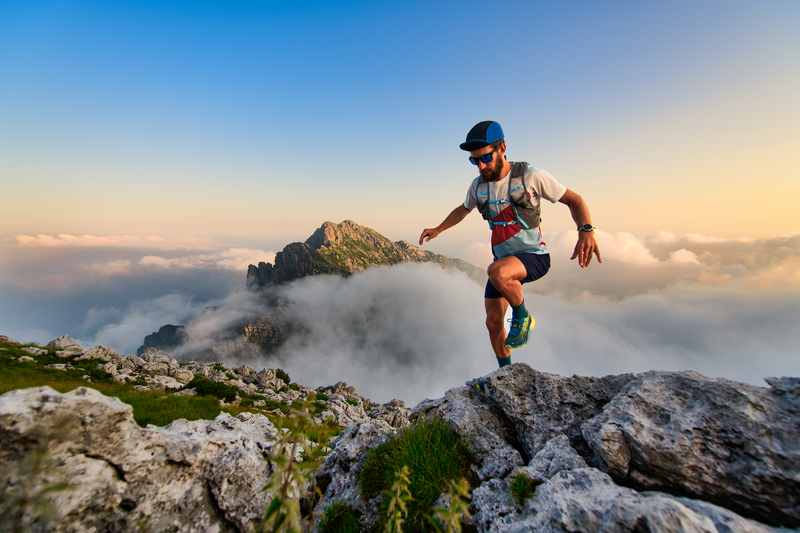
95% of researchers rate our articles as excellent or good
Learn more about the work of our research integrity team to safeguard the quality of each article we publish.
Find out more
MINI REVIEW article
Front. Electron. , 06 December 2021
Sec. Wearable Electronics
Volume 2 - 2021 | https://doi.org/10.3389/felec.2021.793780
This article is part of the Research Topic Wearable and Implantable Electronics for the Next Generation of Human-Machine Interactive Devices View all 7 articles
Along with the industrialization and popularization of the wearable electronics, an increasing number of the wireless sensor nodes (WSNs) are deployed. Nevertheless, the conventional battery-based power supply system has no longer satisfied the requirement of large-scale WSNs in terms of battery life, which emerges the energy harvesting (EH) technique. In order to combine various of energy sources and drive multi-loads, the multi-input single-inductor multi-output (MISIMO) EH interface applied to wearable electronics is spotlighted. In this mini-review article, the solutions for improving power conversion efficiency (PCE) and output quality in MISIMO EH interface are summarized. Furthermore, the future trends of MISIMO EH interface are also presented.
Benefiting from the rapid development of the sensor technologies and the miniaturization of the electronics, the wearable and implantable electronic devices have been widely used in biomedical research Yang et al. (2019); Juteau and Gosselin (2020), motion detection Le et al. (2019); Dabbaghian et al. (2019) and health monitoring Ding et al. (2021); Lee et al., 2020b. In such scenarios, the wireless sensor nodes (WSNs) are in charge of monitoring the physical parameters and transmitting the digitized data. It is highly demanded to prolong the usage time of the WSNs while subjecting to small-volume batteries. The typical power consumption of a WSN is ranging from micro-watts (in idle state) to milli-watts (in active state), which makes it feasible to be powered from the energy harvesting (EH) technique. The harvested energy is a beneficial complement to battery energy, or more promising in the future, capable of fully taking the place of the battery and leading to an energy autonomous WSNs. Figure 1 shows such an autonomous WSN supplied by the energy harvesting interface. The ambient energy sources can be extracted with the energy transducer such as thermoelectric generators (TEG) Chen et al., 2019b; Coustans et al. (2019), photovoltaic (PV) cells Shim et al. (2019); Jeong et al. (2020), triboelectric nanogenerators (TENG) Kara et al. (2021); Niu et al. (2015), biofuel (BF) cells Talkhooncheh et al. (2021); Katic et al. (2018), piezoelectric harvesters (PEH) Chen et al. (2020); Angelov and Nielsen-Lönn, 2020 and RF energy harvester (RFEH) Zeng et al., 2020; Martins and Serdijn, 2021, etc. However, the energy available from a single energy source is normally weak and stochastic, which is severely dominated by the changing environmental conditions. To combine various of energy sources, the multi-input with single-inductor solution is employed to increase the extracted power density while deceasing the volume of the bulky off-chip components. Moreover, considering the different load requirements of the analog signal conditioning, digital signal processing and RF transceiver/receiver blocks, multi-voltage domains are normally required to optimize the performance of each block independently. As a result, a multi-input single-inductor multi-output (MISIMO) energy harvesting interface applied to autonomous WSNs is of the most value and attracts lots of attentions.
