- 1School of Earth Science and Engineering, Sun Yat-Sen University, Zhuhai, China
- 2School of Architectural Engineering, Guangzhou Institute of Science and Technology, Guangzhou, China
- 3Guangdong Provincial Key Lab of Geodynamics and Geohazards, Zhuhai, China
- 4College of Civil and Transportation Engineering, Shenzhen University, Shenzhen, China
- 5Guangxi Beitou Highway Construction and Investment Group Co., Ltd., Nanning, China
- 6224 Harbert Center, Department of Civil Engineering, Auburn University, Auburn, AL, United States
A 24 km urban expressway on the Leizhou Peninsula in southern China required the extensive use of different graduation basaltic residual soils nearby as subgrade filler. In order to fully understand the engineering properties of these Leiqiong basaltic residual soils, four different fine granule contents (with 24.26%, 55.72%, 84.50%, and 94.92%) along the expressway construction sites were selected for preliminary analysis of their chemical composition, oxide content, and microstructure. Five cement contents (1.5%, 3%, 4.5%, 6%, and 9%) were used to improve the residual soils based on the results of the unconfined compressive strength test and consolidated undrained triaxial compression test, the cement improvement index, which can both meet the strength requirement of the roadbed and avoid the brittle failure of soil is determined. The relationship between carrying capacity and moisture content of soil samples after outdoor improvement is studied, and explains why the moisture content corresponding to the maximum CBR of outdoor improved basalt residual soil is higher than the optimal moisture content of indoor improvement test. Parameters investigated include consistency limits, unconfined compressive strength, compressibility, shearing stress, California bearing ratio (CBR), and compactness. The relationship between the Leizhou peninsula basaltic residual soils with different granule sizes, recommended water content ratio, rolling cycle times, and compaction degree are studied outdoors, and the application suggestion of different granule sizes of basaltic residual soils in different grade highway subgrade engineering is put forward. The results are as follows: 1) The content of fine granules greatly influences the physical and mechanical properties of Leizhou peninsula basalt residual soil. 2) Due to the special colloidal state, the cement uniformity error of basalt residual soils with fine granule content of more than 85% are plus or minus 3% when it is mechanically modified, and the improvement recommended content of cement is 6% if it needs as subgrade filling materials after disturbance. 3) It is recommended that the soil moisture content before improvement in high-temperature areas should be Opt+2%, and the cement improvement time should be controlled within 50 min 4) After rolling seven times, the maximum compaction degree of residual soil with fine granule content greater than 85% is less than 95%; it is only suitable for filling embankment structures below the roadbed of highway. The results extend the research on the engineering characteristics of basaltic residual soils and provide practical, improved guidance for the use of basaltic residual soils in road foundation engineering.
1 Introduction
Basaltic residual soil is the residue of basaltic rock formed under the action of physical and chemical differentiation. It is the most widely distributed soil in the tropics. Its properties are complex and affected by the degree of differentiation, solar radiation, climate, and biology. In tropical regions, civil engineering construction requires extensive use of local low-cost basalt residual soil fillers. Zhanjiang Avenue, a high-grade urban expressway on the Leizhou Peninsula in southern China, is under construction. The total length of Zhanjiang Avenue is 24.8 km, and the width of the roadbed is 46 m. Basaltic residual soil is used as the main filling material along the road, and the soil granule size composition varies greatly in the different sections of the road. Some sections have a high gravel content, sandy clay with good grading (Venkatarama Reddy and Latha, 2014), while others have huge, high-plasticity clay with poor water stability and difficult compaction. High-plasticity clay soil accounts for more than 70% of the total soil along the route and is characterized by a high content of fine granules, a high liquid limit, and a large pore ratio. Its physical properties are similar to those of the soft soils studied by Chen et al. (2013); Lee et al. (2005). Its mechanical properties vary greatly before and after soil disturbance and are similar to those reported by Meng et al., Caner et al., and Gutierrez et al. in China’s Hainan Province, in southern Brazil, and in the Malay Peninsula in the presence of highly sensitive basaltic residual soils (yan Meng et al., 2018; Ka and sim, 2003). Ladd et al. (1993); Raihan Taha et al. (2000); Rigo et al. (2006); Caner et al. (2014); Gutierrez et al. (2009) studied the consolidation properties, mechanical characteristics, chemical compositions, and microstructures of highly plastic basaltic residual soils, and they suggest that the high compressive modulus and high sensitivity of some basaltic residual soils may be related to the special cementing substances and soil granule arrangement of the soil masses.
