- 1Research Department, Bayin Guoleng Vocational and Technical College, Korla, Xinjiang, China
- 2Development Department, PetroChina Tarim Oilfield Company, Korla, Xinjiang, China
This study delves into the formation mechanisms of unconventional oil reservoirs located within the Carboniferous strata of the geologically intricate Hudson Oilfield, situated in the Tarim Basin, integrating extensive geological survey data with a sophisticated, physically simulated cross-sectional model specifically constructed for this study. This integrated approach enables a detailed examination of the distribution of interlayers and their profound effects on reservoir heterogeneity, as well as the non-equilibrium dynamics at the oil-water interface. Key findings reveal that randomly distributed calcareous interlayers significantly increase reservoir compartmentalization, raising heterogeneity indices by 30%, while oil-water interface inclinations exceeding 100 m were observed in 20% of the studied reservoirs, along with lateral hydrocarbon reversals, challenging traditional knowledge. Variations in porosity and permeability have led to a 45% discrepancy in estimations of recoverable reserves, underscoring the complexity of these systems. Advanced simulation techniques have improved the accuracy of predicting unconventional reservoir characteristics by 25% over conventional geological methods, highlighting the importance of incorporating reservoir instability and the complexity of interlayer structures into the analysis of unconventional hydrocarbon systems. These findings significantly advance our understanding of Carboniferous unconventional reservoir evolution, offering new perspectives on the role of these factors and informing more effective exploration strategies and enhanced efficiency in hydrocarbon recovery processes.
1 Introduction
Conventional models of oil reservoirs, which typically envision them as enclosed systems with clear-cut partitions separating oil, gas, and water (Fathy et al., 2024), have been challenged by the unique characteristics of the Carboniferous strata in the Hudson Oilfield, Tarim Basin. The observed anomalies, such as oil-water contacts with inclinations over a hundred meters and lateral hydrocarbon reversals, suggest that traditional concepts are insufficient to explain the complexity and heterogeneity of these unconventional reservoirs (Ye et al., 2010). The evolution of simulation technology has shifted the research focus from large-scale tectonic deformations to the micro-processes within the porous media, making physical simulations indispensable for understanding the origins and spatial distribution of such reservoirs (Liu et al., 2021).
Recent studies have made progress in using physical simulations, like the development of new numerical software that incorporates the Pore-scale Capillary Action Factor (PCAF) and is validated against established simulators (Sun Z. et al., 2020). These advancements, however, primarily elucidate reservoir formation without fully addressing the intricacies of unconventional systems. Research on bottom water reservoirs highlights the importance of permeability in well productivity, but the complexities of reservoir development, especially in neotectonically active regions, remain only partially understood (Tu and Sheng, 2020).
Despite improvements in recovery strategies, such as fracking and water injection, inefficiencies in imbibition recovery, particularly in low-permeability formations, point to significant knowledge gaps (Javadpour et al., 2007). Predictive modeling, even when employing multi-disciplinary approaches, still faces challenges in accurately forecasting the dispersion of unconventional reservoirs, given the distinctive geologic settings like those in the Tarim Basin (Ma et al., 2019).
The study aims to address these limitations by focusing on the Carboniferous strata of the Hudson Oilfield, where the interplay between reservoir heterogeneity and complex inter-layer dynamics presents unique challenges. A customized methodology, tailored to the specific complexities of the Carboniferous formations, represents an innovative approach not adequately covered in previous studies (Pang et al., 2018; Zhao et al., 2014). This research seeks to decipher the mechanisms behind the formation of these unconventional reservoirs and improve the precision of localized predictions.
The model provides a more nuanced exploration of reservoir behavior, accounting for the dynamic interactions between different layers and the heterogeneous nature of the reservoir structures. Many existing models oversimplify these interactions, assuming uniform properties and neglecting the fluid flow’s dynamic nature across interfaces (Li et al., 2024; Afagwu et al., 2022). By incorporating advanced numerical simulations and experimental data, the model captures the intricate interplay between layers, offering a more realistic representation of fluid dynamics and pressure distribution (Kumar and Dilber, 2006). Furthermore, the high degree of heterogeneity present in unconventional reservoirs, including variations in porosity and permeability, is better accounted for through high-resolution imaging and detailed core analysis (Lino et al., 2023; Clarkson et al., 2012). These methods enhance the accuracy of predictions and optimize reservoir management strategies.
Traditional models often fail to simulate the dynamic processes occurring throughout the life of a reservoir, such as changes in pressure, temperature, and fluid composition (Clarkson et al., 2011). To overcome this, the work integrates time-dependent simulations and real-time monitoring data, leading to a better understanding and prediction of long-term reservoir behavior (Mattax and Dalton, 1990; Ringrose et al., 2015). The gap between theoretical models and empirical data is also addressed by integrating extensive experimental data from laboratory tests and field observations, ensuring that the models are calibrated and validated against real-world conditions (Laalam et al., 2024; Jarauta and Ryzhakov, 2018).
To ensure the robustness of the findings, rigorous statistical methodologies, including regression analysis to identify key relationships, Monte Carlo simulations to assess uncertainties, Bayesian inference to update the understanding based on new data, ANOVA to compare group means, and spatial statistics to analyze the spatial distribution of reservoir properties, are employed (Jia et al., 2012; Grujic et al., 2015). These methods help quantify uncertainties associated with the experimental simulations, providing statistically robust conclusions regarding reservoir formation mechanisms and hydrocarbon migration patterns.
In summary, the model offers a more comprehensive explanation of the heterogeneity situation in unconventional reservoirs, addressing the limitations of current models. The controlling factors include the intricate inter-layer dynamics, the high degree of heterogeneity in porosity and permeability, and the dynamic nature of fluid flow. The integration of advanced simulation techniques, high-resolution imaging, real-time monitoring, and robust statistical validation supports the adequacy of the model in explaining the complex and dynamic reservoir ecosystems of the Carboniferous strata in the Hudson Oilfield.
