- 1State Key Laboratory of Coal and CBM Co-mining, Jincheng, China
- 2School of Resources and Environmental Engineering, Shandong University of Technology, Zibo, China
- 3School of Geographical Sciences, Shanxi Normal University, Taiyuan, China
- 4Shanxi Institute of Geological Survey Co., Ltd., Taiyuan, Shanxi, China
Iron is a critical redox-active element in geothermal water, and the presence of nanoparticulate Fe is essential in comprehending the intricate cycling of iron and related elements within the natural geothermal ecosystems. In this study, we investigated the mineral properties of Fe-bearing nanoparticles in a hot spring located in Shanxi Province. High-resolution transmission electron microscopy (HRTEM) is utilized for the examination of the morphology, chemical composition, and crystalline structure of Fe-bearing nanoparticles. The findings show that Fe-bearing nanoparticles can exist as single particles measuring 50–200 nm in size, as well as aggregate to form nanoparticle aggregations. The morphology of Fe-bearing nanoparticles mainly includes triangle, axiolitic, and irregular shapes. The selected area electron diffraction patterns reveal the crystal form, amorphous form, and the transition from amorphous to crystalline forms of these nanoparticles. Energy-dispersive X-ray spectroscopy (EDS) analysis indicates that these nanoparticles primarily consist of O and Fe in composition, along with various trace elements including N, Al, Si, Ca, Zn, Cr, Ni, and Mo. These results reveal that goethite and hematite can occur in hot spring. Various in size and modality, tend to cluster into each other, and multiple crystalline states indicate that these iron-bearing nanoparticles are formed through natural processes. In addition, the iron-bearing nanoparticles with biomimetic morphologies (cell-like or microorganism-like shapes) may be produced through microbial activity. The biomimetic properties also imply that these nanoparticles may be readily available for biological processes. Significantly, our findings further validate that the shape of iron oxide nanoparticles can serve as an indicator of pH and temperature of the hot spring.
Introduction
Hot springs contain a wide range and organized combinations of geochemical conditions, which vary over short distances due to ongoing interactions between the hydrothermal fluids, the oxygen-rich atmosphere, and in some cases, seawater (Ward et al., 2018). The elevated temperatures and reducing conditions in hot springs allow for the development of unique ecosystems that significantly differ from those found elsewhere on Earth’s surface today (Inskeep et al., 2005). In certain aspects, these environments and the communities they sustain can resemble those that existed on early Earth and may exist on other celestial bodies. Consequently, studying hot springs can provide insights into the functioning of the geosphere during early Earth (Ward et al., 2018).
In addition, hot springs exhibit diverse chemical compositions, with many elements being biogeochemically significant. Iron, in particular, is a crucial redox-active element in hot springs (Kaasalainen et al., 2017). Moreover, its chemistry and speciation are of great scientific and environmental interest because of its involvement in mineral dissolution and precipitation reactions, sequestration of metal(loid)s, and various biogeochemical processes associated with thermophilic ecosystems (Gunnlaugsson and Arnòrsson, 1982; Stefánsson et al., 2001; Nordstrom et al., 2005; Shock et al., 2010; Kaasalainen and Stefánsson, 2012; McCleskey et al., 2014). It has been extensively documented that iron can be found in water in various forms, including dissolved ferrous (Fe(II)) and ferric (Fe(III)) iron. These iron species can exist either unassociated or complexed with inorganic or organic ligands, as well as in colloidal and particulate forms containing one or both oxidation states (Kinniburgh et al., 1976; Stumm and Sulzberger, 1992; Rue and Bruland, 1995). However, to the best of our knowledge, there have been no studies conducted on the presence of Fe-bearing nanoparticles in hot springs, despite the fact that such nanoparticles are known to occur widely in aquatic and terrestrial environments throughout the Earth’s crust.
Natural nanoparticles are widely distributed in groundwater (Wigginton et al., 2007; Frimmel and Niessner, 2010; Bakshi et al., 2015; Dibyanshu et al., 2023). These nanoparticles are formed from both geochemical and biological processes. Numerous investigations also have shown the presence of a significant quantity of natural in hot spring, such as Tobler et al. found natural SiO2 nanoparticles in the Icelandic geothermal areas (Tobler et al., 2008), Wu et al. confirmed natural MnSiO3, Fe(1-x)S, SiO2, Al2O3, FeSO4 and Na2CO3 in Taiwan hot springs (Wu et al., 2016) and Zuo et al. found numerous natural carbonates, sulfates, and chlorides nanoparticles occurring in geothermal fluids form the central area of Shandong Province (Zuo et al., 2023). In addition, geothermal energy can make higher geothermal gradient, which can serve as one of the driving forces behind the migration of nanoscale materials (Wang et al., 2005). Thus, in the geothermal field often witness pronounced nanomaterial migration (Zhou et al., 2014).
Meanwhile, due to their large surface area and high chemical activity, the nanoparticles can provide valuable insights into their environment (Banfield and Zhang, 2001; Bakshi et al., 2015; Ju et al., 2022). Based on the special physicochemical properties of Fe, Fe-bearing nanoparticles significantly contribute to the immobilization of heavy metals through adsorption or incorporation, as well as affect the variation of pH and redox potential (Eh) through biogeochemical reactions. Moreover, they provide essential nutrients to animals and plants (Guo and Barnard, 2013). Furthermore, Fe-bearing nanoparticles are redistributed by rivers, glaciers, winds, and ocean currents into various ecosystems once formed. In these ecosystems, they can undergo continuous phase transformations, dissolution, and changes in morphology (Hochella et al., 2012). Therefore, it is crucial to conduct further research on Fe-bearing nanoparticles originating from hot springs, including investigating their occurrence, fate, and significance in biogeochemical processes within these ecosystems.
