- 1State Key Laboratory for Fine Exploration and Intelligent Development of Coal Resources, China University of Mining and Technology, Xuzhou, China
- 2School of Safety Engineering, China University of Mining and Technology, Xuzhou, China
With the continuous increase of mining depth, coal and gas outburst poses a significant threat to mining safety. Conducting research on the mechanisms of coal and gas outbursts contributes to understanding the evolutionary process of such incidents, thus enabling accurate prediction and prevention of coal and gas outbursts during mining operations. This paper has developed a comprehensive visual experimental system that is specifically tailored to simulate diverse coal body conditions, ground stress and gas pressures. By monitoring and analyzing the real-time progression of coal fissures during the outburst process, we can obtain valuable insights into the evolution and mechanisms of coal and gas outbursts. Additionally, this study introduces a method to determine the critical threshold for predicting coal and gas outbursts, and the critical gas pressure threshold for Jiulishan Coal Mine (Jiaozuo City, Henan Province, China) is established at 0.6 MPa.
1 Introduction
Coal and gas outbursts are geological hazards characterized by the sudden ejection of fractured coal, rock and gas from the coal seam into the mining space, resulting from the combined effects of ground stress and gas (Li et al., 2023; Liu et al., 2023; Soleimani et al., 2023). The occurrence of coal and gas outbursts generates strong shockwaves with tremendous destructive power, posing significant threats to the safety of miners and mining facilities (Black, 2019a; Mekhtiev et al., 2021). With the continuous growth in energy demand and the increase in mining intensity and depth, the complex interactions among stress, gas, coal and rock have made the geological conditions of mines increasingly complicated, the probability of coal and gas outburst events increases (Li et al., 2019; Kursunoglu and Onder, 2019; Ul’yanova et al., 2019). The intricate mining conditions in deep mines give rise to distinct features of coal and gas outbursts, which are marked by heightened energy levels, intensity, and challenges in prevention and control efforts (Odintsev and Makarov, 2020; Gawor and Wasilewski, 2021; Remennikov et al., 2022; Shang et al., 2023). Therefore, studying the evolutionary processes and mechanisms of coal and gas outbursts holds significant importance for the safe operation of coal mines.
Previous research has conducted extensive research on the mechanisms of coal and gas outbursts and have proposed a range of hypotheses, including the “rheological hypothesis,” “failure of the coal shell hypothesis,” “unified instability theory,” “fluid-solid coupling instability theory,” and the composite hypothesis (Jiang et al., 2015; Yuan, 2017; Jin et al., 2018). These studies still have important guiding significance for current research. In recent years, with the advancement of experimental methods, research on the outburst mechanisms has continued to deepen. Koziel et al. (2022) conducted a study to investigate the prominent energy source in the porous structure of rocks, which is due to the presence of high-pressure gas. The prominence of this energy source is determined by the type of transformation that occurs during the decompression process. Zhao et al. (2022) conducted coal and gas outburst experiments under deep high-stress conditions and studied the stress-gas-pressure-temperature response characteristics of coal and rock around the outburst hole. The experimental findings indicate a significant stress response in the vicinity of the blast hole, with diminishing stress changes as the distance from the blast hole position increases. Based on these observations, one can deduce that the principal energy source for coal and gas outbursts originates from the internal energy of gas.
Coal possesses a complex fractured morphology, cracks and the finely porous structure (Ul’yanova et al., 2019; Godyn and Kozusnikova, 2019), experiences the formation of gas-bearing fractures due to the extension and convergence of microcracks under the ground stress. The cracks act as direct indicators of coal damage, and a comprehensive analysis of their attributes and formations can unveil the processes and mechanisms behind the onset and development of disasters (Odintsev and Makarov, 2020; Gao et al., 2021). Feldman et al. (2017) proposed a model to describe the development of primary fractures in coal seams under rapid unloading conditions, along with criteria for fracture occurrence and fracture timing in the edge areas of the coal seams. The study revealed that in virgin gas-bearing coal seams, fractures are influenced by both rock pressure and gas pressure within the coal seam fissures. Zhu et al. (2022) used mercury intrusion porosimetry and gas adsorption methods to investigate the pore structure characteristics of coal samples from highly gassy coal seams. The results indicated that during the initial stage of crack propagation, the main cracks on the coal samples extended along the direction of natural fractures. As the cracks expanded, the surface fractal dimension of the main cracks increased, and their degree of curvature intensified. The propagation path of the main crack is mainly affected by natural fractures and loads. Odintsev and Shipovskii. (2019) investigated the mechanisms underlying the formation of dynamic fracture in outburst-hazardous coal band under the influence of coal seam explosions. Furthermore, the researchers made estimations regarding the initial timing of crack development under the pressure exerted by liberated methane. The results indicate that the onset of crack development varies significantly, ranging from several tens of seconds to several hours, depending on the distinct mechanical characteristics exhibited by different coal samples.