China’s Code for the Design of Highway Subgrade (JTG D30-2015) (Communications Second Highway Survey and Design Research Institute Co Ltd, 2015) stipulates that the uniformity coefficient (Cu) should be equal to 1–3, and soils with fine granule contents of less than 30% have well-graded characteristics. The direct use of soils with fine granule contents of greater than 50% for roadbed filling without treatment will lead to a variety of serious engineering problems, such as uneven settlement, pavement cracking, and slope collapse (Leshchinsky et al., 1999; Bishop and, 2015; Abboud, 2022; Chen et al., 2023). Regarding the effect of common ordinary silicate cement and lime on the modification of high plasticity clay, Oluwatuyi et al. (2019); Oluwatuyi et al. (2020) found that Lime and cement can greatly stabilize contaminated soils. Bhattacharja and Bhatty (2003) found that the engineering properties and durability of the soil were better after treatment with silicate cement than after treatment with lime. In indoor tests, for different fine granule contents, liquid limits, and chemical compositions, researchers have proposed improvement schemes involving different additives combined with cement. For example, Eze-Uzomaka and Agbo (2010) proposed the use of 6% cement and 30% quarry dust to improve clay soils with liquid limits of >60 and fine granule contents of >90% for the abundant quarry dust resources in Nigeria. Oluwatuyi et al. (2018) found that an 8% by soil weight of milled eggshell and cement mixture in a 1:1 stabilized lateritic soil ratio could be used as a potential subbase material for highway construction. Chen and Wang (2006) reported that the combination of crystalline calcium sulfate (reacting with humic acid) and cement improvement for soft soils with excessive organic matter hinders the efficiency of the cement hydration reaction. Regarding the nature of the soil itself, which may affect the improvement effect, Yang et al. (2013) investigated the effect of the PH of the environment on the soil improvement effect of cement and found that the weak acidity (PH = 4.9–5.9) of basaltic residual soils in subtropical regions causes a 10% decrease in the hydration reaction efficiency of the cement relative to that in neutral soils. Consoli et al. (2011) investigated the relationships between the granule size composition, soil porosity, and cement improvement effect. Zhao et al. (2022) investigated the changes in the micropore structure, saturation permeability coefficient, and strength characteristics of high plasticity powder soils when different proportions of cement and different curing ages were used, and they proposed that high plasticity powder soils should be improved with cement ratio of at least 6% (Consoli et al., 2011). Ikhlef et al. (2014); Veloso Marques et al. (2014) conducted several indoor and field experiments, including microscopic studies, mechanical compaction tests, and field load-bearing plate loading experiments, to investigate the strength generation mechanism of cement-improved soils and the feasibility of using such soils as road base materials (Abd Karim et al., 2014; Mengue et al., 2017). In engineering applications, researchers have found that the highest California Bearing Ratio (CBR) value of chemically improved soil samples corresponds to a moisture content, that is, not the optimum moisture content, i.e., 1%–2% higher than the optimum moisture content (Wang et al., 2022). However, they did not explain the reasons for this phenomenon or whether it is common. Previous research on the engineering properties and improvement methods of basaltic residual soils has mainly been based on indoor experiments, and field experiments have rarely considered the construction environment and the complex intrinsic conditions of the soil itself. For example, the fact that the granule size composition of the soil is different at different locations (Lasisi and Ogunjide, 1984), that the water content of the soil is not a fixed value (Veloso Marques et al., 2014) (varying within an interval of the optimum moisture content), and that the hydration reaction of cement and the temperature of the site affect the change in the water content of the soil, thus affecting the curing efficiency (Osula, 1996), have rarely been considered. When mixing the soil with additives on site, there is a small amount of mechanical error in the mixing process using the cold recycler, but the extent of the error and the resulting consequences are not clear. For basaltic residual soils with different granule size compositions, there is a lack of field application references regarding the compaction effect achieved for different moisture contents and different rolling times, as well as for which part of the subgrade is suitable for filling.
