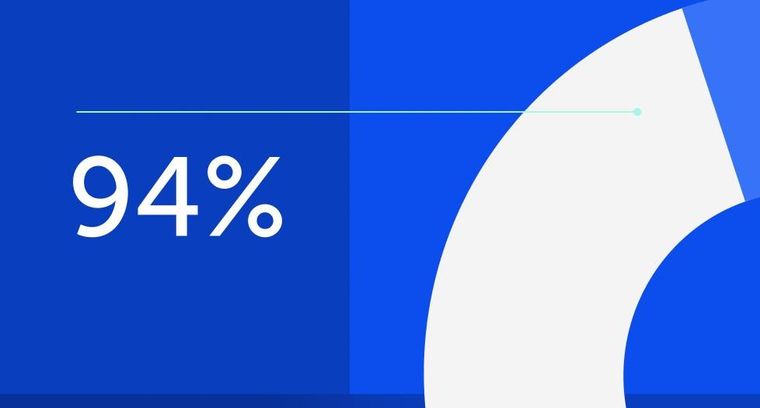
94% of researchers rate our articles as excellent or good
Learn more about the work of our research integrity team to safeguard the quality of each article we publish.
Find out more
ORIGINAL RESEARCH article
Front. Earth Sci., 13 December 2023
Sec. Cryospheric Sciences
Volume 11 - 2023 | https://doi.org/10.3389/feart.2023.1277027
This article is part of the Research TopicNatural Methane Emissions in a Changing Arctic - Implications for Climate and EnvironmentView all 16 articles
Permafrost is widespread in the High Arctic, including the Norwegian archipelago of Svalbard. The uppermost permafrost intervals have been well studied, but the processes at its base and the impacts of the underlying geology have been largely overlooked. More than a century of coal, hydrocarbon, and scientific drilling through the permafrost in Svalbard shows that accumulations of natural gas trapped at the base of permafrost are common. These accumulations exist in several stratigraphic intervals throughout Svalbard and show both thermogenic and biogenic origins. The gas, combined with the relatively young permafrost age, is evidence of ongoing gas migration throughout Svalbard. The accumulation sizes are uncertain, but one case demonstrably produced several million cubic metres of gas over 8 years. Heavier gas encountered in two boreholes on Hopen may be situated in the gas hydrate stability zone. While permafrost is demonstrably ice-saturated and acting as seal to gas in lowland areas, in the highlands permafrost is more complex and often dry and permeable. Svalbard shares a similar geological and glacial history with much of the Circum-Arctic, suggesting that sub-permafrost gas accumulations are regionally common. With permafrost thawing in the Arctic, there is a risk that the impacts of releasing of methane trapped beneath permafrost will lead to positive climatic feedback effects.
Thawing permafrost results in the release of methane gas to the atmosphere (Knoblauch et al., 2018). Methane is a potent greenhouse gas and its release from permafrost acts as a positive climatic feedback loop (Lashof and Ahuja, 1990; Boucher et al., 2009; Howarth et al., 2011). The Arctic is particularly sensitive to climatic changes due to the polar amplification effect and Svalbard is one of the most rapidly warming places today (Aagaard et al., 1987; Divine and Dick, 2006; Van Pelt et al., 2016). Svalbard is, therefore, a critical site for studying the evolution of permafrost and sub-permafrost processes (Isaksen et al., 2000; Christiansen et al., 2010; Hodson et al., 2019; Hornum et al., 2020).
While methane emissions from thawing of the permafrost’s uppermost surface, i.e., the active layer, is relatively well understood (Vonk and Gustafsson, 2013; Knoblauch et al., 2018), the prevalence and volumes of gas accumulations trapped beneath the permafrost, i.e., the “cryospheric cap” (Anthony et al., 2012), have been much less studied. Here we present evidence of such gas accumulations in Svalbard, where the relatively young (e.g., 2–4 kyr in Adventdalen) permafrost (Gilbert et al., 2018) appears to be sealing significant gas accumulations. The gas here may originate from biogenic or thermogenic processes (Hodson et al., 2019; Ohm et al., 2019) and may be in free-gas form or, under the right compositional and thermobaric conditions, in the form of natural gas hydrates (Sloan et al., 2007; Betlem et al., 2019).
Occurrences of gas originating from within or below intervals of permafrost are typically identified in studies on natural gas hydrates and have been documented in both the Russian (Chuvilin et al., 2000; Yakushev and Chuvilin, 2000; Skorobogatov et al., 2007; Makogon and Omelchenko, 2013; Chuvilin et al., 2020) and North American Arctic (Bily and Dick, 1974; Kamath et al., 1987; Majorowicz and Hannigan, 2000; Collett et al., 2011; Nielsen et al., 2014).
Permafrost is defined as ground that remains at <0°C for more than two consecutive years, regardless of fluid content. Physically speaking, ice-saturated permafrost possesses extremely good sealing properties (Keating et al., 2018). However, its effectiveness it is as a long-lived and laterally continuous seal is uncertain. In Svalbard this is reflected by methane emissions at pingos (Hodson et al., 2019) where permafrost demonstrates its local sealing ability but also the prevalence of migration pathways through it. Abrupt changes in hydrogeological flow conditions at the base of the permafrost also indicate the permeability-reducing nature of the permafrost interval (Hornum et al., 2020). In geological terms, permafrost is very short-lived which, in addition to being very shallow and potentially patchy, would typically preclude it from being regarded as a feasible seal for conventional hydrocarbon accumulations at geological timescales of millions of years.
In Svalbard, methane migrating through near-coastal pingos shows characteristics of a biogenic origin (Hodson et al., 2019). Approximately 3 km inland, analysis of gas encountered at the base of permafrost during research drilling indicated a further contribution from thermogenic origins (Ohm et al., 2019). Several hydrocarbon source rocks are encountered in Svalbard, so traces of thermogenic gas are not particularly surprising. What is surprising, and the focus of this study, is the widespread occurrence, both spatial and stratigraphic, of gas accumulations at the base of permafrost in Svalbard.
In this contribution, we provide previously unpublished data from 41 boreholes to systematically review the occurrence of sub-permafrost gas accumulations from Svalbard. We also analyse data from these boreholes to attempt to characterise the permafrost, its thickness and sealing properties.
The Svalbard archipelago is situated in the High Arctic between 74° and 81°N and 15° to 35°E with sub-zero average air temperatures for 8 months of the year. Permafrost in Svalbard is typically thinner than the other pan-Arctic landmasses at the same latitude due to the warming effects of the West Spitsbergen Currentand the effects of glaciers and their erosion (Humlum, 2005).
Permafrost in Svalbard ranges in thickness from more than 500 m in mountainous areas inland to less than 100 m near the coastlines (Humlum, 2005). Continuous sub-sea permafrost is absent offshore Spitsbergen’s west coast (Christiansen et al., 2005), and is not believed to be present offshore elsewhere around Svalbard (Landvik et al., 1988; Humlum et al., 2003), although these areas have been little studied in this respect and may feature locally discontinuous permafrost. Because of the West Spitsbergen Current, air temperatures are several degrees warmer in the west than they are in the east (Przybylak et al., 2014). Although poorly studied in eastern parts, one can reasonably anticipate thicker permafrost due to colder temperatures, as is also shown by numerical modelling of the permafrost-associated gas hydrate stability zone (Betlem et al., 2019). However, thicker insulating snow coverage in coastal settings can also help in preventing winter heat loss from the ground and limit permafrost growth (Humlum et al., 2003). In a more local context, permafrost in the major valley of Adventdalen has been relatively well studied and comprises a thin permafrost interval of 20–30 m (though containing liquid water due to salinity) on the coast, that rapidly thickens to approximately 150 m thick 3 km inland at the Longyearbyen CO2 site, and approximately 220 m thick in the valley at Janssonhaugen, some 15 km from the coast (Figure 1; Isaksen et al., 2000; Harada and Yoshikawa, 1996; Gilbert et al., 2018).
FIGURE 1. Geological map of Svalbard with boreholes and areas of interest investigated in this study. Geological data is courtesy of Norwegian Polar Institute (Dallmann et al., 2015). The locations of detailed maps shown in later figures are highlighted.
Permafrost evolution in Svalbard is largely driven by glacial settings rather than temperature changes. During the Weichselian glacial stage (115 kya to 11.7 kya) Svalbard was covered by thick glacial ice, although the extent and thickness of this ice cover is still debated (e.g., Gataullin et al., 2001; Lambeck, 1996: Winsborrow et al., 2010). Glacial striations in several locations suggest that these glaciers were warm-based for at least parts of the Weichselian glaciation (Humlum et al., 2003; Humlum, 2005). The frictional heat generated from the sliding of warm-based glaciers likely thawed permafrost in major valleys (Humlum et al., 2003). Sedimentological and cryostratigraphic analysis of boreholes in Adventdalen support this (Gilbert et al., 2018), suggesting that the permafrost in Adventdalen formed in the past few thousand years following the dynamic retreat of these warm-based glaciers and ice streams. Whether permafrost survived the Weichselian glaciations is dependent on the persistence of ice-free zones and/or cold based glaciers. Because of this, permafrost in highland areas was more likely to have survived, possibly for several hundred thousand years, through multiple glacial events (Humlum et al., 2003). There is also strong evidence of lowland areas in north-western Svalbard being ice-free at this time which may have enabled the persistence of much older permafrost (Landvik et al., 2003). Valley settings are pertinent to this study as the majority of wellbores have been drilled in valleys or near to the coast for logistical reasons.
Permafrost often poses a challenge to geologists, particularly during drilling (Vrielink et al., 2010), and in the acquisition and processing of seismic data (Johansen et al., 2003; Schmitt et al., 2005). This is because permafrost changes the properties of shallow unlithified sediments to become much more rigid if cemented by ice. Therefore, the permafrost interval in these sedimentary rocks has much faster seismic velocities and can lose mechanical competence as it is drilled through with heated or saline fluids. The near-surface rocks in Svalbard are typically well cemented due to mechanical and chemical compact and very rigid due to deep burial and subsequent uplift (Henriksen et al., 2011).
In a tectonic context, Svalbard represents the exposed north-western part of the Barents Shelf. With the exception of the late Cretaceous and parts of the Neogene, Svalbard exhibits a nearly continuous stratigraphic record from the Devonian to present (Steel and Worsley, 1984; Olaussen et al., 2022). Figure 1 shows the distribution and ages of outcrops in Svalbard and the key wellbore sites for this study. Palaeozoic events from the Caledonian (Gasser, 2014) and Ellesmerian-Svalbardian (Piepjohn, 2000) orogenies are predominantly recorded in the northern parts of Svalbard. From the late Carboniferous to Permian, mixed shallow marine rocks were deposited in localised half-grabens (Bælum and Braathen, 2012; Smyrak-Sikora et al., 2019). From the Triassic to early Cretaceous, clastic deposition occurred in regional-scale basins (Steel and Worsley, 1984). The drainage pattern changed from the west in the early Triassic to the east from the middle Triassic. During this time Svalbard was situated on the northwestern periphery of the largest recorded delta system in Earth’s history (Mørk, 2013; Anell et al., 2014; Klausen et al., 2019). The latest Triassic to middle Jurassic saw much less sedimentation with numerous hiatuses and changes in drainage patterns (Olaussen et al., 2018; Rismyhr et al., 2019). The late Jurassic to early Cretaceous saw greater deposition, including regionally important source rock intervals, and change in drainage due to the opening of the Amerasian Basin (Dypvik and Zakharov, 2012; Koevoets et al., 2018). During the early Cretaceous the development of the High Arctic Large Igneous Province is evident from predominantly mafic dykes and sills in Svalbard (Senger et al., 2014). This likely resulted in major erosion during the late Cretaceous and early Palaeocene (Jochmann et al., 2019).