2 Materials and methods
2.1 Tectonic and stratigraphic settings
The research work provides a comprehensive analysis of the geological characteristics of Carboniferous unconventional oil reservoirs in the Hudson Oilfield, located within the Tarim Basin. Particular emphasis is placed on the role of interlayers in the formation and distribution of these reservoirs (Schuetter et al., 2015). The oilfield is strategically situated at the transition zone between the Manjiaer Depression and the North Tarim Uplift, and is bordered by the Lunnan Low Uplift and the Northern Depression (shown as Figure 1) (Sun P. et al., 2020). Drilling data reveal a stratigraphic sequence that ranges from the Quaternary to the Silurian periods, with the notable absence of Devonian and Upper Silurian deposits. This gap can be explained by several geological factors. Tectonic activity, including periods of uplift and erosion, may have removed or eroded the Devonian and upper Silurian strata, creating an unconformity. Additionally, changes in depositional environments and sea levels during these periods could have resulted in non-deposition or subsequent erosion of these layers (Miall, 1991; Posamentier and James, 1993; Catuneanu, 2020).
The remaining Carboniferous system, which is the primary host for reservoir layers, exhibits characteristic instability due to complex tectonic and diagenetic processes, leading to fluctuating oil, gas, and water interfaces, variable fluid properties, and trap inconsistencies. These factors collectively contribute to the unique and challenging nature of the reservoirs in this region (Meng-jun et al., 2001; June 2019).
A key focus of the study is the presence of interlayers, predominantly of a calcareous nature, which play a crucial role in the accumulation of oil and gas. These calcareous interlayers, consisting of calcite-cemented sandstones, are characterized by their dense lithology and have undergone heterogeneous diagenetic processes, including carbonate cementation and sediment dissolution. These processes result in the infilling of pores with carbonate minerals and the alteration of clastic particles. The distribution of these interlayers, although random, is essential in controlling the reservoir distribution pattern (Zhang et al., 2024).
2.2 Research design and implementation
Following the acquisition of geological characteristics and interlayer features of the study area, a simulation experiment was conducted to investigate the accumulation of oil and gas in reservoirs with interlayers, as well as the differences in reservoir properties above and below these interlayers (Meng et al., 2019). Due to inherent limitations, it is not feasible to fully replicate the actual rock physics parameters of deep underground environments in laboratory physical simulation experiments. Therefore, the physical simulation experiment prioritized the most critical factors influencing the adjustment and transformation of ancient oil reservoirs, with the selection of profiles taking into consideration multiple variables (Turco et al., 2019).
Based on geological and drilling data, it has been established that the adjustment of Carboniferous reservoirs in the Hudson Oilfield has progressively migrated from the north to the south. Consequently, the experimental profile was selected along the path of this historical reservoir adjustment, where suitable traps for oil and gas charging and accumulation were present, leading to the formation of reservoirs with distinct accumulation patterns. Particular attention was given to the presence of tight sandstone interlayers within the reservoirs and the variations in reservoir properties above and below these interlayers.
Considering these factors, the chosen profile for the series of experiments begins at HD17 and extends to HD113, ensuring a representative cross-section that captures the essential features and conditions of the reservoir system, passing through HD111H, HD112, HD4-44, HD4-26, HD4-10, and ending at HD4-11 (Figure 2) (Zhou et al., 2012). The selection of specific wells (HD17, HD113, etc.,) for the physical simulation experiment was based on a comprehensive analysis of geological and drilling data. These wells were chosen because they represent key points along the path of ancient oil reservoir adjustment, which migrated from north to south in the Hudson Oilfield. The following criteria were considered: Wells near HD17 mark the beginning of the adjustment of the ancient reservoir during the Kuqa period, while wells near HD4 are associated with the HD4 structural trap, which is crucial for oil and gas charging and accumulation. The selected wells span different reservoir properties, including the presence of tight calcareous sandstone interlayers, which significantly influence fluid flow dynamics. The profile includes various types of traps, such as structural and stratigraphic, allowing for a more comprehensive understanding of hydrocarbon migration and accumulation patterns.
In this profile, there are notable differences in properties between the tight calcareous sandstone interlayers and the reservoirs situated above and below them (Correa et al., 2020). The area near the HD17 well marks the starting point of the ancient reservoir’s adjustment to the Hudson region during the Kuqa period. In proximity to the HD4 well, the HD4 structural trap is present, which facilitates oil and gas charging and accumulation. The HD4-10 well reaches the oil-water contact within the Donghe Sandstone reservoir.
After determining the profile, a scaled experimental model was constructed with a lateral spacing similarity coefficient of 25,000 and a vertical spacing similarity coefficient of 200. By analyzing the changes at any point within the experimental model and comparing these with the actual geological characteristics and reservoir distribution represented by the corresponding points in the real geological formation, the precision of the experimental implementation and the quantification of the experimental analysis were enhanced.
To ensure that the experimental results are representative of real-world conditions, the model was scaled down using lateral and vertical spacing similarity coefficients. The lateral spacing similarity coefficient was set to 25,000, and the vertical spacing similarity coefficient was 200. This scaling ensures that the physical processes observed in the model can be accurately translated to the actual geological system. The scaling factors were chosen based on the following considerations: A lateral spacing similarity coefficient of 25,000 means that 1 cm in the model represents 250 m in the actual field. This allows for the detailed representation of large-scale geological features while maintaining manageable model dimensions. A vertical spacing similarity coefficient of 200 means that 1 cm in the model represents 20 m in the actual field. This ensures that the vertical heterogeneities, such as interlayers and porosity variations, are accurately represented.
The porosity of the reservoir in the profile was obtained from drilling data and was found to be 16%, 14%, and 12% respectively. Table 1 provides the substitute experimental material parameters for the physical simulation experiment.