Obviously, extensive literatures have carried out the coal and gas outburst simulation experiments. However, the interaction mechanism between various influencing factors is still controversial in the process of prominent events. Therefore, the design of a simulated experimental device for real-time dynamic observation of the fracture evolution of coal during coal and gas outbursts is of great significance for investigating the mechanisms of coal and gas outbursts and improving prevention measures. Meanwhile, the variations in gas pressure, ground stress, and mechanical characteristics of coal are substantial across different mining areas. The complex interaction of various influencing factors greatly impacts the occurrence of coal and gas outbursts, rendering their prediction challenging (Black, 2019b; Amani et al., 2021; Shadrin et al., 2022). Therefore, it is indispensable to formulate an accurate approach to anticipate the crucial thresholds of indicators related to coal and gas outbursts in different mining areas (Agrawal et al., 2023; Zheng et al., 2023).
In this paper, a comprehensive simulation experiment system was designed. The simulation experiments were conducted to investigate the effects of ground stress, gas pressure and coal properties on the occurrence of outbursts. Real-time observation and analysis of the generation, expansion and movement of coal fractures during coal and gas outbursts were conducted, and the experimental data were fitted. The interaction among ground stress, gas pressure and coal properties during coal and gas outbursts was analyzed, and the process of coal cracks during the outburst evolution was determined was analyzed. Additionally, a method was proposed for determining the critical values to predict coal and gas outbursts, and the critical value for gas pressure was found to be 0.6 MPa for the Jiusan Mine (Jiaozuo City, Henan Province, China).
2 Experimental section
2.1 Experimental system
As shown in Figure 1, the coal and gas outburst experiment system consist of a pressure loading device, gas loading device and observation device. The pressure loading device is primarily composed of a computer-controlled electro-hydraulic servo pressure testing machine, which applies stress to the coal to simulate the ground stress by the coal underground. The gas loading device is used to fill the coal samples in the apparatus with gas, and the observation device includes a high-speed camera and a transparent organic glass window. The high-speed camera is positioned facing the transparent window to capture the fracture evolution process of the coal during the outburst. In order to capture and analyze coal and gas outburst processes, the frame rate of the high-speed camera was set to 2,000 frames per second.
2.2 Sample preparation
In coal and gas outburst simulation experiments, the physical and mechanical conditions of the coal body are important factors affecting the outburst process. To compare the impact of different coal types on the outburst, this experiment used both coal briquette and lump coal as the coal samples. By controlling the ratio of coal powder to coal tar, the coal briquette was prepared with different physical and mechanical properties. The preparation of coal briquette was performed directly in the outburst experiment apparatus, the pre-prepared coal powder was loaded into the apparatus in three stages, with each stage being pressurized at 20 MPa. The pressurization was done using a hydraulic press at a rate of 300 N/s, and once the predefined pressure was reached, it was maintained for 30 min. Before pressing the coal briquette, the outburst opening needed to be sealed to ensure proper bonding between the prepared coal and the container wall. For the lump coal outburst experiments, large coal samples were selected from the field and then cut into pieces that matched the volume of the experimental container. These coal pieces were placed in the experimental apparatus, and coal powder was used to fill the gaps between the coal samples and the container wall.
3 Experimental results
3.1 Results of simulation experiments on coal and gas outburst
Different physical and mechanical properties of coal briquette were prepared by changing the ratio of coal powder to coal tar. A stress of 10 MPa was applied to the coal samples to simulate ground stress, and the minimum gas pressure required for coal outburst under this stress was measured. The experimental results are presented in Table 1.
In the absence of coal tar additives, after directly applying pressure to the coal samples, no outbursts occurred at a gas pressure of 0.20 MPa, and only minor fissures appeared. When the gas pressure increases to 0.22 MPa, the outburst phenomenon occurs. However, when a coal briquette made of 7% coal tar was added, no outbursts occurred at a gas pressure of 0.49 MPa, and the outbursts occurred when the gas pressure increased to 0.72 MPa. Similarly, when a coal briquette made of 15% coal tar was added, no outbursts occurred at a gas pressure of 0.58 MPa. The outbursts occurred when the gas pressure increased to 1.25 MPa.
Coal and gas outbursts often occur in coal seams with soft layers, however, with the increase of mining depth, the ground stress and gas pressure increase correspondingly, and the probability of hard coal outburst increases significantly (Yuan et al., 2021; Kursunoglu and Onder, 2023). In the hard coal outburst experiment, when the flap of the outburst mouth is suddenly opened, the coal undergoes a transition from being subjected to three-dimensional forces to being subjected to two-dimensional forces, resulting in the rapid release of stored elastic energy. Table 2 displays the outburst conditions of hard coal. Figure 2 illustrates the distribution of coal fractures after an experiment with a gas pressure of 0.65 MPa and a ground stress of 15 MPa. Multiple fractures appear near the outburst mouth, with small widths and primarily in the vertical direction. Furthermore, the upper part of the coal body experiences more severe damage, resulting in the fragmentation into several larger coal chunks.