In Svalbard and the rest of the Barents Shelf, the Cenozoic geological history is the most important to understand subsurface fluid flow. Maximum burial of the rocks exposed in Svalbard occurred during the Eocene (Dörr et al., 2018) whereas the stratigraphy of the Barents Sea experienced maximum burial and hydrocarbon generation during the late Oligocene to early Miocene (Faleide et al., 1996; Henriksen et al., 2011). From the Eocene to present, major regional events resulted in the cumulative uplift of 1–3 km and is ongoing at present. The Svalbard region experienced the greatest uplift magnitudes, resulting in it being subaerially exposed as an archipelago today (Dimakis et al., 1998; Lasabuda et al., 2018). For this study, the most pertinent geological events are the widespread uplift and erosion due to repeated glacial-interglacial cycles during the past few million years (Dimakis et al., 1998; Landvik et al., 1998). These recent events are still ongoing (Kierulf et al., 2022) and are the most important with respect to the migration and leakage of hydrocarbons from deeper traps to the shallow subsurface (Ohm et al., 2008; Abay et al., 2017).
The prevalence of hydrocarbon shows and gas influxes throughout the stratigraphy can be attributed to the presence of multiple mature source rocks (Figure 2; Ohm et al., 2008). The marine shales of the late Jurassic-early Cretaceous Agardhfjellet Formation and the mid-Triassic Botneheia Formation are both regionally extensive and prolific source rocks, responsible for charging most of the oil and gas discoveries in the Norwegian Barents Sea. In addition, Permian organic-rich shales in the Gipsdalen Group (Braathen et al., 2012) probably represent laterally restricted source rocks. Carboniferous, Cretaceous and Paleogene coal seams have been exploited in Svalbard’s past, with the latter still under production in the Central Tertiary Basin in Adventdalen and Barentsburg. These coal seams are widespread and typically form gas-prone and oil-prone source rocks (Marshall et al., 2015; Uguna et al., 2017).
FIGURE 2. The hydrocarbon exploration wells of Svalbard and key coal and scientific boreholes showing the stratigraphy they penetrated, modified from Senger et al. (2019). The base-permafrost, gas-bearing stratigraphy is shown in the right hand column.
Numerous sandstones and karstified carbonates provide potential reservoirs throughout Svalbard’s stratigraphy (Figure 2), many of which are direct analogues to proven hydrocarbon reservoirs in the Barents Sea (Nøttvedt et al., 1993; Olaussen et al., 2022). For this study the most notable reservoirs are the shallow marine sandstone-dominated Lower Cretaceous Helvetiafjellet and Carolinefjellet formations (Steel et al., 1981; Grundvåg et al., 2019), and the Triassic-Jurassic Kapp Toscana Group deltaic to shoreline deposited siltstone and sandstones (Mørk, 1999; Rismyhr et al., 2019).
The above-mentioned source rocks are also the best candidates for sealing intervals due to their extremely low permeability and high ductility due to high organic content. In addition, the mudstones of the late Palaeocene Basilika Formation and Palaeocene-Eocene Frysjaodden Formation also possess potential sealing properties (Steel et al., 1981). The numerous source rocks, technical oil and gas discoveries, bitumen-stained strata and surface seeps suggest that Svalbard possesses working petroleum systems (Olaussen et al., 2022 and references therein). Nonetheless, none of the eighteen exploration wells drilled onshore Svalbard from 1961 to 1994 resulted in commercially viable discoveries (Senger et al., 2019). Although hydrocarbon accumulations likely first formed tens of millions of years ago when source rocks were at maximum burial (Magoon and Dow, 2000), subsequent tectonic events have undoubtedly caused tertiary fluid migration (Ohm et al., 2008; Abay et al., 2017).
Repeated glaciation and deglaciation of the Barents region through the Quaternary has caused tilting and hydrocarbon spillage and remigration from existing traps (Lasabuda et al., 2018 and references therein: Lerche et al., 1997).Gas, particularly methane, is the dominant hydrocarbon found in Svalbard due to the prevalence of over-mature or gas-prone source rocks (Michelsen and Khorasani, 1991; Ohm et al., 2019; Senger et al., 2019) and active methanogenesis (Hodson et al., 2019). Deglaciation and uplift has reduced confining pressure on subsurface fluids and led to gas exsolution and expansion. Therefore, the subsurface fluid systems in Svalbard are in a state of disequilibrium and widespread hydrocarbon migration is likely ongoing at present (Abay et al., 2017). Evidence of this is manifested as out-of-equilibrium pore pressures (Birchall et al., 2020) and the previously mentioned surface seeps across Spitsbergen.
In Svalbard, the timing of hydrocarbon migration and permafrost formation overlap, meaning there is potential for accumulations to develop beneath the impermeable permafrost. Although numerous hydrocarbon and coal exploration wellbores penetrate the entire permafrost interval, it has rarely been of interest to the operators. However, on detailed inspection of well data, reports, and anecdotal evidence, it is clear that sub-permafrost gas accumulations have been frequently encountered throughout the archipelago (Figure 2). In Adventdalen, sub-permafrost free-gas was first documented in 1967 during coal exploration and encountered again in 1979 (SNSK, 1981). This accumulation was further confirmed and sampled during scientific drilling of the Longyearbyen CO2 Lab between 2008 and 2012 (Huq et al., 2017; Ohm et al., 2019).
Several decades of coal and petroleum exploration as well as research drilling in Svalbard has led to much anecdotal evidence of gas accumulations beneath permafrost. We have attempted to verify this by analysing data from boreholes that have penetrated through the permafrost in Svalbard. These boreholes include eighteen hydrocarbon exploration wells, approximately five hundred coal exploration bores, and ten scientific boreholes, eight of which are from the Longyearbyen CO2 Lab (Olaussen et al., 2019). Coal exploration drilling data are integral to this study and come from some five hundred coal exploration boreholes that were drilled over the course of nearly a century by Store Norske Spitsbergen Kullkompani (SNSK, 1979), although are more fragmentary and proprietary. We identify where gas accumulations occur and where these coincide with the base of permafrost, or the first permeable interval below it. The available well data used in this study are presented in Table 1. Despite good spatial and stratigraphic wellbore coverage in Svalbard (Figures 1, 2), there are still some significant challenges in identifying the presence of sub-permafrost gas accumulations, most of which relate to identifying permafrost and its base in the available data, and eliminating other potential causes of gas influxes.
TABLE 1. Data availability and intervals recorded for the permafrost penetrating boreholes. BHT = bottom hole temperature. For petrophysical data, the start of data refers to the shallowest extent of each well log, with coverage extending from that point to the bottom of the wellbore.
The most obvious evidence of permafrost comes from reliable downhole temperature measurements. However, in industrial boreholes, downhole temperature data are typically obtained during wireline logging and are highly unreliable. This is due to wellbores rarely being allowed to reach thermal equilibrium with the surrounding formations following drilling and fluid circulation. Heating drilling mud was also required to be circulated in these wellbores to prevent them from freezing, making direct temperature measurements of the formations impossible. Therefore, accurate absolute temperature measurements are rare, though temperature trends can be used more qualitatively to estimate the approximate base permafrost position. Wells monitored over longer time periods, such as the scientific boreholes in Adventdalen and at Sysselmannbreen (Juliussen et al., 2010; Olaussen et al., 2019) are relatively rare, but provide much more reliable and precise temperature data.
The uppermost active layer of permafrost (down to ca. 2 m) is well-studied in Svalbard (Rachlewicz and Szczuciński, 2008; Westermann et al., 2010; Strand et al., 2021). Conversely, the base permafrost is rarely the focus of academic study due to its inaccessible nature and, thus, the overwhelming majority of data come from industrial boreholes. The base permafrost has been of little industrial interest resulting in limited data acquisition over the permafrost interval. Downhole logging data over the interval typically comprises only of gamma ray data. Additionally, the very low porosity of Svalbard’s highly compacted rocks (Henriksen et al., 2011) means that the effect of any pore fluid change such as the contrast of ice and water at the base of permafrost, is impossible to identify in petrophysical data alone. However, drilling data, such as fluid influxes into the wellbore do provide reliable evidence as to where impermeable permafrost ice is absent. Table 2 shows the ideal responses of petrophysical and drilling data at the base permafrost boundary and the challenges posed to each method in Svalbard. Further uncertainties as to the precise location of the base of permafrost arise from the fact that the drilling fluid used in the wellbores is typically heated and hypersaline. The final challenge is that the characteristics of the base permafrost boundary is poorly understood. Factors such as water presence, chemistry, heterogenous geology, thermal conductivity and associated dynamic processes make predicting the base permafrost properties challenging It is a reasonable assumption that it is likely a diffuse boundary which adds further uncertainty in identifying it. Nevertheless, the combination of drilling data for identifying formation fluids (e.g., fluid influxes into the wellbore) and petrophysical data for lithological interpretation (e.g., sandstone vs. shale) can indicate where the transition from permafrost ice to liquid water occurs. Fluid influxes with no discernible change in lithology or other trapping features (e.g., faulting) can be found in porous and permeable strata in Adventdalen, Hopen, Tromsøbreen, Gipsdalen, and Petuniabukta (Figure 1). Other indicators that can help identify the position of permafrost include the formation of ice within the wellbore (often referred to as ice-plugging), sudden changes in the character or quantity of drill cuttings, and increases in background gas measurements. The presence of overpressures at the base of permafrost is commonly reported in drilling reports, which, in the absence of lithological boundaries, is strong evidence that permafrost is acting as an impermeable seal.
TABLE 2. Petrophysical and drilling parameters that may identify permafrost and its base. Idealised responses are shown and typically identify the transition from ice to water. The final column shows the complicating factors, all of which are applicable to Svalbard. Perhaps the most pertinent complication to Svalbard is that the geology is comprised of well cemented, compacted, hard rocks.
Similarly, the presence of trapped gas at the base of permafrost (Figure 2) is also testament to its sealing potential. Identifying the presence of gas is relatively simple with evidence in the form of gas influxes into the wellbore. In some cases, such as Adventdalen, Tromsøbreen and Hopen, shallow gas from the base of the permafrost was collected for hydrocarbon analysis. Elevated free gas levels (but not gas dissolved in fluids) recorded in the returning drilling fluid is another good indicator of gas entering the wellbore from the formation, and is measured in drilling fluids returning to the surface and extracted by a gas trap. A variety of factors, including drilling rate, drilling mud type and temperature; lower temperatures can result in lower gas influx detection rates, also due to greater levels of condensation and viscosity (Marum et al., 2019).