With the aim of studying the characteristics of Fe-bearing nanoparticles in hot springs, samples of geothermal surface and well water were collected and analyzed from Taiyuan and Tianzhen cities in Shanxi Province. The use of high-resolution transmission electron microscopy (HRTEM) revealed that substantial amount of natural Fe-bearing nanoparticles occurs in hot spring. Furthermore, this study elucidated the mineral characteristics of these nanoparticles and their significant role in biogeochemical cycles.
Sampling and methodology
Sampling sites
Taiyuan city is located in the Taiyuan Basin, middle of Shanxi Province, central China (Figure 1). The general trend of the study area’s topography is high in the north and low in the south, with higher elevation on the east and west sides and lower elevation in the middle. The terrain gradually descends like a staircase from the mountainous area to the basin area. Taiyuan City has a temperate continental climate with semi-arid characteristics. The winters and springs are long, while the summers are short. The four seasons are distinct, and there is a significant difference in temperatures throughout the year. The distribution of annual precipitation in the study area is highly uneven, with the majority of rainfall occurring in the summer season. The average annual rainfall is about 440 mm/a. The average annual evaporation is 1,000 mm/a. The research area belongs to the Fen River Basin, which has an area of 6,253 km2 within the research area. The aquifer is mainly composed of middle Ordovician limestone, followed by layers of marlstone with gypsum. The temperature of this hot spring is about 60°C, and the pH value is neutral to slightly alkaline.
Tianzhen city is located in the Datong Basin, northern Shanxi Province, central China (Figure 1). The annual average temperature in the region is about 6°C, with an annual average evaporation of about 1,000–2,000 mm, which is much higher than the annual average rainfall of around 400 mm. This indicates a continental semi-arid monsoon climate. The hot reservoir in the research area is mainly composed of gray sand and gravel layers and gray-green sub-clay layers of the lower Neogene Nihewan Formation. This hot reservoir is typically located at depths less than 200 m and is characterized by shallow burial and low temperatures, generally around 40°C. The pH value ranges from 7.33 to 7.89, indicating the weak alkalinity nature.
Analytical methods
Three geothermal water samples were collected from Taiyuan and Tianzhen hot springs in Shanxi Province. A total of approximately 50 nanoparticles and/or nanoparticle aggregations were analyzed. The sampling points are indicated in Figure 1. The samples were collected using 50 mL volumetric flasks. Prior to collection, the flasks were rinsed with a small amount of water and then filled with the water sample, ensuring a volume of over 30 mL. The flasks were tightly sealed and stored until analysis.
Transmission electron microscopy (TEM) was employed to analyze the nanoparticles, with a maximum acceleration voltage of 200 kV. TEM foils were prepared by extracting the nanoparticles and attaching them to Cu grids via Pt welding. These foils were then thinned down to thicknesses of 50–70 nm. High-angle annular dark-field scanning transmission electron microscopy (HAADF-STEM) imaging was carried out using an ultra-high resolution and probe-corrected FEI Titan Themis TEM. The processing of high-resolution transmission electron microscopy (HRTEM) images, including fast Fourier transform (FFT) processing, was conducted using DigitalMicrograph software (version 3.7.4, Gatan). Additionally, the elemental distribution was determined using energy-dispersive X-ray spectrometry (EDS). All the analyses, including TEM and EDS, were performed at the Sinoma Institute of Materials Research (Guangzhou) Co., Ltd. Since the TEM grid is made of copper and is coated with carbon film, the presence of carbon and copper will not be considered in this discussion.
Results
Goethite nanoparticles in hot spring
In Figures 2A, C, E, the nanoparticles appear to have a nearly pseudohexagonal morphology, measuring approximately 50 nm in size. They are primarily observed as aggregates. In Figure 3A, the particle exhibits an irregular shape, with dimensions of 100 × 200 nm. The composition of these nanoparticles in Figures 2, 3, as determined by TEM-EDS analysis, mainly consists of oxygen (O) and iron (Fe), with a small amount of Si, Ca, Zn and Mo (Table 1). Furthermore, the selected area electron diffraction patterns reveal distinctive diffraction rings, with the (010) reflection (with a d-spacing of approximately 0.996 nm) being the most prominent (Figures 2B, D, F, 3B). These findings suggest that these nanoparticles are likely composed of goethite. Due to the absence of clear diffraction spots, it can be inferred that these nanoparticles are in the process of transitioning from the amorphous to the crystalline phase.
