- 1GNS Science, Avalon, Lower Hutt, New Zealand
- 2Volcanic Risk Solutions, School of Agriculture and Environment, Massey University, Palmerston North, New Zealand
- 3School of Earth and Environment, University of Canterbury, Christchurch, New Zealand
Volcanic eruptions can cause significant impacts on communities and infrastructure. There is an increasing need for effective risk assessments to inform decision-making and minimise the impact of volcanic hazards. Vulnerability models play a crucial role in these assessments, connecting the intensity of the hazard with the elements that are exposed to it, allowing for the calculation of potential impact or risk. There has been a large increase in the number of vulnerability models being developed for volcanic risk applications, and there is now a need to identify knowledge gaps for the field to take a strategic approach moving forward. This review aims to provide a high-level overview of the current state of volcanic vulnerability modelling and identify areas for future development. We evaluated 594 vulnerability models covering a range of elements and sectors, including buildings, critical infrastructure, transportation networks, agriculture, and human vulnerability. We reviewed the types of hazard intensity metrics and impact/risk metrics used in the models, modelling methodologies, underpinning data requirements, and uncertainty characterisation. A global clearinghouse for volcanic vulnerability models would be advantageous for the volcanic risk community to identify appropriate vulnerability models quickly and efficiently for their needs. As a first step towards such a clearinghouse, we have uploaded this volcano vulnerability model compilation to a repository and encourage additions/suggestions from the community on its future development. The results of this study will contribute to the advancement of the field and provide valuable insights for future research and development in volcanic risk assessment.
1 Introduction
As society’s exposure to volcanic hazards increases (Chester et al., 2000; Auker et al., 2013; Jenkins et al., 2022), it is necessary to build a comprehensive understanding of how societal elements (e.g., people, buildings, farms) will perform during future volcanic events (Jenkins et al., 2014; Wilson et al., 2014; Deligne et al., 2022). One common method of acquiring this knowledge is through the development of vulnerability models, which describe the relationship between the intensity of a hazard (e.g., tephra deposit thickness) and a corresponding impact to society (e.g., damage, disruption) (Figure 1). However, the efficacy of vulnerability models depends on the quality of data and may require bespoke approaches, which requires considerable knowledge and resources.
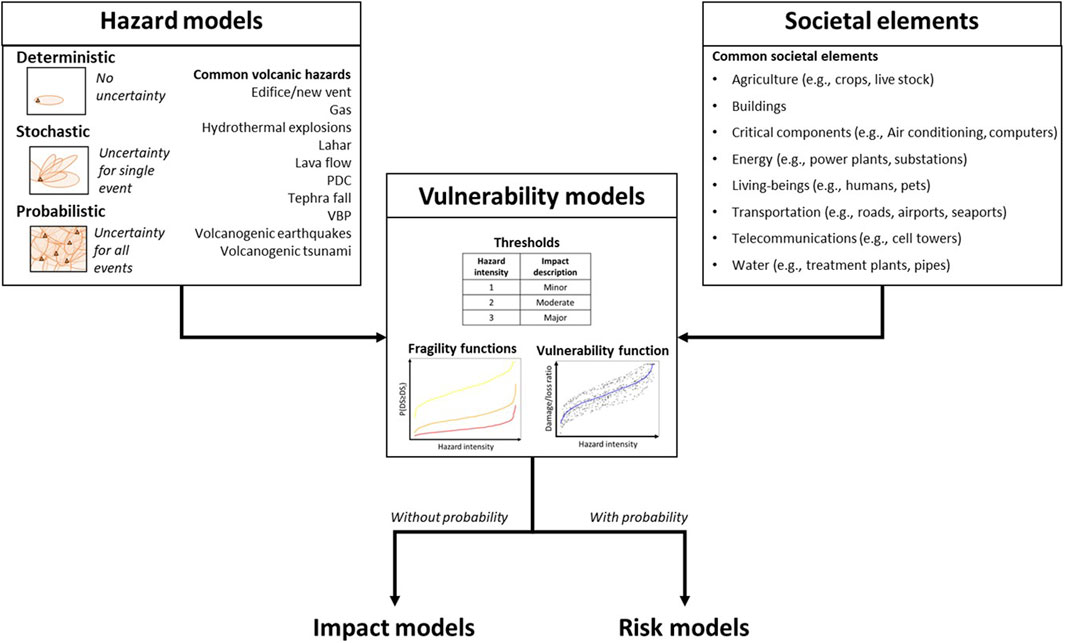
FIGURE 1. Conceptual framework and common terminology used in volcanic impact/risk assessment. Event refers to event-based simulations of hazardous processes. PDC = pyroclastic density current, VBP = volcanic ballistic projectiles.
Russell Blong’s seminal book “Volcanic Hazards: A Sourcebook on the Effects of Eruptions,” presented the most extensive compilation of the known societal impacts of volcanic eruptions (Blong, 1984), and has long served as an important evidence base for undertaking volcanic impact and risk analysis. In the decades since its publication, increasing demand for risk information among decision-makers has deepened and widened our understanding of the effects of volcanic eruptions through a diverse range of studies (see the review articles and references therein of: Wilson et al., 2012; 2014; Jenkins et al., 2014; Hayes et al., 2015; Deligne et al., 2022; Meredith et al., 2023). International initiatives such as the Global Volcano Model (GVM) and Global Assessment of Risk (GAR) 2015 have also put impetus on developing quantitative vulnerability models (Loughlin et al., 2015; Bonadonna et al., 2018). Together, these factors have enabled development of vulnerability models that are used to assess the extent that societal elements are impacted when exposed to volcanic hazards (Deligne et al., 2022). The objective of this contribution is to compile these models and summarise current trends and identify potential future directions to improve vulnerability model development for volcanic impact and risk assessment.