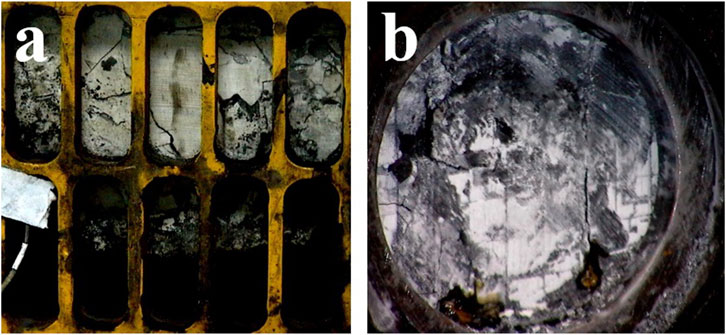
FIGURE 2. Hard coal sample after outburst in 0.65 MPa of gas pressure and 15 MPa of ground stress: (A) The crack fracture of flank, (B) The crack fracture of outburst mouth.
Figure 3 illustrates the distribution of coal fractures after an experiment with a gas pressure of 0.75 MPa and a ground stress of 20 MPa. The outburst mouth exhibits two significant connected and branched shear fractures, with a noticeable increase in width. From the side cracks, it can be observed that the coal near the outburst mouth experiences further damage, resulting in the fragmentation into smaller particles. Furthermore, the fractures of coal located farther from the outburst mouth have certain closure. By considering the results of the side crack observations, it can be concluded that the corner position of the coal block undergoes the most severe damage.
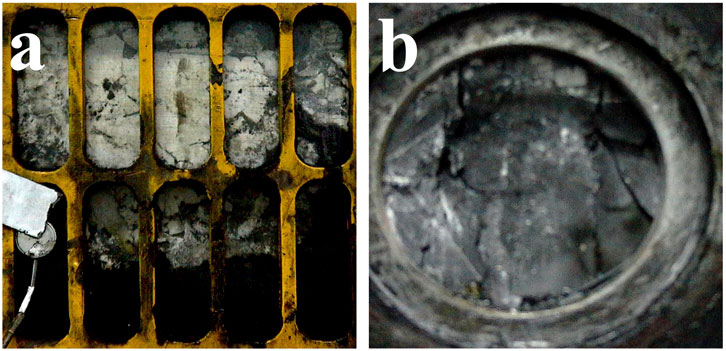
FIGURE 3. Hard coal sample after outburst in 0.75 Mpa of gas pressure and 20 Mpa of ground stress: (A) The crack fracture of flank, (B) The crack fracture of outburst mouth.
Figure 4 illustrates the distribution of coal fractures after an experiment with a gas pressure of 0.6 MPa and a ground stress of 20 MPa. Under the gas pressure of 0.6 MPa and the in-situ stress of 20 MPa, the outburst of hard coal occurred, the dynamic characteristics during the outburst were not significant. The hard coal did not exhibit a pronounced ejection phenomenon, resulting in relatively large coal particles left after the outburst. After the outburst occurs, a hole with a large opening and a small cavity formed inside the coal body, and the coal surrounding the cavity became quite loose. Under this experimental condition, the coal located below the outburst mouth was mostly unaffected by the outburst, and the degree of fragmentation was minimal. The coal located below the outburst mouth was not subjected to a change in stress state when the outburst mouth opened, and the elastic energy in the coal was not rapidly released in a short time, thus not providing a rapid release pathway for the gas within the coal, resulting in minimal damage to the coal. As shown in Table 2, in the case of the same ground stress, when the gas pressure increases, the outburst phenomenon does not occur. This is due to the outburst mouth baffle and the coal block inside the device do not come into contact with each other, and when the outburst occurs and the protruding mouth baffle opens, the coal body is not completely and quickly depressurized, and the stored elastic energy cannot be released quickly.
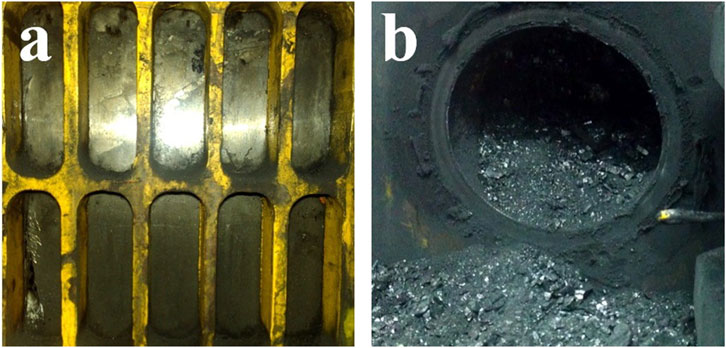
FIGURE 4. Hard coal sample after outburst in 0.6 Mpa of gas pressure and 20 Mpa of ground stress: (A) The crack fracture of flank, (B) The crack fracture of outburst mouth.