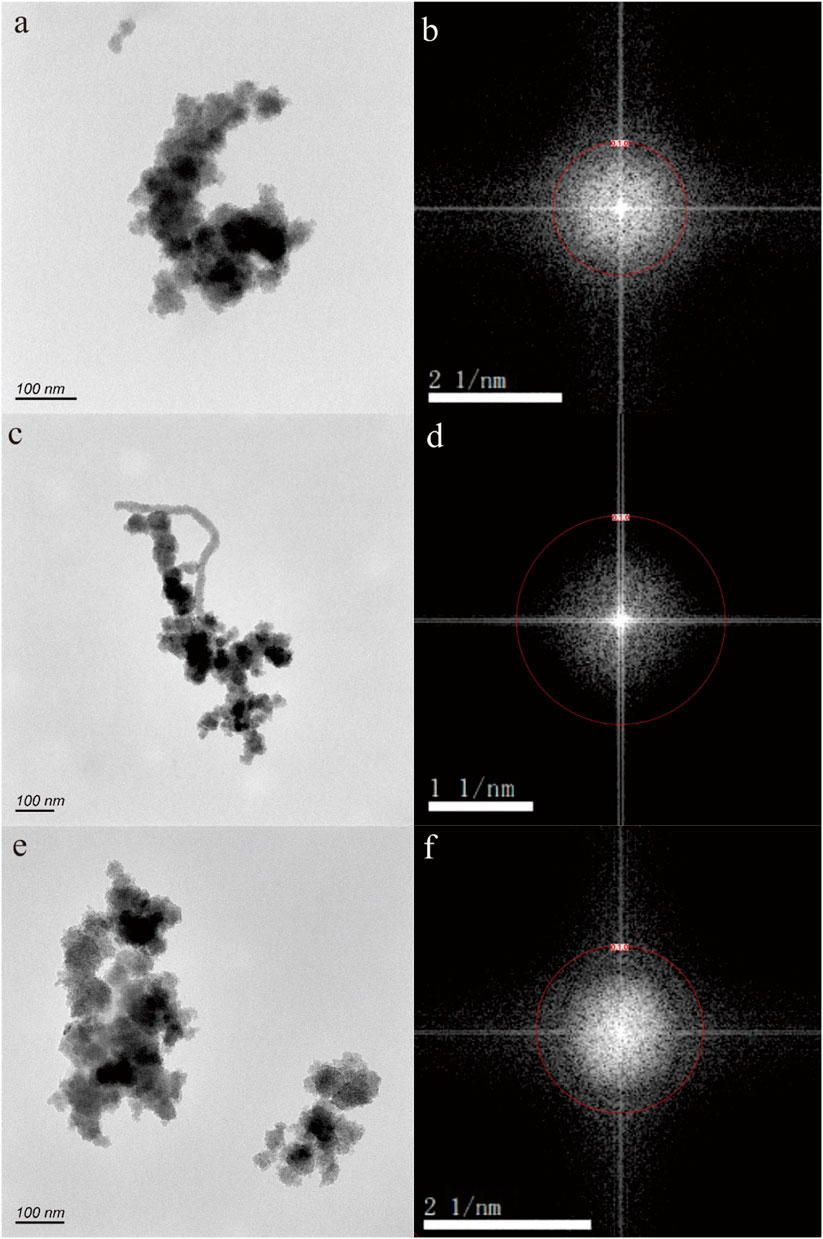
Figure 2. Goethite nanoparticle aggregations (A,C,E) in hot spring. The selected area electron diffraction patterns (B,D,F) reveal distinctive diffraction (010) rings.
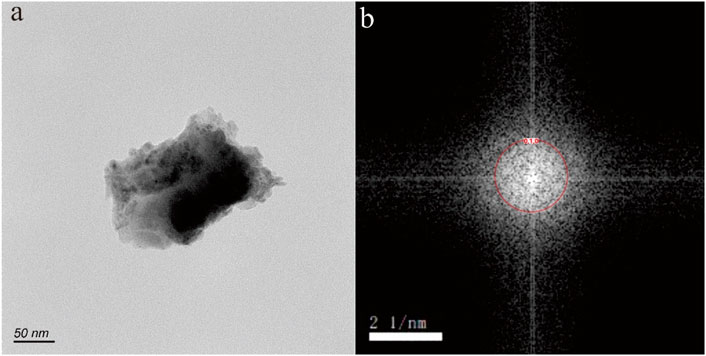
Figure 3. Goethite nanoparticle in hot spring. The HRTEM image (A) shows an irregular shape, with dimensions of 100 × 200 nm. The selected area electron diffraction pattern (B) reveals distinctive diffraction (010) rings.
Hematite nanoparticles in hot spring
Figure 4A shows nanoparticle aggregation consisting of numerous irregular hexagonal nanoparticles measuring approximately 50 nm in size. The composition of this aggregation, as determined by TEM-EDS analysis, is primarily Fe and O, with a small quantity of N, Si, Ca, and Zn. The selected area electron diffraction (SAED) patterns obtained exhibit diffraction rings corresponding to the (−120) and (003) crystallographic orientations (Figure 4B), suggesting that these nanoparticles possess a crystalline structure.
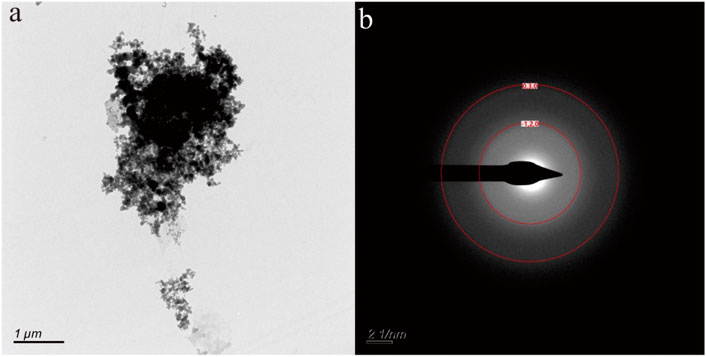
Figure 4. Hematite nanoparticle aggregation (A) in hot spring. The selected area electron diffraction patterns (B) reveal distinctive diffraction (−120) and (030) rings.