However, the MISIMO EH interface faces two main problems. The first one is how to improve the power conversion efficiency (PCE). On the one hand, in the energy-constrained environment, a higher PCE ensures the sufficient power delivering to the loads. On the other hand, the MISIMO EH interface is generally designed to multi-modes for accommodating the various kinds of input and output conditions. It is challenging to optimize the power loss (including conduction, switching and control loss, etc) in different modes. Moreover, the energy transducer inherently has a changeable source resistance, thus the available power to the load can be maximized only if the equivalent input resistance of the converter equals to the source resistance according to the power delivery theory. Therefore, a simultaneous maximum power point tracking (MPPT) technique for each source has to be adopted for improving the end-to-end efficiency. The other problem is how to improve the output quality, including reducing the output voltage ripple and improving the load transient response. Due to the interaction between multi-output when the load condition is changing, the output voltage ripple is introduced. Large voltage ripple in digital circuit leads to high power consumption whereas it impacts the precision of the analog signal conditioning if the circuit is suffered from insufficient power supply rejection ratio (PSRR). To reduce the average power consumption, the WSN normally works in the heavily duty-cycle mode. The load current transits from the idle state to the active state from time to time. Thus the EH interface is highly demanded to have a rapid load transient response within a wide load range. In the MISIMO EH interface with a battery as a supplement device for energy delivery or storage, the interface can be easily kick-started. Nevertheless, the start-up circuit such as a charge-pump Chen et al., 2019c is indispensable if the stored component is a super capacitor.
In this mini-review paper, the state-of-the art solutions to solve these two problems are reviewed and summarized, with the purpose to skeleton the future development of the relating research areas. The paper is organized as follows: Section 2 summarizes the state-of-the-art techniques proposed in MISIMO EH interfaces for improving the PCE and output quality. Section 3 draws the conclusion and presents the future developing trends.
In this section, the state-of-the-art techniques for improving the PCE and the output quality in MISIMO EH interfaces for wearable electronics are reviewed.
Firstly, reducing power loss of the MISIMO EH interface is the straightforward method for the efficiency optimization, which could be categorized as the reduction of the conduction loss, switching loss and the control loss. The conduction loss mainly ascribes to the IR drop of the current flowing path, including the power transistors, the off-chip inductor and the stored components. As a part from the aforementioned conduction loss, MISIMO EH interfaces deliver the extra energy from the source to battery in light load condition and supplement the insufficient energy from battery to load. As a result, the “double-conversion” loss is introduced. The double-conversion rejection technique (DCRT) Kim et al. (2021) lowered the trigger conditions of the source transferring the energy to the load directly, which avoids the excessive participation of the battery, therefore the double-conversion loss can be reduced. Wang et al. (2020) proposed the clockless shortest power path (CSPP) technique, which compares the output voltages and the input voltages with their respective relaxation ranges of the reference voltages, then selects the right input and output to achieve a shortest power path. In addition, turn-on resistance as well as the sizes of the power transistors need to be optimized adaptively to match the various switching modes in MISIMO EH interface. A reconfigurable and extendable Single-Inductor Single-Path three-switch (1P3S) converter has been proposed in Huang and Kuo (2020), which can be reconfigurable or combined to the 2P3S or 2P6S converter according to various input voltages and output voltages, thus the appropriate power switch sizes can be constituted for the suitable applications. Similarly, the 2P3S converter proposed in Wang et al. (2016) is able to eliminate inductor-sharing power switches in a DISIDO converter, thus the reduced conduction loss is achieved under the condition if the stored battery is initially charged and the most portion of the load energy comes from the source. The switch size modulation (SSM) technique is adopted in Amin and Mercier (2018), Kim et al. (2021), Qian et al. (2017). It applied modulated power switch sizes and gate drivers according to various input source energy or load conditions since the inductor current changes in different modes. The switching loss is mainly caused by the parasitic capacitance of the power transistors which are charged before on-period and discharged before off-period. Since the switching loss is proportional to the parasitic capacitance and the switching frequency, modulating the switching frequency to accommodate the different modes is regarded as an effective method to reduce the switching