The impact of ground stress on the outburst of coal briquette is illustrated in Table 3, indicating that no outbursts occurred under the ground stress of 5 MPa and 7.5 MPa. However, when the ground stress increased to 10.41 MPa, an outburst phenomenon occurred, with a mass of 3.2 kg, accounting for 45.7% of the total coal mass. This indicates that coal briquette containing 7% coal tar exhibit outburst phenomena within a certain range of critical stress threshold under a gas pressure of 0.37 MPa.
3.2 The distribution of coal cracks in the outburst process
Coal and gas outbursts are rapid processes that can be completed in just a few seconds. In order to analyze the evolution of cracks during the outburst process, this study conducted continuous observations using a high-speed camera system. Figure 5 presents the evolution of coal cracks captured by the high-speed camera during the moment of outburst, the entire outburst process is less than 0.1 s. After the outburst occurs, the coal in the outburst area is quickly expelled, followed by the emergence of cracks. Initially, the cracks appear at the top of the coal and gradually propagate towards the interior. The crack propagation speed is first fast and then slow, and finally tends to stop. Additionally, when the gas pressure exceeds 0.48 MPa, no cracks were observed during the outburst process.
Figure 6A illustrates the evolution process of coal fractures during the outburst process, while Figures 6B, C depict the displacement and velocity plots of the marked particles in Figure 6A. From Figure 6B, it can be observed that the trajectory of the coal particle motion also approximates a parabolic shape. The particle exhibits significant displacement, moving approximately 0.06 m along the x-axis according to the recorded observations on the graph. After outburst, the motion velocity of the coal particle rapidly increases, reaching a maximum speed of 1.1 m per second, and gradually decreases, reaching its maximum value at 0.15 s. Once the outburst occurs, the coal was expelled rapidly under the driving force of gas pressure.
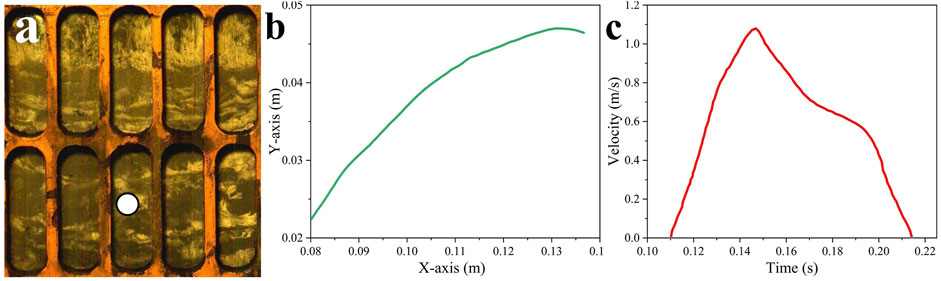
FIGURE 6. (A) Schematic diagram of particle trajectories taken from coal relative position, (B) The trajectory of particle motion, (C) The velocity of particle motion.
4 Discussion
4.1 The analysis of coal and gas outburst laws and influencing factors
In the coal briquette outburst experiment, the occurrence of coal and gas outburst phenomena in the coal body under low coal tar content and small gas pressure was observed, and the frequency of coal outbursts increased as the gas pressure increased. Furthermore, the ability of coal bodies with different intensities to resist outburst varied, resulting in different minimum values of gas pressure required for outburst. During the outburst experiment with hard coal, the in-situ stress led to the continuous accumulation of elastic energy within the coal body. The critical factor for outbursts in hard coal is the release of elastic energy during the decompression process (Rudakov and Sobolev, 2019; Soleimani et al., 2023).
