- 1LC Geoscience Ltd., Truro, United Kingdom
- 2Camborne School of Mines, University of Exeter, Exeter, United Kingdom
Porphyry-type Cu ± Au ± Mo deposits form in the upper (ca. 2–5 km deep) parts of large, long-lived magmatic-hydrothermal systems in which mineralising fluids are thought to be derived from mid-to shallow-crustal magma chambers. Increasingly, however, magmatic systems are viewed as consisting of mush with minor and transient lenses of magma, with mush being a variably packed framework of crystals with interstitial melt and magmatic volatile phase (MVP). In this context, questions remain as to the source (mainly depth) and mechanisms of transport and focussing of the vast volumes of fluids required for shallow level porphyry-type mineralisation. Even more problematic is a paucity of first-order textural evidence for the presence of mush in magmatic-hydrothermal systems, including those which host porphyry-type deposits. To address this, we have studied the aplitic porphyry cupola of the Saginaw Hill magmatic system, Tuscon, Arizona, United States, where magmatic-hydrothermal features are exceptionally well exposed, including a massive silica cap, quartz unidirectional solidification textures (USTs), stockworks of multiple generations of variably mineralised quartz veins and mineralised miarolitic cavities. From field-to micro-scale textural and geochemical studies, particularly observations of vermiform quartz between earlier generations of magmatic quartz and feldspar, we evidence the development of fluid pathways through mush at the magmatic-hydrothermal transition. These are shown to connect and provide fluids and ore constituents to the mineralised miarolitic cavities and early quartz vein stockworks. We suggest that this process should be considered in all new genetic, exploration and numerical models for porphyry and similar types of magmatic-hydrothermal ore-deposits.
1 Introduction
Porphyry deposit-forming fluids are derived from large, long-lived magmatic-hydrothermal systems in subduction- and collision-related tectonic setting (e.g., Richards, 2005; Seedorff et al., 2005; Sillitoe, 2010). Less well understood are the mechanisms by which sufficient volumes of mineralising fluids are extracted and focused into narrow, shallow (ca. 2–5 km) zones of porphyry-style mineralisation.
Traditional models for porphyry deposit formation invoke direct release of mineralising fluids from magmas in stocks, cupolas, and/or dykes in the upper parts of magma chambers (e.g., Dilles, 1987; Cloos, 2001; Richards, 2005; Sillitoe, 2010). However, it is difficult to envisage how the vast volumes of fluids required to form porphyry deposits and their associated alteration could be supplied from such volumetrically small magmatic bodies (Seedorff et al., 2005; Sillitoe, 2010). It has been suggested that fluids could be derived from a larger underlying magma chamber (e.g., Dilles, 1987; Large et al., 2021), but how they migrate and are focussed into the zone of porphyry mineralisation is often overlooked or vague. In addition to this, the classic concept of large melt-dominated magma chambers in the upper crust is being increasingly questioned. From thermal and physical modelling they are unlikely to form or to endure for long enough to generate their varied compositions and textures (Cashman et al., 2017). There is also little evidence from geophysical imaging for large volumes of melt-dominated magma in the upper crust, even below currently active volcanoes (Cashman et al., 2017; Sparks and Cashman, 2017; Jackson et al., 2018).
Increasingly, melt reservoirs are viewed as transcrustal magmatic systems which consist of occasional and transient lenses of magma within what is termed mush, a semi-rigid 3D framework of crystals with interspersed melt and magmatic volatile phase (MVP) (e.g., Cashman et al., 2017). The transition from magma to mush is thought to occur when crystal concentrations reach between 50% and 65% (Cashman et al., 2017; Sparks and Cashman, 2017). The ability of fluids to flow through a semi-crystalline mush is rapidly gaining acceptance (e.g., Cashman et al., 2017; Holness, 2018; Carter et al., 2021; Blundy, 2022). Evidence of this occurring in lower crustal hot zones is from the presence of networks of interstitial sulphides in amphibole-olivine-pyroxene cumulates (Holwell et al., 2022) and experimental studies demonstrating percolative reactive melt flow (Blundy, 2022). Textural evidence for these processes occurring in the shallow crust is, however, still relatively rare, probably due to a lack of vertically extensive exposures (Seedorff et al., 2008) and perhaps because of a reluctance to accept the relatively radical concept of transcrustal mush systems. It has only been reported from the root-zone of one porphyry system (Ann Mason in the Yerington District, Nevada), where Carter et al. (2021) showed interconnected networks of multiple quartz generations joined to mineralised miarolitic cavities within the groundmass of aplitic porphyry dykes. However, without more textural and complementary experimental evidence, the concept of mush in the shallow crust will continue to be questioned, including its importance in the generation of porphyry-type deposits. To further address this, we study the granitic cupola at Saginaw Hill, Tuscon, Arizona, United States (Frank, 1970), where magmatic-hydrothermal features are exceptionally exposed including a massive silica cap, quartz unidirectional solidification textures (USTs), stockworks of multiple generations of variably mineralised quartz veins and mineralised miarolitic cavities. We integrate field to micro-scale textural and geochemical observations to evidence magmatic-hydrothermal fluid flow within mush and its link to mineralisation.