loss. Kuai et al. (2019) proposed the dual-frequency to accommodate different switching modes, where the lower frequency clock CLKL is employed in harvesting mode and the higher frequency clock CLKH is implemented in recycling mode. In the recycling mode, the battery supplements energy to the load to complement the insufficient harvested energy, thus a smoothly high efficiency is maintained over a wide dynamic range. The reversely polarized energy recycling (RPER) technique proposed in Chen et al., 2019c achieves a negative voltage stored in the output capacitor, thus a lower frequency is realized, at the cost of slightly increasing of the conduction loss. Katic et al. (2018) sets four different frequencies (1, 2, 4, and 8 kHz) for different input voltages, which provides the trade-off between the performance and complexity. The control loss mainly comes from the quiescent power consumption of the analog circuits such as bandgap references/comparators and dynamic power consumption of the digital logic control circuits. Reducing the bias current is an efficient method to decrease the control loss in analog circuits Gao et al. (2021). Substituting the analog circuits with digital circuits is getting trendy in many low-power design Paidimarri and Chandrakasan (2017). As one known, the dynamic loss caused by the digital circuits is proportional to the square of the supply voltage. It is necessary to lower the supply voltage of the control logic since its power consumption constitutes a large portion of the control loss compared to the conventional EH interfaces. Therefore, the multi-voltage domain design technique is generally employed to decrease the control loss. When interface the low voltage control signal with the high voltage power devices, level-shifters and gate drivers are normally needed Katic et al., 2018; Chen et al., 2019c; Wang et al. (2020). The event-driven control techniques proposed in Kim et al., 2018; Amin and Mercier, 2018; Wang et al. (2020)feature of clock-less operation. The converter is triggered by the event signal without a system clock. Therefore, both the quiescent and dynamic power loss are reduced. This technique is beneficial particularly in the light load condition since the event-driven pulse frequency can be significantly reduced compared to the conventional clock-driven converters. Moreover, the conduction loss and switching loss are also decreased because of less conduction times of the power transistors.
Secondly, improving the MPPT efficiency is a crucial approach to improve the end-to-end efficiency and maximize the extracted energy. In the MISIMO EH interface that needs to combine the different source energy, it is necessary to achieve the MPPT for each source simultaneously. The fractional open circuit voltage (FOCV) method has been widely used in MPPT owning to its low power consumption and simplicity Chowdary et al. (2016). It samples the open circuit voltage (OCV) of the energy harvester, subsequently the sampled voltage with a division ratio is compared with the input voltage of the converter. The division ratio for each energy source is set to 0.625 (PV), 0.6 (BFC), and 0.5 (TEG) experimentally in Kim et al. (2021), leading to poor adaptation. The OCV is normally sampled form each source periodically for the purpose of decreasing power consumption overhead. If multiple sources are prepared but only one is selected, a relatively large input voltage ripple and a deviation from the MPP voltage could be introduced from the not-chosen sources. The innovative dual-source mode proposed in Liu et al. (2018) extracts the TEG and PV in one switching period, therefore, the input voltage ripple is reduced with a better tracking efficiency. Ashraf (2020) introduced a short period before the inductor energizing phase to compare the available input power of two TEG sources and decide which source is to be selected, the multiplexer block changes the clock frequency for ensuring the systems always work at MPP. Maeng et al. (2021) proposed a calibration method through external register bits to control the division ratio, a higher tracking efficiency can be achieved. Hill climbing method is the other technique to achieve MPPT in MISIMO converters which features of high tracking efficiency at the cost of a relatively complicated logic. It adjusts the on-time of the power transistors to change the equivalent input resistances to different inputs. The programmable capacitor array (PCA) MPPT controller proposed in Qian et al. (2017) employs a high resolution 8-bit capacitor array to adjust the on-time, so that the equivalent input resistance can be adjusted adaptively with a peak tracking efficiency of 99.55%. Huang et al. (2018) proposed a periodic power integrator (PPI) circuit. The input current proportional to the source power is integrated, so that the output level of PPI is increased in case of sufficient source energy. The duty cycle generator with regarding to the output level is then added to extend the on-time, a 99.2% tracking accuracy is achieved. Similarly, the internal power monitor and tracking loop is proposed to automatically adjust the on-time to set the impedance of the power converter to a desired value Bandyopadhyay and Chandrakasan (2012).