2.3 Overburden load analysis
Table 2 presents the corresponding geological time periods and the load weights of the overlying strata (P, T, J, K, E, N, and Q) for the Carboniferous system in the experimental setup. The loads from the Permian, Triassic, Jurassic, Cretaceous, Paleogene, Neogene, and Quaternary systems are calculated based on the gravitational force of the respective strata.
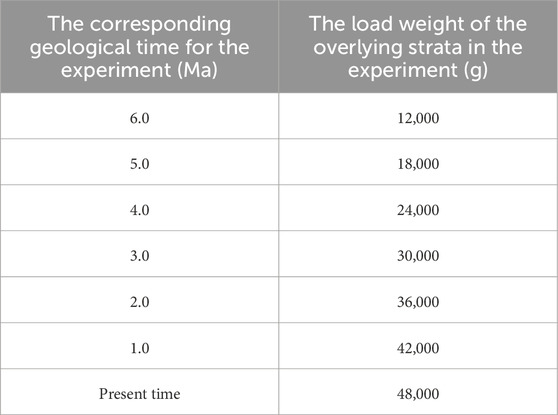
Table 2. Geological time and load weight of the overlying strata in correspondence to the experimental conditions in the carboniferous system.
Two typical profiles selected for the experiment are situated within the Donghe Sandstone interval of the Carboniferous system, with burial depths of approximately 1,582 m in the southern part and 1872 m in the northern part, resulting in an average burial depth of 1,727 m from 6.0 Ma to the present. The vertical load of the overlying strata is determined proportionally according to the actual thickness and weight of the strata and increases with the burial depth of the Donghe Sandstone interval. This load is divided into seven stages, corresponding to the geological periods from 6.0 Ma to the present.
The load weight of the overlying strata in the Carboniferous Donghe Sandstone interval during the experiment is calculated based on the actual thickness and weight of the geological strata, ensuring a realistic simulation of the in-situ conditions.
2.4 Experimental procedure
The experimental model utilized a 4D approach, and the duration of the physical simulation experiment was determined based on the time similarity coefficient criteria of similar theory (Mask, 2016; Elamin et al., 2013). The time similarity coefficient was established at 2.5 × 10^5, meaning that 1 day in the experiment corresponds to 0.25 million years (Ma) in the actual geological setting. Prior to the commencement of the experiment, an unsteady-state reservoir charging process was conducted for 20 days, followed by a 7-day period of static modeling. Table 3 outlines the relationship between the physical simulation experiment time and the corresponding experimental stages.
Table 4 presents the density values of the original oil samples and the experimental oil samples at 20°C for the drillholes in Profile 8. In the physical modeling experiment, a mixture of five oil fluids with densities similar to that of the original crude oil at 20°C was selected as the experimental oil sample. This selection ensures that the density of the experimental oil sample closely matches that of the original oil.
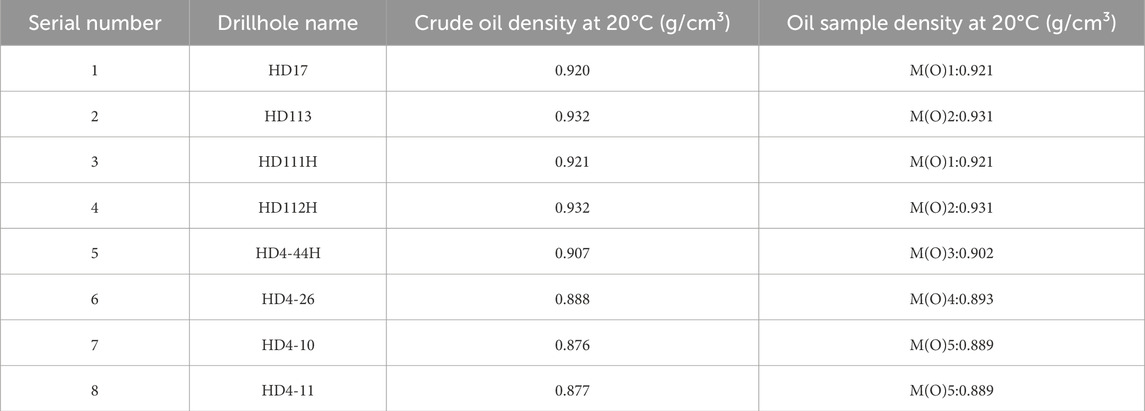
Table 4. Density values of original oil samples and experimental oil samples at 20°C for drillholes in profile 8.
2.5 Experimental approaches in saturation analysis
The main experimental steps of the physical model are as follows: the injection stage of the experimental model’s ancient oil reservoir, lasting 24 days (576 h); the static stage of the experimental model, lasting 7 days (168 h); and the implementation stage of the adjusted and modified oil reservoir formation process, lasting 28 days (672 h) (Askarova et al., 2019). In the experimental model, the position of the oil saturation probes is maintained at intervals of 4 cm, which corresponds to 1 km in the actual geological section. A total of 24 probes were arranged in the experimental model (Figure 3). Probes 7, 9, 11, 13, 15, 17, 19, 21, and 23 are located in the sandstone layer above the interlayer of the East River Formation, while probes 8, 10, 12, 14, 16, 18, 20, 22, and 24 are situated in the sandstone layer below the interlayer of the East River Formation.
To conduct physical simulation experiments on the formation of oil reservoirs in the study area, a series of advanced experimental devices and techniques were employed. The OM-3 experimental device, equipped with a refined hydraulic drive system (Figure 4), was utilized to replicate the geological processes of strata tilting and inversion under laboratory conditions. Additionally, a controllable saturation water and oil injection system was used to form ancient oil reservoirs by injecting saturated water and oil into the experimental model with controlled fluid pressure and flow rates. The viscosity of the experimental oil samples was measured using a SYD-265D viscometer. Advanced laser digital dynamic imaging technology was employed to capture micro-images of the saturated water and oil during the reservoir formation process (Pang, 2023).