As shown in Table 3, conclusions can be drawn from the results of the 4th, 5th, and 6th experimental groups, where coal samples containing 7% coal tar exhibit a range of critical ground stress thresholds for outburst phenomena under a gas pressure of 0.46 MPa. In summary, the Eq. 1 and Eq. 2 can be derived:
To further analyze the impact of pressure of briquette and gas coupling on outbursts, the influence mechanisms of the outburst occurrence was researched under the different outburst mouth when the gas pressure was 0.49 MPa, the experimental findings are presented in Table 4. A layer of sealing rubber film was added to the outburst mouth while maintaining the experimental conditions unchanged. After the induction step of the outburst, the coal mass did not experience an outburst but exhibited a certain degree of swelling. At the moment of the outburst occurrence, the barrier plate on the outburst opening was suddenly removed, resulting in deformation and fragmentation of the coal mass near the opening under the influence of stress, causing it to move towards the outburst mouth. However, due to the obstructive effect of the thin film, no pressure differential was formed around the outburst opening, preventing the outburst of the coal. According to the report of Wang et al. (2018), the release of a certain level of coal stress alone cannot lead to an outburst without the involvement of gas. In the third group of experiments, while keeping other conditions unchanged, there was no contact between the coal mass near the outburst opening and the barrier plate. Therefore, during the outburst moment, when the outburst barrier plate was removed, there was no depressurization, fragmentation, or deformation of the coal mass near the outburst opening. Although there was a pressure difference between the coal seam gas and the atmosphere at this time, the resistance of the coal mass prevented the occurrence of an outburst. The analysis mentioned above leads to the conclusion that the impact of ground stress on outbursts is primarily evident during the occurrence of the outburst, if the ground stress cannot be rapidly released at that moment, its influence on the outburst will be significantly reduced. For instance, when the outburst baffle does not contact with the coal body, even a slight increase in gas pressure will not occur. Similar conclusion has also been obtained in previous studies (Du and Wang, 2019; Wu et al., 2020), further confirming the rationality of this study.
During the excavation of the coal roadway, three zones persist in front of the working face, including the pressure relief zone, the stress concentration zone, and the original stress zone (Zhang et al., 2020). The pressure relief zone consistently exists during subsequent excavation cycles. Therefore, under equivalent conditions of coal strength and ground stress, a greater gas pressure value is required in the original coal seam for conditions conducive to outbursts as compared to the pressure during rock cross-cut coal. To investigate the impact of original gas pressure in the coal seam on coal and gas outbursts during tunnel excavation, this study conducted 7 sets of coal and gas outburst experiments under varying gas pressures. The experimental parameters are presented in Table 5. Under the conditions of coal tar content of 7%, pressure of briquette of 20 MPa, and ground stress of 10 MPa, a low gas pressure will not induce coal outbursts. However, when the gas pressure increases to 0.72 MPa, the outburst phenomenon occurs, and the outburst coal accounts for about 29.3% of the total coal.
The occurrence of coal and gas outburst is affected by many factors. It is of great significance to establish a method to accurately predict the critical value of coal and gas outburst index in different mining areas to reduce the risk of coal and gas outburst in coal mine production (Amani et al., 2021; Soleimani et al., 2023). Taking Jiulishan Coal Mine (Jiaozuo City, Henan Province, China) as an example, the critical value of mine gas pressure for outburst was determined by experiments. The coal briquette containing 7% coal tar was used as the coal sample to meet the requirement of similarity in the physical and mechanical properties of the coal body in the Jiulishan Coal Mine, and the ground stress was approximately 10 MPa calculated based on the burial depth. Preliminary estimation was conducted based on the possible occurrence of gas pressure, starting from 0.30 MPa in the experiments, and no outbursts were observed. Continuing the use of the original coal sample, experiments were conducted with an additional 0.2 MPa of gas pressure under unchanged conditions, and no outbursts occurred. The gas pressure was further increased by 0.2 MPa until reaching 0.72 MPa when an outburst occurred. Then, experiments were continued using the midpoint between the values of gas pressure causing outburst and non-outburst until an outburst occurred. This process continued iteratively until reaching an accuracy of 0.01 MPa. Through experimentation, the critical gas pressure for coal and gas outbursts in the Jiulishan Coal Mine was determined to be 0.6 MPa. The specific experimental steps and methods are shown in Table 6. According to relevant literature data (Zhang, 1995), the gas pressure critical value in the Jiaozuo mining area is 0.6 MPa, which is consistent with the gas pressure critical value determined in this experiment, indicating the accuracy of method used in determining the gas pressure critical value in this experiment.
4.2 The analysis of distribution law of coal cracks in the process of protrusion evolution
The experimental results show that the duration of the coal and gas outburst process is very short, and the time from the beginning to the end of the outburst is less than 0.1 s. After the outburst starts, the coal near the outburst mouth first peels off and pulverizes, and then develops from the surface to the interior and from the shallow to the deep. In order to describe the trajectory of coal ejection from the hole during the outburst, the coal in the experimental device can be divided into three parts: upper, middle, and lower. The upper part refers to the coal section that corresponds to the horizontal height where the outburst mouth is located, the middle part refers to the coal section at the same horizontal height as the outburst mouth, the lower part refers to the coal section below the horizontal height where the outburst mouth is located. The coal in the upper part near the outburst section is directly ejected from the outburst mouth after stripping off the coal wall. The coal far away from the outburst mouth is affected by the combined force of gas pressure and radial stress, and the trajectory of its movement is parabolic ejection, while under the obstruction of the coal in front, the coal that is unable to be ejected falls down under the influence of gravity in the lower part. The coal corresponding to the height of the outburst mouth moves towards the outburst mouth in a near-horizontal manner. The coal in the lower part moves towards the outburst mouth under the influence of gas pressure, and due to the inability to eject horizontally, its trajectory of movement near the outburst head is approximately arched and upward, and it is finally expelled rapidly after reaching the outburst mouth.