In addition to well reports and wireline log data, we also include data from published studies including isotope (Huq et al., 2017), thermobaric (Isaksen et al., 2000; Betlem et al., 2018; Betlem et al., 2019), geophysical (Johansen et al., 2003; Beka et al., 2017) and geochemical (Leythaeuser et al., 1984; Ohm et al., 2008) analyses. We also analysed Russian published literature for areas operated by Trust Arktikugol (Lyutkevich, 1937; Verba, 2013).
For Adventdalen, Tromsøbreen and Hopen (with the latter two found in the appendices) we performed simple modelling to estimate the permafrost thickness in addition to the gas hydrate stability zone using the workflow outlined in Betlem et al. (2019) and further refined in Betlem et al. (2021). The modelling implements gas hydrate phase boundary curves relevant to each location, generated through the HWHYD modelling software (Masoudi and Tohidi, 2005). The model utilises spatial mean annual air temperature after Pryzbylak et al. (2014) and a vertical lapse rate of −6°C per km of elevation and geothermal gradient of 33°C per km of increasing depth. The model assumes steady state conditions and a hydrostatic fluid pressure gradient from the land surface.
Integrating all data provides good evidence as to the presence of ice-bearing permafrost in different geographical settings. From the perspective of permafrost as an impermeable seal we have observed differences in three geographical settings: coastal areas, valleys, and highlands. Figure 3 shows the estimated permafrost thicknesses from all relevant wellbores in this study (see Figure 1 for well locations).
FIGURE 3. A plot of the wellbores in this study showing their elevation and the depth to the base of permafrost seal. Solid well path outlines show where data analysed in this study confirms the presence of permafrost while dashed outlines represent where base permafrost has been reported but data is not available. For the Breinosa wellbore, which shows −7.8° at its coldest point at 78 m (and a TD at 90 m) (Juliussen et al., 2010), we extrapolated the base permafrost the local geothermal gradient of 35°/km (Isaksen et al., 2000; Betlem et al., 2018; Senger et al., 2023). Borehole locations are shown in Figure 1.
In coastal areas in the west, evidence is mixed for the presence of ice-bearing permafrost (Figure 1). Hydrocarbon exploration wells on the west coast acquired limited data over shallow parts but provide no evidence of a sealing permafrost interval. However, wellbore temperature data from the western fjord of Isfjorden shows the evidence of a thin permafrost interval (UNIS CO2 Lab AS, 2015; SNSK, 1994). At Kapp Laila, on the southern Isfjorden coast, the permafrost interval is apparently ice-bearing, while around the town of Longyearbyen the permafrost interval is not, likely due to the presence of saline fluids. At Kapp Amsterdam, near the coal mining settlement of Svea, thin sealing permafrost interval (SNSK, 1986). In the east, where air temperatures are cooler (Pryzbylak et al., 2014), observations indicate thicker ice-bearing permafrost intervals.
Data from valleys show the strongest evidence of sealing permafrost throughout Svalbard. Wellbores in valleys (Figure 1). Wellbores in five valleys in Central Spitsbergen and two on Edgeøya demonstrate this and are discussed in more details in later sections. The majority of these cases are based on the occurrence of overpressure or free gas accumulations in reservoirs without an overlying conventional seal.
In colder highland areas the permafrost interval (by temperature definition) is thicker than adjacent valleys. However, different plateau areas appear to possess differing degrees of sealing ice-bound permafrost. In the Adventdalen area and at Ispallen the permafrost zone is permeable as evident by complete drilling fluid losses into the wellbore wile drilling. Converseley at Slaknosa a gas accumulation, without a conventional top seal was encountered.
We have observed that where permafrost can be identified within wellbores, the sealing characteristics can be classed into three types, shown in Figure 4. In much of central Spitsbergen, where the geology is relatively flat-lying and the permafrost interval is often sandstone dominated, the base permafrost can be directly observed to seal by sudden fluid influx into the wellbore despite no apparent downward change in lithology (Figure 3, example 1). Well data can also give evidence that permafrost is acting as a seal laterally away from a wellbore. Figure 4, example 2 may be representative of Edgeøya where the abnormally pressured reservoir was encountered well below the permafrost interval, but because that reservoir outcrops to the east. The latter example of evidence of permafrost sealing is much less certain because of inherent subsurface uncertainties and the transient nature of subsurface fluid flow. Finally, permafrost may be identifiable but is demonstrably not forming a good seal (Figure 4, example 3), which is the case for some highland areas and possibly the coast near Longyearbyen. Table 3 shows these different sealing types for each borehole in this study.
FIGURE 4. Examples of different types of base permafrost. Example 1 is the most commonly encountered and shows where permafrost within a normally permeable interval transforms it into a seal. In this Tromsøbreen example, methane influxed the wellbore at this base permafrost seal in addition to drilling fluid being lost into the formation which is further evidence of the change in permeability. Example 2 is where permafrost ice exists but the base is above the first impermeable interval. The Raddedalen well on the island of Edgeøya is a rare example of where evidence of the base permafrost exists in the petrophysical data, as shown by the acoustic log skipping and resistivity drop. Example 3 is a case where a permafrost interval demonstrably exists by definition (below 0°C) but apparently lacks any seal, in this case by the coast in Adventdalen, the lack of seal is probably due to fluid salinity.
TABLE 3. Wells showing where gas is and is not present at the base of permafrost. Wells without a bullet either contain no permafrost or no relevant data. Base permafrost type: 1) Sealing within reservoir 2) Lateral seal 3) No seal within permafrost (by temperature definition) interval. n/a = no data or no evidence of permafrost.
Table 3 shows occurrences of where gas has and has not been encountered at the base of the permafrost. The wells in Adventdalen, Tromsøbreen, Hopen and Gipsdalen all indicate gas accumulation at the base of permafrost despite being geologically distinct (detailed case studies from Tromsøbreen and Hopen can be found in the additional material). The case in Adventdalen comes from multiple wellbores drilled by different operators for different research and economic reasons and is discussed in more detail in this section. The cases from Reindalen, Kapp Laila and the Plurdalen and Raddedalen wells on Edgeøya all demonstrate good evidence of sealing permafrost but lack trapped gas (detailed case studies of these can be found in the Supplementary Material).
Despite the frequency of gas being encountered at the base of permafrost, it should be noted that none of the wellbores were attempting to find it, which suggests that the phenomenon is extremely common in the archipelago. Permafrost trapped gas appears to show no particular stratigraphic or spatial prevalence. In Adventdalen and at Tromsøbreen the base of permafrost and underlying methane are situated within Cretaceous sandstones. On the island of Hopen in the southeasternmost part of Svalbard, the gas was encountered in the heterolithic sandstone, siltstone and shales of the Triassic De Geerdalen Formation. In Gipsdalen and at Petuniabukta, gas was encountered in Carboniferous or Permian carbonates.
Shallow gas is typically considered a hazard to drilling and this is exemplified by two SNSK wellbores, one on the plateau of Slaknosa on the southern edge of Reindalen (SNSK, 1991), and one at Kapp Amsterdam near Svea (SNSK, 1986). Both wells encountered blowouts that occurred with enough pressure to eject rock and material from the wellbores and lasted for several hours (until the following day in the Kapp Amsterdam case). The Kapp Amsterdam case is particularly intriguing as the gas came from 33.5 m depth at the base of permafrost (thermistors in the wellbore confirmed this) from glacial moraine sediments deposited some 600 years ago (Kristensen et al., 2009), meaning that the gas had accumulated quickly and recently.
There are numerous wellbores that demonstrate the presence of permafrost without an underlying gas accumulation. At Kapp Laila, Colesbukta and Reindalen no free gas was encountered below the Paleogene and Cretaceous situated base-permafrost intervals. The Carboniferous to Permian carbonates of Edgeøya demonstrate the same.
Svalbard’s largest settlement, Longyearbyen, is located in Adventdalen (Figure 5), and the best studied area of Svalbard in the context of this article (Hodson et al., 2020; Hornum et al., 2020; Johansen et al., 2003; Beka et al., 2017; Betlem et al., 2019; Olaussen et al., 2019 and references therein). The high number and density of scientific and industry data sets provide the most comprehensive data on the base of permafrost seal and underlying fluids. The wells of the Longyearbyen CO2 Lab and coal exploration boreholes of SNSK both show the presence of gas trapped beneath the permafrost in Adventdalen. Figure 6 provides a correlation panel of these wellbores.
FIGURE 5. A Geological Map of Adventdalen showing some of the youngest stratigraphy exposed in Svalbard. The profile A to A′ represents the well correlation in Figure 6 B to B′ the modelled permafrost profile in Figure 7.
FIGURE 6. A Well correlation of base permafrost with all available geological, drilling and petrophysical data. The location of the correlation is shown in Figure 5. The wells in this section highlight the somewhat sporadic nature of data availability over the shallow, permafrost bearing intervals.
At the near-coast drillsite-1 of the Longyearbyen CO2 Lab wells temperature data from DH1 (Figure 4 example 3) and DH2 indicate a thin permafrost interval with the base at approximately 20–30 m (Beka et al., 2017). Although sub-zero temperatures were recorded at this site, the presence of ice is strongly dependent on the pore-fluid salinity. At drillsite-2, wellbores DH3 and DH4 encountered overpressured water at the base permafrost. DH4 and DH5R also encountered significant natural gas together with the water influx that was collected in gas bags for sampling (Huq et al., 2017; Ohm et al., 2019). Temperature logs from DH4 suggest base permafrost from 150 to 200 m depth, but the best evidence of a base permafrost seal comes from the sudden influx of water and gas at approximately 150 m. Cores from nearby wells DH6 and DH7A also show elevated methane levels at this depth. The water and gas influxes occur somewhere towards the middle (i.e., not top of the reservoir) of the sandstone dominated Helvetiafjellet Formation.
In the same area, several hundred coal boreholes, drilled by SNSK over the past century, have penetrated the permafrost interval, although data for these is fragmented (see Figure 5 for well locations). Well 1979-11 was drilled approximately 2 km south of Longyearbyen CO2 Lab drillsite-2 in Endalen. This well encountered water influxes with no mention of gas, although no depths are stated in the report (SNSK, 1980; 1981). Well 1979-10, in the valley of Todalen, encountered methane-rich gas overlying inflowing water at the base of permafrost at a depth between 150 and 200 m (SNSK, 1981; 1982b; Leythaeuser et al., 1984). Well 1967-1, approximately 3 km east of, and geologically updip of 1979-10, reached a depth of 106 m where a gas accumulation was encountered (SNSK, 1981). This well was also the subject of considerable interest by SNSK who investigated the potential of producing the gas commercially. Well 1982-20, at the base of Breinosa and the coal mine Gruve-7, did not encounter gas and took water influxes of 33-42 L per minute at approximately 150 m at the base of permafrost (SNSK, 1982a). Another reported well, named only “first water well” (SNSK, 1982a), in the same area flowed from the same interval at 40–50 L per minute. Water from these two wells had a measured chloride concentration of 1,500 ppm (SNSK, 1982a). A well drilled inside Gruve-7 at approximately 380 m above mean sea level (AMSL) encountered liquid water at 154 m depth.