Thirdly, better switching control schemes lead to further increasing of PCE. When applied to EH for light load wearable electronics, the MISIMO interfaces typically operate in the discontinuous conduction mode (DCM), which allows changing the input harvester without disrupting the operation of the converter. In DCM, the inductor is energized from zero current and deenergized to zero current without the need of complex compensation techniques. Constant on-time (COT) or adaptive on-time (AOT) control methods are widely adopted in DCM operation. However, the converter cannot obtain the maximum PCE when the input voltage varies with the COT control Liu et al. (2018). Chen et al. (2019c) implemented the AOT scheme with adaptive peak-inductor-current (APIC) to obtain high conversion efficiency under different input voltages. To reduce the contention loss, the switch needs to be turned off when the current is zero. It should be noteworthy that if the power transistors are not turned off in time, the reverse current may be introduced in inductor deenergizing phase (off-time) that adversely affects the PCE. Thus to ensure the exact zero current turning off point is indispensable in DCM. Normally, a comparator is utilized to adjudge the zero current crossing point by comparing the inductor nodes with the reference (supply voltage or ground). As the change of the input or output voltage in each switching cycle leads to a variational off-time, the static comparator is normally employed in the analog-based zero-current detector (ZCD) to detect the zero current crossing point continuously. Nevertheless, the static comparator represents a trade-off between comparison delay and power consumption. To solve the above problem and eliminate reverse current, Chen et al., 2019c employed analog-based ZCD controller consisting of two common-gate comparators with mismatched input pair transistors. The deliberate offset compensates the propagation delay of the comparators in two directions. Similarly, Jung et al. (2020) employed an offset detector to detect the positive or negative offset voltage of the comparator, then calibrate the offset adaptively. The ZCD proposed in Kuai et al. (2019) eases the comparator design as it only has to slice two significantly different voltages and compensate the delay by slicing in advantage of the zero-crossing point. The SSM technique deployed in Amin and Mercier (2018) scaled down the size of the power transistors at light load conditions, increasing the turn-on resistance, thus a node voltage with sufficiently high value can be detected by the low-power comparator. Moreover, the comparators with offset added operate in duty-cycled mode to reduce the power consumption. The digital-based ZCD consisting of the dynamic comparator features of none quiescent current consumption and high bandwidth. However, the rate of the off-time calibration is related to the operation frequency of the dynamic comparator. A delay-locked loop (DLL) is utilized in Chen et al., 2019a to accelerate the calibration. Lee et al., 2020a implemented the constant peak-inductor-current (CPIC) approach to fix the off-time under the same output voltage, where the on-time is adaptively changed with different input voltage to satisfy the voltage-second balance. Therefore the operation duration for the off-time calibration can be minimized thereby reducing the power consumption.
The MISIMO energy harvesting interface applied in WSNs is in charge of delivering the high quality output to power the loads which features of the reduced output voltage ripple and the fast load transient response with a wide load range. On the one hand, the relatively large voltage ripple will reduce the noise immunity of digital circuit and impacts the accuracy in analog signal conditioning. The output voltage ripple is mainly caused by the insufficient energy delivering to the loads. In such scenario, the outputs interact each other when a transition introduced by a certain load. In order to reduce the output voltage ripple, a large off-chip capacitor could be selected, however the volume and cost overhead will be introduced. Charging each load in single cycle is an effective method to suppress the output voltage ripple. The inductor charging time under battery power calibration technique was proposed in Amin and Mercier (2018), where the on-time for indcuctor energized from battery is calibrated with a load current indicator, so that each load receives sufficient energy from the battery in a single cycle, reducing the output voltage ripple. Qian et al. (2017) proposed the control mechanism of charging the dual output in single inductor deenergizing phase, which greatly suppressed the output voltage ripple under light load condition. Homoplastically, The buck-based dual-control mode proposed in Kim et al. (2021) charges the first output in indcuctor energizing phase and the second output in deenergizing phase, which also powers the two outputs in a single period resulting a reduced output voltage ripple. Other methods like 2-D AOT with APIC technique implemented in Lee et al., 2020a keeps a fixed ratio of ripple and output voltage values when the output voltage is changing, so as to reduce the output voltage ripple. Nagateja et al. (2019) employed a H-bridge capacitor to combine the AC and DC source energy before subsequently pumping it up, which ensures a continuous inductor current that reduces output voltage ripple by 67%. On the other hand, fast load transient response with a wide load range is also crucial for MISIMO converter since the each output can vary from micro-watts to milli-watts when switching to the active state. Therefore, Qian et al. (2018) proposed the charge sharing control technique, which adds a switch between the stored super capacitor and the load. When the output voltage drops below the threshold voltage in the heavy load mode, the charge stored in the super capacitor is shared through the direct path, achieving a smaller settling time which leads to fast load transient response. Kim et al. (2018) deployed the event-driven control method, the operated switching frequency can be raised to 25 MHz triggered by the event signal. The high-bandwidth design optimally improve the load transient response. The clock skipping algorithm was proposed in Jung et al. (2020), which optimally controls the number of clock skippings according to the load condition. An increasing the number of clocks occurrences under the condition from light load to heavy load, resulting in a fast transient response for load regulation.