After the outburst starts, the outburst mouth of the coal body opens, the coal body is thrown out, and the broken coal accumulates near the outburst hole. A slight movement towards the outburst mouth can be observed in the accumulated coal after a short interval following the first outburst. This is because the crushed coal accumulated after the first outburst obstructs the gas outflow channel, leading to an increase in gas pressure inside the hole. When the gas pressure within the hole reaches a high level, the broken coal in the hole will be forcefully expelled by the gas pressure, resulting in a decrease in the gas pressure within the hole. The broken coal in the cavity will be forcefully ejected when the gas pressure reaches a high level. As the difference between the gas pressure inside the hole and the gas pressure in the coal wall increases, when the pressure difference reaches a certain level, another outburst may occur. Therefore, coal and gas outbursts are not completed in a single occurrence, but rather in an intermittent development process, and similar results were also observed by Cheng et al. (2023).
4.3 The analysis of the interaction of stress, gas pressure and coal body in the process of protrusion
The interaction of stress, gas pressure and coal body in the process of protrusion is a complex problem, in order to analyze this interaction in depth, this paper carries out several sets of experiments and selects the typical experimental results to illustrate them.
According to Figure 7, the relationship between the coal solidity coefficient and gas pressure can be obtained as shown in Eq. 3:
In Eq. 3, it can be seen that the gas pressure required to reach the protrusion condition increases as the coal body firmness coefficient increases.
Also, Eq. 4 can be obtained from Figure 8:
According to Eq. 4, it can be seen that the gas pressure required for protrusion decreases as the ground stress increases. However, when the gas pressure reaches a certain value, protrusion may still occur even if the ground stress is small. Specifically, whether or not protrusion occurs also depends on the physical and mechanical properties of the coal body.
Eq. 5 can be obtained by substituting Eq. 3 into Eq. 4:
It follows that the relationship between the three elements that determine the critical value is shown in Eq. 6.
5 Conclusion
In this paper, a protrusion simulation experiment system that integrates ground stress, gas pressure and coal properties is developed. Through the simulation experiment, the roles of ground stress, gas pressure and coal properties in outburst were studied, and the outburst experiment result data are fitted. With the increase of coal hardness, the gas pressure and ground stress required for outburst increase. In addition, the relationship between gas pressure and ground stress is also found, that is, increasing ground stress will lead to a decrease in the gas pressure required for outburst. It is worth noting that when the gas pressure reaches a certain value, protrusion is able to occur even if the ground stress is small. In addition, this paper proposes a method to determine the critical value for coal and gas protrusion prediction, and studies and determines the critical value of gas pressure of 0.6 MPa in Jiulishan Coal Mine (Jiaozuo City, Henan Province, China).
Data availability statement
The raw data supporting the conclusion of this article will be made available by the authors, without undue reservation.
Author contributions
JO: Conceptualization, Data curation, Formal Analysis, Funding acquisition, Investigation, Methodology, Project administration, Software, Writing–original draft, Writing–review and editing. EW: Funding acquisition, Writing–review and editing. ZL: Conceptualization, Data curation, Formal Analysis, Investigation, Writing–review and editing. NL: Methodology, Project administration, Resources, Writing–review and editing. HL: Software, Supervision, Validation, Visualization, Writing–review and editing. XW: Formal Analysis, Resources, Visualization, Writing–review and editing.
Funding
The author(s) declare financial support was received for the research, authorship, and/or publication of this article. This work was supported by the National Natural Science Foundation of China (No. 51974305) and the National Key R&D Program of China (2022YFC3004705).
Conflict of interest
The authors declare that the research was conducted in the absence of any commercial or financial relationships that could be construed as a potential conflict of interest.
Publisher’s note
All claims expressed in this article are solely those of the authors and do not necessarily represent those of their affiliated organizations, or those of the publisher, the editors and the reviewers. Any product that may be evaluated in this article, or claim that may be made by its manufacturer, is not guaranteed or endorsed by the publisher.