2 Geological setting
The Saginaw Hill system, located 15 km southwest of Tuscon, Arizona, is a composite pluton mainly comprised of quartz latite porphyry and quartz monzonite porphyry (Figure 1). It has a total surface outcrop of approximately ∼0.3 km2, and has intrusive (chilled) and faulted contacts with limestone and greywacke units of the Cretaceous Amole Group (Frank, 1970; Bain et al., 2022). Recent zircon U-Pb dating of porphyry rocks and dykes from Saginaw Hill yielded an age of 60.0 ± 0.6 Ma (Greig, 2021), similar to that of the Sierrita-Esperanza (∼55 Ma), Mission Pima (∼58 Ma), and Silver Bell (∼68 Ma) porphyry systems (Singer et al., 2008), which were situated close to Saginaw Hill prior to Tertiary tilting and extension (Stavast et al., 2008).
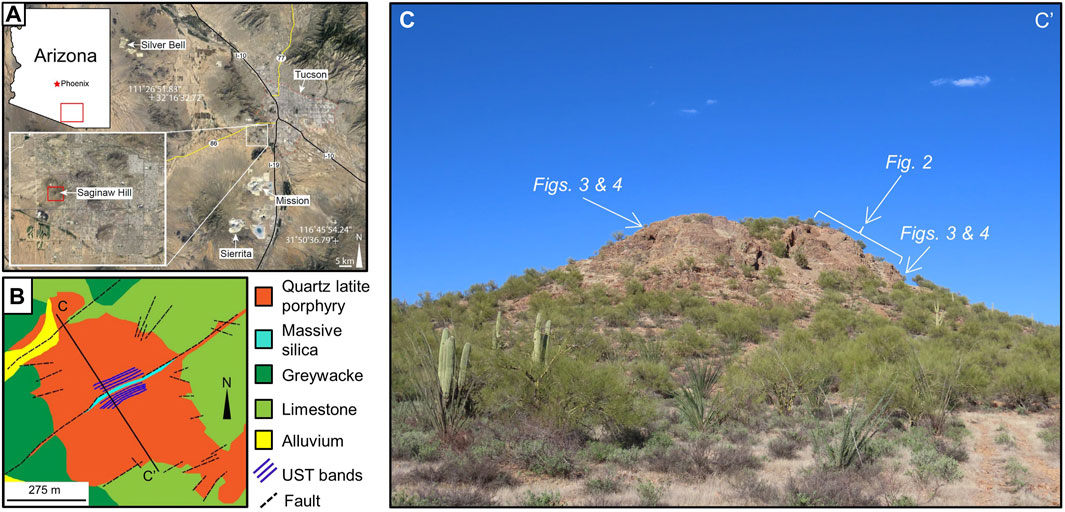
Figure 1. (A, B), Location and simplified geological map of Saginaw Hill (from Bain et al., 2022, after Frank, 1970); (C), Field photograph looking NE at Saginaw Hill, with locations of subsequent figures annotated. UST, unidirectional solidification textures.
Exposed on Saginaw Hill is a sericitically altered aplitic porphyry cupola zone of the system which hosts significant magmatic-hydrothermal features. This includes a several meter thick zone of massive homogeneous quartz which runs along the sub-vertical northeast-striking Papago Queen fault (Frank, 1970). Bain et al. (2022) describe this as a massive quartz vein, however it cannot be traced along strike away from Saginaw Hill and historic drill holes did not intercept it at depth (Frank, 1970), so it is more likely a massive silica body or cap (see Kirwin, 2005; Carter and Williamson, 2022) that has been tilted and juxtaposed against the Papago Queen fault. The massive silica cap transitions southward into a ∼10 m zone of spectacular sub-vertical/sub-parallel rhythmically layered quartz-aplite unidirectional solidification textures (USTs), with quartz comprising from 75% to 95% of the rock volume (Frank, 1970; Bain et al., 2022; Figure 2). The UST zone grades further out for ∼30 m into locally intense stockworks of anastomosing and sheeted Cu-mineralised quartz veins as well as Cu-mineralised miarolitic cavities within the quartz latite porphyry (Figure 3), with quartz veins comprising up to ∼45% to the rock volume (Frank, 1970).