The observed base permafrost in the coal and scientific wellbores show broad agreement with the modelled permafrost, by temperature definition (Figure 7). However, on the plateaus in this area, drilling mud losses into permeable strata in the permafrost interval indicate that a permafrost seal is absent. On Platåberget, the permafrost base is well defined due to its presence in the Gruve-3 coal mine (location in Figure 5); a drilling summary report (SNSK, 1982a) documents two wells drilled at approximately 400 m AMSL total drilling fluid losses at 160–170 m MD. This is within the permafrost interval based on the presence of permafrost in the coal mine. Similarly, on Breinosa, where the Gruve-7 coal mine is situated some 15 km to the east, wells 81-05 and 81-06 both encountered total fluid losses at a similar depth of 170 m (SNSK, 1982a), within the permafrost interval (Juliussen et al., 2010). Similar losses occurred in several intervals between 106 and 196 m in well 19-2011 on Operafjellet, a plateau on the northern side of Adventdalen (SNSK, 2011a; SNSK, 2011b; SNSK, 2011c; SNSK, 2011d), also well within the permafrost interval as freezing was encountered within the wellbore at 132 m depth.
FIGURE 7. Modelled permafrost thickness through Adventdalen with the profile shown in Figure 5. The model parameters are discussed in the methods section but note that the permafrost interval is entirely based on temperature rather than ice thickness or presence. Modelling suggests that gas hydrates are not stable at this location based on a methane composition and hydrostatic pressures, at higher fluid pressures or heavier gas compositions gas hydrates be stable at the base of permafrost here. Modelled mean annual air temperatures are shown for reference in the highland areas and at sea level. Dashed lines represent contours of depth below land surface.
Five pingos are situated along the northern edge of Adventdalen. Four of them provide active migration pathways through the permafrost leading to the discharge of brackish springs and high concentrations of methane (up to and marginally exceeding the solubility limit of 41 mg L-1) (Hodson et al., 2020). At the easternmost pingos, the chloride concentrations and the d13C isotopic composition of both the methane and dissolved CO2 are similar to those described in the wellbore records above.
SNSK commissioned flow analysis work to be carried out on the 1967-1 well in July 1975 and the results of these two test runs are shown in Supplementary Figure S1B. Here it is clear the well responded to pressure drawdown. However, flow rates were still significantly lower than those recorded over the first year. Supplementary Figure S1C shows the pressure build-up when the well was shut in (effectively closed from the atmosphere) between the two test runs. The quick return to pre-drawdown pressures indicates, somewhat unsurprisingly, a good natural pressure support in the well. Ultimately the gas here was deemed by Statoil, and consequently SNSK, to be an uneconomic accumulation locally trapped by permafrost (SNSK, 1981).
Well 1967-1 and 1979-10 also encountered gas, likely the same gas accumulation, while well 1982-20 encountered permafrost over the same stratigraphic interval and well 1979-11 is probably down-dip of the gas-water interface. Intermittent flow from the 1967-1 well was monitored between October 1967 and July 1975 (SNSK, 1981). The first year of this production was continuous and monitored as shown in Supplementary Figure S1C. An initial wellhead gas pressure of 14 bar was recorded (SNSK, 1981) with relatively slow pressure and production decline over time. This indicates that the gas accumulation is in the order of millions of cubic-metres and has well connected pressure support. If the aquifer pressure is known then the length of the methane gas column can be calculated from this pressure. It is clear the aquifer is not at hydrostatic pressure from the surface due to the repeated influxes and water flow from wells 1979-10, 1979-11 and in the CO2 lab research boreholes. Unfortunately, these pressures were not measured.
Gas samples were taken by hydrocarbon exploration wells at Tromsøbreen and Hopen, and from the Longyearbyen CO2 Lab wellbores in Adventdalen (Figure 8). Three samples each were taken at Tromsøbreen and Hopen and their compositions were analysed and are shown in Table 4. A comprehensive analysis of the gas at the Longyearbyen CO2 was carried out by Ohm et al. (2019), Huq et al. (2017), and a rudimentary analysis of the 1967-1 Adventdalen coal exploration gas discovery was carried out by SNSK (1981). These analyses of the gas accumulations and analysis of seeps of the pingo systems in Adventdalen (Hodson et al., 2019) show that these base-permafrost accumulations are methane dominated. The more extensive analysis of the Longyearbyen CO2 Lab gas provides a more complex story throughout the entire stratigraphy with contributions from biogenic and thermogenic sources (Huq et al., 2017; Ohm et al., 2019). Analysis at Tromsøbreen was taken from gas much deeper than that encountered at base permafrost but also suggests the gas is comprised of methane. However, it shows this gas is relatively dry although still likely to be thermogenic due to the sample depth of 768 m and its extraction directly from the Agardhfjellet Formation source rock (Norsk Polar Navigasjon a/S, 1977b; Norsk Polar Navigasjon a/S, 1977a). However, on Hopen the gas, sampled from approximately 150 m depth, is much wetter and clearly of a thermogenic origin. Figure 8 shows the wetness of gas from the three locations: wetter gas means it has a greater component of heavier hydrocarbon molecules such as ethane or propane.
FIGURE 8. Gas wetness from samples taken in wells from Adventdalen (Ohm et al., 2019), Tromsøbreen (Norsk Polar Navigasjon A/S, 1977b) and Hopen (Norske Fina, 1972a). The Hopen gas is much heavier and thus more prone to form hydrates at lower pressures and higher temperatures than methane.
TABLE 4. Geochemical data from samples taken at the hydrocarbon exploration wells at Tromsøbreen-1 and Hopen-1. A comprehensive analysis of gas from the Longyearbyen CO2 Lab Ohm et al. (2019) demonstrates gas at the base of permafrost in Adventdalen is almost entirely methane (>99%).
The composition of the gas is important in understanding its potential phase in the subsurface. Phase diagrams for the gas compositions and thermobaric conditions at Hopen, and at Adventdalen and Tromsøbreen are shown in Supplementary Figure S2. While the dry gas at Tromsøbreen and Adventdalen is unlikely to be in hydrate form at their points of discovery, the gas at Hopen is much wetter. As a consequence, it is more susceptible to be thermodynamically stable as gas hydrates (Betlem et al., 2019).
Theoretically, the permafrost interval should form an extremely effective seal or “cryogenic cap.” If ice-bound it is highly impermeable, often thick, and can self-heal. The story is a little more complex and the permafrost seal-forming process is poorly understood. An effective permafrost seal is demonstrable in various locations in Svalbard by the presence of gas and abnormally pressured water at the base of permafrost. In the most data rich area of Svalbard, Adventdalen, it appears that the permafrost zone is ice-saturated in valleys and forms an effective seal. By contrast, the plateau settings show a permeable permafrost interval. The previously described drilling losses in wells on the plateaus of Platåberget, Breinosa, Operafjellet, Lunckefjellet and Ispallen (SNSK, 2013c; SNSK, 2013a; SNSK, 2013b; SNSK, 2014) occurred in known permafrost intervals (Juliussen et al., 2010). The lack of impermeable permafrost in the highlands is probably simply due to being situated far above the water table (Figure 9). Pressures from the 1967-1 wellbore indicate a hydraulic head between 80 and 100 m above sea level (Table 5) while the base permafrost is considerably higher in the pleateau. Therefore valleys have an ample supply of pore-water in the permafrost interval as it forms downwards in time, while the plateaus do not. Conversely, the gas blowout of the 1990-12 well on Slaknosa plateau, 25 km to the southeast, demonstrates that a permafrost seal is still possible in plateau settings and the story is far from simple. Although data from the Slaknosa blowout is limited to drilling reports (SNSK, 1991), the geological setting of relatively flat and unfaulted sandstones, and their up-dip outcropping in steep cliffs to the north, and high enough gas flow rates into the wellbore at depth to cause a blowout all suggest a permafrost sealed (both vertically and laterally) free gas accumulation in a high permeability reservoir.
FIGURE 9. Schematic cross section of the permafrost interval at Platåberget and Adventdalen showing the potentially contrasting seal effectiveness. Artesian pressures in the valley wellbore 1967-1 suggest an elevated water table (Table 5) which still sits below the base of permafrost in the mountain. The lack of water supply from below during permafrost formation leads to a dry and permeable permafrost interval and subsequent drilling losses. Similar drilling fluid losses appear common in the permafrost interval in several plateau areas in Svalbard. In the valleys the permafrost interval forms through the water table and results in a thick impermeable ice seal. Coal gas was a common hazard in mine 3 and it is plausible that the lack of a permafrost seal is why wellbores on Platåberget did not encounter gas.
TABLE 5. Aquifer pressure calculation from a known virgin wellhead pressure of 14 bar (Supplementary Figure S1A) in well 1967-1 and the possible range of a gas-water contact from wellbore 1971-10 (Figure 6). The low case uses a saline water pressure gradient of 0.10067 bar/m while high case uses freshwater gradient of 0.09795 bar/m.
Gas accumulations beneath the permafrost are common and widespread regardless of stratigraphy (Figures 2, 4; Table 3) which demonstrates the good sealing potential. Abnormal pressures are common at the base of permafrost in several locations in Svalbard which demonstrates the sealing properties of the overlying permafrost. The best data is in Adventdalen where sudden, slightly saline, water influxes occur at the base of permafrost in the Helvetiafjellet Formation. The strong and sustained flow rates indicate appreciable lateral connectivity within the aquifer, indicating an artesian origin of overpressure. The current view of this overpressure is attributed to the formation of permafrost (Hornum et al., 2020) but the high flow rates (Magnabosco et al., 2014), reservoir connectivity and its outcropping beneath the fjord to the west (Blinova et al., 2012) discount this. The effectiveness of a permafrost seal is demonstrated by the high overpressures that have been encountered and it is reasonable to consider that a permafrost seal can withstand significant buoyancy pressures to withhold large gas columns. It seems more likely that the accumulations are regulated laterally by natural pathways through the permafrost at pingos, fjords, or glaciers.
Natural pathways through the cryospheric cap, even in areas of thick permafrost, are present in the form of pingos, springs, warm-based glaciers, and beneath the fjords. At the Reindalen petroleum exploration borehole, elevated gas readings and shows were encountered at 120 m in the wellbore (Supplementary Figure S8) and may represent a migration pathway at the base of permafrost where no trap exists.
For gas to accumulate beneath the permafrost a trap must be present. The undulating base of permafrost can form a trap and seal itself, or it may act as the top seal in combination with the underlying geology, these examples are shown in Figure 10. In valley settings where the base permafrost forms a synclinal structure it is more likely that accumulations are situated within combination traps. This is further supported by the fact the regional and local geology in Svalbard is rarely flat and contains multiple lithological seals and reservoirs. In these traps a combination of structural geology, lithology and permafrost properties contribute to developing hydrocarbon accumulations. This mechanism can be attributed to the gas accumulation in Adventdalen (Figure 11). The combination of this combination trap type and the ice-saturated seals may explain why gas accumulations have been frequently encountered in valleys rather than migrating and accumulating beneath shallower permafrost in highlands. Smaller accumulations, such as the one encountered in Gipsdalen, may be restricted to localised undulations in the base-permafrost.
