBr3@AG, and (C) CsPbBr1I2@AG composites. (Insets: photographic images of corresponding blue, green, and red LED respectively). EL spectra of a constructed WLED based on PNC-encapsulated SiO2/PMMA operated at (D) different time intervals and (E) different driving currents. (F) CIE chromaticity coordinates, and color gamut of different LEDs fabricated from CsPbCl2Br1@AG, CsPbBr3@AG, and CsPbBr1I2@AG composites excited by GaN blue chip. (A–C,F) are reproduced with permission from Chen et al. (2022). Copyright 2021, Elsevier B.V. (D,E) are reproduced with permission from Naresh et al. (2021). Copyright 2020, Springer-Verlag GmbH.
As another example, Lu et al. synthesized CsPbBr1.5I1.5 NCs and grew them in situ on SrHAp [strontium hydroxyphosphate, Sr5(PO4)3OH] nanorods (Lu et al., 2021). The CsPbBr1.5I1.5@SrHAp samples showed both the intrinsic luminescence from the matrix and the emission of CsPbBr1.5I1.5 PNCs, which result in the tunable multi-color emissions in a single-phase phosphor by changing the amounts of SrHAp matrix or adjusting the excitation wavelengths. The corresponding WLED had white light emission with the chromaticity coordinates (0.343, 0.313), a CCT of 4905 K, a CRI of 91.8, and an LE of 17.8 Lumens/Watt, respectively.
Later, Z. Chen et al. encapsulated CsPbX3 (X = Cl, Br, I) PNCs are encapsulated into super-hydrophobic silica aerogels (SiO2 AGs) to form a protective layer for resisting destruction by external forces (Chen et al., 2022). The CsPbCl2Br1@AG, CsPbBr3@AG, and CsPbBr1I2@AG composites were synthesized with narrow emissions that replaced traditional phosphors as color converters. Stable white light emission is generated by two ways i.e., coupling separate CsPbBr3@AG/CsPbBr1I2@AG (WLED-1) or coupling YAG: Ce3+/CsPbBr1I2@AG (WLED-2) composites on GaN blue chip. As shown in Figures 10A–C, the EL spectra of CsPbCl2Br1@AG, CsPbBr3@AG, and CsPbBr1I2@AG composites powders emitting blue, green, and red light can be excited by the GaN blue chip. The fabricated WLED-1 had a LE of 14.55 Lumens/Watt, Ra of 94.9, and CCT of 5477 K, while the WLED-2 had an LE of 79.53 Lumens/Watt, CCT 5834 K, and Ra of 86.7, respectively. Finally, CsPbX3@AG@PS composite film based on dual protection of aerogel and PS polymer, the composite film achieved CIE of (0.3010, 0.3108) as shown in Figure 10F.
Again, Padhiar et al. reported the change in optical properties of CsPbBr3 NCs by mixing Nd3+ trivalent lanthanide halide cations (Padhiar et al., 2022). The Nd3+ cations, when incorporated into CsPbBr3 NCs, reduced the nonradiative recombination more efficiently. As a result of this, the PL QY has been significantly increased to 91%. A WLED device was fabricated using the CsPbBr3/Nd3+ NCs integrated with an ultraviolet (UV) LED chip, producing a cool white light emission with a CIE value at (0.32, 0.34). Furthermore, Sun et al. performed the synthesis of three-color perovskite phosphors, i.e., blue-emitting CsPbBr3 NCs synthesized at 20°C, green-emitting CsPbBr3 NCs synthesized at 80°C, and red-emitting Mn-doped PEA2PbBr4 NCs were coupled together to generate warm white light emission with a CRI value of 96 (Sun et al., 2022).
In addition to those, Shi et al. synthesized 0D-Cs4PbX6 NCs and adjusted the bandgap via Mn doping (Mn:Cs4PbX6) (Shi et al., 2022). CsPb(Cl/Br)3, CsPbBr3, and Mn:Cs4PbCl6 NCs were mixed in a toluene solution of PS to prepare blue, green, and red luminescent films, respectively. The WLED device was designed by placing the films as color conversion layers above a 280-nm LED chip. The color coordinates of the white LED in the CIE chromaticity diagram are (0.3229, 0.3037), which are located in the white area with a CCT of 5586 K. The summary of all the parameters of the fabricated WLEDs by using red, green, and blue-emitting halide perovskites embedded on a UV LED chip is shown in Table 3.