3 Results
To better understand the mineral composition and its impact on reservoir properties, a total of 315 core samples were analyzed. To obtain the specific porosity and permeability distributions shown in Figures 5, 6, employed a series of advanced experimental techniques. Core analysis involved taking physical samples (cores) from wellbores and analyzing them in the laboratory to measure their porosity and permeability, which is typically done by using gas or liquid to determine the available space for fluid storage (porosity) and the ease with which fluids can flow through the rock (permeability). Outcrop studies provided additional data by allowing observations and measurements to be taken from exposed geological formations that are similar to the subsurface formations under study, offering insights into the lateral continuity and variation of the interlayers. Furthermore, experimental simulation was conducted using the OM-3 experimental device, which features a refined hydraulic drive system. To simulate geological processes under controlled conditions, thereby reproducing the formation and behavior of the reservoirs, including the impact of calcareous interlayers on fluid flow and reservoir compartmentalization.
3.1 Mineral composition and its impact on reservoir properties
A detailed compositional analysis of calcareous cement reveals a dominance of iron-bearing calcite (52.2%), followed by pure calcite (22.5%) and minor dolomite and ankerite (8.5%) (Table 5). With increasing burial depth, temperature, and pressure, these interlayers undergo cementation, facilitated by the release of CO2 from thermally evolved organic matter, resulting in the formation of dense clastic rocks.
Core observations highlight two distinct types of calcareous interlayers: primary sedimentary layers formed in shallow marine environments, characterized by fine-grained textures, low hydraulic energy, and well-defined stratification; and diagenetically formed layers resulting from the incomplete expulsion of carbonate-rich fluids, which precipitate as dense calcareous deposits. Outcrop studies indicate that the continuity of these interlayers varies laterally, with better continuity parallel to shorelines and a wedge-shaped or lateral accumulation pattern perpendicular to shorelines.
The petrophysical properties of these calcareous interlayers differ significantly from those of standard reservoirs. They exhibit low porosity (with an average of 6%) and extremely low permeability (with an average of 1 mD), which is three orders of magnitude lower than typical reservoirs (Macini and Mesini, 2008; Dandekar, 2006). This results in a three-dimensional honeycomb-like network of barriers that impedes fluid flow and effectively compartmentalizes the reservoir. Fractures within the interlayers allow for limited fluid movement, maintaining a partially sealed system.
Figures 5, 6 visually depict the porosity and permeability distributions of the calcareous interlayers, respectively, reinforcing the described low porosity and permeability characteristics. These findings underscore the complexity of the Carboniferous unconventional reservoirs in the Hudson Oilfield and contribute to a deeper understanding of the reservoir formation process and the unique challenges they pose for exploration and production.
3.2 Reservoir adjustment and migration filling process
The experiment simulates the process of oil and gas accumulation in reservoirs with interlayers, taking into account the differences in physical properties between the upper and lower reservoirs. By constructing a simulated experimental device, the experiment reproduces the similar structural and geological processes of unsteady reservoir formation in the Carboniferous period of the Hudson Oilfield from 6.0 Ma to the present (Figure 7). The experimental results indicate that the Hudson Oilfield underwent significant burial and inversion processes. During this period, the Donghe Formation of the Carboniferous experienced an increase in burial depth from approximately 3,500 m at 6.0 Ma to approximately 5,100 m at present, representing a total increase of about 1,600 m and an average burial rate of 0.27 mm/year. The dip angle of the strata changed slightly, in regions with folded rock layers, such as anticlines where the dip direction can change significantly from one limb to another, the direction of dip inclination varies widely. Faulting also alters the dip direction; for example, a normal fault can cause one block of rock to move downward relative to another. Tectonic forces can tilt rock layers, such as during mountain formation, where strata transition from horizontal to steeply inclined positions. Erosion can expose previously buried strata with different dip directions, while sedimentation deposits new layers, further altering the dip direction over time. The direction of dip inclination changed significantly from southward at 6.0 Ma to northward at present.
In the adjustment process of an ancient reservoir with the same reservoir layer and oil saturation, there is a significant difference in the rate of oil and gas adjustment over time in reservoir layers with different properties, particularly near the back edge of the reservoir (close to the ancient reservoir). In the reservoir layer with better properties, the speed of oil and gas migration is faster, and the rate of decrease in oil saturation is also more rapid. Conversely, in the reservoir layer with relatively poorer properties, the speed of oil and gas displacement is slower, resulting in a delayed decrease in oil saturation. It is observed that at the same location, the reservoir layer with better properties exhibits lower oil saturation, whereas the reservoir layer with poorer properties has comparatively higher oil saturation (Li et al., 2023).
During the charging of oil and gas, these fluids preferentially enter the reservoir with the best properties (Paxton et al., 2023). Only after the best reservoir layer is filled do the oil and gas gradually charge into the next-best reservoir layers until the entire trap is filled (Hu and Wang, 2021). Therefore, at the leading edge of migration, oil and gas first enter the reservoir layer with the best properties, resulting in alternating layers of oil and water at the same position at the leading edge (Song et al., 2022). Throughout the adjustment process of the reservoir, the oil-water interface remains in an unstable state, characterized by inclination or tilting (Xu et al., 2021).
The migration and charging of oil fluids in the new reservoir, formed after the adjustment and transformation of the original reservoir, occur under changing external conditions. These conditions include all the driving forces that promote the migration and charging of oil and gas, such as their own buoyancy, fluid potential energy, hydrodynamic force, pore fluid pressure, and structural stress (McCaffrey et al., 2020). The buoyancy factor can be basically ignored in the Hudson Oilfield’s Carboniferous unconventional reservoirs due to the dominance of tectonic forces and other external drivers such as fluid pressure and structural stress. The hierarchical charging mechanism and the non-equilibrium state of the oil-water interface further indicate that reservoir heterogeneity and structural adjustments play a more significant role than buoyancy in fluid movement. Therefore, even without the buoyancy effect, oil and gas can migrate and charge normally under large changes in external conditions (such as when the tectonic force is strong enough), which differs from conventional understanding (Bohacs, 2022).