References
Agrawal, H., Durucan, S., Cao, W., Korre, A., and Shi, J.-Q. (2023). Rockburst and gas outburst forecasting using a probabilistic risk assessment framework in longwall top coal caving faces. Rock Mech. Rock Eng. 56 (10), 6929–6958. doi:10.1007/s00603-022-03076-3
Amani, K., Najafi, M., and Rafiee, R. (2021). Prediction of coal and gas outburst risk by fuzzy rock engineering system. Environ. Earth Sci. 80 (15), 491. doi:10.1007/s12665-021-09782-5
Black, D. J. (2019a). Review of coal and gas outburst in australian underground coal mines. Int. J. Min. Sci. Technol. 29 (6), 815–824. doi:10.1016/j.ijmst.2019.01.007
Black, D. J. (2019b). Review of current method to determine outburst threshold limits in Australian underground coal mines. Int. J. Min. Sci. Technol. 29 (6), 859–865. doi:10.1016/j.ijmst.2019.03.002
Cheng, L., Xu, J., Peng, S., Yang, H., Jiao, F., Zhou, B., et al. (2023). Dynamic behavior of outburst two-phase flow in a coal mine t-shaped roadway: the formation of impact airflow and its disaster-causing effect. Int. J. Min. Sci. Technol. 33 (8), 1001–1017. doi:10.1016/j.ijmst.2023.03.011
Du, F., and Wang, K. (2019). Unstable failure of gas-bearing coal-rock combination bodies: insights from physical experiments and numerical simulations. Process Saf. Environ. Prot. 129, 264–279. doi:10.1016/j.psep.2019.06.029
Feldman, E. P., Kalugina, N. A., and Meln'ik, T. N. (2017). Role of unloading and filtration of gas in the development of main cracks in coal seams. J. Appl. Mech. Tech. Phys. 58 (1), 155–164. doi:10.1134/s0021894417010175
Gao, K., Huang, P., Liu, Z., Liu, J., Shu, C., and Qiao, G. (2021). Coal-rock damage characteristics caused by blasting within a reverse fault and its resultant effects on coal and gas outburst. Sci. Rep. 11 (1), 19158. doi:10.1038/s41598-021-98581-w
Gawor, M., and Wasilewski, S. l. (2021). Investigations of dynamic properties of an integrated methane and rock outburst sensor. Measurement 186, 110178. doi:10.1016/j.measurement.2021.110178
Godyn, K., and Kozusnikova, A. (2019). Microhardness of coal from near-fault zones in coal seams threatened with gas-geodynamic phenomena, upper silesian coal basin, Poland. Energies 12 (9), 1756. doi:10.3390/en12091756
Jiang, C., Xu, L., Li, X., Tang, J., Chen, Y., Tian, S., et al. (2015). Identification model and indicator of outburst-prone coal seams. Rock Mech. Rock Eng. 48 (1), 409–415. doi:10.1007/s00603-014-0558-0
Jin, K., Cheng, Y., Ren, T., Zhao, W., Tu, Q., Dong, J., et al. (2018). Experimental investigation on the formation and transport mechanism of outburst coal-gas flow: implications for the role of gas desorption in the development stage of outburst. Int. J. Coal Geol. 194, 45–58. doi:10.1016/j.coal.2018.05.012
Koziel, K., Nowakowski, A., Sitek, L., and Skoczylas, N. (2022). Rock and gas outbursts in copper mines: use of brazilian tests to evaluate the work of disintegration of rock resulting from stresses produced by gas present in its porous structure. Rock Mech. Rock Eng. 55 (10), 6209–6225. doi:10.1007/s00603-022-02955-z
Kursunoglu, N., and Onder, M. (2019). Application of structural equation modeling to evaluate coal and gas outbursts. Tunn. Undergr. Space Technol. 88, 63–72. doi:10.1016/j.tust.2019.02.017
Kursunoglu, N., and Onder, M. (2023). Coal and gas outburst risk assessment using cluster analysis method. Acta Montan. Slovaca 28 (2), 325–343. doi:10.46544/AMS.v28i2.06
Li, H., Li, X., Fu, J., Zhu, N., Chen, D., Wang, Y., et al. (2023). Experimental study on compressive behavior and failure characteristics of imitation steel fiber concrete under uniaxial load. Constr. Build. Mater. 399 (8), 132599. doi:10.1016/j.conbuildmat.2023.132599
Li, J., Hu, Q., Yu, M., Li, X., Hu, J., and Yang, H. (2019). Acoustic emission monitoring technology for coal and gas outburst. Energy Sci. Eng. 7 (2), 443–456. doi:10.1002/ese3.289
Liu, H., Li, X., Yu, Z., Tan, Y., Ding, Y., Chen, D., et al. (2023). Influence of hole diameter on mechanical properties and stability of granite rock surrounding tunnels. Phys. Fluids 35 (6), 064121. doi:10.46544/AMS.v28i2.06
Mekhtiev, A. D., Yurchenko, A. V., Ozhigin, S. G., Neshina, E. G., and Al'kina, A. D. (2021). Quasi-distributed fiber-optic monitoring system for overlying rock mass pressure on roofs of underground excavations. J. Min. Sci. 57 (2), 354–360. doi:10.1134/s1062739121020198