Fe-bearing nanoparticles in hot spring
Figures 5–7 depict nanoparticles with complex compositions as observed through TEM-EDS analysis, and their amorphous forms as confirmed by selected area electron diffraction patterns. Due to the intricate composition and uncertain lattice, the mineral origin of these particles cannot be determined. In Figures 5A, C, two nanoparticle aggregations consist of numerous small irregular hexagonal nanoparticles measuring approximately 50 nm in size. These biomimetic morphologies suggest biological resemblance. Results from TEM-EDS analysis indicate the presence of N, O, Si, Ca, Fe, and Zn in these nanoparticles. In Figures 6A, B, the nanoparticles exhibit distinct shapes reminiscent of microorganisms or nanobacteria, and their composition also consists of O, Si, Ca, Fe, and Zn. Figures 7B, D displays two irregular nanoparticles measuring around 200 nm in size, with a composition comprising O, Si, Al, and Fe. The selected area electron diffraction patterns confirm that these particles are in amorphous forms (Figures 5B, D, 6B, D, 7B, D).
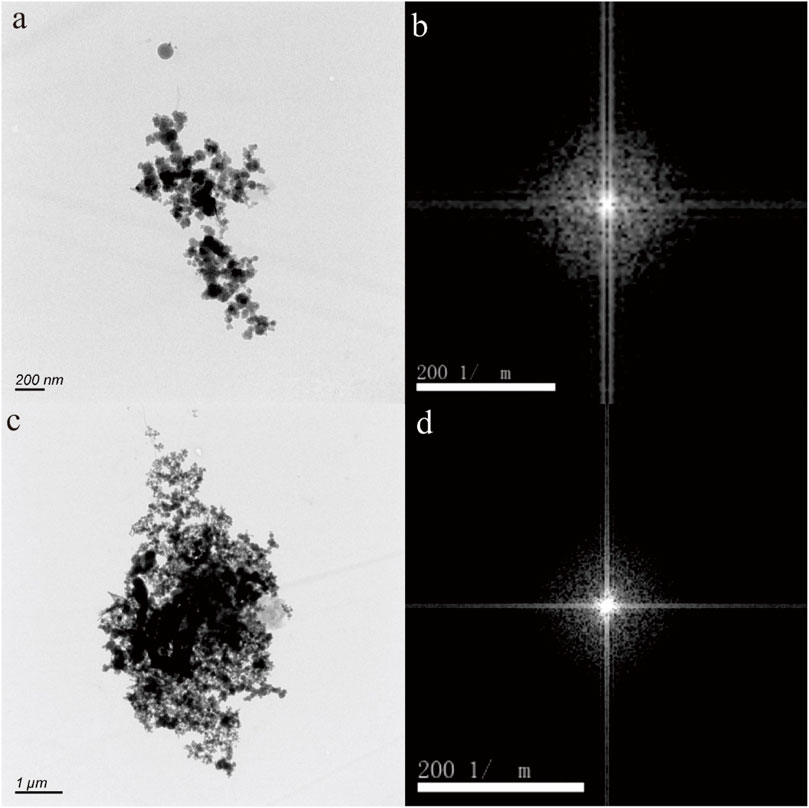
Figure 5. Fe-bearing nanoparticle aggregations (A,C) in hot spring. The selected area electron diffraction patterns (B,D) show the amorphous form.
Discussion
The mineral characteristics of Fe-bearing nanoparticles in hot springs
According to the HRTEM images (Figures 2–7), the Fe-bearing nanoparticles mainly range in size from 50 to 200 nm. The morphology of the Fe-bearing nanoparticles in the hot spring varies and includes triangular, axiolitic, and irregular shapes. Among these, the majority of Fe-bearing nanoparticles exhibit biomimetic morphologies, resembling cells or microorganisms. Furthermore, these Fe-bearing nanoparticles with biomimetic morphologies often aggregate, suggesting that they may undergo a growth process (Murad and Schwertmann, 1983).
The selected area electron diffraction patterns indicate that the Fe-bearing nanoparticles found in hot springs can exhibit crystal form, amorphous form, and a transition from amorphous to crystalline forms. This transition from amorphous to crystalline forms suggests the growth process of these nanoparticles in hot springs.
The chemical composition of Fe-bearing nanoparticles primarily consists of Fe and O, but there are also various trace elements present in these nanoparticles, including Si, S, Ca, Zn, Cr, Ni, and Mo. This finding suggests that Fe-bearing nanoparticles have the ability to transport elements over significant distances in hot springs.
Origin of Fe-bearing nanoparticles in hot spring
Nanomaterials have gained significant attention in recent years due to their potential applications in technology and medicine (Kim et al., 2010). Consequently, the production of engineered nanoparticles has experienced a significant surge, with their usage extending to various industries, consumer goods, and environmental remediation practices (Ju-Nam and Lead, 2008). For instance, engineered Fe-oxide nanoparticles hold promise for in-situ remediation of polluted soils (Braunschweig et al., 2013). As a result, the majority of nanoparticles present in the environment are synthetic or human-made (Handy et al., 2008; Hochella jr et al., 2008). These synthetic nanoparticles typically exhibit regular shapes, such as spheres or cubes, and possess a pure composition.
However, a fraction of the global pool of environmental Fe minerals is constantly present as nanoparticles. In contrast, solid bulk minerals are exposed to various processes such as shearing, straining, weathering, and dissolutive reactions, which also result in the formation of nanoparticles (Hochella et al., 2012). Therefore, Fe-bearing nanoparticles can also be a natural occurrence. Indeed, natural Fe-bearing nanoparticles have been discovered in the air, soil, icebergs, glaciers, and water in various environmental settings (Raiswell et al., 2008; 2009; Wu et al., 2016). Additionally, these natural nanoparticles typically have irregular shapes and complex elemental compositions, distinguishing them from engineered nanoparticles (Liu et al., 2020; 2021; Tan et al., 2021).