In order to comprehensively understand the engineering properties of basaltic residual soils, four types of basaltic residual soils with P (fine granule contents) of 24%, 56%, 85%, and 95% were selected. Their physical and mechanical properties were analyzed to gain a systematic understanding of the basic properties of basaltic residual soils with different granule size compositions. For the high-sensitivity basaltic residual soil with the largest quantity and the worst physical properties (p > 85%), we studied its compression and deformation characteristics through consolidation experiments and unconfined lateral compressive strength experiments (ASTM International, 2017; ASTM International, 2011b), and then we analyzed the main components of the cementing material inside the soil samples through chemical experiments (ASTM International, 2011c). The soil samples with insufficient bearing capacities were mixed with cement (1.5%–9%) to determine the changes in the workability properties, compaction characteristics, curing characteristics, and bearing capacity (CBR value) (Sariosseiri and Muhunthan, 2009; Uddin et al., 1997) and to investigate the soil damage patterns during modified unconfined compressive strength and consolidation (without drainage) triaxial compression experiments. The mechanical error range of the cold recycling machine is studied by field test to ensure that the minimum cement content in the fluctuation range meets the subgrade strength requirements and that the maximum does not cause strong brittle failure. The relationships between the granule contents of the soil with the optimal cement content ratio at the construction site, the number of rolling cycles, and the degree of compaction under different water contents were investigated. Reference values for the filling parameter of basaltic residual soils with different granule size compositions were also determined. The results of this study extend the research on the engineering characteristics of basaltic residual soils and provide practical guidance for the use of basaltic residual soils in road foundation engineering.
2 Soil sampling locations and experimental materials