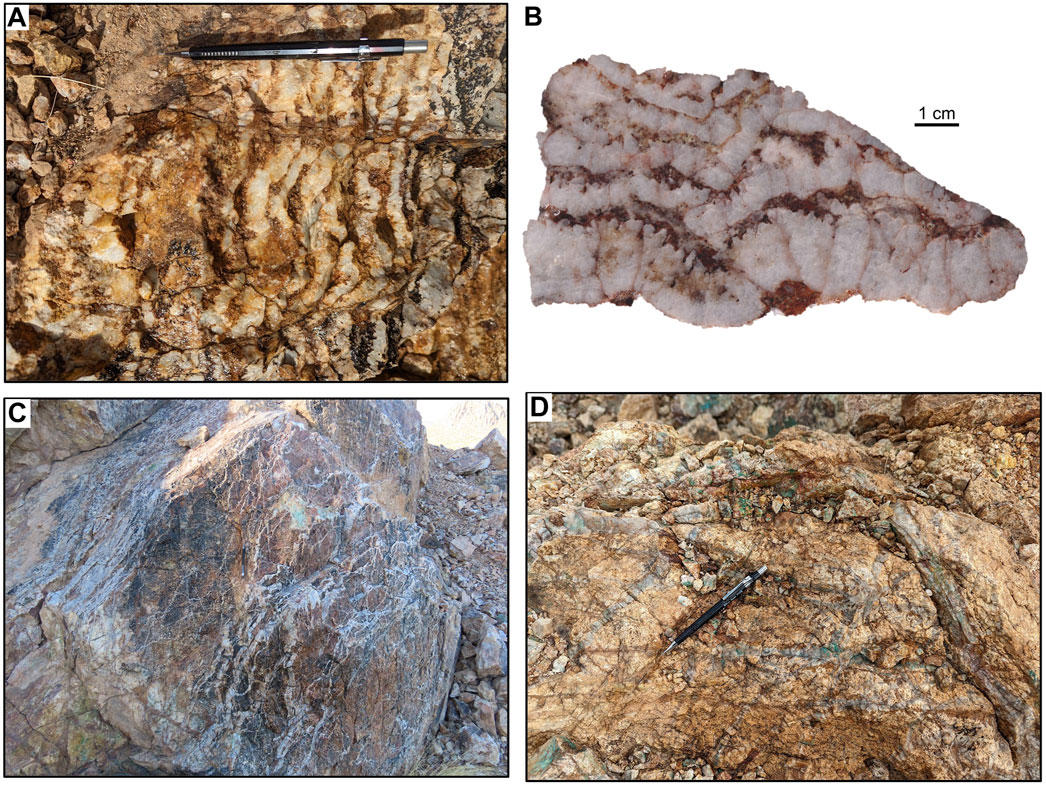
Figure 2. (A, B), Field photograph and polished slab of the quartz-aplite unidirectional solidification texture (UST) layers; (C), Field photograph showing the UST layers (top left) transitioning into anastomosing quartz veins (bottom right). Pencil in middle of photograph for scale; (D), Field photograph, a few metres downhill from (C), of quartz stockwork veining in the Saginaw Hill quartz latite porphyry. Some veins are seen hosting secondary Cu mineralisation, which probably is the result of in-situ oxidation of primary sulphides.
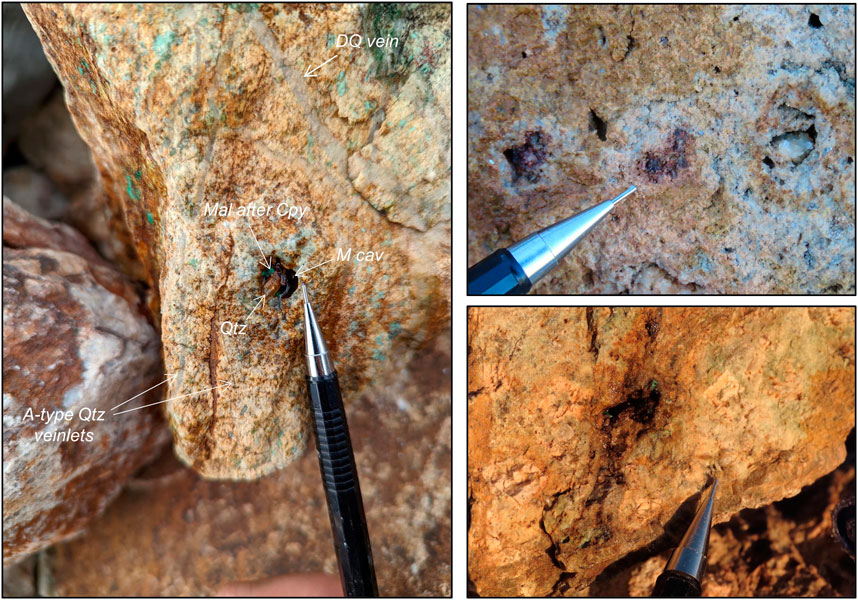
Figure 3. Field photographs of mineralised miarolitic cavities (undoubted textural evidence for fluid exsolution; Candela, 1997) in the Saginaw Hill quartz latite porphyry. All examples are a few metres outward from the UST zone at the crest of Saginaw Hill. The miarolitic cavities are seen closely associated with early DQ and A-type quartz veins (nomenclature after Gustafson and Hunt, 1975; Cernuschi et al., 2023). M Cav, miarolitic cavity; Qtz, quartz; Mal, malachite; Cpy, chalcopyrite.
3 Methods
Field observations and samples of mineralised miarolitic cavities were taken from Saginaw Hill, a few metres outward from the UST layers, between the mineralised quartz veins. A subset of samples were prepared as 30 μm-thick polished thin sections for optical microscopy, QEMSCAN® automated mineralogy, and SEM-EDS-CL imaging and EPMA analysis at Camborne School of Mines, University of Exeter, United Kingdom. The SEM-CL images presented have been false coloured on a linear look-up table to allow for better visualisation of the quartz generations. EPMA was used to measure Ti concentrations in quartz. The lower limit of detection for Ti was 24 ppm, calculated as 3σ above the average background counts, and analytical uncertainty was ±14 ppm, estimated from the repeat analysis of a quartz reference material provided by A. Audétat (Audétat et al., 2014). Quartz crystallisation temperatures were calculated using the TitaniQ geothermometer of Wark and Watson (2006), using an aTiO2 of one given the presence of rutile in the assemblage. Full methods are described in Supplementary Material S1. QEMSCAN®, SEM-CL and EPMA data are presented in Supplementary Material S2–S4.