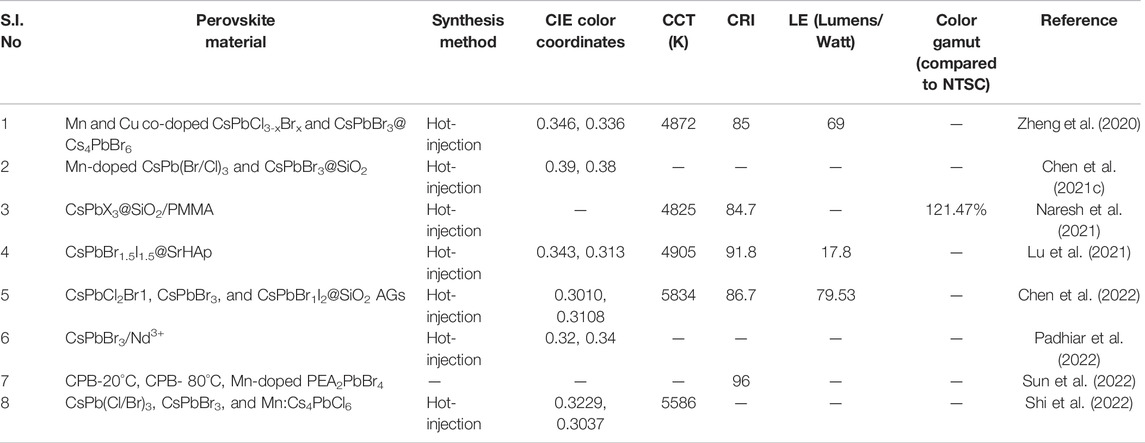
TABLE 3. Chart for different parameters of WLEDs fabricated by embedding green, red, and blue-emitting perovskite NCs upon a UV LED chip.
Concluding Remarks and Perspectives
This review basically emphasizes the current research progress and the potential of perovskite conversion layer in WLEDs. The WLEDs highly require energy efficiency, high CRI, easily tunable CCT, high luminous intensity, but at the same time need to be cost-effective suitable for their production at a large scale. In the last two decades, many phosphors have been investigated regarding WLEDs, which are currently going to reach the saturation point in terms of having new alternate phosphors and lack of optimization in the recent ones. Also, the PL QY of the phosphor-converted WLED system is low and CCT is quite high, resulting in bluish or cool WLED. By replacing the conventional phosphors with perovskite NCs, the CCT value can be optimized to a lower possible forming a natural white light. However, their instability against various environmental factors, such as humidity, temperature, light, and polar solvents, still remains an issue. On the other hand, the optical properties of these perovskite materials need to be taken care of, or the minimized optical loss has to be controlled during the integration of these materials into polymers such as silicone resin. One of the various strategies is to encapsulate the perovskite NCs with suitable metal oxides, lower dimensional perovskites, MOFs, long chained polymers, etc., which increase their stability and optical properties. As a result, these composites show great potential as color conversion layers for WLEDs, making them extra durable and efficient at the same time. For obtaining a decent WLED, a CIE value of (0.33, 0.33), a CRI value of nearly 80, and an LE in the range of 20–60 Lumens/Watt are necessary. It can also be observed that emission intensity while operation of WLEDs increases at high currents leads to excess heating that degrades the NC conversion layers. Therefore, the device fabrication method should be in such a way that it will deliver a maximum output at even low currents. This can reduce the overheating of WLEDs and prevent the degradation of emitting layers from degradation, overall increasing the working periods of WLEDs. The stability of perovskite NCs against the halide exchange process also proves their potential capability in lithography (Huang et al., 2020). The top-down lithographic method combining photolithography, electron-beam lithography, O2 plasma etching, and argon milling can be applied to construct multi-color patterned perovskite films layer by layer, thereby taking efficient WLED from perovskite conversion layers one step further. This paves the way for high-quality flat display technologies.
We also studied the synthesis process of various metal halide perovskite NCs and their subsequent use for WLED applications. The LARP synthetic procedure is one of the most favored synthetic protocols for perovskite NCs because it is a very facile and cost-effective RT process that allows large-scale production to yield high-quality perovskite NCs. The stability of perovskites NCs, precisely the heat stability, and stability against halide exchange are some of the prime aspects for producing efficient WLEDs working for longer periods of time. Compared with organic–inorganic hybrid perovskites, including large organic cations like MA or FA, the heat stability is found to be better for all inorganic perovskites, mostly for Cs-based perovskites, which is also a reason that CsPbX3 perovskite NCs are mainly used as color converters (Li et al., 2019). Furthermore, the encapsulation also inhibits halide exchange between NCs containing different halide anions (Gao et al., 2021). Another aspect is the widely tunable bandgap of these NCs, which covers most of the visible spectrum region, and the PL spectra generally have high color purity and narrow FWHM (Protesescu et al., 2015). As a result, mixing different perovskites emitting in the blue, green, or red region for white light emission would be effective. On the other hand, encapsulation of perovskite NCs in polymer matrices also results in highly flexible films, which are beneficial for future display technologies (Tang et al., 2021). These properties open ways for a more efficient combination of colors to obtain a perfect white ligiht emission closer to CIE color coordinates (0.33, 0.33), a CRI value of 96, and an LE of 101.27 Lumens/Watt. The lead-halide perovskite WLEDs are facing the toxicity issue for the Pb element present inside the perovskite structure which causes health and environmental issues (Ke and Kanatzidis, 2019; Ning and Gao, 2019; Zhang et al., 2019). Use of nontoxic lead-free perovskites as conversion layers can tackle such an issue. Interestingly, lead-free perovskites have improved thermodynamic and chemical stability which makes them potential candidates for future lead-free WLEDs. However, the emission QY and stability of lead-free perovskite NCs are still not comparable to lead-based perovskite materials, preventing the development of high-performance LEDs. Many efforts are required for the improvement of the emission properties of lead-free perovskites that can have superior optical properties that can replace lead-based perovskite materials for LEDs.
Author Contributions
All authors equally contributed to writing the manuscript.