The soil around the project is mainly weathered from basaltic, with a total area of about 440.50 km2, and the main weathering period was the Late Pleistocene. The soil samples collected from this area were mainly brown-red, purple-red, or red-white clay. The geological profile based on basaltic weathering is shown in Figure 1A. Most soils 15–22 m below the surface are clay, with local inclusions of medium sand and coarse sand. The climate in southern China is hot and humid, and the annual average temperature is 22.5°C. A fine granule is a granule with a diameter of less than 0.075 mm. In this study, four common basaltic residual soils with significantly different fine granule contents (refer as p values) were selected and labeled TH (p = 24.26%), ZH (p = 55.72%), ZB (p = 84.50%), and DH (p = 94.92%) (Figure 1B). Due to the high degree of cementation of the undisturbed soils (undisturbed) from sites ZB and DH, it was necessary to carry out sensitivity experiments. In order to retain its structure and avoid disturbance, a sharp ring knife (diameter is 100 mm, height is 300 mm) was used to penetrate into the soil and collect the sample, which was then sealed for transportation and preservation and opened during the experiment.
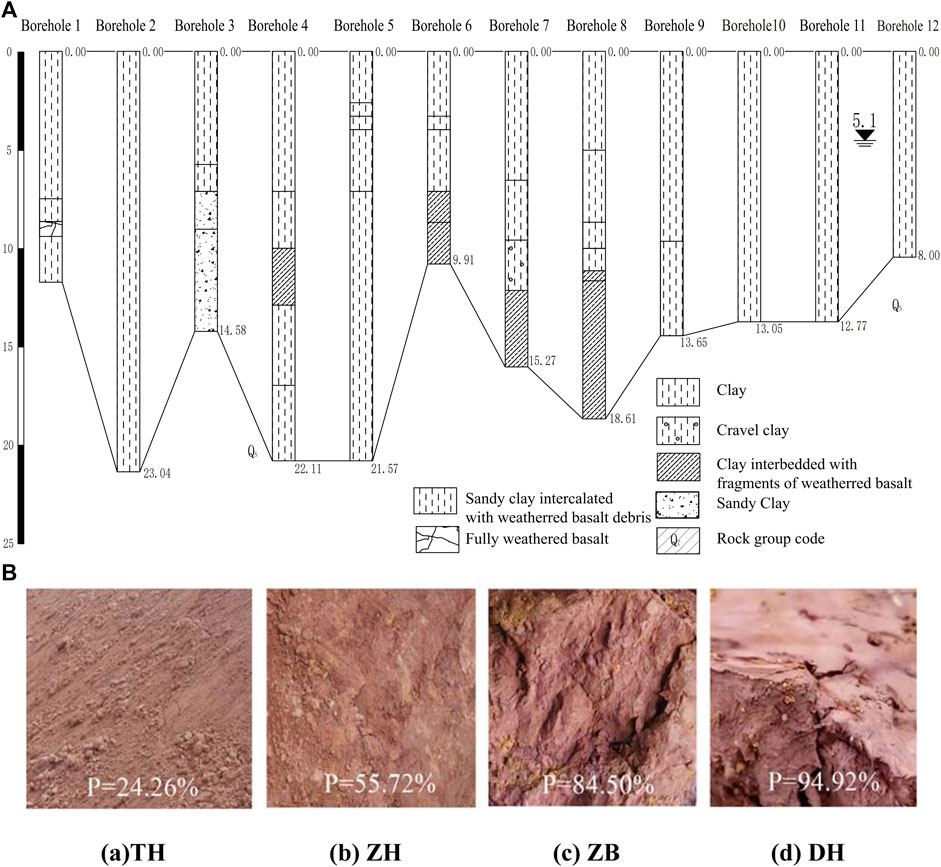
FIGURE 1. Geological section and basaltic residual soils. (A) Geological section of the Zhanjiang Avenue construction site. (B) Four basaltic residual soils with different grain size compositions.
3 Experimental method
After a preliminary study of the basic physical properties of the four soil samples, different proportions of cement (0%, 1.5%, 3%, 4.5%, 6%, and 9% of dry soil weight) were added to ZH, ZB, and DH (as all of their natural CBR were less than 8%), and Atterberg limit tests, curing tests, standard compaction tests, California bearing ratio tests were conducted to test the effects of their engineering characteristics, with different proportions of cement. The experimental procedure for the CBR test is shown in Figure 2A. A universal material instrument was used for the static compaction of the cement-improved samples, and all samples were soaked for 96 h. The dial indicator readings before and after soaking were recorded to calculate the expansion rate. The CBR value of the soil samples after soaking was measured by using a penetrometer, and the improvement effect of the different proportions of cement on the soil samples under field conditions was simulated.
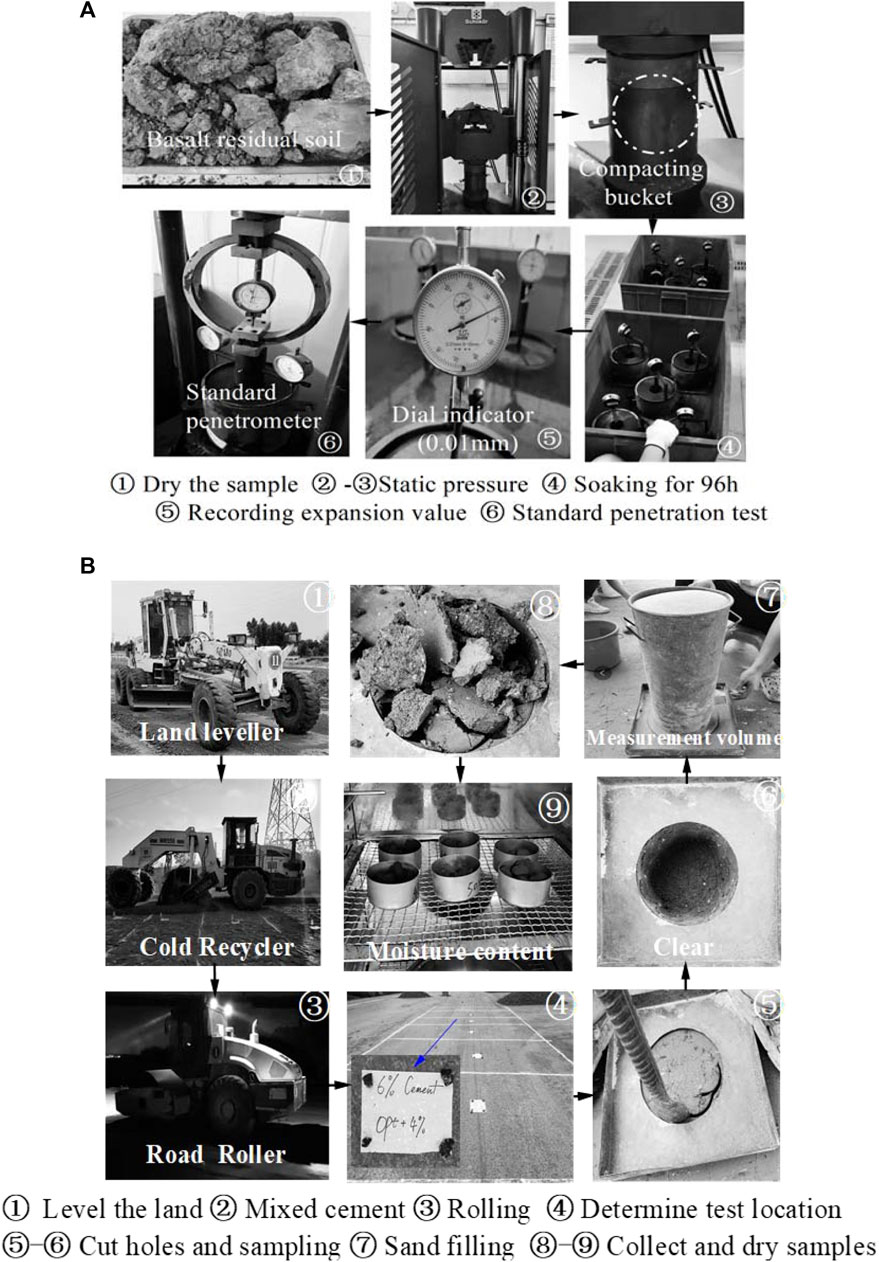
FIGURE 2. Workflow of the experiment. (A) The workflow of the California bearing ratio (CBR) experiments. (B) The workflow of the field rolling experiment.
In order to more accurately understand the influence of outdoor soil moisture content fluctuation on cement improvement, CBR experiments were conducted on ZH, ZB, and DH with the same proportion of cement under six different moisture contents (Opt-4%, Opt-2%, Opt, Opt+2%, Opt+4%, and Opt+6%). W is the abbreviation for the moisture content, whereas Opt stands for the optimum moisture content.