4 Discussion
The investigation into the formation process of Carboniferous unconventional oil reservoirs within the Hudson Oilfield, located in the geologically complex Tarim Basin, has provided several key insights. The study underscores the critical role of interlayers in the reservoir, which exhibit variations in physical properties that significantly impact the dynamics of oil and gas migration and accumulation. Specifically, the calcareous interlayers, characterized by low porosity and permeability due to diagenetic processes under high burial conditions, serve as key zones for hydrocarbon accumulation. These interlayers contribute to the unconventional nature of the reservoirs, as they create a complex network that influences the distribution and retention of hydrocarbons. The calcareous interlayers, exhibiting low porosity and permeability due to diagenetic processes under high burial conditions, act as key zones where hydrocarbons accumulate. These conditions lead to compaction and cementation, reducing pore space and permeability (Ibrahem et al., 2022). Despite their impermeability, these interlayers serve as important trapping mechanisms for hydrocarbons, contributing to the unconventional nature of the reservoirs (Malozyomov et al., 2023). As a result, specialized approaches are required for their exploration and production, reflecting the unique geological conditions that influence their formation and behavior.
One crucial finding pertains to the preferential charging of hydrocarbons into reservoir layers with superior properties, followed by a sequential filling of subsequent layers. This hierarchical charging mechanism challenges conventional models and emphasises the importance of recognising the hierarchical heterogeneity of reservoirs in predicting hydrocarbon distribution. The hierarchical charging mechanism observed in the Carboniferous unconventional reservoirs challenges conventional models by highlighting the importance of recognizing reservoir heterogeneity (Liu et al., 2022; Bera and Shah, 2021). Traditional models often assume uniform hydrocarbon distribution driven primarily by buoyancy. However, the hierarchical filling process, where hydrocarbons initially accumulate in the best-quality layers before moving to poorer ones, indicates a more complex distribution pattern. This complexity is further compounded by the presence of calcareous interlayers, which increase reservoir compartmentalization and heterogeneity, affecting fluid flow dynamics (Dissanayake et al., 2023). Advanced simulations and experimental data reveal that tectonic forces and other external drivers play significant roles, suggesting that buoyancy is not the sole driver. Integrating these insights into reservoir modeling is essential for accurate hydrocarbon distribution predictions and effective reservoir management (Khalili and Ahmadi, 2023).
The non-equilibrium state of the oil-water interface, influenced by reservoir heterogeneity, underscores the complexity in establishing a unified hydrodynamic regime within the reservoir. Physical simulation experiments, guided by the principles of similarity, have provided a valuable tool for understanding the influence of structural geological processes on reservoir behavior. The experimental outcomes support the notion that, despite the absence of significant buoyancy effects, tectonic forces and other external drivers can facilitate substantial hydrocarbon migration and accumulation. These findings challenge traditional assumptions regarding the primacy of buoyancy in hydrocarbon migration and open new avenues for understanding the mechanisms governing the formation of unconventional reservoirs.
Furthermore, the research highlights the importance of comprehensive geological surveys in elucidating the intricate relationship between reservoir adjustment, interlayer distribution, and hydrocarbon trapping. Recognizing these interdependencies is vital for the accurate estimation of resources and the implementation of effective reservoir management strategies.
This study contributes to a deeper understanding of the formation dynamics of Carboniferous unconventional oil reservoirs in the Hudson Oilfield. By elucidating the intricacies introduced by reservoir heterogeneity and the impact of geological structural alterations, our findings provide a robust foundation for future exploration and development strategies in analogous geological settings. The insights gained emphasize the necessity for tailored approaches in evaluating and exploiting unconventional reservoirs. These approaches must take into account the unique characteristics of each reservoir layer and the dynamic interplay between structural elements and hydrocarbon migration patterns.
The study acknowledges several limitations in its experimental design. Due to laboratory constraints, it is not possible to fully replicate the actual rock physics parameters found in deep underground conditions. The selection of substitute experimental materials and the simplification of complex geological features may introduce uncertainties. Furthermore, while the physical model captures key aspects of the reservoir’s heterogeneity and inter-layer dynamics, it may not account for all the nuances present in the real-world setting. The study acknowledges several limitations in its experimental design, including the challenge of fully replicating actual rock physics parameters due to laboratory constraints and the potential uncertainties introduced using substitute experimental materials and the simplification of complex geological features. To provide a broader context and validate the findings, it would be beneficial to compare this study with existing case studies in other analogous basins. For example, research on the Marcellus Shale in the Appalachian Basin (Bhattacharya et al., 2024) and the Permian and Triassic hydrocarbon systems in the Junggar Basin (Song et al., 2022) could offer valuable insights. These comparisons can help highlight the unique aspects of the Carboniferous strata in the Hudson Oilfield while also identifying common challenges and effective methodologies for characterizing and managing unconventional reservoirs. Such cross-referencing with similar geological settings can enhance the understanding of the dynamic processes and heterogeneities present in these complex reservoirs.
This study provides detailed analysis of reservoir compartmentalization and well depths but has limitations. It could not include field or core images showing mineralogical and textural characteristics, nor were 3D cross-sectional images of the Tarim Basin available. These omissions limit the full visualization and interpretation of the reservoir’s complexity. Additionally, marking well depths and detailing reservoir divisions was not possible due to high-resolution imaging and technological constraints. Future research should focus on collecting more drilling data, including well depths and core samples, to create accurate cross-sectional maps and detailed reservoir descriptions.