This mini-review article summarizes the techniques in MISIMO EH interface appied in the wearable electronics. In terms of improving the PCE, several methods to reduce the power loss consisting of the conduction loss, switching loss and control loss are introduced. Furthermore, improving the MPPT efficiency and accuracy of ZCD are also the effective approach to improve the PCE. In terms of improving the output quality, several techniques to suppress the output voltage ripple and achieve fast load transient response are presented. The pros and cons of the representative techniques employed in MISIMO EH interfaces are organized in Table 1.
The future trends of MISIMO EH interface are miniaturization by shrinking the size or avoiding the usage of the off-chip components. It is because along with the industrialization and popularization of the wearable electronics, an increasing number of the WSNs would be deployed, the relatively large off-chip components is no longer matched with the smart sensor nodes. One possible solution proposed in battery-based SIMO buck converter Chen and Fayed (2015) is raising the operation frequency, thus all the passive components including the inductor and capacitors could be integrated on-chip, eventually leading to a compact system solution. In addition, the techniques to reduce cross-regulation needs further investigation. Since the multi-input have different electrical characteristics whereas the multi-outputs typically have different load requirements, how to suppress their interaction is still a challenge for MISIMO EH interface. Cross-regulation suppression is relatively well-addressed in battery-based SIMO buck converter while still immature in MISIMO EH interface. In conclusion, it is foreseeable that with the development of the aforementioned techniques, the fully on-chip autonomous WSNs will be generalized in wearable electronics.
All authors listed have made a substantial, direct, and intellectual contribution to the work and approved it for publication.
This research is supported in part by the National Key Research and Development Program of China under Grant No. 2019YFB2204500; and in part by the Shanghai Pujiang Talent under Grant No. 20PJ1408600.
The authors declare that the research was conducted in the absence of any commercial or financial relationships that could be construed as a potential conflict of interest.
All claims expressed in this article are solely those of the authors and do not necessarily represent those of their affiliated organizations, or those of the publisher, the editors and the reviewers. Any product that may be evaluated in this article, orclaim that may be made by its manufacturer, is not guaranteed or endorsed by the publisher.
Amin, S. S., and Mercier, P. P. (2018). Misimo: A Multi-Input Single-Inductor Multi-Output Energy Harvesting Platform in 28-nm Fdsoi for Powering Net-Zero-Energy Systems. IEEE J. Solid-state Circuits. 53, 3407–3419. doi:10.1109/JSSC.2018.2865467
Angelov, P., and Nielsen-Lonn, M. (2020). A Fully Integrated Multilevel Synchronized-Switch-Harvesting-On-Capacitors Interface for Generic Pehs. IEEE J. Solid-state Circuits. 55, 2118–2128. doi:10.1109/JSSC.2020.2979178
Ashraf, M. (2020). A Maximum Power-Point Tracking Multiple-Input Thermal Energy Harvesting Module. AEU - Int. J. Electronics Commun. 121, 153231. doi:10.1016/j.aeue.2020.153231
Bandyopadhyay, S., and Chandrakasan, A. P. (2012). Platform Architecture for Solar, Thermal, and Vibration Energy Combining with Mppt and Single Inductor. IEEE J. Solid-state Circuits. 47, 2199–2215. doi:10.1109/JSSC.2012.2197239