FIGURE 10. The different trapping mechanisms permafrost can provide. Undulations in the base permafrost alone may form traps, which may be large under mountains if the permafrost seal is effective. Combination traps require permafrost to contribute a lesser sealing surface area and appears to be the mechanism for trapping gas in Adventdalen (Figure 11).
FIGURE 11. The potential combination-trapping style of the Adventdalen gas accumulation based on well observations.
Gas trapped in hydrate form under the right thermobaric conditions is the exception to the previously discussed trapping mechanisms. The gas sampled at Hopen is a strong candidate to originate from hydrates. The heavier gas composition (Table 4) means it has a greater propensity to form hydrates at a given depth and temperature. Though it should be noted many regional hydrate stability zone models assume a hydrostatic fluid pressure from the land surface, while our observations of water-free intervals in highlands suggest this is unlikely to be the case.
In some cases, a permafrost seal is present but lacks an underlying gas accumulation. This can be explained by either a lack of charge or the lack of trap. Given the numerous thermogenic (Abay et al., 2017; Senger et al., 2019; Olaussen et al., 2022) and biogenic (Hodson et al., 2020) hydrocarbon seeps, flares, and sheer prevalence of shallow gas accumulations throughout the sedimentary outcrops of Svalbard, it is unlikely that these result from a lack of charge, with the exception of locations where there are no source rocks (e.g., Edgeøya and northern Svalbard). Where accumulations do not exist beneath sealing permafrost it is highly likely due to the lack of a trapping geometry (e.g., Figure 10).
Gas originating from permafrost is typically attributed to a biogenic origin, primarily because thermogenic gas is generated and migrates on much longer timescales. While biogenic gas is undoubtedly a contributor to sub-permafrost gas in Svalbard (Hodson et al., 2019), thermogenic gas is also clearly a major contributor in several locations (Ohm et al., 2019). Considering this, the lack of any accumulations or significant shows in the wells on Edgeøya is probably due to the lack of any significant source rock.
Approximately 60% of wells in the Barents Shelf offer hydrocarbon shows (Senger et al., 2020), indicating that the basin has at one point in the past been almost saturated with hydrocarbons. The large ultra-shallow discoveries like Wisting, containing relatively non-biodegraded oil, are evidence of more geologically recent hydrocarbon migration. This recent migration is almost certainly driven by major recent uplift over the past thousands to hundreds-of-thousands of years (Henriksen et al., 2011).
Svalbard itself has undergone the greatest uplift of anywhere in the Barents region in the geologically recent past. Recent uplift has enabled gas to escape directly from the source rocks from fracturing and unloading, and from deeper accumulations due to tilting and changes in pressure-volume-temperature (PVT) conditions. The formation of permafrost has effectively added an additional sealing interval for migrating gas before reaching the atmosphere.
Supplementary Figure S11 is a petroleum systems chart with a focus on sub-permafrost accumulations in Svalbard. Clearly, all other elements of the petroleum system must be present prior to migration taking place. Here the timescales are binary with source, reservoirs and lithological seals forming tens or hundreds of millions of years ago. Conversely, permafrost stability in Svalbard is on much shorter timescales and location dependent; permafrost in valleys likely thawed beneath warm-based glaciers, with most permafrost growth occurring over the last 4,000 years, though localised permafrost in high mountains may have survived for as much as 700,000 years (Humlum, 2005; Gilbert et al., 2018). The most critical elements in this petroleum system are undoubtedly the most recent: permafrost seal formation and gas migration.
The geologically recent and severe uplift means that present-day depths of source rocks is not representative of their maturity or gas generating potential. Indeed, uplift has almost certainly driven migration from deeper pre-existing structures (Cavannah et al., 2006; Ohm et al., 2008; Henriksen et al., 2011; Chand et al., 2012) and from the numerous source rocks (Ohm et al., 2018; Birchall et al., 2020). This has been ongoing throughout the Pleistocene and predates permafrost formation. Therefore, the critical moment for most sub-permafrost gas accumulations in Svalbard is the timing of permafrost formation itself. The exception to this is the case of Kapp Amsterdam, where the formation of reservoir, seal, and migration have all occurred within the last 600 years.
Gas migration will occur through permeable intervals, typically at the crest of structures. Faults may aid the movement of gas from deeper structures, particularly during uplift and fault reactivation as appears to be the case at Reindalen which sits on the Billefjorden Fault Zone (Bælum and Braathen, 2012). The discovery of shale gas in Adventdalen (Ohm et al., 2019) also shows that source rocks still internally trap large amounts of gas. This gas will have migrated directly out of source rocks during uplift due to gas expansion and rock fracturing.
While gas accumulations beneath permafrost have been frequently encountered in wellbores, it is important to remember that none of these were actually looking for it, indeed most hydrocarbon exploration wells aim to avoid such shallow gas accumulations (Ronen et al., 2012). In this study, of eighteen hydrocarbon wells in Svalbard, eight show good evidence of permafrost (44%). Four of these permafrost bearing wells show free gas accumulations at the base of permafrost (22% of all wells or 50% of permafrost bearing wells), three clearly show no presence of an accumulation while one contains gas shows. Expanding this to all wells in this study, 18 show evidence of permafrost and 9 of these show evidence of a gas accumulation (50%), though the coal wells for this study were obviously biased to areas of interest. This is an extremely high success rate for something that was not being targeted, and thus highlights the likelihood that these gas accumulations are very common. For reference, the Barents Shelf has one of the highest technical success rates in the world at just below 50% (Norskpetroleum, 2020) for prospects that have been specifically targeted using advanced geological and geophysical methods.
As with conventional hydrocarbon accumulations, the size of sub-permafrost accumulations probably varies significantly. The accumulation in Adventdalen is relatively significant, but also of little economic interest; the 1967-1 well produced in excess of 2.5 million cubic metres of gas between 1967 and 1975 (SNSK, 1981). Despite this, these accumulations may still provide an alternative and cleaner energy source than coal, which is presently used to generate power in Svalbard. Unfortunately, the data are quite poor because the well was also periodically shut in over this time. The use of geophysical methods in assessing accumulations is challenged; high seismic velocities of rigid, compacted rocks results in low acoustic impedance and imaging the base of permafrost or fluid escape features has been impossible in Svalbard. This is highlighted by the mapping of flares and pockmarks in Isfjorden, which show a clear link with underlying faults, but no manifestation in good seismic data (Roy et al., 2015; Rodes et al., 2023).
Because the sub-permafrost accumulations are relatively shallow and under lower pressure, the gas will be much less dense, and thus voluminous, than conventional deeper accumulations. The exception to this is if the gas is in hydrate form where methane concentrations are 160 times higher than in free gas form (Majorowicz and Hannigan, 2000).
Given the sparse data and a sampling bias due to exploration boreholes typically targeting specific intervals or structures, it is difficult to be quantitative with respect to the size and frequency of these accumulations. What is evident is that permafrost is acting as an ultimate seal to these accumulations, that they are numerous, and, based on the only occurrence where flow was recorded, on the orders of million cubic metres.
Based on the occurrences in Svalbard, the prerequisites for sub-permafrost gas to accumulate are, firstly, an impermeable (ice-saturated) permafrost layer, secondly, a source of gas and, finally, gas migration at a time after permafrost formation. Much of the Circum-Arctic shares a similar geological history with Svalbard. A major source of migrating gas in Svalbard is likely from the Mesozoic source rocks (Ohm et al., 2019), which can also be found in the Russian and North American Arctic (Leith et al., 1993; Polyakova, 2015). Recent uplift caused by isostatic rebound has left fluids in the subsurface on the Barents and Svalbard out of pressure equilibrium and driving present-day migration (Birchall et al., 2020). Svalbard shares its Pleistocene glacial history with the Circum-Arctic (Batchelor et al., 2019) so it is not unreasonable to expect sub-permafrost gas accumulations to be regionally widespread. Indeed, gas emanating from zones of permafrost is well-documented onshore and offshore in the Russian Arctic, particularly in hydrocarbon provinces (Chuvilin et al., 2020 and references therein) and as natural gas hydrates (Yakushev and Chuvilin, 2000).
Because methane is a potent greenhouse gas and the Arctic is warming faster than anywhere else on Earth (Lind et al., 2018), the release of sub-permafrost gas accumulations in Svalbard may contribute a positive climatic feedback effect. Strand et al. (2021) show the active layer is thickening at approximately 1.6 cm/year in Adventdalen. Assuming permafrost continues to thaw at this rate then most permafrost trapped gas should remain relatively stable. However, permafrost will not simply thaw from above, but also laterally from the coastlines, in addition to having complex mechanical implications in the strata overlying these accumulations.
Although gas at the base of permafrost has been encountered frequently during more than 50 years of drilling in Svalbard, it has not been systematically studied or widely recognised until now. In this study we have provided a synthesis of historical and modern observations and their implications. Our key findings are:
• Gas accumulations trapped at the base of permafrost occur throughout the archipelago in several stratigraphic intervals.
• The gas accumulations provide evidence for ongoing hydrocarbon migration
• Gas encountered in wellbores on Hopen is compositionally heavier and likely within the gas hydrate stability zone
• Permafrost is a good seal in valleys but appears to possess permeable intervals in highland areas
• Groundwater flow below permafrost is much greater than previously documented
• There is evidence of relatively thick coastal permafrost, particularly in eastern Svalbard
Shallow gas associated with permafrost has been documented throughout much of the Circum-Arctic (Nielsen et al., 2014; Chuvilin et al., 2020; Hodson et al., 2020; Minshull et al., 2020). Given the shared geological and glacial history, it is very likely that the gas accumulations we document in Svalbard are more widespread.
The historical nature of the data and reports means they are available in hard-copy only. Reports referenced in this article are proprietary to their respective companies. Requests to access these datasets should be directed to a2ltc0B1bmlzLm5v. For an overview of historical reports used in this study see: https://zenodo.org/records/5919082.
TB: Conceptualization, Data curation, Investigation, Methodology, Project administration, Visualization, Writing–original draft, Writing–review and editing. MJ: Conceptualization, Data curation, Investigation, Resources, Validation, Writing–review and editing. PB: Methodology, Software, Validation, Writing–review and editing. KS: Conceptualization, Methodology, Supervision, Validation, Writing–review and editing. AH: Supervision, Validation, Writing–review and editing. SO: Supervision, Validation, Writing–review and editing.
The author(s) declare financial support was received for the research, authorship, and/or publication of this article. This research is funded by the Research Centre for Arctic Petroleum Exploration (ARCEx) partners and the Research Council of Norway (grant number: 228107), CLIMAGAS (grant number: 284764) and the Norwegian CCS Research Centre (grant number: 257579). Data mining was supported by the Svalbard Rock Vault project financed through a Svalbard Strategic Grant from the Research Council of Norway (#295781).