In this study, it was observed that most Fe-bearing nanoparticles and nanoparticle aggregations in hot springs have irregular shapes. The composition of these nanoparticles and aggregations is also found to be complex. Based on these findings, we propose that the Fe-bearing nanoparticles in hot springs have a natural origin. Specifically, some of the Fe-bearing nanoparticles with biomimetic morphologies, such as cell-like or microorganism-like shapes, may be formed as a result of microbial activity. Similar morphologies have been observed in other aquatic environments as well (Wu et al., 2016). On the other hand, Fe-bearing nanoparticles without biomimetic morphologies may be formed through weathering of minerals or as growth nuclei in super-saturated fluids. Additionally, since nanoparticle aggregations are commonly found in hot springs, the aggregation process (i.e., ripening) may be the main mechanism responsible for the growth of Fe-bearing nanoparticles in this ecosystem.
Potentially bioavailable properties of Fe-bearing nanoparticles
Dissolved Fe in the form of Fe-bearing nanoparticles exhibits different behavior compared to aqueous inorganic species, as their reactivity can change over time (Raiswell et al., 2010; Raiswell, 2011). Iron nanoparticles are considered to be intermediate in terms of their physical and chemical properties between soluble and particulate forms, and their small size enhances their solubility and reactivity by several orders of magnitude. This suggests that nanoparticles may play a significant role in acting as a reservoir of iron that can be transformed into a form that is available for biological processes. Furthermore, studies conducted on cultures have shown that freshly-prepared, poorly-ordered colloids containing Fe can provide the necessary Fe for cell growth, whereas more crystalline phases are not bioavailable (Wells et al., 1983; Rich and Morel, 1990). The solubility and reactivity of Fe nanophase are crucial factors in the biogeochemical cycling of Fe in various aquatic environments, including seawater systems and acid mine drainage environments (Raiswell et al., 2008). Hot springs, characterized by a diverse range of chemical compositions and surface temperatures that can range from ambient to 100°C, and pH levels varying from less than 2 to 10, also exhibit a wide range of total dissolved Fe concentrations spanning over five orders of magnitude, from less than 1 μg L−1 to over 100 mg L−1 (Gunnlaugsson and Arnòrsson, 1982; Stefánsson et al., 2001; Inskeep et al., 2005; Nordstrom et al., 2005; Shock et al., 2010; Kaasalainen and Stefánsson, 2012; McCleskey et al., 2014; Pope and Brown, 2014). Therefore, due to their high temperatures and diverse chemical compositions, hot springs are expected to provide favorable conditions for the proliferation of biological activity associated with Fe-bearing nanoparticles.
In this study, the morphological structures of Fe-bearing nanoparticles resemble those commonly found in terrestrial bacteria, and some nanoparticles contain biophile elements such as S and N. One of the most noteworthy characteristics of Fe-bearing nanoparticles in hot springs is their bioavailability, as indicated by their resemblance to bacteria (McKay et al., 1996; Shao et al., 2023). Furthermore, the size and number of these nanoparticles generally increase in culture, suggesting that they might have an impact on the circulation and availability of elements in the environment (Young et al., 2009; Peng et al., 2011).
Environmental indicator of iron oxide nanoparticles
The physical and chemical characteristics of water, including temperature, pH, ion concentration, oxygen supersaturation, organic matter content, and redox potential (Eh), can vary. These conditions fluctuate across different locations and depths, impacting microbial activities and the formation of biotic iron oxides. Additionally, iron oxide nanostructures are commonly found in various ecosystems, making them useful for monitoring environmental conditions due to their measurable physical and chemical properties (Guo and Barnard, 2013).
In this study, various iron oxide nanoparticles, such as goethite and hematite, are found in the hot spring. Goethite is commonly observed in aquatic environments with both acidic and alkaline pH levels (Guo and Barnard, 2013). In specific environments that are rich in silicate, nanogoethite has been reported as the prevailing precipitated phase. However, in most cases, it is transformed from other less stable oxyhydroxide nanoparticles (e.g., ferrihydrite and lepidocrocite) in water.
The morphology of goethite nanoparticles is predominantly acicular or needle-like, primarily because the (010) surface possesses high surface energy and undergoes more rapid coarsening (Cornell and Schwertmann, 2003). However, in this study, the goethite nanoparticles largely exist as aggregates, exhibiting a nearly pseudohexagonal morphology. This morphology is indicative of the alkaline conditions present, as well as the presence of silicates (Cornell et al., 1987), which aligns with the chemical characteristics of the hot springs studied (Ma, 2007; Zhang, 2013).
The morphology of hematite exhibits significant variation and is highly sensitive to environmental conditions, particularly when compared to goethite (Cornell and Schwertmann, 2003). In natural settings, hematite nanoparticles are predominantly formed through the dehydration of oxyhydroxides, such as goethite, or through a combination of aggregation and internal rearrangements, as observed in ferrihydrite. The typical shapes of hematite crystals, whether on the nano or macro scale, include rounded, rhombohedral, and platy forms. The coarsely crystalline morphology of hematite often exposes the (001) basal plane, which is believed to be associated with high-temperature processes exceeding 100°C (Catling and Moore, 2003). In this study, the hematite nanoparticles primarily exhibited irregular hexagonal morphologies, similar to nanoparticle aggregation, particularly under neutral pH conditions and at low temperatures (Madden et al., 2010). Additionally, the nanoparticles were predominantly coarsely crystalline, with the (−120) and (003) crystal planes observed, suggesting that they cannot be formed under high-temperature conditions. These findings align with the results obtained for goethite nanoparticles and are in accordance with the chemical characteristics of hot springs.
Above all, our results demonstrate that the morphology of iron oxide nanoparticles is closely related to the pH and temperature of the hot spring. Furthermore, these particles can be utilized as indicators to assess environmental conditions.