Conflict of Interest
The authors declare that the research was conducted in the absence of any commercial or financial relationships that could be construed as a potential conflict of interest.
Publisher’s Note
All claims expressed in this article are solely those of the authors and do not necessarily represent those of their affiliated organizations, or those of the publisher, the editors, and the reviewers. Any product that may be evaluated in this article, or claim that may be made by its manufacturer, is not guaranteed or endorsed by the publisher.
Acknowledgments
SB acknowledges to Department of Science and Technology (DST), India (Award number# DST/INSPIRE/04/2017/000530), and Science and Engineering Research Board (SERB), India (Award number# SRG/2019/000093), for financial support.
References
Ahmed, G. H., Yin, J., Bakr, O. M., and Mohammed, O. F. (2021). Successes and Challenges of Core/Shell Lead Halide Perovskite Nanocrystals. ACS Energ. Lett. 6, 1340–1357. doi:10.1021/acsenergylett.1c00076
Akkerman, Q. A., D’Innocenzo, V., Accornero, S., Scarpellini, A., Petrozza, A., Prato, M., et al. (2015). Tuning the Optical Properties of Cesium Lead Halide Perovskite Nanocrystals by Anion Exchange Reactions. J. Am. Chem. Soc. 137, 10276–10281. doi:10.1021/jacs.5b05602
Akkerman, Q. A., Rainò, G., Kovalenko, M. V., and Manna, L. (2018). Genesis, Challenges and Opportunities for Colloidal lead Halide Perovskite Nanocrystals. Nat. Mater 17, 394–405. doi:10.1038/s41563-018-0018-4
Baekelant, W., Coutino-Gonzalez, E., Steele, J. A., Roeffaers, M. B. J., and Hofkens, J. (2017). Form Follows Function: Warming White LEDs Using Metal Cluster-Loaded Zeolites as Phosphors. ACS Energ. Lett. 2, 2491–2497. doi:10.1021/acsenergylett.7b00765
Baranov, D., Fieramosca, A., Yang, R. X., Polimeno, L., Lerario, G., Toso, S., et al. (2021). Aging of Self-Assembled Lead Halide Perovskite Nanocrystal Superlattices: Effects on Photoluminescence and Energy Transfer. ACS Nano 15, 650–664. doi:10.1021/acsnano.0c06595
Bhaumik, S. (2019). Oriented Attachment of Perovskite Cesium Lead Bromide Nanocrystals. ChemistrySelect 4, 4538–4543. doi:10.1002/slct.201900142
Bhaumik, S., Veldhuis, S. A., Ng, Y. F., Li, M., Muduli, S. K., Sum, T. C., et al. (2016). Highly Stable, Luminescent Core-Shell Type Methylammonium-Octylammonium lead Bromide Layered Perovskite Nanoparticles. Chem. Commun. 52, 7118–7121. doi:10.1039/c6cc01056c
Boles, M. A., Ling, D., Hyeon, T., and Talapin, D. V. (2016). The Surface Science of Nanocrystals. Nat. Mater 15, 141–153. doi:10.1038/nmat4526
Burda, C., Chen, X., Narayanan, R., and El-Sayed, M. A. (2005). Chemistry and Properties of Nanocrystals of Different Shapes. Chem. Rev. 105, 1025–1102. doi:10.1021/cr030063a
Chen, C., Zhang, L., Shi, T., Liao, G., and Tang, Z. (2019). Controllable Synthesis of All Inorganic Lead Halide Perovskite Nanocrystals with Various Appearances in Multiligand Reaction System. Nanomaterials 9, 1751. doi:10.3390/nano9121751
Chen, R., Xu, Y., Wang, S., Xia, C., Liu, Y., Yu, B., et al. (2021). Zinc Ions Doped Cesium lead Bromide Perovskite Nanocrystals with Enhanced Efficiency and Stability for white Light-Emitting Diodes. J. Alloys Compd. 866, 158969. doi:10.1016/j.jallcom.2021.158969
Chen, Z., He, H., Wen, Z., Cui, Z., Mei, S., Yang, D., et al. (2021). Highly Efficient Mn-Doped CsPb(Br/Cl)3 Mixed-Halide Perovskite via a Simple Large-Scale Synthesis Method. Mater. Sci. Eng. B 273, 115426. doi:10.1016/j.mseb.2021.115426
Chen, Z., Mei, S., He, H., Wen, Z., Cui, Z., Yang, B., et al. (2021). Rapid Large-Scale Synthesis of Highly Emissive Solid-State Metal Halide Perovskite Quantum Dots across the Full Visible Spectrum. Opt. Laser Technol. 143, 107369. doi:10.1016/j.optlastec.2021.107369
Chen, Z., Zhao, J., Zeng, R., Liu, X., Zou, B., and Xiang, W. (2022). High Efficiency Fluorescent Perovskite Quantum Dots Encapsulated in Superhydrophobic Silica Aerogel for Wide Color Gamut Backlight Displays. Chem. Eng. J. 433, 133195. doi:10.1016/j.cej.2021.133195