4 Field-to micro-scale observations of quartz veinlets and related mineralisation
Miarolitic cavities are undoubted textural evidence of magmatic-hydrothermal fluid exsolution (Candela, 1997). Numerous quartz-lined miarolitic cavities can be found in the quartz latite porphyry on the slopes of Saginaw Hill, outward from the UST bands and are closely associated with stockworks of multiple quartz vein generations, including likely deep quartz (DQ) veins (continuous, sugary to massive; see Cernuschi et al., 2023) as well as sinuous, sugary, A-type quartz veinlets (nomenclature of Gustafson and Hunt, 1975) (Figure 3). The cavities range from <1 to several cm across, and some are mineralised with secondary malachite, pseudomalachite and chrysocolla, which likely formed in situ from the oxidation of primary chalcopyrite. They are locally abundant, with several dozen per m2 of outcrop.
From QEMSCAN® mineralogical maps and SEM-EDS-CL imaging, the rhyolitic groundmass hosting the cavities is comprised of K-feldspar and polycrystalline, branching, highly interconnected multiple generations of vermiform quartz (moderate to dark CL response), in addition to discrete magmatic quartz grains (brighter CL response) (Figure 4). Phenocrysts of magmatic quartz are also present along with relict phenocrysts of plagioclase and/or K-feldspar that appear to have been entirely replaced by secondary sericite. This alteration appears particularly prevalent around cavities and in certain channels of the aplitic groundmass, and does not appear to be fracture controlled. This may be an expression of coarse muscovite-type alteration (see Runyon et al., 2019), which is being increasingly recognised as a late, relatively low-temperature overprint in the exposed root zones of porphyry systems in Arizona and Nevada.
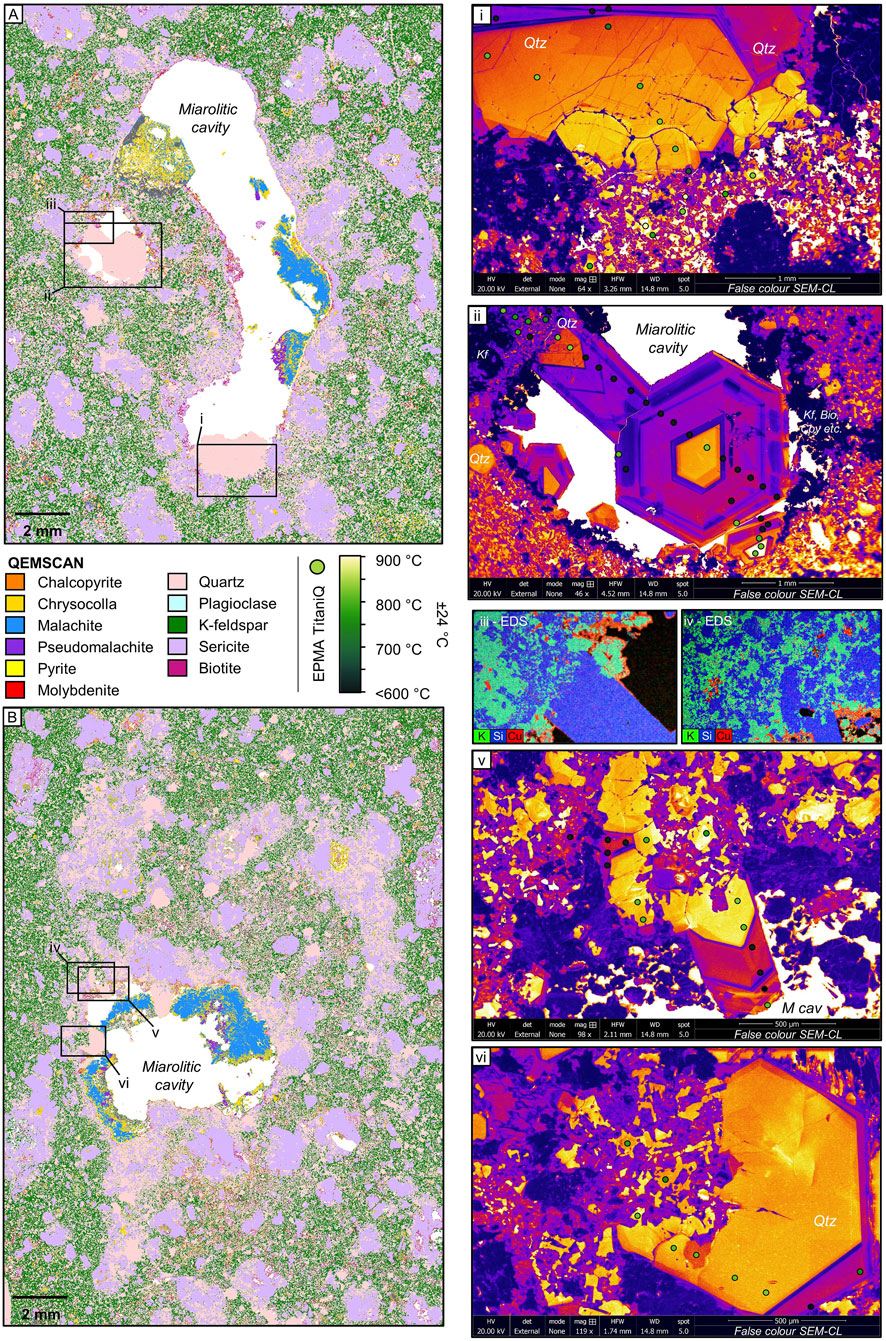
Figure 4. (A, B), QEMSCAN® mineralogical maps of thin sections of mineralised miarolitic cavities; i, ii, v and vi, false colour SEM-CL images of quartz at the margins of the miarolitic cavities and in the interconnected aplitic groundmass of the Saginaw Hill quartz latite porphyry. Note that the bright CL quartz generations (bright yellow-white) in the aplitic groundmass have very jagged edges, i.e., likely resorbed. Overlying are spots of TitaniQ thermometry (method of Wark and Watson, 2006). Inset iii and iv are SEM-EDS elemental maps of ii and iii. Qtz, quartz; Kf, K-feldspar; Bio, biotite; Cpy, chalcopyrite; M cav, miarolitic cavity. The white inside the miarolitic cavities is resin (filling open space) from thin section preparation.