We used a global stock take of volcanic vulnerability models by Fitzgerald et al. (2023) as the underpinning dataset in our review (see their freely available report for their detailed methodology). We have endeavoured to comprehensively review a diverse compilation of vulnerability models, but it is inevitably non-exhaustive. This is because: 1) some vulnerability models may be unpublished (e.g., due to them being proprietary) and therefore unsearchable, 2) our own language barriers, which limited our ability to find models published in non-English documents, and 3) models may have been subsequently published since the development of the Fitzgerald et al. (2023) vulnerability model stock take report. Despite these limitations, we are confident our compilation is generally representative of the trends in volcanic vulnerability model development.
The Fitzgerald et al. (2023) review found there are many types of vulnerability models that have been developed and used in volcanology cover physical, socio-economic, systemic, and human vulnerability (Wilson et al., 2014; Bonadonna et al., 2021; Fitzgerald et al., 2023). Our focus in this mini review are those models that link hazard intensity to some societal element to estimate an impact. Some vulnerability models do not take this approach, and for some types of vulnerability (social vulnerability in particular) it may be more appropriate to take a hazard-agnostic approach that does not incorporate hazard intensity. Similarly, crisis decision support models (e.g., evacuation cost:benefit models or clean-up duration models) are not included, as we consider these out of scope of the present study. We have also not included threshold models for airspace closure (Biass et al., 2014; Scaini et al., 2014) due to the set standards and recommendations put forward by International Civil Aviation Organization (ICAO) and regulatory authorities. All of these models, whilst a fundamental aspect of understanding and identifying risk reduction strategies, are beyond the scope of analysis considered in this contribution.
2 Discussion and overview of published volcano vulnerability models
We have included 594 individual vulnerability models, published in 51 unique publications (academic papers, theses, technical reports) in our review (see Supplementary Material for full list). Below we provide an overview of some key vulnerability model characteristics we have identified from this compilation.
2.1 Hazard intensity and risk/impact metrics
The use of a hazard intensity metric (HIM) is an important consideration when developing a vulnerability model as it is the fundamental linkage between a hazardous process and a consequence when a given societal element is exposed to it. Numerous HIMs are used across different vulnerability models (Figure 2B). For tephra fall, both loading and thickness of tephra deposits are relatively easy to measure in the field and model via existing hazard models (e.g., Ash3d, Tephra2). This makes them convenient and widely used HIMs for vulnerability models. Many flow hazards (e.g., pyroclastic density currents (PDC) and lahar) have relied upon a relatively simple presence/absence relationship (also referred to as a binary relationship) where exposure equals a complete loss, destruction, total loss of service/function, or fatality. When used in a risk model, these effectively define the impact as resulting from a hazard intensity of≥0 or =/= 0 (Deligne et al., 2017). However, it is acknowledged that this assumption, while representative of the dominant impact level, is unlikely to be capturing the diversity of impacts possible and reflects the lack of data informing model development (Jenkins et al., 2013; Meredith et al., 2022). One key challenge is that volcanic hazards can have multiple impact modes (e.g., force, temperature, and chemical) (Jenkins et al., 2014; Wilson et al., 2014; Ligot et al., 2023). There are currently no vulnerability models that can handle these complex multi-mode impact interactions, and few available hazard models providing multiple hazard intensity metrics to apply within risk assessment frameworks to capture this diversity. A further challenge lies in the difficulty of distinguishing between these impact modes to determine the primary mechanism that initiated the impact. For example, it may be difficult to identify the relative damage contributions to a collapsed building that was exposed to ground-shaking from earthquakes, heavy tephra loading, and a lahar. This makes collecting empirical post-eruption field data, with both a clear hazard intensity and impact mechanisms and contextual factors a real challenge (Blong, 2003; Jenkins et al., 2017; Meredith et al., 2022). Whilst this may seem like a trivial academic issue, it can have considerable consequences for insurance payouts where policy wording may cover one form of damage but not another (Blong et al., 2017).