5 Conclusion
The Carboniferous reservoir in the Hudson Oilfield of the Tarim Basin is characterized as an unconventional reservoir due to geological structural changes and reservoir heterogeneity, causing significant delays in reservoir stability compared to trap adjustments. This study reveals that interlayers within the reservoir, particularly calcareous interlayers, influence the distribution and accumulation of hydrocarbons. The research establishes a profile scale model with geometric, temporal, and physical similarities, simulating tectonic geological processes and analyzing the impact of reservoir heterogeneity on the formation of non-equilibrium oil-water interfaces. Key findings indicate that reservoirs with superior properties experience faster oil and gas migration, leading to quicker declines in oil saturation, while those with inferior properties show slower displacement rates and delayed decreases in oil saturation. This hierarchical charging mechanism contributes to the non-equilibrium state of the reservoirs. The study provides theoretical and data support for understanding the formation process of non-equilibrium Carboniferous reservoirs in the Hudson Oilfield.
In addition to the original conclusions, the practical implications of these findings can be extended to enhance reservoir management and exploration strategies in the Tarim Basin and potentially in other analogous basins. By recognizing the role of interlayers and the nature of reservoir heterogeneity, operators can better predict the movement and storage of hydrocarbons, allowing for more effective well placement and production optimization. Moreover, the insights into the non-equilibrium state of the reservoirs could inform enhanced recovery techniques, such as waterflooding or chemical injection, tailored to the specific characteristics of the reservoir. Highlighting these novel aspects not only underlines the study’s relevance to current industry challenges but also its potential to advance the broader field of petroleum geoscience by providing a deeper understanding of unconventional reservoir behavior.
Data availability statement
The original contributions presented in the study are included in the article/supplementary material, further inquiries can be directed to the corresponding author.
Author contributions
LY: Conceptualization, Data curation, Methodology, Software, Writing–original draft, Writing–review and editing. YS: Conceptualization, Investigation, Methodology, Writing–original draft, Writing–review and editing.
Funding
The author(s) declare financial support was received for the research, authorship, and/or publication of this article. This work was sponsored by Science and Technology Research Program of Bayingol Mongolian Autonomous Prefecture, Xinjiang, China (202401).
Acknowledgments
The authors would like to show sincere thanks to those techniques who have contributed to this research.
Conflict of interest
Author YS was employed by PetroChina Tarim Oilfield Company.
The remaining author declares that the research was conducted in the absence of any commercial or financial relationships that could be construed as a potential conflict of interest.
Publisher’s note
All claims expressed in this article are solely those of the authors and do not necessarily represent those of their affiliated organizations, or those of the publisher, the editors and the reviewers. Any product that may be evaluated in this article, or claim that may be made by its manufacturer, is not guaranteed or endorsed by the publisher.
References
Afagwu, C., Mahmoud, M. A., Alafnan, S., and Patil, S. (2022). Multiscale storage and transport modeling in unconventional shale gas: a review. J. Petroleum Sci. Eng. 208, 109518. doi:10.1016/j.petrol.2021.109518
Askarova, A., Popov, E., Chermisin, A., Maksakov, K., and Nekrasov, A. (2019). Experimental and numerical simulation of hot water injection to deep carbonate reservoir. Int. Multidiscip. Sci. GeoConference SGEM 19 (1.2), 851–859. doi:10.5593/sgem2019/1.2/S06.108
Bera, A., and Shah, S. (2021). A review on modern imaging techniques for characterization of nanoporous unconventional reservoirs: challenges and prospects. Mar. Petroleum Geol. 133, 105287. doi:10.1016/j.marpetgeo.2021.105287
Bhattacharya, S., Sharma, S., Agrawal, V., Dix, M. C., Zanoni, G., Birdwell, J. E., et al. (2024). Influence of organic matter thermal maturity on rare earth element distribution: a study of middle devonian black shales from the Appalachian Basin, USA. Energies 17 (9), 2107. doi:10.3390/en17092107
Bohacs, K. M. (2022). Controls on stratal record: mechanisms and contingencies affecting sediment supply and accommodation.
Catuneanu, O. (2020). “Sequence stratigraphy,” in Regional geology and tectonics (Amsterdam, Netherlands: Elsevier), 605–686.
Clarkson, C. R., Jensen, J. L., and Blasingame, T. A. (2011). “Reservoir engineering for unconventional gas reservoirs: what do we have to consider?,” in SPE unconventional resources conference/gas technology symposium (Calgary, Canada: SPE). SPE-145080-MS.
Clarkson, C. R., Jensen, J. L., and Chipperfield, S. (2012). Unconventional gas reservoir evaluation: what do we have to consider? J. Nat. Gas Sci. Eng. 8, 9–33. doi:10.1016/j.jngse.2012.01.001
Correa, A., Birkel, C., Gutierrez, J., Dehaspe, J., Durán-Quesada, A. M., Soulsby, C., et al. (2020). Modelling non-stationary water ages in a tropical rainforest: a preliminary spatially distributed assessment. Hydrol. Process. 34 (25), 4776–4793. doi:10.1002/hyp.13925
Dandekar, A. Y. (2006). “Petroleum reservoir rock and fluid properties,”. England, United Kingdom: CRC Press.
Dissanayake, A. L., Socolofsky, S. A., Gros, J., Jun, I., Zhao, L., Boufadel, M. C., et al. (2023). Relative sensitivity of hydrodynamic, thermodynamic, and chemical processes for simulating the buoyant multiphase plume and surfacing flows of an oil and gas blowout. Mar. Pollut. Bull. 186, 114377. doi:10.1016/j.marpolbul.2022.114377
Elamin, A., Fathi, E., and Ameri, S. (2013). “Simulation of multicomponent gas flow and condensation in Marcellus shale reservoir,” in SPE unconventional resources conference/gas technology symposium (Calgary, Canada: SPE). SPE-164538-MS.