From SEM-EDS-CL images (Figure 4), the cavities contain well defined, zoned, multiple generations of inwardly grown bright, moderate and dark CL quartz. From TitaniQ temperature estimates, the bright CL quartz crystallised at ∼700°C–840°C (∼80–200 ppm Ti), moderate CL quartz <600°C–700°C (∼24–80 ppm Ti) and the dark CL quartz <∼600°C (<24 ppm Ti). Our temperature estimate for the dark CL quartz is an approximate maximum as constrained from the EPMA lower detection limit for Ti, hence the dark CL quartz could have crystallised at any temperature below 600°C. The relationship between quartz CL response and TitaniQ temperature aligns with previous studies (e.g., Müller et al., 2010; Carter and Williamson, 2022; Müller et al., 2023), including that of Carter et al. (2021) which used Total-IBA to ground-truth CL responses against Ti concentrations in quartz in mineralised miarolitic cavities.
The vermiform quartz in the aplitic groundmass has the same CL response as the quartz in the miarolitic cavities. Further, the moderate to dark CL quartz appears to form continuous, interconnected networks between the earlier bright CL quartz. The bright CL quartz in the aplitic groundmass has notably irregular, jagged, edges, indicating that it was either brecciated or, where in contact with the dark CL quartz, partially resorbed (Figures 4A–D). The interconnected quartz networks can be traced continuously to the edges of the prepared thin sections. Their high degree of connectivity in 2D must mean even higher orders of connectivity in 3D. Cu-bearing oxide and carbonate phases (likely secondary after primary chalcopyrite) can be found as inclusions in all of the quartz generations, but within the miarolitic cavities, mineralisation appears to post-date the earliest, brightest CL quartz generation.
5 Textural development and mineralisation in the Saginaw Hill cupola
At Saginaw Hill, we interpret the massive silica body and USTs to have formed as a result of undercooling which occurred during emplacement of magma into the cupola of the pluton and rapid pressure fluctuations due to rhythmical “first-type boiling.” Their close association with abundant continuous, sugary to massive DQ quartz veins (nomenclature after Cernuschi et al., 2023) and A-type (sugary, sinuous and intermittent) quartz veinlets (nomenclature after Gustafson and Hunt, 1975) suggests that they were produced close to the magmatic-hydrothermal transition. This model follows those previously suggested for similar textures in porphyry deposit-forming magmatic-hydrothermal systems elsewhere (e.g., Kirwin, 2005; Carter and Williamson, 2022; Müller et al., 2023).
The aplitic groundmass of the Saginaw Hill cupola shows direct evidence for the exsolution of mineralising fluids and the magmatic-hydrothermal transition, mainly the presence of mineralised miarolitic cavities closely associated with DQ and A-type quartz veins (Figures 3, 4). From our micro-textural and geochemical observations, the aplitic groundmass contains multiple generations of paragenetically late interconnected vermiform quartz which directly link to mineralised miarolitic cavities (Figure 4). We interpret these as recording the pathways of mineralising fluids which flowed through spaces between earlier formed quartz, feldspar and accessory phases in a mush; i.e., palaeo-permeability which allowed ore-forming fluids to ascend from larger volumes of underlying magmas. Such a mechanism has been previously suggested for the formation of vermiform quartz and mineralised miarolitic cavities in aplitic dykes of the Yerington district, Nevada (Carter et al., 2021). Both potassic and sericitic alteration in the Saginaw Hill cupola also appear to have occurred due to such fluid flow since there are no obvious fracture pathways in the aplitic groundmass. From these observations, the mineralisation and potassic and sericitic alteration associated with the vermiform quartz can be interpreted as intra-mush auto-metasomatic.