Chen, C.-W., and Fayed, A. (2015). A Low-Power Dual-Frequency Simo Buck Converter Topology With Fully-Integrated Outputs and Fast Dynamic Operation in 45 Nm Cmos. IEEE J. Solid-state Circuits. 50, 2161–2173. doi:10.1109/JSSC.2015.2422782
Chen, I.-C., Liang, C.-W., and Tsai, T.-H. (2019a). A Single-Inductor Dual-Input Dual-Output DC-DC Converter for Photovoltaic and Piezoelectric Energy Harvesting Systems. IEEE Trans. Circuits Syst. 66, 1763–1767. doi:10.1109/TCSII.2019.2921349
Chen, M., Yu, H., Wang, G., and Lian, Y. (2019b). A Batteryless Single-Inductor Boost Converter With 190 Mv Self-Startup Voltage for Thermal Energy Harvesting Over a Wide Temperature Range. IEEE Trans. Circuits Syst. 66, 889–893. doi:10.1109/TCSII.2018.2869328
Chen, P.-H., Cheng, H.-C., and Lo, C.-L. (2019c). A Single-Inductor Triple-Source Quad-Mode Energy-Harvesting Interface With Automatic Source Selection and Reversely Polarized Energy Recycling. IEEE J. Solid-state Circuits. 54, 2671–2679. doi:10.1109/JSSC.2019.2917549
Chen, Z., Law, M.-K., Mak, P.-I., Zeng, X., and Martins, R. P. (2020). Piezoelectric Energy-Harvesting Interface Using Split-Phase Flipping-Capacitor Rectifier With Capacitor Reuse for Input Power Adaptation. IEEE J. Solid-state Circuits. 55, 2106–2117. doi:10.1109/JSSC.2020.2989873
Chowdary, G., Singh, A., and Chatterjee, S. (2016). An 18 Na, 87% Efficient Solar, Vibration and Rf Energy-Harvesting Power Management System With a Single Shared Inductor. IEEE J. Solid-State Circuits. 51, 2501–2513. doi:10.1109/JSSC.2016.2585304
Coustans, M., Krummenacher, F., and Kayal, M. (2019). A Fully Integrated 60 mV Cold-Start Circuit for Single Coil DC-DC Boost Converter for Thermoelectric Energy Harvesting. IEEE Trans. Circuits Syst. 66, 1668–1672. doi:10.1109/TCSII.2019.2922683
Dabbaghian, A., Yousefi, T., Fatmi, S. Z., Shafia, P., and Kassiri, H. (2019). A 9.2-g Fully-Flexible Wireless Ambulatory Eeg Monitoring and Diagnostics Headband With Analog Motion Artifact Detection and Compensation. IEEE Trans. Biomed. Circuits Syst. 13, 1141–1151. doi:10.1109/TBCAS.2019.2936327
Ding, X., Clifton, D., Ji, N., Lovell, N. H., Bonato, P., Chen, W., et al. (2021). Wearable Sensing and Telehealth Technology With Potential Applications in the Coronavirus Pandemic. IEEE Rev. Biomed. Eng. 14, 48–70. doi:10.1109/RBME.2020.2992838
Gao, Z., Chen, M., Liu, K., Zhao, J., Li, Y., and Wang, G. (2021). An Asynchronous AC-DC Boost Converter With Event-Driven Voltage Regulator and 94% Efficiency for Low-Frequency Electromagnetic Energy Harvesting. IEEE Trans. Circuits Syst. 68, 2563–2567. doi:10.1109/TCSII.2021.3055949
Huang, C.-J., Ma, Y.-S., Yang, W.-H., Lin, Y.-T., Kuo, C.-C., Chen, K.-H., et al. (2018). “A 99.2% Tracking Accuracy Single-Inductor Quadruple-Input-Quadruple-Output Buck-Boost Converter Topology With Periodical Interval Perturbation and Observation Mppt,” in 2018 IEEE Asian Solid-State Circuits Conference (A-SSCC), 171–174. doi:10.1109/ASSCC.2018.8579308
Huang, P.-C., and Kuo, T.-H. (2020). A Reconfigurable and Extendable Single-Inductor Single-Path Three-Switch Converter for Indoor Photovoltaic Energy Harvesting. IEEE J. Solid-state Circuits. 55, 1998–2008. doi:10.1109/JSSC.2020.2987722
Jeong, J., Shim, M., Maeng, J., Park, I., and Kim, C. (2020). An Efficiency-Aware Cooperative Multicharger System for Photovoltaic Energy Harvesting Achieving 14% Efficiency Improvement. IEEE Trans. Power Electron. 35, 2253–2256. doi:10.1109/TPEL.2019.2939170
Jung, J.-H., Jung, Y.-H., Hong, S.-K., and Kwon, O.-K. (2020). A High Peak Output Power and High Power Conversion Efficiency Simimo Converter Using Optimal On-Time Control and Hybrid Zero Current Switching for Energy Harvesting Systems in Iot Applications. IEEE Trans. Power Electron. 35, 8261–8275. doi:10.1109/TPEL.2019.2963513