Correa-Baena, J.-P., Abate, A., Saliba, M., Tress, W., Jesper Jacobsson, T., Grätzel, M., et al. (2017). The Rapid Evolution of Highly Efficient Perovskite Solar Cells. Energy Environ. Sci. 10, 710–727. doi:10.1039/c6ee03397k
Crawford, M. H. (2009). LEDs for Solid-State Lighting: Performance Challenges and Recent Advances. IEEE J. Select. Top. Quan. Electron. 15, 1028–1040. doi:10.1109/jstqe.2009.2013476
Cuan, J., Zhang, D., Xing, W., Han, J., Zhou, H., and Zhou, Y. (2021). Confining CsPbX3 Perovskites in a Hierarchically Porous MOF as Efficient and Stable Phosphors for white LED. Chem. Eng. J. 425, 131556. doi:10.1016/j.cej.2021.131556
D'Andrade, B. W., and Forrest, S. R. (2004). White Organic Light-Emitting Devices for Solid-State Lighting. Adv. Mater. 16, 1585–1595. doi:10.1002/adma.200400684
Dai, Q., Duty, C. E., and Hu, M. Z. (2010). Semiconductor-Nanocrystals-Based White Light-Emitting Diodes. Small 6, 1577–1588. doi:10.1002/smll.201000144
Dongdong, Y., Shuangyi, Z., Yubo, Z., Huaxin, W., and Zhigang, Z. (2022). Highly Efficient Emission and High-CRI Warm white Light-Emitting Diodes from Ligand-Modified CsPbBr3 Quantum Dots. Opto-Electron Adv. 5, 200075. doi:10.29026/oea.2022.200075
Dou, B., Du, W., Huang, F., Ma, H., Hua, Y., Zhang, J., et al. (2020). A Next-Generation Wide Color Gamut WLED with Improved Spectral Performance in Phosphor Composite Functional Solid. Ceramics Int. 46, 27126–27133. doi:10.1016/j.ceramint.2020.07.190
Du, K., He, L., Song, S., Feng, J., Li, Y., Zhang, M., et al. (2021). In Situ Embedding Synthesis of Highly Stable CsPbBr3/CsPb2 Br5 @PbBr(OH) Nano/Microspheres through Water Assisted Strategy. Adv. Funct. Mater. 31, 2103275. doi:10.1002/adfm.202103275
Fang, Z., Wu, K., Wang, L., Xu, D., Wang, W., Lin, Y., et al. (2021). Highly Efficient and Blue-Excitable Mn-Doped PEA2Pb(Br/I)4 Perovskite for Solid Lighting. J. Lumin. 237, 118155. doi:10.1016/j.jlumin.2021.118155
Gao, F., Yang, W., Liu, X., Li, Y., Liu, W., Xu, H., et al. (2021). Highly Stable and Luminescent Silica-Coated Perovskite Quantum Dots at Nanoscale-Particle Level via Nonpolar Solvent Synthesis. Chem. Eng. J. 407, 128001. doi:10.1016/j.cej.2020.128001
García de Arquer, F. P., Armin, A., Meredith, P., and Sargent, E. H. (2017). Solution-processed Semiconductors for Next-Generation Photodetectors. Nat. Rev. Mater. 2, 16100. doi:10.1038/natrevmats.2016.100
Huang, G., Huang, Y., Xu, W., Yao, Q., Liu, X., Ding, C., et al. (2019). Cesium lead Halide Perovskite Nanocrystals for Ultraviolet and Blue Light Blocking. Chin. Chem. Lett. 30, 1021–1023. doi:10.1016/j.cclet.2018.12.028
Huang, X., Xiao, X., and Dong, G. (2020). Metal Halide Perovskites Functionalized by Patterning Technologies. Adv. Mater. Technol. 5, 2000513. doi:10.1002/admt.202000513
Jia, C., Li, H., Meng, X., and Li, H. (2018). CsPbX3/Cs4PbX6 Core/shell Perovskite Nanocrystals. Chem. Commun. 54, 6300–6303. doi:10.1039/c8cc02802h
Kamat, P. V., Pradhan, N., Schanze, K., Weiss, P. S., Buriak, J., Stang, P., et al. (2020). Challenges and Opportunities in Designing Perovskite Nanocrystal Heterostructures. ACS Energ. Lett. 5, 2253–2255. doi:10.1021/acsenergylett.0c01216
Kar, M. R., Ray, S., Patra, B. K., and Bhaumik, S. (2021). State of the Art and Prospects of Metal Halide Perovskite Core@shell Nanocrystals and Nanocomposites. Mater. Today Chem. 20, 100424. doi:10.1016/j.mtchem.2021.100424
Kazes, M., Udayabhaskararao, T., Dey, S., and Oron, D. (2021). Effect of Surface Ligands in Perovskite Nanocrystals: Extending in and Reaching Out. Acc. Chem. Res. 54, 1409–1418. doi:10.1021/acs.accounts.0c00712
Ke, W., and Kanatzidis, M. G. (2019). Prospects for Low-Toxicity lead-free Perovskite Solar Cells. Nat. Commun. 10, 965. doi:10.1038/s41467-019-08918-3