Interconnected miarolitic cavities have been recognised in granitic porphyry intrusions hosting the Baja de la Alumbrera porphyry Cu-Au deposit, Argentina (Harris et al., 2004), and other granitic systems (Candela and Blevin, 1995), however not in the context of a magmatic-hydrothermal mush system. That mush microstructures were not recognised in these studies is probably because the necessary micro-scale imaging and analysis was not applied, and due to a paucity of previous direct physical evidence and models for the occurrence of mush in magmatic systems (see Introduction). Our new observations from Saginaw Hill suggest that fluid flow through mush, and associated mineralisation, may occur in the aplitic cupolas of plutons in porphyry-forming magmatic-hydrothermal systems. This builds on the suggestion of such processes occurring, on a much smaller scale, in aplitic dykes which feed porphyry mineralisation in the Yerington district, as evidenced by Carter et al. (2021).
The key implication of our observations of the aplitic mush microtexture is that fluids can be decoupled from silicate melts and crystals and travel upwards until they precipitate ore and gangue minerals, almost certainly by the process of percolative reactive flow (Blundy, 2022). This would allow a much larger volume of fluids to be involved in mineralisation, sourced from much more extensive portions of the underlying (sub-cupola) mush system, and over much longer time periods than previously assumed.
Prolonged fluid flow through an episodically open mush system aligns with recent geochronological studies of porphyry deposit formation which suggest that magmatic-hydrothermal mineralisation was much longer lived and generally relatively late compared with the shallow magmatic parts of the system, occurring over periods in excess of 1.5 Myrs after emplacement of host magmatic rocks (e.g., Romero Kojima et al., 2010; Stein, 2014; Spencer et al., 2015; Chang et al., 2017; Chiaradia and Caricchi, 2017; Li et al., 2017; Carter et al., 2022). This longevity can be explained by ongoing mush processes focussing fluids from deeper, longer-lived portions of a multi-intrusive and probably stepwise evolving mush system.
The suggested mechanisms and timescales by which mineralising fluids migrate and are focussed into the relatively narrow and shallow zones of porphyry-style mineralisation should be incorporated into future porphyry formation and exploration models. They also highlight the need to re-address the temporal-thermal frameworks that form the basis of numerical simulations of porphyry and similar magmatic-hydrothermal systems.
Data availability statement
The original contributions presented in the study are included in the article/Supplementary Material, further inquiries can be directed to the corresponding authors.
Author contributions
LC: Conceptualization, Formal Analysis, Investigation, Methodology, Writing–original draft, Writing–review and editing. BW: Conceptualization, Writing–review and editing.
Funding
The author(s) declare financial support was received for the research, authorship, and/or publication of this article. LC was supported by LC Geoscience Ltd. BW received funding from NERC Highlight Topic award “From arc magmas to ores (FAMOS): A mineral systems approach” [NE/P017452/1].
Acknowledgments
This research was allied to the NERC Highlight Topic award “From arc magmas to ores (FAMOS): A mineral systems approach” [NE/P017452/1], and members of the consortium are thanked for the fruitful discussions. M. Pfau, D. Laux, M. Poodts and I. Simon are thanked for field support. G. K. Rollinson, J. C. Andersen and S. Broom-Fendley (Camborne School Mines, University of Exeter) are thanked for QEMSCAN® analysis and EPMA support respectively. A. Audétat is thanked for the piece of quartz reference material for EPMA analysis. F. Cernuschi and Axel Müller are thanked for their constructive comments.
Conflict of interest
Author LC is a director of LC Geoscience Ltd.
The remaining author declares that the research was conducted in the absence of any commercial or financial relationships that could be construed as a potential conflict of interest.
Publisher’s note
All claims expressed in this article are solely those of the authors and do not necessarily represent those of their affiliated organizations, or those of the publisher, the editors and the reviewers. Any product that may be evaluated in this article, or claim that may be made by its manufacturer, is not guaranteed or endorsed by the publisher.