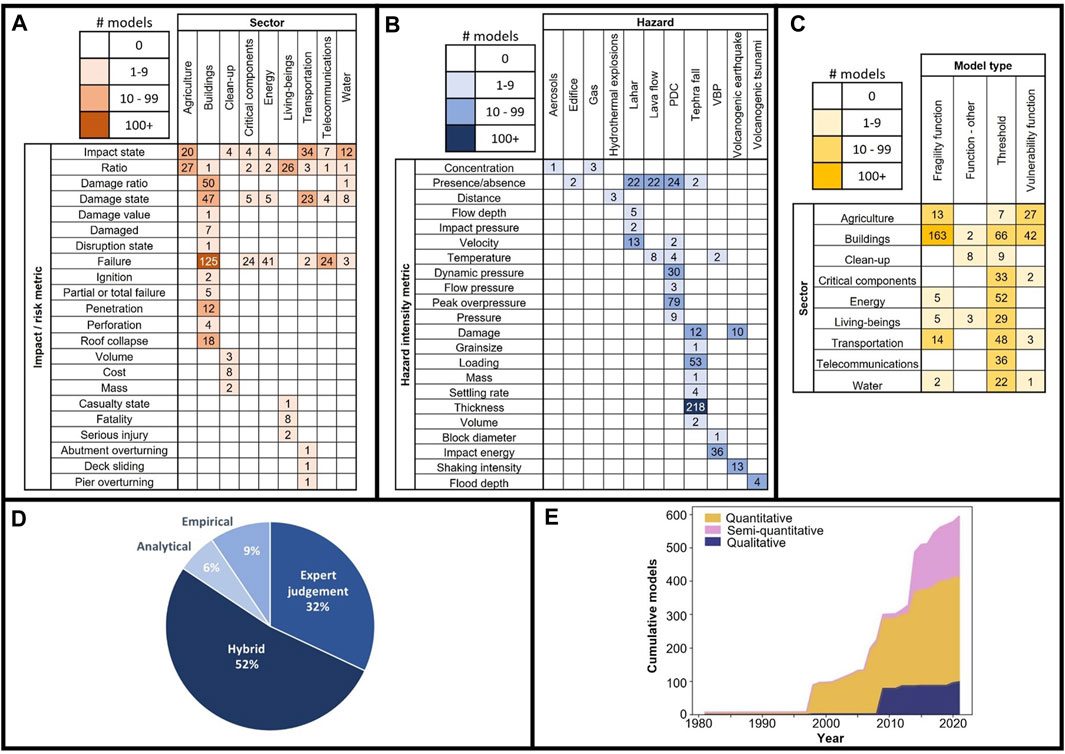
FIGURE 2. Overview vulnerability models contained within the review. (A) Risk/impact metric used in vulnerability models for different sectors, (B) Hazard types and the corresponding HIMs that are used, (C) Model types compared to sectors, (D) Derivation method of volcano vulnerability models, (E) Cumulative number of published vulnerability models classified by data type of the impact/risk metric.
A risk or impact metric describes how the consequences of a hazard are being expressed or calculated within a risk or impact assessment (e.g., monetary value, type of damage). A diverse range of risk/impact metrics have been used across different sectors, but the vast majority relate to a description of damage (e.g., failure, damage state, damage ratio) (Figure 2A).
2.2 Types of vulnerability models
Fragility functions (see Figure 1) for buildings are the most prevalent model in the review dataset (Figure 2C). This is because of traditional focus on building damage assessment for life safety reasons and a wide variety of building typologies across the world (Valentine, 1998; Pomonis et al., 1999; Spence et al., 2005; Zuccaro et al., 2008; Jenkins et al., 2014; Williams et al., 2017). For infrastructure vulnerability, the typical approach to date has been to identify a trigger point HIM threshold for different levels of physical impact or disruption. These thresholds are usually identified based on the minimum hazard intensity; these impacts have been observed resulting from eruptions (Wilson et al., 2012; Wilson et al., 2014). Vulnerability functions have been most dominantly developed for agriculture and buildings (Figure 2C). These two sectors often have a focus on assessing financial loss for insurance purposes (Magill et al., 2006; Wilson and Kaye, 2007; Zuccaro et al., 2013; Deligne et al., 2017; Leder et al., 2017; Craig et al., 2021), which may explain the dominance of vulnerability functions. While financial loss is also crucial for infrastructure damage (e.g., insurance and reinsurance for municipal authorities), it has been more common to identify an impact state, and then apply this within functionality, or economic disruption models at a regional scale (Blake et al., 2017; Mossoux et al., 2019; Santos et al., 2023). Life safety risk assessment requires models that link hazard intensity to human consequence (e.g., injury or fatality). Most vulnerability models used for this purpose apply threshold-based models, possibly due to these assessments being conservative (Newhall, 1982; Deligne et al., 2018; Fitzgerald, 2019) and limited available empirical information for robust statistical models, such as fragility functions.
2.3 Derivation methods
Vulnerability models have generally been derived in one of five ways through: 1) empirical observations of hazard-asset interactions during opportunistic field-based studies following volcanic eruptions (e.g., Blong, 2003), 2) physical experimental studies (e.g., Craig, 2015; Ligot, 2022), 3) expert judgement or elicitation methods (e.g., Maqsood 2014), 4) analytical frameworks (e.g., computational limit state analysis: Petrazzuoli and Zuccaro et al., 2004), or 5) by hybrid approaches that combine data from each or some of the preceding methods (e.g., Jenkins et al., 2014; Wilson et al., 2017). Each of these approaches have their advantages and shortcomings. For example, empirical observations may not include impacts at low hazard intensities due to a common focus on the most effected societal elements, and analytical approaches may lack real world validation. Most vulnerability models developed for volcanic hazards have been derived by hybrid methods (Figure 2D) (Jenkins et al., 2014; Wilson et al., 2014), and most commonly by combining field-based empirical data with expert judgement. These hybrid methods have been useful to supplement limited observational data or to reapply observational data in a different context (e.g., applying a model to a similar asset typology in another country). For example, fragility functions developed by Wilson et al. (2017) for a variety of infrastructure systems utilized relatively few observational datapoints of varying degrees of data quality in combination with expert judgement. Since there are relatively few empirical datasets for volcanic impacts compared to other perils (e.g., earthquakes or flooding) hybrid methods have been an important approach to developing useful vulnerability models across multiple sectors for different volcanic hazards.
3 Discussion and future research opportunities
In recent years there has been an increase in the availability of volcano vulnerability models (Figure 2E). We believe there is an opportunity to now build a community of practice to discuss issues around data standards, model testing/validation, and to importantly explore relationships between model developers and model users. Given the volume and diversity of vulnerability models, a sustainable and updated clearinghouse of vulnerability models would be advantageous for the volcanic risk research and practice community. This will require more community input than we have undertaken in this review, but we hope the initial compilation here can serve as a starting point. As a first step, we have uploaded this volcano vulnerability model compilation to Github (https://github.com/NZVHRM/Global_vulnerability_models), and welcome and encourage suggestions and contributions from the wider community to turn this into a useful community-maintained virtual clearinghouse for present and future workers.