Due to the high content of fine granules in ZB and DH in the construction sites, the consolidation tests and unconfined compressive strength tests were carried out on undisturbed and remolded samples. In order to study the influence of the cement content on the failure mode of the improved soils, the unconfined compressive strength of DH, which had the worst engineering properties and was the most widely distributed throughout the project area, was tested after modification with different proportions of cement. The experiments were divided into two groups to study whether the improved soil needed constant watering. In group 1, all of the samples were placed in a sealed container with constant humidity for 7 days, and then they were tested. In group 2, all of the samples were soaked for 1 day after 7 days of curing, and then they were tested. In addition, consolidated undrained triaxial compression tests were conducted on the DH with 6%, 9%, and 12% cement contents to simulate soil failure under a load after high proportion cement improvement. The sample mold used for the triaxial test was a specially customized cylinder with a diameter of 7 cm and a height of 16.4 cm. (Note: The reason why only DH is selected for the unconfined compressive strength test and triaxial compression test is that the p-value of DH and the degree of sensitivity are the largest, so the proportion of cement mixing error is also the largest.)
The process of the field compaction test is shown in Figure 2B 1) According to the optimal water content and its fluctuation range (from Opt−4% to Opt+6%), four test sections with lengths of 30 m, widths of 8 m, and thicknesses of 0.3 m were paved. Six parallel experiments with different water contents (Opt−4%, Opt−2%, Opt, Opt+2%, Opt+4%, and Opt+6%) were conducted for each soil sample from sites TH, ZH, ZB, and DH. 2) The moisture content was measured every 15 min during the sun-drying stage. Cement (except for TH) was added to the surface of the soil sample immediately after the moisture content reached the required level. The mixture was evenly mixed and smoothed using a cold recycling machine. 3) Using no less than an 18t roller for the rolling, the compaction tests began from the second rolling. The compactness of the soil was recorded after each rolling, and the rolling was repeated until the degree of compaction remained stable.