Kong, Z.-C., Liao, J.-F., Dong, Y.-J., Xu, Y.-F., Chen, H.-Y., Kuang, D.-B., et al. (2018). Core@Shell CsPbBr3@Zeolitic Imidazolate Framework Nanocomposite for Efficient Photocatalytic CO2 Reduction. ACS Energ. Lett. 3, 2656–2662. doi:10.1021/acsenergylett.8b01658
Kovalenko, M. V., Protesescu, L., and Bodnarchuk, M. I. (2017). Properties and Potential Optoelectronic Applications of lead Halide Perovskite Nanocrystals. Science 358, 745–750. doi:10.1126/science.aam7093
Kramer, I. J., and Sargent, E. H. (2014). The Architecture of Colloidal Quantum Dot Solar Cells: Materials to Devices. Chem. Rev. 114, 863–882. doi:10.1021/cr400299t
Krames, M. R., Shchekin, O. B., Mueller-Mach, R., Mueller, G. O., Zhou, L., Harbers, G., et al. (2007). Status and Future of High-Power Light-Emitting Diodes for Solid-State Lighting. J. Display Technol. 3, 160–175. doi:10.1109/jdt.2007.895339
Li, X., Tan, Y., Lai, H., Li, S., Chen, Y., Li, S., et al. (2019). All-Inorganic CsPbBr3 Perovskite Solar Cells with 10.45% Efficiency by Evaporation-Assisted Deposition and Setting Intermediate Energy Levels. ACS Appl. Mater. Inter. 11, 29746–29752. doi:10.1021/acsami.9b06356
Li, X., Cai, W., Guan, H., Zhao, S., Cao, S., Chen, C., et al. (2021). Highly Stable CsPbBr3 Quantum Dots by Silica-Coating and Ligand Modification for white Light-Emitting Diodes and Visible Light Communication. Chem. Eng. J. 419, 129551. doi:10.1016/j.cej.2021.129551
Li, X., Cao, F., Yu, D., Chen, J., Sun, Z., Shen, Y., et al. (2017). All Inorganic Halide Perovskites Nanosystem: Synthesis, Structural Features, Optical Properties and Optoelectronic Applications. Small 13, 1603996. doi:10.1002/smll.201603996
Li, X., Wei, Y., Dang, P., Xiao, X., Xiao, H., Zhang, G., et al. (2022). Enhancing the Stability of Perovskite Quantum Dots CsPbX3 (X=Cl, Br, I) by Encapsulation in Porous Y2O3 Nanoparticles for WLED Applications. Mater. Res. Bull. 146, 111592. doi:10.1016/j.materresbull.2021.111592
Li, Y., Zhang, X., Huang, H., Kershaw, S. V., and Rogach, A. L. (2020). Advances in Metal Halide Perovskite Nanocrystals: Synthetic Strategies, Growth Mechanisms, and Optoelectronic Applications. Mater. Today 32, 204–221. doi:10.1016/j.mattod.2019.06.007
Li, Z. J., Hofman, E., Li, J., Davis, A. H., Tung, C. H., Wu, L. Z., et al. (2018). Photoelectrochemically Active and Environmentally Stable CsPbBr3/TiO2 Core/Shell Nanocrystals. Adv. Funct. Mater. 28, 1704288. doi:10.1002/adfm.201704288
Li, Z., Zhou, F., Yao, H., Ci, Z., Yang, Z., and Jin, Z. (2021). Halide Perovskites for High-Performance X-ray Detector. Mater. Today 48, 155–175. doi:10.1016/j.mattod.2021.01.028
Lin, C. C., Meijerink, A., and Liu, R.-S. (2016). Critical Red Components for Next-Generation White LEDs. J. Phys. Chem. Lett. 7, 495–503. doi:10.1021/acs.jpclett.5b02433
Liu, M., Yazdani, N., Yarema, M., Jansen, M., Wood, V., and Sargent, E. H. (2021). Colloidal Quantum Dot Electronics. Nat. Electron. 4, 548–558. doi:10.1038/s41928-021-00632-7
Lu, Y., Zhang, C., Yuan, S., Zhang, C., Wang, J., Kong, H., et al. (2021). Novel CsPbBr1.5I1.5@SrHAp Single-phase white-light Emitting Phosphors: Facile Synthesis, Formation Process, Enhanced Stability, and Color-Tunable Luminescence. Chem. Eng. J. 426, 130809. doi:10.1016/j.cej.2021.130809
Mei, E., Li, J., He, Q., Tong, Y., Liu, R., Fan, H., et al. (2022). POE Enhanced Stabilities of CsPbX3 (X = Br, I) Perovskite and Their white LED Application. J. Energ. Chem. 67, 193–200. doi:10.1016/j.jechem.2021.10.005
Mondal, R. K., Adhikari, S., Chatterjee, V., and Pal, S. (2021). Recent Advances and Challenges in AlGaN-Based Ultra-violet Light Emitting Diode Technologies. Mater. Res. Bull. 140, 111258. doi:10.1016/j.materresbull.2021.111258
Nakamura, S., Mukai, T., and Senoh, M. (1994). Candela‐class High‐brightness InGaN/AlGaN Double‐heterostructure Blue‐light‐emitting Diodes. Appl. Phys. Lett. 64, 1687–1689. doi:10.1063/1.111832
Nakamura, S., Mukai, T., and Senoh, M. (1994). High‐brightness InGaN/AlGaN Double‐heterostructure blue‐green‐light‐emitting Diodes. J. Appl. Phys. 76, 8189–8191. doi:10.1063/1.357872