Conclusion
The mineral characteristics of Fe-bearing nanoparticles in hot springs from Taiyuan and Tianzhen City, Shanxi Province, central China were investigated using HRTEM. The nanoparticles exhibited various shapes, including triangles, axiolitic shapes, and irregular shapes, with some of them being aggregated. The crystalline nature of these nanoparticles varied, with some showing a crystal form, others displaying an amorphous form, and some transitioning from amorphous to crystalline forms. The composition of these nanoparticles primarily consisted of O, and Fe. Additionally, Si, S, Ca, Zn, Cr, Ni and Mo were also detected in certain nanoparticles. It was confirmed that goethite and hematite nanoparticles were present in the hot spring. The characteristics of these Fe-bearing nanoparticles suggest that they have a natural origin. Among them, the nanoparticles with biomimetic morphologies may be the result of microbial activity. The biomimetic morphology and presence of biophile elements in these Fe-bearing nanoparticles indicate their bioavailable properties. The morphologies of goethite and hematite nanoparticles reflect the alkaline and lower temperature conditions of the hot spring, which is consistent with the chemical characteristics of hot springs. These findings demonstrate that the morphology of iron oxide nanoparticles can serve as an environmental indicator.
Data availability statement
The original contributions presented in the study are included in the article/Supplementary Material, further inquiries can be directed to the corresponding author.
Author contributions
ZC: Writing–original draft. RL: Writing–original draft. GW: Writing–original draft. BL: Writing–original draft. YC: Writing–original draft. LZ: Writing–review and editing. PZ: Writing–original draft. YW: Writing–review and editing. CC: Writing–review and editing.
Funding
The author(s) declare that financial support was received for the research, authorship, and/or publication of this article. This work was supported by the National Natural Science Foundation of China (grant number 42102076); the Open Foundation of State Key Laboratory of Coal and CBM Co-mining (grant number 2022KF26); and the Natural Science Foundation of Shandong Province (grant number ZR2021QD037).
Conflict of interest
Author CC was employed by Shanxi Institute of Geological Survey Co., Ltd.
The remaining authors declare that the research was conducted in the absence of any commercial or financial relationships that could be construed as a potential conflict of interest.
Publisher’s note
All claims expressed in this article are solely those of the authors and do not necessarily represent those of their affiliated organizations, or those of the publisher, the editors and the reviewers. Any product that may be evaluated in this article, or claim that may be made by its manufacturer, is not guaranteed or endorsed by the publisher.
References
Bakshi, S., He, Z. L., and Harris, W. G. (2015). Natural nanoparticles: implications for environment and human health. Crit. Rev. Environ. Sci. Technol. 45 (8), 861–904. doi:10.1080/10643389.2014.921975
Banfield, J. F., and Zhang, H. (2001). Nanoparticles in the environment. Rev. Mineralogy Geochem. 44 (1), 1–58. doi:10.2138/rmg.2001.44.01
Braunschweig, J., Bosch, J., and Meckenstock, R. U. (2013). Iron oxide nanoparticles in geomicrobiology: from biogeochemistry to bioremediation. New Biotechnol. 30 (6), 793–802. doi:10.1016/j.nbt.2013.03.008
Catling, D. C., and Moore, J. M. (2003). The nature of coarse-grained crystalline hematite and its implications for the early environment of Mars. Icarus 165 (2), 277–300. doi:10.1016/S0019-1035(03)00173-8
Cornell, R. M., Giovanoli, R., and Schindler, P. W. (1987). Effect of silicate species on the transformation of ferrihydrite into goethite and hematite in alkaline media. Clays Clay Minerals 35, 21–28. doi:10.1346/ccmn.1987.0350103
Cornell, R. M., and Schwertmann, U. (2003). The iron oxides: structure, properties, reactions, occurrences, and uses, 664. Weinheim: Wiley VCH.
Dibyanshu, K., Chhaya, T., and Raychoudhury, T. (2023). A review on the fate and transport behavior of engineered nanoparticles: possibility of becoming an emerging contaminant in the groundwater. Int. J. Environ. Sci. Technol. 20 (4), 4649–4672. doi:10.1007/s13762-021-03835-9
Frimmel, F. H., and Niessner, R. (2010). Nanoparticles in the water cycle: properties, analysis and environmental relevance. Dordrecht: Springer Science and Business Media LLC. doi:10.1007/978-3-642-10318-6
Gunnlaugsson, E., and Arnòrsson, S. (1982). The chemistry of iron in geothermal systems in Iceland. J. Volcanol. Geotherm. Res. 14 (3-4), 281–299. doi:10.1016/0377-0273(82)90066-X
Guo, H., and Barnard, A. S. (2013). Naturally occurring iron oxide nanoparticles: morphology, surface chemistry and environmental stability. J. Mater. Chem. A 1 (1), 27–42. doi:10.1039/C2TA00523A
Handy, R. D., Owen, R., and Valsami-Jones, E. (2008). The ecotoxicology of nanoparticles and nanomaterials: current status, knowledge gaps, challenges, and future needs. Ecotoxicology 17, 315–325. doi:10.1007/s10646-008-0206-0
Hochella, M. F., Aruguete, D., Kim, B., and Madden, A. S. (2012). Naturally occurring inorganic nanoparticles: general assessment and a global budget for one of earth’s last unexplored major geochemical components. Nature’s nanostructures, 1–31.