The pH value of the soil was measured using a conventional pH meter. The mineral composition of the soil samples was analyzed via X-ray diffraction (XRD); and the chemical composition and free metal oxide content of the soil samples were analyzed via X-ray fluorescence (XRF). The microstructure of the soil samples before and after the improvement were compared using scanning electron microscopy (SEM).
4 Results and analysis
4.1 Physical properties
The fine granule content (i.e., the content of the granules with diameters of less than 0.075 mm) is defined as the p-value. Table 1 shows the difference in physical properties of basalt residual soils in different regions (including Leizhou Peninsula). Table 2 and Figure 3 show that the fine granules in ZB and DH account for 85%–95% of the total mass, the liquid limit is more than 50%, and the CBR value is less than 3%. Thus, they are classified as poor soils in engineering. However, the compressibilities of ZB and DH are low (compressibility coefficient of less than 0.3), they are somewhat acidic, and the natural void ratios are greater than 1.2. The properties of TH (p < 25%, CBR = 14.4%) indicate that there is no need for cement improvement, and TH can be directly used as subgrade filling for highways of all grades (Communications Second Highway Survey and Design Research Institute Co Ltd, 2015). The soil samples were loose when dry (Figure 1B). The engineering properties of ZH are between those of TH and ZB. The expansion rates of DH and ZB are smaller than that of the same type of basaltic residual soil, which may be related to the presence of cemented Fe-Al oxides (Schwertmann, 1993).
The positions of the four basaltic residual soils in the plastic diagram are significantly different (Figure 4). TH is located in the CL area, indicating that it is low-plastic sandy clay. ZB and DH have similar properties (both located in the CH area) and are classified as clays with a high plasticity index. Compared with TH and ZH, ZB and DH have smaller maximum dry densities and higher optimal water contents (Figure 5), and their application conditions as roadbed fillers are stricter, requiring physical or chemical modifications prior to use.
SEM was used to analyze the microstructure of TH, ZH, ZB, and DH, including the details of the fine granules and pore distribution. There were more coarse granules in TH and obvious coarse sand–coarse sand, coarse sand–medium sand, and medium sand–medium sand contacts in the coarse-grained occlusion body. Fine granules can fill the spaces between the soil skeleton better, and there will be some large and medium pores (Figure 6A), but the soil sample is easily compacted.
Since the fine granule content of ZH was 130% greater than that of TH, the proportion of medium and micropores in ZH was high, although the macropore content was low. As shown in Figure 6B, the fine granule aggregates exhibited a flocculent distribution, and they filled in the pores, making the coarse granules bite shallowly. The coarse granule aggregates were mainly composed of medium sand–fine sand and fine sand–fine sand contacts, and some were in indirect contact through the fine granule aggregates. The soil skeleton’s density was insufficient. As shown in Figures 6C, D, ZB and DH were flocculent, and the coarse granule contents of these soils were small. The viscous minerals and free oxides in the clay granules caused the fine granules to aggregate through cementation, attaching to or directly wrapping around the coarse granules, and the friction is small. When shear displacement occurred, the shear strengths of ZB and DH were borne by the cohesive force between the fine granules and cementing materials.
4.2 Consolidation characteristics and sensitivity analysis
The consolidation pressure was set to 50, 100, 200, 400, 800, 1,600, and 3,200 kPa of imposed vertical stress. The compression coefficient varied with the pressure (Figure 7). In the initial stage of compression, the compression coefficients of the undisturbed ZB and DH were much smaller than those of the remolded soils, indicating that the pores in the undisturbed soil were small in the long-term over-consolidated state, and the structural strength formed by the granule cementation could resist a certain amount of vertical pressure. After a series of mixing steps, the skeleton formed by the cementation of a large number of fine granules in the remolded ZB and DH soils was destroyed, the strength was weakened, and the number of pores increased. In the low-pressure range (i.e., 50 –800 kPa), the compression of the remolded soil was much larger than that of the undisturbed soil.