Nakamura, S., Senoh, M., and Mukai, T. (1993). High‐power InGaN/GaN Double‐heterostructure Violet Light Emitting Diodes. Appl. Phys. Lett. 62, 2390–2392. doi:10.1063/1.109374
Nardelli, A., Deuschle, E., de Azevedo, L. D., Pessoa, J. L. N., and Ghisi, E. (2017). Assessment of Light Emitting Diodes Technology for General Lighting: A Critical Review. Renew. Sustain. Energ. Rev. 75, 368–379. doi:10.1016/j.rser.2016.11.002
Naresh, V., Kim, B. H., and Lee, N. (2021). Synthesis of CsPbX3 (X = Cl/Br, Br, and Br/I)@SiO2/PMMA Composite Films as Color-Conversion Materials for Achieving Tunable Multi-Color and white Light Emission. Nano Res. 14, 1187–1194. doi:10.1007/s12274-020-3170-5
Nedelcu, G., Protesescu, L., Yakunin, S., Bodnarchuk, M. I., Grotevent, M. J., and Kovalenko, M. V. (2015). Fast Anion-Exchange in Highly Luminescent Nanocrystals of Cesium Lead Halide Perovskites (CsPbX3, X = Cl, Br, I). Nano Lett. 15, 5635–5640. doi:10.1021/acs.nanolett.5b02404
Ning, W., and Gao, F. (2019). Structural and Functional Diversity in Lead‐Free Halide Perovskite Materials. Adv. Mater. 31, 1900326. doi:10.1002/adma.201900326
Padhiar, M. A., Wang, M., Ji, Y., Yang, Z., and Bhatti, A. S. (2022). Tuning Optical Properties of CsPbBr3 by Mixing Nd3+ Trivalent Lanthanide Halide Cations for Blue Light Emitting Devices. Nanotechnology 33, 175202. doi:10.1088/1361-6528/ac4b2e
Pan, A., He, B., Fan, X., Liu, Z., Urban, J. J., Alivisatos, A. P., et al. (2016). Insight into the Ligand-Mediated Synthesis of Colloidal CsPbBr3 Perovskite Nanocrystals: The Role of Organic Acid, Base, and Cesium Precursors. ACS Nano 10, 7943–7954. doi:10.1021/acsnano.6b03863
Park, B. w., and Seok, S. I. (2019). Intrinsic Instability of Inorganic-Organic Hybrid Halide Perovskite Materials. Adv. Mater. 31, 1805337. doi:10.1002/adma.201805337
Phillips, J. M., Coltrin, M. E., Crawford, M. H., Fischer, A. J., Krames, M. R., Mueller-Mach, R., et al. (2007). Research Challenges to Ultra-efficient Inorganic Solid-State Lighting. Laser Photon. Rev. 1, 307–333. doi:10.1002/lpor.200710019
Protesescu, L., Yakunin, S., Bodnarchuk, M. I., Bertolotti, F., Masciocchi, N., Guagliardi, A., et al. (2016). Monodisperse Formamidinium Lead Bromide Nanocrystals with Bright and Stable Green Photoluminescence. J. Am. Chem. Soc. 138, 14202–14205. doi:10.1021/jacs.6b08900
Protesescu, L., Yakunin, S., Bodnarchuk, M. I., Krieg, F., Caputo, R., Hendon, C. H., et al. (2015). Nanocrystals of Cesium Lead Halide Perovskites (CsPbX3, X = Cl, Br, and I): Novel Optoelectronic Materials Showing Bright Emission with Wide Color Gamut. Nano Lett. 15, 3692–3696. doi:10.1021/nl5048779
Qi, F., Shao, X., Ma, Y., Sun, Y., Zhu, J., Yin, P., et al. (2022). Improved Luminescent Performances of CsPbI3 Perovskite Quantum Dots via Optimizing the Proportion of boron-silicate Glass and Precipitation Processing. Opt. Mater. 124, 111981. doi:10.1016/j.optmat.2022.111981
Raja, S. N., Bekenstein, Y., Koc, M. A., Fischer, S., Zhang, D., Lin, L., et al. (2016). Encapsulation of Perovskite Nanocrystals into Macroscale Polymer Matrices: Enhanced Stability and Polarization. ACS Appl. Mater. Inter. 8, 35523–35533. doi:10.1021/acsami.6b09443
Roduner, E. (2006). Size Matters: Why Nanomaterials Are Different. Chem. Soc. Rev. 35, 583–592. doi:10.1039/b502142c
Sasabe, H., and Kido, J. (2011). Multifunctional Materials in High-Performance OLEDs: Challenges for Solid-State Lighting. Chem. Mater. 23, 621–630. doi:10.1021/cm1024052
Schmidt, L. C., Pertegás, A., González-Carrero, S., Malinkiewicz, O., Agouram, S., Mínguez Espallargas, G., et al. (2014). Nontemplate Synthesis of CH3NH3PbBr3 Perovskite Nanoparticles. J. Am. Chem. Soc. 136, 850–853. doi:10.1021/ja4109209
Schubert, E. F., and Kim, J. K. (2005). Solid-State Light Sources Getting Smart. Science 308, 1274–1278. doi:10.1126/science.1108712