In the below subsections we highlight a few areas we believe warrant continued attention from the volcano vulnerability model development community to consider as the field progresses and evolves.
3.1 Ensuring vulnerability models are robust and trusted
Uncertainty is ubiquitous in volcanic risk and impact assessments. Addressing uncertainty within vulnerability models has been inconsistent, but there is a growing emphasis that it is effectively incorporated and communicated. Fragility functions have uncertainty inherently built into them, and recent efforts to quantify the uncertainty around fragility function curves using statistical techniques such as bootstrapping bring this field into line with other comparable risk assessment fields (Williams et al., 2019). Thresholds are often used with an implicit assumption of a uniform probability between each threshold bin. This approach is suitable when there is limited knowledge regarding the relationship between hazard intensity and its subsequent impact. Moving forward, enhanced treatment of model uncertainty through additional data and statistical techniques (e.g., Bayesian inference), and communication of confidence in the model should be encouraged. This will ensure continued improvement in model performance, and critically decision-makers are able to make informed decisions based on their risk/impact assessment outputs.
It is important that vulnerability models are reliable and trusted to ensure that robust and salient risk information is provided to decision-makers. We note the performance accuracy of many vulnerability models remains untested and there are currently a limited number of examples of vulnerability model validation and benchmarking. This is partially due to limited empirical data to inform validation and benchmarking studies. Evaluating the performance accuracy of vulnerability models can be a challenging endeavour to undertake as it requires high quality impact datasets to compare with modelled results, but they are currently limited in number (Deligne et al., 2022). Further, impact datasets can present their own data quality challenges (Hayes et al., 2019; Meredith et al., 2022) that may make it difficult to directly compare model outputs with a realized event. None-the-less, increased use of model benchmarking and cross-validation processes will give users more confidence in the model accuracy, as well as identify new research gaps and opportunities for improvement.
3.2 Improving access and reuse of existing vulnerability models
Reuse of vulnerability models is beneficial to reduce duplication of effort and speed of analysis when conducting applied or operational risk/impact assessments. One barrier to reuse of vulnerability models is the format they are often made available, which is mostly in academic journal articles. For example, it is difficult to identify relevant curve parameters from figures in journal articles alone. Consistently applied principles when reporting the methods and results of vulnerability models would help reduce this barrier (e.g., fitting method and number of data points). Adopting open data principles has also been suggested as one way that can help overcome barriers for re-use of hazard models and data in volcanology (Tierz, 2020). Likewise, “FAIR” (findability, accessibility, interoperability, and reusability) (Wilkinson et al., 2016; Stall et al., 2019) data principles will possibly be crucial to further development and innovation in this field. With the widespread use of code sharing, collaboration, and version control services many of the practical barriers for model and code sharing are rapidly decreasing. Although, we acknowledge that several important issues remain related to robustness of open data, documentation and metadata standards, legal considerations (e.g., intellectual property and privacy policies) and data security that need to be considered.
The selection of appropriate vulnerability models for use in different contexts (e.g., geographical or climatological) is an important consideration for robust risk assessments. Documentation of rationale behind the selection of any given vulnerability model is an important best practice. Comprehensive guidance on selecting vulnerability models has been developed for the Global Earthquake Model (Rossetto et al., 2014). As vulnerability model development for volcanic eruptions continues it may become useful to consider a similar approach.
3.3 Making better use of existing data to create future vulnerability models
There are a relatively limited number of empirical datasets used to develop vulnerability models. Volcanic impacts databases would allow the community to make better use of pre-existing data as well as help streamline workflows for future vulnerability model development. Databases allow for impacts to be classified by a standardized taxonomy that would facilitate greater sharing of data across case studies, which may help enhance vulnerability model development and drive new thinking in this space. Standardized taxonomies have been developed for a number of perils. For example, the Global Assessment of Risk (GAR) 2015 had a focus on standardized taxonomies (Maqsood et al., 2014). Similarly, the Global Earthquake Model (GEM) has developed guidelines for the development of empirical vulnerability models, which includes consideration of the data quality of the underpinning databases (Rossetto et al., 2014). The concept of a volcanic impacts database has been suggested in previous studies (Wilson et al., 2014), and there are many existing databases focused on differing issues in volcanology (see the review by Andrews et al., 2022). There is already the Volcanic Fatalities Database (Brown et al., 2017), and other global databases which include impact information in an ad hoc manner, but comprehensive compilations of volcanic impacts are often restricted to tables within academic papers or reports. This approach suffers from limited updates and case studies are often focused on only the most impactful events. However, sustainable long-term database management remains a key barrier in this space and a solution to this remains elusive (Andrews et al., 2022).