Supplementary material
The Supplementary Material for this article can be found online at: https://www.frontiersin.org/articles/10.3389/feart.2024.1426111/full#supplementary-material
References
Audétat, A., Garbe-Schönberg, D., Kronz, A., Pettke, T., Rusk, B., Donovan, J. J., et al. (2014). Characterisation of a natural quartz crystal as a reference material for microanalytical determination of Ti, Al, Li, Fe, Mn, Ga and Ge. Geostand. Geoanalytical Res. 39 (2), 171–184. doi:10.1111/j.1751-908X.2014.00309.x
Bain, W. M., Lecumberri-Sanchez, P., Marsh, E. E., and Steele-MacInnis, M. (2022). Fluids and melts at the magmatic-hydrothermal transition, recorded by unidirectional solidification textures at Saginaw Hill, Arizona, USA. Econ. Geol. 117, 1543–1571. doi:10.5382/econgeo.4952
Blundy, J. (2022). Chemical differentiation by mineralogical buffering in crustal hot zones. J. Petrology 63, 1–36. doi:10.1093/petrology/egac054
Candela, P. A. (1997). A review of shallow, ore-related granites: textures, volatiles, and ore metals. J. Petrology 38 (12), 1619–1633. doi:10.1093/petroj/38.12.1619
Candela, P. A., and Blevin, P. L. (1995). Do some miarolitic granites preserve evidence of magmatic volatile phase permeability? Econ. Geol. 90 (8), 2310–2316. doi:10.2113/gsecongeo.90.8.2310
Carter, L. C., Tapster, S. R., Williamson, B. J., Buret, Y., Selby, D., Rollinson, G. K., et al. (2022). A rapid change in magma plumbing taps porphyry copper deposit-forming magmas. Sci. Rep. 12, 17272. doi:10.1038/s41598-022-20158-y
Carter, L. C., and Williamson, B. J. (2022). Textural indicators of mineralisation potential in porphyry magmatic systems—a framework from the archetypal Yerington District, Nevada. Ore Geol. Rev. 143, 104783. doi:10.1016/j.oregeorev.2022.104783
Carter, L. C., Williamson, B. J., Tapster, S. R., Costa, C., Grime, G. W., and Rollinson, G. K. (2021). Crystal mush dykes as conduits for mineralising fluids in the Yerington porphyry copper district, Nevada. Nev. Commun. Earth Environ. 2, 59. doi:10.1038/s43247-021-00128-4
Cashman, K. V., Stephen, R. J., and Blundy, J. D. (2017). Vertically extensive and unstable magmatic systems: a unified view of igneous processes. Science 355, 6331. doi:10.1126/science.aag3055
Cernuschi, F., Dilles, J. H., Osorio, J., Proffett, J. M., and Kouzmanov, K. (2023). A reevaluation of the timing and temperature of copper and molybdenum in porphyry deposits. Econ. Geol. 118 (5), 931–965. doi:10.5382/econgeo.5032
Chang, J., Li, J.-W., Selby, D., Liu, J.-C., and Deng, X.-D. (2017). Geological and chronological constraints on the long-lived Eocene Yulong porphyry Cu-Mo deposit, Eastern Tibet: implications for the lifespan of giant porphyry Cu deposits. Econ. Geol. 112 (7), 1719–1746. doi:10.5382/econgeo.2017.4527
Chiaradia, M., and Caricchi, L. (2017). Stochastic modelling of deep magmatic controls on porphyry copper deposit endowment. Sci. Rep. 7, 44523. doi:10.1038/srep44523
Cloos, M. (2001). Bubbling magma chambers, cupolas, and porphyry copper deposits. Int. Geol. Rev. 43, 285–311. doi:10.1080/00206810109465015
Dilles, J. H. (1987). Petrology of the Yerington Batholith, Nevada: evidence for evolution of porphyry copper ore fluids. Econ. Geol. 82, 1750–1789. doi:10.2113/gsecongeo.82.7.1750
Frank, T. R. (1970). Geology and mineralization in the Saginaw Hill area, Pima County, Arizona [Ph.D. Thesis]. Tuscon: University of Arizona, 1–213. Available at: https://repository.arizona.edu/handle/10150/554066.
Greig, R. E. (2021). Superposed magmatic and hydrothermal systems, and the evolution of the Laramide arc and porphyry copper province, southwestern North America [Ph.D. thesis]. Tucson: University of Arizona. Available at: http://hdl.handle.net/10150/660104.
Gustafson, L. B., and Hunt, J. P. (1975). The porphyry copper deposit at El Salvador, Chile. Econ. Geol. 70, 857–912. doi:10.2113/gsecongeo.70.5.857
Harris, A. C., Kremenetsky, V. S., White, N. C., and Steele, D. A. (2004). Volatile Phase Separation in Silicic Magmas at Bajo de la Alumbrera Porphyry Cu-Au Deposit, NW Argentina. Resour. Geol. 54, 341–356. doi:10.1111/j.1751-3928.2004.tb00210.x
Holness, M. B. (2018). Melt segregation from silicic crystal mushes: a critical appraisal of possible mechanisms and their microstructural record. Contributions Mineralogy Petrology 173, 48. doi:10.1007/s00410-018-1465-2
Holwell, D. A., Fiorentini, M. L., Knott, T. R., McDonald, I., Blanks, D. E., Campbell McCuaig, T., et al. (2022). Mobilisation of deep crustal sulfide melts as a first order control on upper lithospheric metallogeny. Nat. Commun. 13, 573. doi:10.1038/s41467-022-28275-y
Jackson, M. D., Blundy, J., and Sparks, R. S. J. (2018). Chemical differentiation, cold storage and remobilization of magma in the Earth’s crust. Nature 564, 405–409. doi:10.1038/s41586-018-0746-2
Kirwin, D. J. (2005). “Unidirectional solidification textures associated with intrusion-related Mongolian mineral deposits,” in Geodynamics and metallogeny of Mongolia with special emphasis on copper and gold deposits: society of economic geologists-international association for the genesis of ore deposits field trip, 2005: IAGOD guidebook series 11: London. Editors R. Seltmann, O. Gerel, and D. J. Kirwin (Centre for Russian and Central EurAsian Mineral Studies, Natural History Museum), 63–84.