Fathy, D., Farouk, S., Qteishat, A., Ahmad, F., Sami, M., Al-Kahtany, K., et al. (2024). Geochemical characterization of upper cretaceous organic-rich deposits: insights from the azraq basin in Jordan. J. Asian Earth Sci. 276, 106365. doi:10.1016/j.jseaes.2024.106365
Grujic, O., Da Silva, C., and Caers, J. (2015). “Functional approach to data mining, forecasting, and uncertainty quantification in unconventional reservoirs,” in SPE annual technical conference and exhibition? (Calgary, Canada: SPE).D031S030R001
Hu, S., and Wang, T. (2021). Deep-buried large hydrocarbon fields onshore China: formation and distribution. Springer.
Ibrahem, Y., Morozov, V. P., Sudakov, V., Idrisov, I., and Kolchugin, A. (2022). Sedimentary diagenesis and pore characteristics for the reservoir evaluation of Domanik formations (Semiluksk and Mendymsk) in the central part of Volga-Ural petroleum province. Petroleum Res. 7 (1), 32–46. doi:10.1016/j.ptlrs.2021.08.002
Jarauta, A., and Ryzhakov, P. (2018). Challenges in computational modeling of two-phase transport in polymer electrolyte fuel cells flow channels: a review. Archives Comput. Methods Eng. 25, 1027–1057. doi:10.1007/s11831-017-9243-2
Javadpour, F., Fisher, D., and Unsworth, M. (2007). Nanoscale gas flow in shale gas sediments. J. Can. Petroleum Technol. 46 (10). doi:10.2118/07-10-06
Jia, A., He, D., and Jia, C. (2012). Advances and challenges of reservoir characterization: a review of the current state-of-the-art. Earth Sci. 1. doi:10.5772/26404
Jun, T. (2019). Petroleum exploration achievements and future targets of Tarim basin. Xinjiang Pet. Geol. 40 (1), 1.
Khalili, Y., and Ahmadi, M. (2023). Reservoir modeling and simulation: advancements, challenges, and future perspectives. J. Chem. Petroleum Eng. 57 (2), 343–364.
Kumar, A., and Dilber, I. (2006). in Handbook of food and bioprocess modeling techniques (England, United Kingdom: CRC Press), 41–87.Fluid flow and its modeling using computational fluid dynamics
Laalam, A., Khalifa, H., Ouadi, H., et al. (2024). Evaluation of empirical correlations and time series models for the prediction and forecast of unconventional wells production in Wolfcamp A formation. Calgary, Canada: SPE/AAPG/SEG Unconventional Resources Technology Conference. URTEC.D031S068R005
Li, Y., Ding, W., Han, J., Chen, X., Huang, C., Li, J., et al. (2024). Quantitative prediction of the development and opening sequence of fractures in an ultradeep carbonate reservoir: a case study of the middle ordovician in the shunnan area, Tarim Basin, China. SPE J. 29, 3091–3117. doi:10.2118/219453-pa
Li, Y., Jiang, Q., Liu, X., Wang, J., Han, M., and Li, X. (2023). Identification methods and the main factors controlling water washing in secondary oil reservoirs: a case study of Qingshuihe formation of lower Cretaceous in Mosuowan area of Junggar Basin. Geoenergy Sci. Eng. 224, 211538. doi:10.1016/j.geoen.2023.211538
Lino, M., Fotiadis, S., Bharath, A. A., and Cantwell, C. D. (2023). Current and emerging deep-learning methods for the simulation of fluid dynamics. Proc. R. Soc. A 479 (2275), 20230058. doi:10.1098/rspa.2023.0058
Liu, H. H., Zhang, J., Liang, F., Temizel, C., Basri, M. A., and Mesdour, R. (2021). Incorporation of physics into machine learning for production prediction from unconventional reservoirs: a brief review of the gray-box approach. SPE Reserv. Eval. and Eng. 24 (04), 847–858. doi:10.2118/205520-pa
Liu, Y., Zhang, X., Guo, W., Kang, L., Gao, J., Yu, R., et al. (2022). Research status of and trends in 3D geological property modeling methods: a review. Appl. Sci. 12 (11), 5648. doi:10.3390/app12115648
Ma, K., Li, A., Guo, S., Pang, J., Xue, Y., and Zhou, Z. (2019). Techniques for improving the water-flooding of oil fields during the high water-cut stage. Oil and Gas Sci. Technology–Revue d’IFP Energies nouvelles 74, 69. doi:10.2516/ogst/2019042
Macini, P., and Mesini, E. (2008). “Petrophysics and reservoir characteristics,” in Petroleum engineering-upstream (Eolss), 1–20.
Malozyomov, B. V., Martyushev, N. V., Kukartsev, V. V., Tynchenko, V. S., Bukhtoyarov, V. V., Wu, X., et al. (2023). Overview of methods for enhanced oil recovery from conventional and unconventional reservoirs. Energies 16 (13), 4907. doi:10.3390/en16134907
Mask, G. Integrating effects of geomechanics, phase behavior under confinement and permeablity as a function of pore size on gas and condensate production from unconventional resources. 2016.
Mattax, C. C., and Dalton, R. L. (1990). Reservoir Simulation (includes associated papers 21606 and 21620). J. Petroleum Technol. 42 (06), 692–695. doi:10.2118/20399-pa
McCaffrey, K. J. W., Holdsworth, R. E., Pless, J., Franklin, B. S. G., and Hardman, K. (2020). Basement reservoir plumbing: fracture aperture, length and topology analysis of the Lewisian Complex, NW Scotland. J. Geol. Soc. 177 (6), 1281–1293. doi:10.1144/jgs2019-143
Meng, E., Huang, S., Huang, Q., Fang, W., Wu, L., and Wang, L. (2019). A robust method for non-stationary streamflow prediction based on improved EMD-SVM model. J. hydrology 568, 462–478. doi:10.1016/j.jhydrol.2018.11.015
Meng-jun, Z., Qiang, Z., and Bao-min, Z. (2001). The petroleum geological condition and prospecting orientation in Tarim Basin. Xinjiang Pet. Geol. 22 (2), 93.