Juteau, N., and Gosselin, B. (2020). Wearable Wireless-Enabled Oscillometric Sphygmomanometer: A Flexible Ambulatory Tool for Blood Pressure Estimation. IEEE Trans. Biomed. Circuits Syst. 14, 1287–1298. doi:10.1109/TBCAS.2020.3026992
Kara, I., Becermis, M., Kamar, M. A.-A., Aktan, M., Dogan, H., and Mutlu, S. (2021). A 70-to-2 V Triboelectric Energy Harvesting System Utilizing Parallel-Sshi Rectifier and Dc-Dc Converters. IEEE Trans. Circuits Syst. 68, 210–223. doi:10.1109/TCSI.2020.3025468
Katic, J., Rodriguez, S., and Rusu, A. (2018). A High-Efficiency Energy Harvesting Interface for Implanted Biofuel Cell and Thermal Harvesters. IEEE Trans. Power Electron. 33, 4125–4134. doi:10.1109/TPEL.2017.2712668
Kim, H., Maeng, J., Park, I., Jeon, J., Lim, D., and Kim, C. (2021). A 90.2% Peak Efficiency Multi-Input Single-Inductor Multi-Output Energy Harvesting Interface With Double-Conversion Rejection Technique and Buck-Based Dual-Conversion Mode. IEEE J. Solid-State Circuits. 56, 961–971. doi:10.1109/JSSC.2020.3025722
Kim, S., Vaidya, V., Schaef, C., Lines, A., Krishnamurthy, H., Weng, S., et al. (2018). “A Single-Stage, Single-Inductor, 6-Input 9-Output Multi-Modal Energy Harvesting Power Management IC for 100µW-120MW Battery-Powered IoT Edge Nodes,” in 2018 IEEE Symposium on VLSI Circuits, 195–196. doi:10.1109/VLSIC.2018.8502301
Kuai, Q., Wan, Q., and Mok, P. K. T. (2019). “A Dual-Frequency Dual-Input-Dual-Output Interface for Thermoelectric Energy Harvesting and Recycling With 82.9% Efficiency,” in ESSCIRC 2019 - IEEE 45th European Solid State Circuits Conference (ESSCIRC), 137–140. doi:10.1109/ESSCIRC.2019.8902863
Le, V. L., Yoo, T., Kim, J. E., Baek, K.-H., and Kim, T. T.-H. (2019). A 213.7-μw Gesture Sensing System-On-Chip With Self-Adaptive Motion Detection and Noise-Tolerant Outermost-Edge-Based Feature Extraction in 65 Nm. IEEE Solid-state Circuits Lett. 2, 123–126. doi:10.1109/LSSC.2019.2935560
Lee, H.-H., Liu, C.-W., Takamiya, M., and Chen, P.-H. (2020a). Single-Inductor Dual-Input Dual-Output Battery-Pv Hybrid System With 2-d Adaptive On-Time Control for Internet of Things. IEEE Trans. Circuits Syst. 67, 1069–1078. doi:10.1109/TCSI.2019.2950330
Lee, S., Gandla, S., Naqi, M., Jung, U., Youn, H., Pyun, D., et al. (2020b). All-day Mobile Healthcare Monitoring System Based on Heterogeneous Stretchable Sensors for Medical Emergency. IEEE Trans. Ind. Electron. 67, 8808–8816. doi:10.1109/TIE.2019.2950842
Liu, C.-W., Lee, H.-H., Liao, P.-C., Chen, Y.-L., Chung, M.-J., and Chen, P.-H. (2018). Dual-Source Energy-Harvesting Interface With Cycle-By-Cycle Source Tracking and Adaptive Peak-Inductor-Current Control. IEEE J. Solid-state Circuits. 53, 2741–2750. doi:10.1109/JSSC.2018.2844358
Maeng, J., Park, I., Shim, M., Jeong, J., and Kim, C. (2021). A High-Voltage Dual-Input Buck Converter With Bidirectional Inductor Current for Triboelectric Energy-Harvesting Applications. IEEE J. Solid-state Circuits. 56, 541–553. doi:10.1109/JSSC.2020.3012991
Martins, G. C., and Serdijn, W. A. (2021). An RF Energy Harvesting and Power Management Unit Operating Over −24 to +15 dBm Input Range. IEEE Trans. Circuits Syst. 68, 1342–1353. doi:10.1109/TCSI.2020.3041175
Nagateja, T., Chen, S.-Q., Chu, L.-C., Chen, K.-H., Lin, Y.-H., Lin, S.-R., et al. (2019). “A Single-Inductor Triple-Output Converter With an Automatic Detection of DC or AC Energy Harvesting Source for Supplying 93% Efficiency and 0.05mV/mA Cross Regulation to Wearable Electronics,” in 2019 IEEE Asian Solid-State Circuits Conference (A-SSCC), 49–52. doi:10.1109/A-SSCC47793.2019.9056895
Niu, S., Liu, Y., Zhou, Y. S., Wang, S., Lin, L., and Wang, Z. L. (2015). Optimization of Triboelectric Nanogenerator Charging Systems for Efficient Energy Harvesting and Storage. IEEE Trans. Electron. Devices. 62, 641–647. doi:10.1109/TED.2014.2377728