3.4 Taking advantage of new data sources for future vulnerability models
Remote sensing data have been widely used in humanitarian mapping following a variety of disasters, and recently specifically for volcanic eruptions via the Copernicus Emergency Management Service (CEMS) (e.g., Taal 2020: European CommissionJoint Research Centre, (2020); La Palma 2021; European CommissionJoint Research Centre, (2021)). Ground-based images have been used effectively to assess exposure, impact, and hazard intensity previously (e.g., Spence et al., 1997; Hayes et al., 2019; Meredith et al., 2022). Work has also been undertaken to explore how big Earth observation data can be used for assessing volcanic impacts and development of quantitative frameworks for key impact metrics (Biass et al., 2021; Biass et al., 2022). The use of crowd-sourced data for impact assessment is currently receiving increased attention in the hydrometeorological field (Harrison and Johnson, 2016; Sadler et al., 2018; Harrison et al., 2021), and may be a useful approach to consider for gathering volcanic impacts data. Each of these approaches can also assist with obtaining exposure datasets characteristics, which is also important for understanding characterizing the diversity of impacts that can occur at a given hazard intensity (Pittore et al., 2018; Scaini et al., 2022). While these emerging methodologies may not yet provide the same level of detail or robustness as typical ground surveys (Williams et al., 2020), they hold promise in substantially influencing data collection approaches by enabling broader spatial coverage and addressing data gaps more effectively. Therefore, it is reasonable to anticipate that remote-based studies will be an important source of data for vulnerability models in the coming years. However, working with local experts and/or communities will remain an important component for the successful interpretation of these datasets and their implementation within vulnerability models. As such, it will likely be important to consider how remotely obtained data can be integrated with traditional impact data collection approaches to produce next-generation vulnerability models.
4 Conclusion
Understanding risk is the foundation of sustainable development and is listed as priority one of the Sendai Framework for Disaster Risk Reduction (UNISDR, 2015). One component of this is the development of robust risk models that can help facilitate risk-informed decision-making for disaster risk reduction. There have been large strides made in the volcano vulnerability model development space over the last few decades, which has transformed our ability to assess volcanic impact/risk. Fundamental to this has been a clear view of end-use and interdisciplinary collaborations to ensure outputs are useful, usable, and used. It is important that the momentum built in this field continues, particularly in collection of empirical (and modelled) data to inform vulnerability model development.
Author contributions
JH: Data curation, Formal Analysis, Methodology, Visualization, Writing–original draft, Writing–review and editing. RF: Conceptualization, Data curation, Formal Analysis, Methodology, Visualization, Writing–review and editing. TW: Conceptualization, Methodology, Writing–review and editing. AW: Methodology, Writing–original draft, Writing–review and editing. JW: Methodology, Writing–original draft, Writing–review and editing. GL: Conceptualization, Methodology, Writing–review and editing.
Funding
The author(s) declare financial support was received for the research, authorship, and/or publication of this article. This project was supported by the New Zealand Ministry of Business, Innovation and Employment (MBIE) through the Natural Hazard and Risk programme (Strategic Science Investment Fund, contract C05X1702). We also thank to the National Science Challenges via the Resilience to Nature’s Challenges Volcano Programme and the Determining Volcanic Risk in Auckland (DEVORA) Programme. RF also received funding from MBIE Science Whitinga Fellowship 21-MAU-018.
Acknowledgments
We thank Nick Varely for editorial support. We also thank Chiara Scaini, Julia Crummy, and Noa Liggot for providing informative review comments that helped improve the quality of this manuscript. We thank Christina Magill and Vinod Sadashiva for helpful suggestions throughout the development of this manuscript. We also thank Sophia Tsang, Heather Craig, Carol Stewart, Daniel Blake, George Williams and Nicole Allen who reviewed the initial stock take for errors, and provided additional contributions to the stock take.
Conflict of interest
The authors declare that the research was conducted in the absence of any commercial or financial relationships that could be construed as a potential conflict of interest.
Publisher’s note
All claims expressed in this article are solely those of the authors and do not necessarily represent those of their affiliated organizations, or those of the publisher, the editors and the reviewers. Any product that may be evaluated in this article, or claim that may be made by its manufacturer, is not guaranteed or endorsed by the publisher.
Supplementary material
The Supplementary Material for this article can be found online at: https://www.frontiersin.org/articles/10.3389/feart.2023.1278283/full#supplementary-material
References
Andrews, B. J., Costa, F., Venzke, E., and Widiwijayanti, C. (2022). Databases in volcanology. Bull. Volcanol. 84, 92. doi:10.1007/s00445-022-01597-x
Auker, M. R., Sparks, R. S. J., Siebert, L., Crosweller, H. S., and Ewert, J. (2013). A statistical analysis of the global historical volcanic fatalities record. J. Appl. Volcanol. 2, 2. doi:10.1186/2191-5040-2-2
Biass, S., Jenkins, S., Lallemant, D., Ning Lim, T., Williams, G., Yun, S.-H., et al. (2021). “Remote sensing of volcanic impacts,” in Forecasting and planning for volcanic hazards, risks, and disasters (Amsterdam, Netherland: Elsevier), 473–491. doi:10.1016/B978-0-12-818082-2.00012-3
Biass, S., Jenkins, S. F., Aeberhard, W. H., Delmelle, P., and Wilson, T. (2022). Insights into the vulnerability of vegetation to tephra fallouts from interpretable machine learning and big Earth observation data. Nat. Hazards Earth Syst. Sci. 22, 2829–2855. doi:10.5194/nhess-22-2829-2022
Biass, S., Scaini, C., Bonadonna, C., Folch, A., Smith, K., and Höskuldsson, A. (2014). A multi-scale risk assessment for tephra fallout and airborne concentration from multiple Icelandic volcanoes – Part 1: hazard assessment. Nat. Hazards Earth Syst. Sci. 14, 2265–2287. doi:10.5194/nhess-14-2265-2014
Blake, D. M., Deligne, N. I., Wilson, T. M., Lindsay, J. M., and Woods, R. (2017). Investigating the consequences of urban volcanism using a scenario approach II: insights into transportation network damage and functionality. J. Volcanol. Geotherm. Res. 340, 92–116. doi:10.1016/j.jvolgeores.2017.04.010
Blong, R. J. (1984). Volcanic hazards: a sourcebook on the effects of eruptions. Sydney, Australia: Academic Press.