We sincerely appreciate access to Store Norske Spitsbergen Kulkompani’s vast internal archives and to the data from the Longyearbyen CO2 lab project (http://co2-ccs.unis.no). We also thank Sarah Strand for fruitful discussions on permafrost dynamics. We are very grateful for the very constructive feedback from two reviewers who have undoubtedly improved the quality of this article.
Author MJ was employed by Store Norske Spitsbergen Kulkompani AS.
The remaining authors declare that the research was conducted in the absence of any commercial or financial relationships that could be construed as a potential conflict of interest.
All claims expressed in this article are solely those of the authors and do not necessarily represent those of their affiliated organizations, or those of the publisher, the editors and the reviewers. Any product that may be evaluated in this article, or claim that may be made by its manufacturer, is not guaranteed or endorsed by the publisher.
The Supplementary Material for this article can be found online at: https://www.frontiersin.org/articles/10.3389/feart.2023.1277027/full#supplementary-material
Aagaard, K., Foldvik, A., and Hillman, S. (1987). The West Spitsbergen Current: disposition and water mass transformation. J. Geophys. Res. Oceans 92, 3778–3784. doi:10.1029/JC092iC04p03778
Abay, T., Karlsen, D., Lerch, B., Olaussen, S., Pedersen, J., and Backer-Owe, K. (2017). Migrated petroleum in outcropping Mesozoic sedimentary rocks in Spitsbergen: organic geochemical characterization and implications for regional exploration. J. Petroleum Geol. 40, 5–36. doi:10.1111/jpg.12662
Anell, I. M., Braathen, A., and Olaussen, S. (2014). The triassic–early jurassic of the northern Barents shelf: a regional understanding of the longyearbyen CO2 reservoir. Nor. Geol. Tidsskr. 94, 83–98.
Anthony, K. M. W., Anthony, P., Grosse, G., and Chanton, J. (2012). Geologic methane seeps along boundaries of Arctic permafrost thaw and melting glaciers. Nat. Geosci. 5, 419–426. doi:10.1038/ngeo1480
Bælum, K., and Braathen, A. (2012). Along-strike changes in fault array and rift basin geometry of the Carboniferous Billefjorden Trough. Svalbard, Nor. Tectonophys. 546, 38–55. doi:10.1016/j.tecto.2012.04.009
Batchelor, C. L., Margold, M., Krapp, M., Murton, D. K., Dalton, A. S., Gibbard, P. L., et al. (2019). The configuration of Northern Hemisphere ice sheets through the Quaternary. Nat. Commun. 10, 3713–3810. doi:10.1038/s41467-019-11601-2
Beka, T. I., Senger, K., Autio, U. A., Smirnov, M., and Birkelund, Y. (2017). Integrated electromagnetic data investigation of a Mesozoic CO2 storage target reservoir-cap-rock succession, Svalbard. J. Appl. Geophys. 136, 417–430. doi:10.1016/j.jappgeo.2016.11.021
Betlem, P., Midttømme, K., Jochmann, M., Senger, K., and Olaussen, S. (2018). “Geothermal gradients on svalbard,” in First EAGE/IGA/DGMK Joint Workshop on Deep Geothermal Energy, Arctic, Norway, November 2018.
Betlem, P., Roy, s., Birchall, T., Hodson, A., Noormets, R., Römer, M., et al. (2021). Modelling of the gas hydrate potential in Svalbard’s fjords. J. Nat. Gas Sci. Eng. 94, 104127. doi:10.1016/j.jngse.2021.104127
Betlem, P., Senger, K., and Hodson, A. (2019). 3D thermobaric modelling of the gas hydrate stability zone onshore central Spitsbergen, Arctic Norway. Mar. Petroleum Geol. 100, 246–262. doi:10.1016/j.marpetgeo.2018.10.050
Bily, C., and Dick, J. (1974). Naturally occurring gas hydrates in the Mackenzie Delta, NWT. Bull. Can. Petroleum Geol. 22, 340–352. doi:10.35767/gscpgbull.22.3.340
Birchall, T., Senger, K., Hornum, M., Olaussen, S., and Braathen, A. (2020). Underpressure in the northern Barents shelf: causes and implications for hydrocarbon exploration. AAPG Bull. 38, 2267–2295. doi:10.1306/02272019146
Birkenmajer, K., Nagy, J., and Dallmann, W. K. (1992). Markhambreen, spitsbergen. Tromsø: Norsk Polarinstitutt.Geological map svalbard 1:100,000
Blinova, M., Inge Faleide, J., Gabrielsen, R. H., and Mjelde, R. (2012). Seafloor expression and shallow structure of a fold-and-thrust system, Isfjorden, west Spitsbergen. Polar Res. 31, 11209. doi:10.3402/polar.v31i0.11209
Boucher, O., Friedlingstein, P., Collins, B., and Shine, K. P. (2009). The indirect global warming potential and global temperature change potential due to methane oxidation. Environ. Res. Lett. 4, 044007. doi:10.1088/1748-9326/4/4/044007
Braathen, A., Bælum, K., Maher, H., and Buckley, S. J. (2012). Growth of extensional faults and folds during deposition of an evaporite-dominated half-graben basin; the Carboniferous Billefjorden Trough. Svalbard, Nor. J. Geol. 91, 137.
Cavanagh, A. J., Primio, R. D., Scheck-Wenderoth, M., and Horsfield, B. (2006). Severity and timing of cenozoic exhumation in the southwestern Barents Sea. J. Geol. Soc. 163, 761–774. doi:10.1144/0016-76492005-146
Chand, S., Thorsnes, T., Rise, L., Brunstad, H., Stoddart, D., Bøe, R., et al. (2012). Multiple episodes of fluid flow in the SW Barents Sea (Loppa High) evidenced by gas flares, pockmarks and gas hydrate accumulation. Earth Planet. Sci. Lett. 331-332, 305–314. doi:10.1016/j.epsl.2012.03.021
Christiansen, H. H., Etzelmüller, B., Isaksen, K., Juliussen, H., Farbrot, H., Humlum, O., et al. (2010). The thermal state of permafrost in the Nordic area during the International Polar Year 2007–2009. Permafr. Periglac. Process. 21, 156–181. doi:10.1002/ppp.687
Christiansen, H. H., French, H. M., and Humlum, O. (2005). Permafrost in the Gruve-7 mine, adventdalen, svalbard. Norsk Geografisk Tidsskrift-Norwegian J. Geogr. 59, 109–115. doi:10.1080/00291950510020592
Chuvilin, E., Ekimova, V., Davletshina, D., Sokolova, N., and Bukhanov, B. (2020). Evidence of gas emissions from permafrost in the Russian arctic. Geosciences 10, 383. doi:10.3390/geosciences10100383
Chuvilin, E., Yakushev, V. S., and Perlova, E. (2000). Gas and possible gas hydrates in the permafrost of bovanenkovo gas field, yamal peninsula, west siberia. Polarforschung 68, 215–219.
Collett, T. S., Lee, M. W., Agena, W. F., Miller, J. J., Lewis, K. A., Zyrianova, M. V., et al. (2011). Permafrost-associated natural gas hydrate occurrences on the Alaska North Slope. Mar. Petroleum Geol. 28, 279–294. doi:10.1016/j.marpetgeo.2009.12.001
Dallmann, W. K., Elvevold, S., Gerland, S., Hormes, A., Majka, J., Ottemöller, L., et al. (2015). Geoscience atlas of svalbard. Tromsø, Norway: Norsk Polarinstitutt.
Dimakis, P., Braathen, B. I., Faleide, J. I., Elverhøi, A., and Gudlaugsson, S. T. (1998). Cenozoic erosion and the preglacial uplift of the Svalbard–Barents Sea region. Tectonophysics 300, 311–327. doi:10.1016/S0040-1951(98)00245-5
Divine, D. V., and Dick, C. (2006). Historical variability of sea ice edge position in the Nordic Seas. J. Geophys. Res. Oceans 111. doi:10.1029/2004JC002851
Dörr, N., Lisker, F., Jochmann, M., Rainer, T., Schlegel, A., Schubert, K., et al. (2018). “Subsidence, rapid inversion, and slow erosion of the Central Tertiary Basin of Svalbard: evidence from the thermal evolution and basin modeling,” in Circum-arctic structural events: tectonic evolution of the arctic margins and trans-arctic links with adjacent orogens. Editors K. Piepjohn, J. V. Strauss, R. Lutz, and W. C. McClelland (Boulder, Colorado: The Geological Society of America), 169–188.
Dypvik, H., and Zakharov, V. (2012). Fine-grained epicontinental Arctic sedimentation–mineralogy and geochemistry of shales from the Late Jurassic-Early Cretaceous transition. Nor. J. Geology/Norsk Geol. Forening 92, 65–87.
Faleide, J. I., Solheim, A., Fiedler, A., Hjelstuen, B. O., Andersen, E. S., and Vanneste, K. (1996). Late Cenozoic evolution of the western Barents Sea-Svalbard continental margin. Glob. Planet. Change 12, 53–74. doi:10.1016/0921-8181(95)00012-7
Gasser, D. (2014). The Caledonides of Greenland, Svalbard and other Arctic areas: status of research and open questions. Geol. Soc. Lond. Spec. Publ. 390, 93–129. doi:10.1144/SP390.17
Gilbert, G. L., O'Neill, H. B., Nemec, W., Thiel, C., Christiansen, H. H., and Buylaert, J. P. (2018). Late Quaternary sedimentation and permafrost development in a Svalbard fjord-valley, Norwegian high Arctic. Sedimentology 65, 2531–2558. doi:10.1111/sed.12476
Grundvåg, S.-A., Jelby, M. E., Śliwińska, K. K., Nøhr-Hansen, H., Aadland, T., Sandvik, S. E., et al. (2019). Sedimentology and palynology of the Lower Cretaceous succession of central Spitsbergen: integration of subsurface and outcrop data. Nor. J. Geol. 99, 253–284. doi:10.17850/njg006
Harada, K., and Yoshikawa, K. (1996). Permafrost age and thickness near Adventfjorden, Spitsbergen. Polar Geogr. 20, 267–281. doi:10.1080/10889379609377607
Henriksen, E., Bjørnseth, H., Hals, T., Heide, T., Kiryukhina, T., Kløvjan, O., et al. (2011). Chapter 17 Uplift and erosion of the greater Barents Sea: impact on prospectivity and petroleum systems. Geol. Soc. Lond. Memoirs 35, 271–281. doi:10.1144/M35.17
Hodson, A. J., Nowak, A., Holmlund, E., Redeker, K. R., Turchyn, A. V., and Christiansen, H. H. (2019). Seasonal dynamics of methane and carbon dioxide evasion from an open system pingo: lagoon pingo, svalbard. Front. Earth Sci. 30, 1–12. doi:10.3389/feart.2019.00030
Hodson, A. J., Nowak, A., Senger, K., Redeker, K., Christiansen, H. H., Jessen, S., et al. (2020). Open system pingos as hotspots for sub-permafrost methane emission in Svalbard. Cryosphere Discuss., 1–21.