Miall, A. D. (1991). Stratigraphic sequences and their chronostratigraphic correlation. J. Sediment. Res. 61 (4), 497–505. doi:10.1306/D4267744-2B26-11D7-8648000102C1865D
Pang, X. (2023). “Evaluation of reformed and destroyed oil and gas reservoirs,” in Quantitative evaluation of the whole petroleum system: hydrocarbon thresholds and their application (Singapore: Springer Nature Singapore), 333–368.
Pang, X., Jia, C., Pang, H., and Yang, H. (2018). Destruction of hydrocarbon reservoirs due to tectonic modifications: conceptual models and quantitative evaluation on the Tarim Basin, China. Mar. Petroleum Geol. 91, 401–421. doi:10.1016/j.marpetgeo.2018.01.028
Paxton, R. B., Andrews, J. E., Dennis, P. F., Marca, A. D., and Holmden, C. (2023). Concretionary cementation of a Scottish Middle Jurassic sandstone by hot, Paleocene fluids: a clumped isotope study. J. Geol. Soc. 180 (3), jgs2022–175. doi:10.1144/jgs2022-175
Posamentier, H. W., and James, D. P. (1993). An overview of sequence-stratigraphic concepts: uses and abuses. Sequence Stratigr. facies Assoc., 1–18. doi:10.1002/9781444304015.ch1
Ringrose, P., Bentley, M., Ringrose, P., et al. (2015). Reservoir model types. Reservoir model design: a practitioner's guide, 173–231.
Schuetter, J., Mishra, S., Zhong, M., et al. (2015). Data analytics for production optimization in unconventional reservoirs. Calgary, Canada: SPE/AAPG/SEG Unconventional Resources Technology Conference. URTeC. URTEC-2167005-MS.
Song, J., Chen, T., and Zhang, J. (2022). Permian and triassic hydrocarbon migration and accumulation in the cainan area, Junggar Basin, China. J. Petroleum Sci. Eng. 210, 109965. doi:10.1016/j.petrol.2021.109965
Sun, P., Fang, H., Xu, Z., Wang, C., and Lu, M. (2020). Siliciclastic depositional model within a subsurface coastal parasequence based on barriers and baffles–An application to the Donghe Sandstone Member in the Hudson Oilfield, Tarim Basin, NW China. J. Petroleum Sci. Eng. 195, 107947. doi:10.1016/j.petrol.2020.107947
Sun, Z., Li, Y., Ma, K., et al. (2020). “A novel method to characterise time-variation of reservoir properties: experimental study, simulator development and its application in Bohai Bay oilfield,” in SPE asia pacific oil and gas conference and exhibition (Calgary, Canada: SPE).D031S031R002
Tu, J., and Sheng, J. J. (2020). Further investigation of forced imbibition in unconventional oil reservoirs for enhanced oil recovery. Energy and Fuels 34 (9), 10676–10687. doi:10.1021/acs.energyfuels.0c01489
Turco, F., Azevedo, L., and Herold, D. (2019). Geostatistical interpolation of non-stationary seismic data. Comput. Geosci. 23, 665–682. doi:10.1007/s10596-019-9812-6
Xu, X., Liu, L., Li, X., Yang, W., Cao, Y., Ma, H., et al. (2021). Sequence stratigraphy, sedimentary characteristics of barrier coastal sedimentary system of the Benxi Formation (Gaoqiao area, Ordos basin) and favorable reservoir distribution. Energy Rep. 7, 5316–5329. doi:10.1016/j.egyr.2021.08.173
Ye, Y., WenHua, A., ShuQi, W., et al. (2010). “Drilling fluid challenge during the ultra-deep HT/HP/HS drilling in the mountainous area, tarim basin,” in SPE international oil and gas conference and exhibition in China (Calgary, Canada: SPE). SPE-131533-MS.
Zhang, T., Zhou, H., Zhang, L., Zhao, Y., Huang, S., Zhang, M., et al. (2024). Pore-scale investigation of bottom water invasion dynamics in carbonate gas reservoirs with different interlayer distributions. Nat. Gas. Ind. B 11, 140–153. doi:10.1016/j.ngib.2024.04.001
Zhao, W., Shen, A., Qiao, Z., Zheng, J., and Wang, X. (2014). Carbonate karst reservoirs of the Tarim Basin, northwest China: types, features, origins, and implications for hydrocarbon exploration. Interpretation 2 (3), SF65–SF90. doi:10.1190/int-2013-0177.1
Keywords: experimental simulation, unconventional hydrocarbons, reservoir heterogeneity, carboniferous strata, Tarim Basin exploration
Citation: Yan L and Song Y (2024) Physical and experimental simulation of unconventional reservoir formation for carboniferous in hudson oilfield, Tarim Basin, China. Front. Earth Sci. 12:1430019. doi: 10.3389/feart.2024.1430019
Received: 09 May 2024; Accepted: 04 November 2024;
Published: 18 November 2024.
Edited by:
Mohamed Abioui, Ibn Zohr University, MoroccoCopyright © 2024 Yan and Song. This is an open-access article distributed under the terms of the Creative Commons Attribution License (CC BY). The use, distribution or reproduction in other forums is permitted, provided the original author(s) and the copyright owner(s) are credited and that the original publication in this journal is cited, in accordance with accepted academic practice. No use, distribution or reproduction is permitted which does not comply with these terms.
*Correspondence: Lingling Yan, eWFubGluZ2xpbmdAeGpieXh5LmNvbQ==