Blong, R. (2003). Building damage in rabaul, Papua New Guinea, 1994. Bull. Volcanol. 65, 43–54. doi:10.1007/s00445-002-0238-x
Blong, R., Tillyard, C., and Attard, G. (2017). “Insurance and a volcanic crisis—a tale of one (big) eruption, two insurers, and innumerable insureds,” in Observing the volcano world, C. J. Fearnley, D. K. Bird, K. Hayneset al. (Cham, Switzerland: Springer International Publishing), 585–599. doi:10.1007/11157_2016_42
Bonadonna, C., Biass, S., Calder, E., Frischknecht, C., Gregg, C., Jenkins, S., et al. (2018). 1st IAVCEI/GVM workshop: “from volcanic hazard to risk assessment.” Geneva, Switzerland: University of Geneva, 27–29.
Bonadonna, C., Frischknecht, C., Menoni, S., Romerio, F., Gregg, C. E., Rosi, M., et al. (2021). Integrating hazard, exposure, vulnerability and resilience for risk and emergency management in a volcanic context: the ADVISE model. J. Appl. Volcanol. 10, 7. doi:10.1186/s13617-021-00108-5
Brown, S. K., Jenkins, S. F., Sparks, R. S. J., Odbert, H., and Auker, M. R. (2017). Volcanic fatalities database: analysis of volcanic threat with distance and victim classification. J. Appl. Volcanol. 6, 15. doi:10.1186/s13617-017-0067-4
Chester, D. K., Degg, M., Duncan, A. M., and Guest, J. E. (2000). The increasing exposure of cities to the effects of volcanic eruptions: a global survey. Environ. Hazards 2, 89–103. doi:10.3763/ehaz.2000.0214
Craig, H. M. (2015). Agricultural vulnerability to tephra fall impacts. Canterbury, UK: University of Canterbury.
Craig, H. M., Wilson, T. M., Magill, C., Stewart, C., and Wild, A. J. (2021). Agriculture and forestry impact assessment for tephra fall hazard: fragility function development and New Zealand scenario application. Volcanica 4, 345–367. doi:10.30909/vol.04.02.345367
Deligne, N. I., Horspool, N., Canessa, S., Matcham, I., Williams, G. T., Wilson, G., et al. (2017). Evaluating the impacts of volcanic eruptions using RiskScape. J. Appl. Volcanol. 6, 18. doi:10.1186/s13617-017-0069-2
Deligne, N. I., Jenkins, S. F., Meredith, E. S., Williams, G. T., Leonard, G. S., Stewart, C., et al. (2022). From anecdotes to quantification: advances in characterizing volcanic eruption impacts on the built environment. Bull. Volcanol. 84, 7. doi:10.1007/s00445-021-01506-8
Deligne, N. I., Jolly, G. E., Taig, T., and Webb, T. H. (2018). Evaluating life-safety risk for fieldwork on active volcanoes: the volcano life risk estimator (VoLREst), a volcano observatory’s decision-support tool. J. Appl. Volcanol. 7, 7. doi:10.1186/s13617-018-0076-y
European Commission, Joint Research Centre (JRC) (2020). Taal Volcano eruption in Philippines (2020-01-13). Brussels Belgium: European Commission, Joint Research Centre.
European Commission, Joint Research Centre (JRC) (2021). Volcano eruption in La Palma, Spain (2021-09-19). Brussels Belgium: European Commission, Joint Research Centre.
Fitzgerald, R. H. (2019). Improving volcanic ballistic hazard assessment through field and laboratory approaches. Cincinnati, OH, USA: University of Cincinnati.
Fitzgerald, R. H., Wilson, T. M., Hayes, J. L., Weir, A. M., Williams, J. H., Leonard, G. S., et al. (2023). A stocktake of global volcanic vulnerability models to inform future volcanic risk research in Aotearoa New Zealand: GNS Science. doi:10.21420/NQ3E-9M78
Harrison, S. E., and Johnson, P. A. (2016). Crowdsourcing the disaster management cycle. Int. J. Inf. Syst. Crisis Response Manag. 8, 17–40. doi:10.4018/IJISCRAM.2016100102
Harrison, S. E., Potter, S. H., Prasanna, R., Doyle, E. E., and Johnston, D. (2021). Where oh where is the data?‘: identifying data sources for hydrometeorological impact forecasts and warnings in Aotearoa New Zealand. Int. J. Disaster Risk Reduct. 66, 102619. doi:10.1016/j.ijdrr.2021.102619
Hayes, J. L., Calderón, B. R., Deligne, N. I., Jenkins, S. F., Leonard, G. S., McSporran, A. M., et al. (2019). Timber-framed building damage from tephra fall and lahar: 2015 Calbuco eruption, Chile. J. Volcanol. Geotherm. Res. 374, 142–159. doi:10.1016/j.jvolgeores.2019.02.017
Hayes, J. L., Wilson, T. M., and Magill, C. (2015). Tephra fall clean-up in urban environments. J. Volcanol. Geotherm. Res. 304, 359–377. doi:10.1016/j.jvolgeores.2015.09.014
Jenkins, S. F., Biass, S., Williams, G. T., Hayes, J. L., Tennant, E. M., Yang, Q., et al. (2022). “Evaluating and ranking Southeast Asia’s exposure to explosive volcanic hazards,” in Natural hazards and Earth system sciences discussion (Göttingen, Germany: Copernicus Publications). doi:10.5194/nhess-2021-320
Jenkins, S. F., Day, S. J., Faria, B. V. E., and Fonseca, JFBD (2017). Damage from lava flows: insights from the 2014–2015 eruption of Fogo, Cape Verde. J. Appl. Volcanol. 6, 6. doi:10.1186/s13617-017-0057-6
Jenkins, S. F., Komorowski, J.-C., Baxter, P. J., Spence, R., Picquout, A., Lavigne, F., et al. (2013). The Merapi 2010 eruption: an interdisciplinary impact assessment methodology for studying pyroclastic density current dynamics. J. Volcanol. Geotherm. Res. 261, 316–329. doi:10.1016/j.jvolgeores.2013.02.012
Jenkins, S. F., Spence, R. J. S., Fonseca, JFBD, Solidum, R., and Wilson, T. (2014). Volcanic risk assessment: quantifying physical vulnerability in the built environment. J. Volcanol. Geotherm. Res. 276, 105–120. doi:10.1016/j.jvolgeores.2014.03.002
Leder, J., Wenzel, F., Daniell, J. E., and Gottschämmer, E. (2017). Loss of residential buildings in the event of a re-awakening of the Laacher See Volcano (Germany). J. Volcanol. Geotherm. Res. 337, 111–123. doi:10.1016/j.jvolgeores.2017.02.019
Ligot, N. (2022), Crop vulnerability to tephra fall in volcanic regions: field, experimental and modelling approaches. Ottignies-Louvain-la-Neuve, Belgium: UCLouvain.