Large, S. J. E., Buret, Y., Wotzlaw, J., Karakas, O., Guillong, M., von Quadt, A., et al. (2021). Copper-mineralised porphyries sample the evolution of a large-volume silicic magma reservoir from rapid assembly to solidification. Earth Planet. Sci. Lett. 563, 116877. doi:10.1016/j.epsl.2021.116877
Li, Y., Selby, D., Condon, D., and Tapster, S. (2017). Cyclic magmatic-hydrothermal evolution in porphyry systems: high-precision U-Pb and Re-Os geochronology constraints on the Tibetan qulong porphyry Cu-Mo deposit*. Econ. Geol. 112 (6), 1419–1440. doi:10.5382/econgeo.2017.4515
Müller, A., Herrington, R., Armstrong, R., Seltmann, R., Kirwin, D. J., Stenina, N. G., et al. (2010). Trace elements and cathodoluminescence of quartz in stockwork veins of Mongolian porphyry-style deposits. Miner. Deposita 45 (7), 707–727. doi:10.1007/s00126-010-0302-y
Müller, A., Kirwin, D., and Seltmann, R. (2023). Textural characterization of unidirectional solidification textures related to Cu–Au deposits and their implication for metallogenesis and exploration. Miner. Deposita 58, 1211–1235. doi:10.1007/s00126-023-01175-x
Richards, J. P. (2005). “Cumulative factors in the generation of giant calc-alkaline porphyry Cu deposits,”. Super porphyry copper and gold deposits: a global perspective. Editor T. M. Porter (Adelaide: PGC Publishing), 1, 7–25.
Romero Kojima, B. S., Wong, C., Barra, F., Véliz, W., and Ruiz, J. (2010). Molybdenite mineralization and Re-Os geochronology of the Escondida and Escondida Norte porphyry deposits, northern Chile. Resour. Geol. 61 (1), 91–100. doi:10.1111/j.1751-3928.2010.00150.x
Runyon, S. E., Seedorff, E., Barton, M. D., Steele-MacInnis, M., Lecumberri-Sanchez, P., and Mazdab, F. K. (2019). Coarse muscovite veins and alteration in porphyry systems. Ore Geol. Rev. 113, 103045. doi:10.1016/j.oregeorev.2019.103045
Seedorff, E., Barton, M. D., Stavast, W. J. A., and Maher, D. J. (2008). Root zones of porphyry systems: extending the porphyry model to depth. Econ. Geol. 103, 939–956. doi:10.2113/gsecongeo.103.5.939
Seedorff, E., Dilles, J. H., Proffett, J. M., Einaudi, M. T., Zurcher, L., Stavast, W. J. C., et al. (2005). Porphyry deposits: characteristics and origin of hypogene features. Econ. Geol. 100, 251–298. doi:10.5382/AV100.10
Sillitoe, R. H. (2010). Porphyry copper systems. Econ. Geol. 105, 3–41. doi:10.2113/gsecongeo.105.1.3
Singer, D. A., Berger, V. I., and Moring, B. C. (2008). Porphyry copper deposits of the world: database and grade and tonnage models. Reston, United States: U.S. Geological Survey. Available at: http://pubs.usgs.gov/of/2008/1155 (Open-File Report 2008-1155).
Sparks, R. S. J., and Cashman, K. V. (2017). Dynamic magma systems: implications for forecasting volcanic activity. Elements 13, 35–40. doi:10.2113/gselements.13.1.35
Spencer, E. T., Wilkinson, J. J., Creaser, R. A., and Seguel, J. (2015). The distribution and timing of molybdenite mineralization at the El Teniente Cu-Mo porphyry deposit, Chile. Econ. Geol. 110 (2), 387–421. doi:10.2113/econgeo.110.2.387
Stavast, W. J. A., Butler, R. F., Seedorff, E., Barton, M. D., and Ferguson, C. A. (2008). Tertiary tilting and dismemberment of the Laramide arc and related hydrothermal systems, Sierrita Mountains, Arizona. Econ. Geol. 103, 629–636. doi:10.2113/gsecongeo.103.3.629
Stein, H. J. (2014). “Dating and tracing the history of ore formation,” in Treatise on geochemistry. Editors H. D. Holland, and K. K. Turekian Second Edition (Oxford: Elsevier), 13, 87–118.
Keywords: porphyry, copper, mush, miarolitic cavities, magmatic-hydrothermal, fluid exsolution
Citation: Carter LC and Williamson BJ (2024) Migration and focusing of porphyry deposit-forming fluids through aplitic mush of the Saginaw Hill cupola, Arizona, United States. Front. Earth Sci. 12:1426111. doi: 10.3389/feart.2024.1426111
Received: 30 April 2024; Accepted: 05 June 2024;
Published: 01 July 2024.
Edited by:
Massimo Chiaradia, University of Geneva, SwitzerlandReviewed by:
Axel Müller, University of Oslo, NorwayFederico Cernuschi, Independent Researcher, Punta del Este, Uruguay
Copyright © 2024 Carter and Williamson. This is an open-access article distributed under the terms of the Creative Commons Attribution License (CC BY). The use, distribution or reproduction in other forums is permitted, provided the original author(s) and the copyright owner(s) are credited and that the original publication in this journal is cited, in accordance with accepted academic practice. No use, distribution or reproduction is permitted which does not comply with these terms.
*Correspondence: Lawrence C. Carter, bC5jLmNhcnRlckBleGV0ZXIuYWMudWs=