Paidimarri, A., and Chandrakasan, A. P. (2017). A Wide Dynamic Range Buck Converter With Sub-nw Quiescent Power. IEEE J. Solid-state Circuits. 52, 3119–3131. doi:10.1109/JSSC.2017.2747217
Qian, Y., Lu, D., He, J., and Hong, Z. (2018). An On-Chip Transformer-Based Self-Startup Hybrid Sidito Converter for Thermoelectric Energy Harvesting. IEEE Trans. Circuits Syst. 65, 1673–1677. doi:10.1109/TCSII.2017.2773564
Qian, Y., Zhang, H., Chen, Y., Qin, Y., Lu, D., and Hong, Z. (2017). A SIDIDO DC-DC Converter With Dual-Mode and Programmable-Capacitor-Array MPPT Control for Thermoelectric Energy Harvesting. IEEE Trans. Circuits Syst. 64, 1. doi:10.1109/TCSII.2016.2627551
Shim, M., Jeong, J., Maeng, J., Park, I., and Kim, C. (2019). Fully Integrated Low-Power Energy Harvesting System With Simplified Ripple Correlation Control for System-On-A-Chip Applications. IEEE Trans. Power Electron. 34, 4353–4361. doi:10.1109/TPEL.2018.2863390
Talkhooncheh, A. H., Yu, Y., Agarwal, A., Kuo, W. W.-T., Chen, K.-C., Wang, M., et al. (2021). A Biofuel-Cell-Based Energy Harvester With 86% Peak Efficiency and 0.25-v Minimum Input Voltage Using Source-Adaptive Mppt. IEEE J. Solid-State Circuits. 56, 715–728. doi:10.1109/JSSC.2020.3035491
Wang, C., Zhao, K., Li, Z., and Xiong, Q. (2020). Single-Inductor Dual-Input Triple-Output Buck-Boost Converter With Clockless Shortest Power Path Control Strategy for IoT Nodes. IEEE Trans. Power Electron. 35, 2044–2052. doi:10.1109/TPEL.2019.2919945
Wang, Y.-H., Huang, Y.-W., Huang, P.-C., Chen, H.-J., and Kuo, T.-H. (2016). A Single-Inductor Dual-Path Three-Switch Converter With Energy-Recycling Technique for Light Energy Harvesting. IEEE J. Solid-state Circuits. 51, 2716–2728. doi:10.1109/JSSC.2016.2598222
Yang, G., Pang, G., Pang, Z., Gu, Y., Mantysalo, M., and Yang, H. (2019). Non-Invasive Flexible and Stretchable Wearable Sensors With Nano-Based Enhancement for Chronic Disease Care. IEEE Rev. Biomed. Eng. 12, 34–71. doi:10.1109/RBME.2018.2887301
Keywords: wearable electronics, wireless sensor nodes, energy harvesting, MISIMO, power conversion efficiency, output quality
Citation: Gao Z, Wang S, Li Y and Chen M (2021) Review of the Multi-Input Single-Inductor Multi-Output Energy Harvesting Interface Applied in Wearable Electronics. Front. Electron. 2:793780. doi: 10.3389/felec.2021.793780
Received: 12 October 2021; Accepted: 10 November 2021;
Published: 06 December 2021.
Edited by:
Sohmyung Ha, New York University Abu Dhabi, United Arab EmiratesReviewed by:
Mohammadreza Ashraf, Shahrood University of Technology, IranCopyright © 2021 Gao, Wang, Li and Chen. This is an open-access article distributed under the terms of the Creative Commons Attribution License (CC BY). The use, distribution or reproduction in other forums is permitted, provided the original author(s) and the copyright owner(s) are credited and that the original publication in this journal is cited, in accordance with accepted academic practice. No use, distribution or reproduction is permitted which does not comply with these terms.
*Correspondence: Mingyi Chen, bXljaGVuQHNqdHUuZWR1LmNu
Disclaimer: All claims expressed in this article are solely those of the authors and do not necessarily represent those of their affiliated organizations, or those of the publisher, the editors and the reviewers. Any product that may be evaluated in this article or claim that may be made by its manufacturer is not guaranteed or endorsed by the publisher.
Research integrity at Frontiers
Learn more about the work of our research integrity team to safeguard the quality of each article we publish.