Ligot, N., Bogaert, P., Biasse, S., Lobet, G., and Delmelle, P. (2023). Grain size modulates volcanic ash retention on crop foliage and potential yield loss. Nat. Hazards Earth Syst. Sci. 23 (4), 1355–1369. doi:10.5194/nhess-23-1355-2023
Loughlin, S. C., Vye-Brown, C., Sparks, R. S. J., Brown, S. K., Barclay, J., Calder, E., et al. (2015). “An introduction to global volcanic hazard and risk,” in Global volcanic hazards and risk, S. C. Loughlin, S. Sparks, S. K. Brown, S. F. Jenkins, and C. Vye-Brown, (Cambridge, MA, USA: Cambridge University Press), 1–80. doi:10.1017/CBO9781316276273.003
Magill, C., Blong, R., and McAneney, J. (2006). VolcaNZ—a volcanic loss model for Auckland, New Zealand. J. Volcanol. Geotherm. Res. 149, 329–345. doi:10.1016/j.jvolgeores.2005.09.004
Meredith, E. S., Jenkins, S. F., Hayes, J. L., Deligne, N. I., Lallemant, D., Patrick, M., et al. (2022). Damage assessment for the 2018 lower East Rift Zone lava flows of Kīlauea volcano, Hawai'i. Bull. Volcanol. 84 (7), 65. doi:10.1007/s00445-022-01568-2
Meredith, E. S., Jenkins, S. F., Hayes, J. L., Lallemant, D., Deligne, N. I., Teng, N. R. X., et al. (2023). “Lava flow impacts on the built environment: insights from a new global dataset,” in Review. doi:10.21203/rs.3.rs-2621512/v1
Mossoux, S., Kervyn, M., and Canters, F. (2019). Assessing the impact of road segment obstruction on accessibility of critical services in case of a hazard. Nat. Hazards Earth Syst. Sci. 19, 1251–1263. doi:10.5194/nhess-19-1251-2019
Newhall, C. G. (1982). A method for estimating intermediate and long-term risks from volcanic activity, with an example from Mount St. Helens. Washington, DC, USA: United States Geological Survey.
Pittore, M., Haas, M., and Megalooikonomou, K. G. (2018). Risk-oriented, bottom-up modeling of building portfolios with faceted taxonomies. Front. Built Environ. 4, 41. doi:10.3389/fbuil.2018.00041
Pomonis, A., Spence, R., and Baxter, P. (1999). Risk assessment of residential buildings for an eruption of furnas volcano, são miguel, the azores. J. Volcanol. Geotherm. Res. 92, 107–131. doi:10.1016/S0377-0273(99)00071-2
Rossetto, T., D’Ayala, D., Ioannou, I., and Meslem, A. (2014). “Evaluation of existing fragility curves,” in SYNER-G: typology definition and fragility functions for physical elements at seismic risk. Editors K. Pitilakis, H. Crowley, and A. M. Kaynia (Dordrecht, Netherlands: Springer Netherlands), 47–93.