Shen, Y., Tang, H., Liu, F., Lin, K., Lu, J., Yan, C., et al. (2021). Stable Cyan and white Light-Emitting Diodes Enabled by Branched Cations Sterically Stabilized 2D/3D Perovskites. Chem. Eng. J. 423, 130160. doi:10.1016/j.cej.2021.130160
Shi, W., Zhang, X., Matras-Postolek, K., and Yang, P. (2022). Mn-derived Cs4PbX6 Nanocrystals with Stable and Tunable Wide Luminescence for white Light-Emitting Diodes. J. Mater. Chem. C 10, 3886–3893. doi:10.1039/d2tc00014h
Shirasaki, Y., Supran, G. J., Bawendi, M. G., and Bulović, V. (2013). Emergence of Colloidal Quantum-Dot Light-Emitting Technologies. Nat. Photon 7, 13–23. doi:10.1038/nphoton.2012.328
Shu, B., Chang, Y., Dong, L., Chen, L., Wang, H., Yang, S., et al. (2021). Highly Stable CsPbBr3 Perovskite Quantum Dots Incorporated in Aluminum Stearate. J. Lumin. 234, 117962. doi:10.1016/j.jlumin.2021.117962
Sum, T. C., and Mathews, N. (2014). Advancements in Perovskite Solar Cells: Photophysics behind the Photovoltaics. Energ. Environ. Sci. 7, 2518–2534. doi:10.1039/c4ee00673a
Sun, S., Yuan, D., Xu, Y., Wang, A., and Deng, Z. (2016). Ligand-Mediated Synthesis of Shape-Controlled Cesium Lead Halide Perovskite Nanocrystals via Reprecipitation Process at Room Temperature. ACS Nano 10, 3648–3657. doi:10.1021/acsnano.5b08193
Sun, Y., Li, Y., Zhang, W., Zhu, P., Zhu, H., Qin, W., et al. (2022). Simultaneous Synthesis, Modification, and DFT Calculation of Three‐Color Lead Halide Perovskite Phosphors for Improving Stability and Luminous Efficiency of WLEDs. Adv. Opt. Mater. 10, 2101765. doi:10.1002/adom.202101765
Tang, X., Kothalawala, N. L., Zhang, Y., Qian, D., Kim, D. Y., and Yang, F. (2021). Water-driven CsPbBr3 Nanocrystals and Poly(methyl Methacrylate)-CsPbBr3 Nanocrystal Films with Bending-Endurable Photoluminescence. Chem. Eng. J. 425, 131456. doi:10.1016/j.cej.2021.131456
Tang, Y., He, X., Zhang, Y., Yuan, H., Xin, Y., Ren, X., et al. (2022). Anchoring of Red Perovskite Nanocrystals on YAG:Ce Phosphor for High Color Rendering index WLEDs. J. Alloys Compd. 899, 163347. doi:10.1016/j.jallcom.2021.163347
Tsao, J. Y., Saunders, H. D., Creighton, J. R., Coltrin, M. E., and Simmons, J. A. (2010). Solid-state Lighting: an Energy-Economics Perspective. J. Phys. D: Appl. Phys. 43, 354001. doi:10.1088/0022-3727/43/35/354001
Veldhuis, S. A., Boix, P. P., Yantara, N., Li, M., Sum, T. C., Mathews, N., et al. (2016). Perovskite Materials for Light-Emitting Diodes and Lasers. Adv. Mater. 28, 6804–6834. doi:10.1002/adma.201600669
Wang, L., Xie, R.-J., Suehiro, T., Takeda, T., and Hirosaki, N. (2018). Down-Conversion Nitride Materials for Solid State Lighting: Recent Advances and Perspectives. Chem. Rev. 118, 1951–2009. doi:10.1021/acs.chemrev.7b00284
Wang, S., Bi, C., Yuan, J., Zhang, L., and Tian, J. (2018). Original Core–Shell Structure of Cubic CsPbBr3@Amorphous CsPbBrx Perovskite Quantum Dots with a High Blue Photoluminescence Quantum Yield of over 80. ACS Energ. Lett. 3, 245. doi:10.1021/acsenergylett.7b01243
Wang, Y., He, J., Chen, H., Chen, J., Zhu, R., Ma, P., et al. (2016). Ultrastable, Highly Luminescent Organic-Inorganic Perovskite-Polymer Composite Films. Adv. Mater. 28, 10710–10717. doi:10.1002/adma.201603964
Wood, V., and Bulović, V. (2010). Colloidal Quantum Dot Light-Emitting Devices. Nano Rev. 1, 5202. doi:10.3402/nano.v1i0.5202
Xu, H., Wang, J., Xuan, T., Lv, C., Hou, J., Zhang, L., et al. (2019). Convenient and Large-Scale Synthesis of High-Quality, All-Inorganic lead Halide Perovskite Nanocrystals for white Light-Emitting Diodes. Chem. Eng. J. 364, 20–27. doi:10.1016/j.cej.2019.01.152
Xu, Y., Lou, S., Xia, C., Xuan, T., and Li, H. (2020). Controllable Synthesis of All Inorganic lead Halide Perovskite Nanocrystals and white Light-Emitting Diodes Based on CsPbBr3 Nanocrystals. J. Lumin. 222, 117132. doi:10.1016/j.jlumin.2020.117132
Xuan, T., Huang, J., Liu, H., Lou, S., Cao, L., Gan, W., et al. (2019). Super-Hydrophobic Cesium Lead Halide Perovskite Quantum Dot-Polymer Composites with High Stability and Luminescent Efficiency for Wide Color Gamut White Light-Emitting Diodes. Chem. Mater. 31, 1042–1047. doi:10.1021/acs.chemmater.8b04596