Hochella Jr, M. F., Lower, S. K., Maurice, P. A., Penn, R. L., Sahai, N., Sparks, D. L., et al. (2008). Nanominerals, mineral nanoparticles, and earth systems. Science 319 (5870), 1631–1635. doi:10.1126/science.1141134
Inskeep, W. P., Ackerman, G. G., Taylor, W. P., Kozubal, M., Korf, S., and Macur, R. E. (2005). On the energetics of chemolithotrophy in nonequilibrium systems: case studies of geothermal springs in Yellowstone National Park. Geobiology 3 (4), 297–317. doi:10.1111/j.1472-4669.2006.00059.x
Ju, Y., Li, X., Ju, L., Feng, H., Tan, F., Cui, Y., et al. (2022). Nanoparticles in the Earth surface systems and their effects on the environment and resource. Gondwana Res. 110, 370–392. doi:10.1016/j.gr.2022.02.012
Ju-Nam, Y., and Lead, J. R. (2008). Manufactured nanoparticles: an overview of their chemistry, interactions and potential environmental implications. Sci. total Environ. 400 (1-3), 396–414. doi:10.1016/j.scitotenv.2008.06.042
Kaasalainen, H., and Stefánsson, A. (2012). The chemistry of trace elements in surface geothermal waters and steam, Iceland. Chem. Geol. 330, 60–85. doi:10.1016/j.chemgeo.2012.08.019
Kaasalainen, H., Stefánsson, A., and Druschel, G. K. (2017). Geochemistry and speciation of Fe (II) and Fe (III) in natural geothermal water, Iceland. Appl. Geochem. 87, 146–157. doi:10.1016/j.apgeochem.2017.10.021
Kim, B. Y. S., Rutka, J. T., and Chan, W. C. W. (2010). Nanomedicine. N. Engl. J. Med. 363 (25), 2434–2443. doi:10.1056/nejmra0912273
Kinniburgh, D. G., Jackson, M. L., and Syers, J. K. (1976). Adsorption of alkaline earth, transition, and heavy metal cations by hydrous oxide gels of iron and aluminum. Soil Sci. Soc. Am. J. 40 (5), 796–799. doi:10.2136/sssaj1976.03615995004000050047x
Liu, R., Cao, J., and Hu, G. (2021). Revealing a new transport form of natural material by naturally occurring spherical amorphous silica particles in soil aerosol. Chem. Geol. 559, 119950. doi:10.1016/j.chemgeo.2020.119950
Liu, R., Cao, J., Luo, S., Liao, Y., and Chen, J. (2020). Particulates in the ascending gas caused by the 2013 Ya'an earthquake: a new matter released by earthquake. Geol. J. 55 (2), 1216–1226. doi:10.1002/gj.3487
Ma, R. (2007). Water-rock interaction and genesis of low-medium temperature thermal groundwater in carbonate reservoir: a case study at Taiyuan. Shanxi: China University of Geosciences.
Madden, A. S., Hamilton, V. E., Madden, M. E., Larson, P. R., and Miller, M. A. (2010). Low-temperature mechanism for formation of coarse crystalline hematite through nanoparticle aggregation. Earth Planet. Sci. Lett. 298 (3-4), 377–384. doi:10.1016/j.epsl.2010.08.014
McCleskey, R. B., Chiu, R. B., Nordstrom, D. K., Campbell, K. M., Roth, D. A., Ball, J. W., et al. (2014). Water chemistry data for selected springs, geysers, and streams in yellowstone national park, Wyoming, beginning 2009. doi:10.5066/F7M043FS
McKay, D. S., Gibson Jr, E. K., Thomas-Keprta, K. L., Vali, H., Romanek, C. S., Clemett, S. J., et al. (1996). Search for past life on Mars: possible relic biogenic activity in Martian meteorite ALH84001. Science 273 (5277), 924–930. doi:10.1126/science.273.5277.924
Murad, E., and Schwertmann, U. (1983). The influence of aluminium substitution and crystallinity on the Mössbauer spectra of goethite. Clay Miner. 18 (3), 301–312. doi:10.1180/claymin.1983.018.3.07
Nordstrom, D. K., Ball, J. W., and McCleskey, R. B. (2005). Ground water to surface water: chemistry of thermal outflows in Yellowstone National Park. Geotherm. Biol. Geochem. Yellowst. Natl. Park, 73–94.