Sadler, J. M., Goodall, J. L., Morsy, M. M., and Spencer, K. (2018). Modeling urban coastal flood severity from crowd-sourced flood reports using Poisson regression and Random Forest. J. Hydrology 559, 43–55. doi:10.1016/j.jhydrol.2018.01.044
Santos, J., Roquel, KIDZ, Lamberte, A., Tan, R. R., Aviso, K. B., Tapia, J. F. D., et al. (2023). Assessing the economic ripple effects of critical infrastructure failures using the dynamic inoperability input-output model: a case study of the Taal Volcano eruption. Sustain. Resilient Infrastructure 8, 68–84. doi:10.1080/23789689.2022.2127999
Scaini, C., Biass, S., Galderisi, A., Bonadonna, C., Folch, A., Smith, K., et al. (2014). A multi-scale risk assessment for tephra fallout and airborne concentration from multiple Icelandic volcanoes – Part 2: vulnerability and impact. Nat. Hazards Earth Syst. Sci. 14, 2289–2312. doi:10.5194/nhess-14-2289-2014
Scaini, C., Peresan, A., Tamaro, A., Poggi, V., and Barnaba, C. (2022). Can high-school students contribute to seismic risk mitigation? Lessons learned from the development of a crowd-sourced exposure database. Int. J. Disaster Risk Reduct. 69, 102755. doi:10.1016/j.ijdrr.2021.102755
Spence, R. J. S., Kelman, I., Baxter, P. J., Zuccaro, G., and Petrazzuoli, S. (2005). Residential building and occupant vulnerability to tephra fall. Nat. Hazards Earth Syst. Sci. 5, 477–494. doi:10.5194/nhess-5-477-2005
Stall, S., Yarmey, L., Cutcher-Gershenfeld, J., Hanson, B., Lehnert, K., Nosek, B., et al. (2019). Make scientific data FAIR. Nature 570, 27–29. doi:10.1038/d41586-019-01720-7
Tierz, P. (2020). Long-term probabilistic volcanic hazard assessment using open and non-open data: observations and current issues. Front. Earth Sci. 8, 257. doi:10.3389/feart.2020.00257
United Nations Office for Disaster Risk Reduction (2015). Sendai framework for disaster risk reduction 2015–2030. Geneva, Switzerland: United Nations Office for Disaster Risk Reduction.
Valentine, G. A. (1998). Damage to structures by pyroclastic flows and surges, inferred from nuclear weapons effects. J. Volcanol. Geotherm. Res. 87, 117–140. doi:10.1016/S0377-0273(98)00094-8
Wilkinson, M. D., Dumontier, M., Aalbersberg, I. J., Appleton, G., Axton, M., Baak, A., et al. (2016). The FAIR Guiding Principles for scientific data management and stewardship. Sci. Data 3, 160018. doi:10.1038/sdata.2016.18
Williams, G. T., Jenkins, S. F., Biass, S., Wibowo, H. E., and Harijoko, A. (2020). Remotely assessing tephra fall building damage and vulnerability: kelud Volcano, Indonesia. J. Appl. Volcanol. 9, 10. doi:10.1186/s13617-020-00100-5
Williams, G. T., Kennedy, B. M., Lallemant, D., Wilson, T. M., Allen, N., Scott, A., et al. (2019). Tephra cushioning of ballistic impacts: quantifying building vulnerability through pneumatic cannon experiments and multiple fragility curve fitting approaches. J. Volcanol. Geotherm. Res. 388, 106711. doi:10.1016/j.jvolgeores.2019.106711
Williams, G. T., Kennedy, B. M., Wilson, T. M., Fitzgerald, R., Tsunematsu, K., and Teissier, A. (2017). Buildings vs. ballistics: quantifying the vulnerability of buildings to volcanic ballistic impacts using field studies and pneumatic cannon experiments. J. Volcanol. Geotherm. Res. 343, 171–180. doi:10.1016/j.jvolgeores.2017.06.026
Wilson, G., Wilson, T. M., Deligne, N. I., Blake, D. M., and Cole, J. W. (2017) Framework for developing volcanic fragility and vulnerability functions for critical infrastructure. J. Appl. Volcanol. 6:14–24. doi:10.1186/s13617-017-0065-6
Wilson, G., Wilson, T. M., Deligne, N. I., and Cole, J. W. (2014). Volcanic hazard impacts to critical infrastructure: a review. J. Volcanol. Geotherm. Res. 286, 148–182. doi:10.1016/j.jvolgeores.2014.08.030
Wilson, T., and Kaye, G. (2007). Agricultural fragility estimates for volcanic ash fall hazards. Lower Hutt, New Zealand: GNS Science.
Wilson, T. M., Stewart, C., Sword-Daniels, V., Leonard, G. S., Johnston, D. M., Cole, J. W., et al. (2012). Volcanic ash impacts on critical infrastructure. Phys. Chem. Earth 46, 5–23. doi:10.1016/j.pce.2011.06.006
Zuccaro, G., Cacace, F., Spence, R. J. S., and Baxter, P. J. (2008). Impact of explosive eruption scenarios at Vesuvius. J. Volcanol. Geotherm. Res. 178, 416–453. doi:10.1016/j.jvolgeores.2008.01.005
Keywords: volcanic risk, fragility, vulnerability, volcanic hazard, eruption, disaster, resilience
Citation: Hayes JL, Fitzgerald RH, Wilson TM, Weir A, Williams J and Leonard G (2024) Linking hazard intensity to impact severity: mini review of vulnerability models for volcanic impact and risk assessment. Front. Earth Sci. 11:1278283. doi: 10.3389/feart.2023.1278283
Received: 16 August 2023; Accepted: 29 December 2023;
Published: 12 January 2024.
Edited by:
Nick Varley, University of Colima, MexicoReviewed by:
Chiara Scaini, National Institute of Oceanography and Applied Geophysics, ItalyJulia Crummy, The Lyell Centre, United Kingdom
Noa Ligot, Université Catholique de Louvain, Belgium
Copyright © 2024 Hayes, Fitzgerald, Wilson, Weir, Williams and Leonard. This is an open-access article distributed under the terms of the Creative Commons Attribution License (CC BY). The use, distribution or reproduction in other forums is permitted, provided the original author(s) and the copyright owner(s) are credited and that the original publication in this journal is cited, in accordance with accepted academic practice. No use, distribution or reproduction is permitted which does not comply with these terms.
*Correspondence: Josh L. Hayes, ai5oYXllc0BnbnMuY3JpLm56
†These authors have contributed equally to this work and share first authorship