Hornum, M. T., Hodson, A. J., Jessen, S., Bense, V., and Senger, K. (2020). Numerical modelling of permafrost spring discharge and open-system pingo formation induced by basal permafrost aggradation. Cryosphere Discuss. 14, 4627–4651. doi:10.5194/tc-14-4627-2020
Howarth, R. W., Santoro, R., and Ingraffea, A. (2011). Methane and the greenhouse-gas footprint of natural gas from shale formations. Clim. change 106, 679–690. doi:10.1007/s10584-011-0061-5
Humlum, O. (2005). Holocene permafrost aggradation in Svalbard. London: Geological Society. doi:10.1144/GSL.SP.2005.242.01.11
Humlum, O., Instanes, A., and Sollid, J. L. (2003). Permafrost in Svalbard: a review of research history, climatic background and engineering challenges. Polar Res. 22, 191–215. doi:10.3402/polar.v22i2.6455
Huq, F., Smalley, P. C., Mørkved, P. T., Johansen, I., Yarushina, V., and Johansen, H. (2017). The Longyearbyen CO2 Lab: fluid communication in reservoir and caprock. Int. J. Greenh. Gas Control 63, 59–76. doi:10.1016/j.ijggc.2017.05.005
Hynne, I. B. (2010). Depositional environment on eastern svalbard and central spitsbergen during carnian time (late triassic): a sedimentological investigation of the De Geerdalen Formation, Institutt for geovitenskap og petroleum. Trondheim: Norwegian University of Science and Technology.
Isaksen, K., Mühll, D. V., Gubler, H., Kohl, T., and Sollid, J. L. (2000). Ground surface-temperature reconstruction based on data from a deep borehole in permafrost at Janssonhaugen, Svalbard. Ann. Glaciol. 31, 287–294. doi:10.3189/172756400781820291
Jochmann, M. M., Augland, L. E., Lenz, O., Bieg, G., Haugen, T., Grundvåg, S. A., et al. (2019). Sylfjellet: a new outcrop of the Paleogene van mijenfjorden Group in svalbard. arktos 1-22. doi:10.1007/s41063-019-00072-w
Johansen, T. A., Digranes, P., van Schaack, M., and Lønne, I. (2003). Seismic mapping and modeling of near-surface sediments in polar areas. Geophysics 68, 566–573. doi:10.1190/1.1567226
Juliussen, H., Jochmann, M., and Christiansen, H. H. (2010). “High-mountain permafrost temperature monitoring in central Svalbard; implications for Arctic coal mining,” in Third European Confreence on Permafrost; EUCOP III, Svalbard, June 2010.
Kamath, A., Godbole, S., Ostermann, R., and Collett, T. (1987). Evaluation of the stability of gas hydrates in northern Alaska. Cold Regions Sci. Technol. 14, 107–119. doi:10.1016/0165-232X(87)90026-7
Keating, K., Binley, A., Bense, V., Van Dam, R. L., and Christiansen, H. H. (2018). Combined geophysical measurements provide evidence for unfrozen water in permafrost in the adventdalen valley in Svalbard. Geophys. Res. Lett. 45, 7606–7614. doi:10.1029/2017GL076508
Kierulf, H. P., Kohler, J., Boy, J.-P., Geyman, E. C., Mémin, A., Omang, O. C. D., et al. (2022). Time-varying uplift in Svalbard—an effect of glacial changes. Geophys. J. Int. 231, 1518–1534. doi:10.1093/gji/ggac264
Klausen, T. G., Nyberg, B., and Helland-Hansen, W. (2019). The largest delta plain in Earth’s history. Geology 47, 470–474. doi:10.1130/G45507.1
Knoblauch, C., Beer, C., Liebner, S., Grigoriev, M. N., and Pfeiffer, E.-M. (2018). Methane production as key to the greenhouse gas budget of thawing permafrost. Nat. Clim. Change 8, 309–312. doi:10.1038/s41558-018-0095-z
Koevoets, M. J., Hammer, Ø., Olaussen, S., Senger, K., and Smelror, M. (2018). Integrating subsurface and outcrop data of the middle Jurassic to Lower Cretaceous Agardhfjellet formation in central Spitsbergen. Nor. J. Geol. 98, 1–34. doi:10.17850/njg98-4-01
Kristensen, L., Benn, D. I., Hormes, A., and Ottesen, D. (2009). Mud aprons in front of Svalbard surge moraines: evidence of subglacial deforming layers or proglacial glaciotectonics? Geomorphology 111, 206–221. doi:10.1016/j.geomorph.2009.04.022
Landvik, J., Mangerud, J., and Salvigsen, O. (1988). “Glacial history and permafrost in the Svalbard area,” in Proceedings of the 5th International Conference on Permafrost, Trondheim, Norway, August 1988.
Landvik, J. Y., Bondevik, S., Elverhøi, A., Fjeldskaar, W., Mangerud, J., Salvigsen, O., et al. (1998). The last glacial maximum of Svalbard and the Barents Sea area: ice sheet extent and configuration. Quat. Sci. Rev. 17, 43–75. doi:10.1016/S0277-3791(97)00066-8
Landvik, J. Y., Brook, E. J., Gualtieri, L., Raisbeck, G., Salvigsen, O., and Yiou, F. o. (2003). Northwest Svalbard during the last glaciation: ice-free areas existed. Geology 31, 905–908. doi:10.1130/g19703.1
Lasabuda, A., Laberg, J. S., Knutsen, S.-M., and Safronova, P. (2018). Cenozoic tectonostratigraphy and pre-glacial erosion: a mass-balance study of the northwestern Barents Sea margin, Norwegian Arctic. J. Geodyn. 119, 149–166. doi:10.1016/j.jog.2018.03.004
Lashof, D. A., and Ahuja, D. R. (1990). Relative contributions of greenhouse gas emissions to global warming. Nature 344, 529–531. doi:10.1038/344529a0
Leith, T., Weiss, H., Mørk, A., Elvebakk, G., Embry, A., Brooks, P., et al. (1993). “Mesozoic hydrocarbon source-rocks of the Arctic region,” in Norwegian petroleum society special publications (Amsterdam, Netherlands: Elsevier), 1–25. doi:10.1016/B978-0-444-88943-0.50006-X
Lerche, I., Yu, Z., Tørudbakken, B., and Thomsen, R. O. (1997). Ice loading effects in sedimentary basins with reference to the Barents Sea. Mar. Petroleum Geol. 14, 277–338. doi:10.1016/s0264-8172(96)00059-1
Leythaeuser, D., Mackenzie, A., Schaefer, R. G., and BjorØy, M. (1984). A novel approach for recognition and quantification of hydrocarbon migration effects in shale-sandstone sequences. AAPG Bull. 68, 196–219. doi:10.1306/AD4609FE-16F7-11D7-8645000102C1865D
Lind, S., Ingvaldsen, R. B., and Furevik, T. (2018). Arctic warming hotspot in the northern Barents Sea linked to declining sea-ice import. Nat. Clim. change 8, 634–639. doi:10.1038/s41558-018-0205-y
Lord, G. S., Solvi, K. H., Ask, M., Mørk, A., Hounslow, M. W., and Paterson, N. W. (2014). The hopen member: a new member of the Triassic De Geerdalen Formation. Svalbard, Nor. Pet. Dir. Bull. 11, 81–96.
Lyutkevich, E. M. (1937). Geology of tertiary coals of spitzbergen, Isfjord region. Tr. Arkticheskogo Instituta 76, 7–24.
Magnabosco, C., Braathen, A., and Ogata, K. (2014). Permeability model of tight reservoir sandstones combining core-plug and Miniperm analysis of drillcore. Longyearbyen CO2 Lab, Svalbard, Nor. J. Geol. 94, 189–200.
Magoon, L., and Dow, W. (2000). Mapping the petroleum system-an investigative technique to explore the hydrocarbon fluid system. AAPG Mem. 73, 53–68.
Majewski, W., and Zajaczkowski, M. (2007). Benthic foraminifera in Adventfjorden, Svalbard: last 50 years of local hydrographic changes. J. Foraminifer. Res. 37, 107–124. doi:10.2113/gsjfr.37.2.107
Majorowicz, J., and Hannigan, P. (2000). Stability zone of natural gas hydrates in a permafrost-bearing region of the Beaufort—mackenzie Basin: study of a feasible energy Source1 (geological Survey of Canada contribution No. 1999275). Nat. Resour. Res. 9, 3–26. doi:10.1023/A:1010105628952
Makogon, Y., and Omelchenko, R. (2013). Commercial gas production from Messoyakha deposit in hydrate conditions. J. Nat. Gas Sci. Eng. 11, 1–6. doi:10.1016/j.jngse.2012.08.002
Marshall, C., Large, D. J., Meredith, W., Snape, C. E., Uguna, C., Spiro, B. F., et al. (2015). Geochemistry and petrology of Palaeocene coals from Spitsbergen—Part 1: oil potential and depositional environment. Int. J. Coal Geol. 143, 22–33. doi:10.1016/j.coal.2015.03.006
Marum, D. M., Afonso, M. D., and Ochoa, B. B. (2019). Optimization of the gas-extraction process in a new mud-logging system. SPE Drill. Complet. 35, 001–013. doi:10.2118/198909-PA
Masoudi, R., and Tohidi, B. (2005). Estimating the hydrate stability zone in the presence of salts and/or organic inhibitors using water partial pressure. J. Petroleum Sci. Eng. 46, 23–36. doi:10.1016/j.petrol.2004.10.002
Michelsen, J. K., and Khorasani, G. K. (1991). A regional study on coals from Svalbard; organic facies, maturity and thermal history. Bull. Société Géologique Fr. 162, 385–397.
Minshull, T. A., Marín-Moreno, H., Betlem, P., Bialas, J., Buenz, S., Burwicz, E., et al. (2020). Hydrate occurrence in Europe: a review of available evidence. Mar. Petroleum Geol. 111, 735–764. doi:10.1016/j.marpetgeo.2019.08.014
Mørk, M. B. E. (1999). Compositional variations and provenance of triassic sandstones from the Barents shelf. J. Sediment. Res. 69, 690–710. doi:10.2110/jsr.69.690
Mørk, M. B. E. (2013). Diagenesis and quartz cement distribution of low-permeability Upper Triassic–Middle Jurassic reservoir sandstones, Longyearbyen CO2 lab well site in Svalbard, Norway. AAPG Bull. 97, 577–596. doi:10.1306/10031211193
Nielsen, T., Laier, T., Kuijpers, A., Rasmussen, T. L., Mikkelsen, N. E., and Nørgård-Pedersen, N. (2014). Fluid flow and methane occurrences in the Disko Bugt area offshore West Greenland: indications for gas hydrates? Geo-Marine Lett. 34, 511–523. doi:10.1007/s00367-014-0382-2
Norsk Polar Navigasjon A/S, (1977a). Geological report - tromsøbreen No. 1. Stabekk, Norway: Norsk Polar Navigasjon.
Norsk Polar Navigasjon A/S, (1977b). Final drilling report - tromsøbreen No. 1. Stabekk, Norway: Norsk Polar Navigasjon.