Odintsev, V. N., and Makarov, V. V. (2020). Dynamic fracture of gas-bearing coal seam during zonal disintegration. J. Min. Sci. 56 (6), 932–946. doi:10.1134/s106273912006006x
Odintsev, V. N., and Shipovskii, I. E. (2019). Simulating explosive effect on gas-dynamic state of outburst-hazardous coal band. J. Min. Sci. 55 (4), 556–566. doi:10.1134/s1062739119045904
Remennikov, A., Kalubadanage, D. M., Yang, X., and Ren, T. (2022). The development of specialised modular protective structure on continuous miners against coal burst hazards. Int. J. Prot. Struct. 13 (2), 182–208. doi:10.1177/20414196211069574
Rudakov, D., and Sobolev, V. (2019). A mathematical model of gas flow during coal outburst initiation. Int. J. Min. Sci. Technol. 29 (5), 791–796. doi:10.1016/j.ijmst.2019.02.002
Shadrin, A. V., Diyuk, Y. A., and Teleguz, A. S. (2022). Substantiation of informational system for coal seam outburst danger continuous prediction with high level of the result veracity. Russ. J. Earth Sci. 22 (4), ES4001–21. doi:10.2205/2022es000799
Shang, S., Zhang, Q., Zhao, Y., Diao, Y., Yin, J., Che, Y., et al. (2023). Experimental research of the geo-stress evolution law and effect in the intact coal and gas outburst process. Front. Earth Sci. 10, 1036165. doi:10.3389/feart.2022.1036165
Soleimani, F., Si, G., Roshan, H., and Zhang, Z. (2023). Numerical modelling of coal and gas outburst initiation using energy balance principles. Fuel 334, 126687. doi:10.1016/j.fuel.2022.126687
Ul'yanova, E. V., Malinnikova, O. N., Pashichev, B. N., and Malinnikova, E. V. (2019). Microstructure of coal before and after gas-dynamic phenomena. J. Min. Sci. 55 (5), 701–707. doi:10.1134/s1062739119056063
Wang, H., Zhang, B., Yuan, L., Yu, G., and Wang, W. (2018). Gas release characteristics in coal under different stresses and their impact on outbursts. Energies 11 (10), 2661. doi:10.3390/en11102661
Wu, X., Peng, Y., Xu, J., Yan, Q., Nie, W., and Zhang, T. (2020). Experimental study on evolution law for particle breakage during coal and gas outburst. Int. J. Coal Sci. Technol. 7 (1), 97–106. doi:10.1007/s40789-019-00284-1
Yuan, A., Fu, G., and Hou, J. (2021). A multiscale structural analysis of soft and hard coal deposits in deep high-gas coal seams. Adv. Civ. Eng. 2021, 1–11. doi:10.1155/2021/8865038
Yuan, L. (2017). Scientific conception of precision coal mining. J. China Coal Soc. 42 (1), 1–7. doi:10.13225/j.cnki.jccs.2016.1661
Zhang, G. (1995). The prediction of coal and gas outburst in Jiaozuo mining area. J. Jiaozuo Min. Inst. 14 (1), 71–75.
Zhang, H., Wen, Z., Yao, B., and Chen, X. (2020). Numerical simulation on stress evolution and deformation of overlying coal seam in lower protective layer mining. Alexandria Eng. J. 59 (5), 3623–3633. doi:10.1016/j.aej.2020.06.011
Zhao, B., Wen, G., Ma, Q., Sun, H., Yan, F., and Nian, J. (2022). Distribution characteristics of pulverized coal and stress-gas pressure-temperature response laws in coal and gas outburst under deep mining conditions. Energy Sci. Eng. 10 (7), 2205–2223. doi:10.1002/ese3.1129
Zheng, X., Lai, W., Lei, Z., and Sheng, X. (2023). Quantitative evaluation of the indexes contribution to coal and gas outburst prediction based on machine learning. Fuel 338, 127389. doi:10.1016/j.fuel.2023.127389
Keywords: coal and gas outburst, spatio-temporal evolution, ground stress, gas pressure, coal properties
Citation: Ou J, Wang E, Li Z, Li N, Liu H and Wang X (2023) Experimental study of coal and gas outburst processes influenced by gas pressure, ground stress and coal properties. Front. Earth Sci. 11:1303996. doi: 10.3389/feart.2023.1303996
Received: 28 September 2023; Accepted: 12 December 2023;
Published: 29 December 2023.
Edited by:
Zhibo Zhang, University of Science and Technology Beijing, ChinaReviewed by:
Geng Jiabo, Jiangxi University of Science and Technology, ChinaKaizong Xia, Chinese Academy of Sciences (CAS), China
Copyright © 2023 Ou, Wang, Li, Li, Liu and Wang. This is an open-access article distributed under the terms of the Creative Commons Attribution License (CC BY). The use, distribution or reproduction in other forums is permitted, provided the original author(s) and the copyright owner(s) are credited and that the original publication in this journal is cited, in accordance with accepted academic practice. No use, distribution or reproduction is permitted which does not comply with these terms.
*Correspondence: Enyuan Wang, ZXl3YW5nY3VtdEAxNjMuY29t