Peng, H. H., Martel, J., Lee, Y. H., Ojcius, D. M., and Young, J. D. (2011). Serum-derived nanoparticles: de novo generation and growth in vitro, and internalization by mammalian cells in culture. Nanomedicine 6 (4), 643–658. doi:10.2217/nnm.11.24
Pope, J., and Brown, K. L. (2014). Geochemistry of discharge at Waiotapu geothermal area, New Zealand–trace elements and temporal changes. Geothermics 51, 253–269. doi:10.1016/j.geothermics.2014.01.006
Raiswell, R. (2011). Iceberg-hosted nanoparticulate Fe in the Southern Ocean: mineralogy, origin, dissolution kinetics and source of bioavailable Fe. Deep Sea Res. Part II Top. Stud. Oceanogr. 58 (11-12), 1364–1375. doi:10.1016/j.dsr2.2010.11.011
Raiswell, R., Benning, L. G., Davidson, L., Tranter, M., and Tulaczyk, S. (2009). Schwertmannite in wet, acid, and oxic microenvironments beneath polar and polythermal glaciers. Geology 37 (5), 431–434. doi:10.1130/G25350A.1
Raiswell, R., Benning, L. G., Tranter, M., and Tulaczyk, S. (2008). Bioavailable iron in the Southern Ocean: the significance of the iceberg conveyor belt. Geochem. Trans. 9, 7–9. doi:10.1186/1467-4866-9-7
Raiswell, R., Vu, H. P., Brinza, L., and Benning, L. G. (2010). The determination of labile Fe in ferrihydrite by ascorbic acid extraction: methodology, dissolution kinetics and loss of solubility with age and de-watering. Chem. Geol. 278 (1-2), 70–79. doi:10.1016/j.chemgeo.2010.09.002
Rich, H. W., and Morel, F. M. (1990). Availability of well-defined iron colloids to the marine diatom Thalassiosira weissflogii. Limnol. Oceanogr. 35 (3), 652–662. doi:10.4319/lo.1990.35.3.0652
Rue, E. L., and Bruland, K. W. (1995). Complexation of iron (III) by natural organic ligands in the Central North Pacific as determined by a new competitive ligand equilibration/adsorptive cathodic stripping voltammetric method. Mar. Chem. 50 (1-4), 117–138. doi:10.1016/0304-4203(95)00031-L
Shao, G., Li, C., Liu, R., Zhang, P., Zuo, L., and Wang, Y. (2023). Silicified microorganisms and microorganism-like particles in the groundwater of an abandoned coal mine. Mine Water Environ. 42 (3), 489–499. doi:10.1007/s10230-023-00945-3
Shock, E. L., Holland, M., Amend, J. P., Osburn, G. R., and Fischer, T. P. (2010). Quantifying inorganic sources of geochemical energy in hydrothermal ecosystems, Yellowstone National Park, USA. Geochimica Cosmochimica Acta 74 (14), 4005–4043. doi:10.1016/j.gca.2009.08.036
Stefánsson, A., Gıslason, S. R., and Arnórsson, S. (2001). Dissolution of primary minerals in natural waters: II. Mineral saturation state. Chem. Geol. 172 (3-4), 251–276. doi:10.1016/S0009-2541(00)00262-X
Stumm, W., and Sulzberger, B. (1992). The cycling of iron in natural environments: considerations based on laboratory studies of heterogeneous redox processes. Geochimica Cosmochimica Acta 56 (8), 3233–3257. doi:10.1016/0016-7037(92)90301-X
Tan, X., Bo, B., Zhang, P., Shao, G., Liu, R., and Wang, K. (2021). Carbonaceous nanoparticles in Zibo hot springs: implications for the cycling of carbon and associated elements. Environ. Chem. Lett. 19, 4009–4014. doi:10.1007/s10311-021-01267-y
Tobler, D. J., Stefánsson, A., and Benning, L. G. (2008). In-situ grown silica sinters in Icelandic geothermal areas. Geobiology 6 (5), 481–502. doi:10.1111/j.1472-4669.2008.00179.x
Wang, L., Li, C., Liu, S., Li, H., Xu, M., Yu, D., et al. (2005). Terrestrial heat flow distribution in kuqa foreland basin, tarim, NW China. Petroleum Explor. Dev. 32 (4), 79–83.
Ward, L. M., ldei, A., Nakagawa, M., et al. (2018). Thermophilic lithotrophy and phototrophy in an lntertidal, lron-rich, geothermal spring. Environ. Sci. Geol. bioRxiv, 2018. doi:10.1101/428698
Wells, M. L., Zorkin, N. G., and Lewis, A. G. (1983). The role of colloid chemistry in providing a source of iron to phytoplankton. J. Mar. Res. 41 (4), 731–746. doi:10.1357/002224083788520478
Wigginton, N. S., Haus, K. L., and Hochella Jr, M. F. (2007). Aquatic environmental nanoparticles. J. Environ. Monit. 9 (12), 1306–1316. doi:10.1039/B712709J
Wu, C. Y., Martel, J., Wong, T. Y., Young, D., Liu, C. C., Lin, C. W., et al. (2016). Formation and characteristics of biomimetic mineralo-organic particles in natural surface water. Sci. Rep. 6 (1), 28817. doi:10.1038/srep28817
Young, J. D., Martel, J., Young, L., Wu, C. Y., Young, A., and Young, D. (2009). Putative nanobacteria represent physiological remnants and culture by-products of normal calcium homeostasis. PloS one 4 (2), e4417. doi:10.1371/journal.pone.0004417
Zhang, W. B. (2013). Fluid geochemistry of springs in the basin and range Province on the northwest of being. China Earthquake Administratiuon: Institute of earthquake science.
Zhou, S. C., Liu, X. H., Tong, C. H., and Hu, B. (2014). Application research of geogas survey in prospecting concealed ore. Acta Geol. Sin. 88 (4), 736–754.
Keywords: Iron, geothermal water, nanoparticulate Fe, environmental indicator, TEM
Citation: Chen Z, Liu R, Wang Y, Lu B, Cui Y, Zuo L, Zhang P, Wang Y and Cao C (2024) Biomimetic Fe-bearing nanoparticles in hot spring: morphology, origin and potential bioavailable Fe. Front. Earth Sci. 12:1404788. doi: 10.3389/feart.2024.1404788
Received: 21 March 2024; Accepted: 28 May 2024;
Published: 11 June 2024.
Edited by:
Hongye Feng, Chinese Academy of Geological Sciences, ChinaReviewed by:
Zebang Yi, Guilin University of Technology, ChinaTianyuan Xu, China University of Mining and Technology, China
Hongling Bu, Guangdong University of Technology, China
Copyright © 2024 Chen, Liu, Wang, Lu, Cui, Zuo, Zhang, Wang and Cao. This is an open-access article distributed under the terms of the Creative Commons Attribution License (CC BY). The use, distribution or reproduction in other forums is permitted, provided the original author(s) and the copyright owner(s) are credited and that the original publication in this journal is cited, in accordance with accepted academic practice. No use, distribution or reproduction is permitted which does not comply with these terms.
*Correspondence: Rui Liu, bGl1cnVpQHNkdXQuZWR1LmNu