Norsk Polarinstitutt: Svalbardkartet, (2015).Norsk Polarinstitutt, Tromsø: Norsk Polarinstitutt WebGIS.
Norske Fina, A./S. (1972a). Geological report - well: plurdalen No. 1. Stabekk, Norway: Norske Fina.
Norske Fina, A./S. (1972b). Final drilling report - well: plurdalen No.1, 71. Stabekk, Norway: Norske Fina.
Norskpetroleum, (2020). Exploration activity. https://www.norskpetroleum.no/en/exploration/exploration-activity/.
Nøttvedt, A., Cecchi, M., Gjelberg, J., Kristensen, S., Lønøy, A., Rasmussen, A., et al. (1993). “Svalbard-Barents Sea correlation: a short review,” in Norwegian petroleum society special publications (Amsterdam, Netherlands: Elsevier), 363–375.
Ohm, S., Larsen, L., Olaussen, S., Senger, K., Birchall, T., Demchuk, T., et al. (2019). Discovery of shale gas in organic-rich jurassic successions, adventdalen, central spitsbergen, Norway. Nor. J. Geol. 99, 28. doi:10.17850/njg007
Ohm, S. E., Karlsen, D. A., and Austin, T. (2008). Geochemically driven exploration models in uplifted areas: examples from the Norwegian Barents Sea. AAPG Bull. 92, 1191–1223. doi:10.1306/06180808028
Olaussen, S., Grundvåg, S.-A., Senger, K., Anell, I., Betlem, P., Birchall, T., et al. (2022). The svalbard carboniferous to cenozoic composite tectono-stratigraphic element. London, Memoirs: Geological Society. doi:10.1144/M57-2021-36
Olaussen, S., Larssen, G. B., Helland-Hansen, W., Johannessen, E. P., Nøttvedt, A., Riis, F., et al. (2018). Mesozoic strata of kong karls land, svalbard, Norway; a link to the northern Barents Sea basins and platforms. Nor. J. Geol. 98, 1–69. doi:10.17850/njg98-4-06
Olaussen, S., Senger, K., Braathen, A., Grundvåg, S. A., and Mørk, A. (2019). You learn as long as you drill; research synthesis from the Longyearbyen CO2 Laboratory, Svalbard, Norway. Nor. J. Geol. 99, 157–187. doi:10.17850/njg008
Osterkamp, T., and Payne, M. (1981). Estimates of permafrost thickness from well logs in northern Alaska. Cold Regions Sci. Technol. 5, 13–27. doi:10.1016/0165-232X(81)90037-9
Piepjohn, K. (2000). The svalbardian-ellesmerian deformation of the old red sandstone and the pre-devonian basement in NW spitsbergen (svalbard). London: Geological Society. doi:10.1144/GSL.SP.2000.180.01.31
Polargas Prospektering, K. B. (1988). Geological report - tromsøbreen No. 2. Oslo, Norway: Polargas Prospektering.
Polyakova, I. (2015). Petroleum source rocks of the Arctic region. Lithology Mineral Resour. 50, 26–49. doi:10.1134/S002449021406008X
Przybylak, R., Arazny, A., Nordli, O., Finkelnburg, R., Kejna, M., Budzik, T., et al. (2014). Spatial distribution of air temperature on Svalbard during 1 year with campaign measurements. Int. J. Climatol. 34, 3702–3719. doi:10.1002/joc.3937
Rachlewicz, G., and Szczuciński, W. (2008). Changes in thermal structure of permafrost active layer in a dry polar climate. Petuniabukta, Svalbard, Pol. Polar Res. 29, 261–278.
Rismyhr, B., Bjærke, T., Olaussen, S., Mulrooney, M., and Senger, K. (2019). Facies, palynostratigraphy and sequence stratigraphy of the wilhelmøya subgroup (upper triassic–middle jurassic) in western central spitsbergen, svalbard. Nor. J. Geol. 99, 35–64. doi:10.17850/njg001
Rodes, N., Betlem, P., Senger, K., Römer, M., Hodson, A., Liira, M., et al. (2023). Active gas seepage in western Spitsbergen fjords, Svalbard archipelago: spatial extent and geological controls. Front. Earth Sci. 11, 2023. doi:10.3389/feart.2023.1173477
Ronen, S., Rokkan, A., Bouraly, R., Valsvik, G., Larson, L., Ostensvig, E., et al. (2012). Imaging shallow gas drilling hazards under three Forties oil field platforms using ocean-bottom nodes. Lead. Edge 31, 465–469. doi:10.1190/tle31040465.1
Roy, S., Hovland, M., Noormets, R., and Olaussen, S. (2015). Seepage in isfjorden and its tributary fjords, West Spitsbergen. Mar. Geol. 363, 146–159. doi:10.1016/j.margeo.2015.02.003
Schmitt, D., Welz, M., and Rokosh, C. (2005). High-resolution seismic imaging over thick permafrost at the 2002 Mallik drill site. Geol. Surv. Can. Bull. 585, 125.
Schulson, E. M. (2001). Brittle failure of ice. Eng. Fract. Mech. 68, 1839–1887. doi:10.1016/S0013-7944(01)00037-6
Senger, K., Birchall, T., Betlem, P., Ogata, K., Ohm, S., Olaussen, S., et al. (2020). Resistivity of reservoir sandstones and organic rich shales on the Barents Shelf: implications for interpreting CSEM data. Geosci. Front. 64, 101063. doi:10.1016/j.gsf.2020.08.007
Senger, K., Brugmans, P., Grundvåg, S.-A., Jochmann, M. M., Nøttvedt, A., Olaussen, S., et al. (2019). Petroleum, coal and research drilling onshore Svalbard: a historical perspective. Nor. J. Geol. 99, 30. doi:10.17850/njg99-3-1
Senger, K., Nuus, M., Balling, N., Betlem, P., Birchall, T., Christiansen, H. H., et al. (2023). The subsurface thermal state of Svalbard and implications for geothermal potential. Geothermics 111, 102702. doi:10.1016/j.geothermics.2023.102702
Senger, K., Tveranger, J., Ogata, K., Braathen, A., and Planke, S. (2014). Late mesozoic magmatism in svalbard: a review. Earth-Science Rev. 139, 123–144. doi:10.1016/j.earscirev.2014.09.002
Skorobogatov, V. A., Yakushev, V. S., and Chuvilin, E. M. (2007). “Sources of natural gas within permafrost; North-West, Siberia,” in Permafrost Proceedings Seventh International Conference, Collection Nordicana, Canada, June 2007.
Sloan, E. D., Koh, C. A., and Koh, C. (2007). Clathrate hydrates of natural gases. Boca Raton, Florida, United States: CRC Press.
Smyrak-Sikora, A., Johannessen, E. P., Olaussen, S., Sandal, G., and Braathen, A. (2019). Sedimentary architecture during Carboniferous rift initiation–the arid billefjorden trough, svalbard. J. Geol. Soc. 176, 225–252. doi:10.1144/jgs2018-100
Steel, R., Dalland, A., Kalgraff, K., and Larsen, V. (1981). The Central Tertiary Basin of Spitsbergen: sedimentary development of a sheared-margin basin. AAPG Mem. 7, 647–664.
Steel, R. J., and Worsley, D. (1984). “Svalbard's post-Caledonian strata - an atlas of sedimentational patterns and paleogeographic evolution,” in Petroleum geology of the north European margin. Editor A. M. Spencer (London: Graham & Trotman), 109–135. doi:10.1007/978-94-009-5626-1_9
Strand, S. M., Christiansen, H. H., Johansson, M., Åkerman, J., and Humlum, O. (2021). Active layer thickening and controls on interannual variability in the Nordic Arctic compared to the circum-Arctic. Permafr. Periglac. Process. 32 (1), 47–58. doi:10.1002/ppp.2088
Uguna, J. O., Carr, A. D., Marshall, C., Large, D. J., Meredith, W., Jochmann, M., et al. (2017). Improving spatial predictability of petroleum resources within the Central Tertiary Basin, Spitsbergen: a geochemical and petrographic study of coals from the eastern and western coalfields. Int. J. Coal Geol. 179, 278–294. doi:10.1016/j.coal.2017.06.007
UNIS CO2 Lab AS (UNIS – The University Centre in Svalbard) (2015). The longyearbyen CO2 lab - phase 2 final report. Longyearbyen, Svalbard: The University Centre in Svalbard, 2015.
van Pelt, W. J., Kohler, J., Liston, G., Hagen, J. O., Luks, B., Reijmer, C., et al. (2016). Multidecadal climate and seasonal snow conditions in Svalbard. J. Geophys. Res. Earth Surf. 121, 2100–2117. doi:10.1002/2016JF003999
Verba, M. (2013). Sedimentary cover reservoir of Svalbard archipelago. Neftegazov. Geol. Teor. I Prakt. 8, 1–45. doi:10.17353/2070-5379/5_2013
Vonk, J. E., and Gustafsson, Ö. (2013). Permafrost-carbon complexities. Nat. Geosci. 6, 675–676. doi:10.1038/ngeo1937
Vrielink, H. J., Bradford, J. S., Basarab, L., and Ubaru, C. C. (2010). “Successful application of casing-while-drilling technology in a Canadian arctic permafrost application,” in IADC/SPE Drilling Conference, Orlando, Florida, USA, March 2010. doi:10.2118/111806-MS
Westermann, S., Wollschläger, U., and Boike, J. (2010). Monitoring of active layer dynamics at a permafrost site on Svalbard using multi-channel ground-penetrating radar. Cryosphere 4, 475–487. doi:10.5194/tc-4-475-2010
Keywords: permafrost, natural gas, cryosphere, greenhouse gas, methane, Arctic
Citation: Birchall T, Jochmann M, Betlem P, Senger K, Hodson A and Olaussen S (2023) Permafrost trapped natural gas in Svalbard, Norway. Front. Earth Sci. 11:1277027. doi: 10.3389/feart.2023.1277027
Received: 13 August 2023; Accepted: 30 October 2023;
Published: 13 December 2023.
Edited by:
Jochen Knies, Geological Survey of Norway, NorwayReviewed by:
Sunil Vadakkepuliyambatta, National Centre for Polar and Ocean Research (NCPOR), IndiaCopyright © 2023 Birchall, Jochmann, Betlem, Senger, Hodson and Olaussen. This is an open-access article distributed under the terms of the Creative Commons Attribution License (CC BY). The use, distribution or reproduction in other forums is permitted, provided the original author(s) and the copyright owner(s) are credited and that the original publication in this journal is cited, in accordance with accepted academic practice. No use, distribution or reproduction is permitted which does not comply with these terms.
*Correspondence: Thomas Birchall, dG9tQGdlb2JpcmNoLmNvbQ==
Disclaimer: All claims expressed in this article are solely those of the authors and do not necessarily represent those of their affiliated organizations, or those of the publisher, the editors and the reviewers. Any product that may be evaluated in this article or claim that may be made by its manufacturer is not guaranteed or endorsed by the publisher.
Research integrity at Frontiers
Learn more about the work of our research integrity team to safeguard the quality of each article we publish.