Yan, F., Tan, S. T., Li, X., and Demir, H. V. (2019). Light Generation in Lead Halide Perovskite Nanocrystals: LEDs, Color Converters, Lasers, and Other Applications. Small 15, 1902079. doi:10.1002/smll.201902079
Yang, B., Mei, S., He, H., Zhu, Y., Hu, R., Zou, J., et al. (2022). Lead Oxide Enables lead Volatilization Pollution Inhibition and Phase Purity Modulation in Perovskite Quantum Dots Embedded Borosilicate Glass. J. Eur. Ceram. Soc. 42, 258–265. doi:10.1016/j.jeurceramsoc.2021.09.052
Yang, M., Peng, H.-s., Zeng, F.-l., Teng, F., Qu, Z., Yang, D., et al. (2018). In Situ silica Coating-Directed Synthesis of Orthorhombic Methylammonium lead Bromide Perovskite Quantum Dots with High Stability. J. Colloid Interf. Sci. 509, 32–38. doi:10.1016/j.jcis.2017.08.094
Yang, Z., Gao, M., Wu, W., Yang, X., Sun, X. W., Zhang, J., et al. (2019). Recent Advances in Quantum Dot-Based Light-Emitting Devices: Challenges and Possible Solutions. Mater. Today 24, 69–93. doi:10.1016/j.mattod.2018.09.002
Zeng, F.-l., Yang, M., Qin, J.-l., Teng, F., Wang, Y.-q., Chen, G.-x., et al. (2018). Ultrastable Luminescent Organic-Inorganic Perovskite Quantum Dots via Surface Engineering: Coordination of Methylammonium Bromide and Covalent Silica Encapsulation. ACS Appl. Mater. Inter. 10, 42837–42843. doi:10.1021/acsami.8b14677
Zhang, H., Wang, X., Liao, Q., Xu, Z., Li, H., Zheng, L., et al. (2017). Embedding Perovskite Nanocrystals into a Polymer Matrix for Tunable Luminescence Probes in Cell Imaging. Adv. Funct. Mater. 27, 1604382. doi:10.1002/adfm.201604382
Zhang, J., Hodes, G., Jin, Z., and Liu, S. (2019). All‐Inorganic CsPbX3 Perovskite Solar Cells: Progress and Prospects. Angew. Chem. Int. Ed. 58, 15596–15618. doi:10.1002/anie.201901081
Zhang, Y., Li, G., She, C., Liu, S., Yue, F., Jing, C., et al. (2021). Room Temperature Preparation of Highly Stable Cesium lead Halide Perovskite Nanocrystals by Ligand Modification for white Light-Emitting Diodes. Nano Res. 14, 2770–2775. doi:10.1007/s12274-021-3283-5
Zhao, X., Jia, W., Wang, H., Yan, G., Sun, Y., Tang, X., et al. (2022). Preparation of CH3NH3PbBr3 Perovskite Quantum Dots Composites with High Photoluminescence Quantum Yield and Good Stability. J. Lumin. 245, 118749. doi:10.1016/j.jlumin.2022.118749
Zhao, X., and Park, N.-G. (2015). Stability Issues on Perovskite Solar Cells. Photonics 2, 1139–1151. doi:10.3390/photonics2041139
Zheng, Y., Yuan, X., Yang, J., Li, Q., Yang, X., Fan, Y., et al. (2020). Cu Doping-Enhanced Emission Efficiency of Mn2+ in Cesium lead Halide Perovskite Nanocrystals for Efficient white Light-Emitting Diodes. J. Lumin. 227, 117586. doi:10.1016/j.jlumin.2020.117586
Keywords: metal halide perovskite nanocrystals, color converters, white light-emitting diodes, color quality, efficiency
Citation: Mohapatra A, Kar MR and Bhaumik S (2022) Recent Progress and Prospects on Metal Halide Perovskite Nanocrystals as Color Converters in the Fabrication of White Light-Emitting Diodes. Front. Electron. Mater. 2:891983. doi: 10.3389/femat.2022.891983
Received: 08 March 2022; Accepted: 04 April 2022;
Published: 04 May 2022.
Edited by:
Wei You, University of North Carolina at Chapel Hill, United StatesReviewed by:
Giuseppe Maria Paternò, Politecnico di Milano, ItalyZhiwen Jin, Lanzhou University, China
Copyright © 2022 Mohapatra, Kar and Bhaumik. This is an open-access article distributed under the terms of the Creative Commons Attribution License (CC BY). The use, distribution or reproduction in other forums is permitted, provided the original author(s) and the copyright owner(s) are credited and that the original publication in this journal is cited, in accordance with accepted academic practice. No use, distribution or reproduction is permitted which does not comply with these terms.
*Correspondence: Saikat Bhaumik, cy5iaGF1bWlrQGlvY2IuaWN0bXVtYmFpLmVkdS5pbg==