Basaltic residual soil with a long weathering time and deep burial depth is often in a normal or over-consolidated state, and its structure is stable. When severely disturbed by outside forces, the natural structure of the soil will be destroyed, and the cementing materials between the soil granules and the equilibrium system composed of soil granules, ions, and water molecules will be broken, resulting in a decrease in the cohesive strength of the soil and increased compressibility (ASTM International, 2011a; ASTM International, 2011b). Figure 8 shows the stress–strain curve for the undisturbed and remolded DH obtained through unconfined compression tests. It can be seen that the peak stress of the undisturbed soil was 138 kPa, which was about 3.5 times that of the remolded soil (41 kPa). When the peak stress was not reached, the stress increased rapidly with increasing strain, and the soil was elastic. After exceeding the peak value, the soil began to undergo plastic deformation, and the internal structure began to be damaged. The stress gradually decreased with increasing strain, and it finally reached a relatively stable stress state (i.e., the residual stress state). Based on the test results presented in Table 3, the average unconfined compressive strength of the undisturbed ZB and DH basaltic residual soils was 161.7 and 141.6 kPa, respectively, and their average sensitivities were 4.1 and 4.0, making them highly sensitive soil. According to China’s Highway Subgrade Design Code (JTG D30-2015) (Communications Second Highway Survey and Design Research Institute Co Ltd, 2015), the bearing capacity Pk of the lower embankment (1.5–2.0 m below the pavement) should be greater than 98 kPa. Although the fine granule contents of the undisturbed DH and ZB soils were high, their special structure resulted in the soil strength meeting the bearing capacity requirements for a lower embankment. Thus, ZB and DH can be directly used to construct a lower embankment structure, but very careful protection is needed to ensure that it is not disturbed and its structure is not damaged. If soil with these special structures needs to be transported over long distances before being used as filler, the mechanical disturbance will destroy the structure of the soil, resulting in difficult compaction and an insufficient bearing capacity.
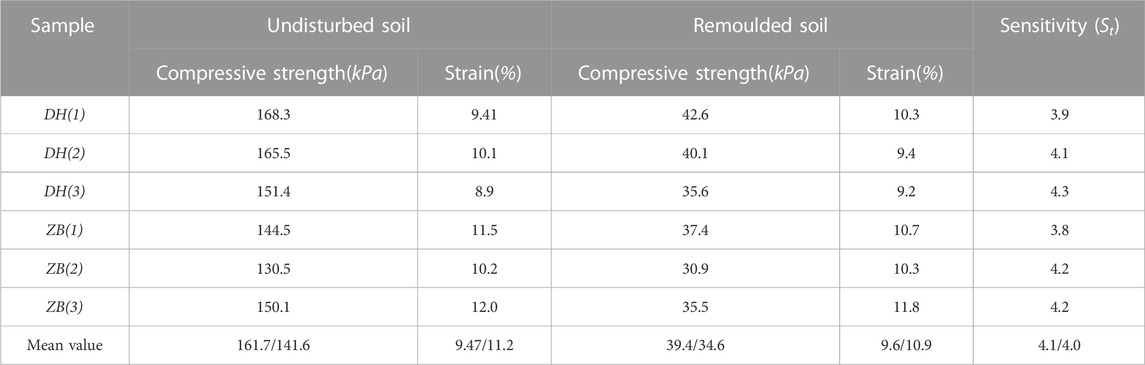
TABLE 3. Comparison of unconfined compressive strengths of undisturbed and remolded basaltic residual soil samples (ZB and DH).
4.3 Mineral composition and chemical analysis
In the XRD analysis, continuous scanning and biaxial driving were used. The starting angle was 10° and the ending angle was 80°. As shown in Figure 9 and Table 4, the main mineral components of the four soil samples are quartz, kaolin and illite. The fine particles in TH and ZH contain more kaolin and illite with strong hydrophilic properties, but their compression and permeability are not affected by kaolin and illite due to the small content of fine particles in their interior. ZB and DH have high fine particle content, with illite and kaolin more than 75% of the total mass. Being long-located in the deep surface and below the water table (As shown in Figure 1), the compaction leads to the formation of colloids in the state of super-consolidation, with high cementation and low compression performance (Figure 7).
It can be seen from Table 5 that most of the soluble MgO, CaO, Na2O, and K2O in the chemical components of ZB and DH were dissolved during seepage, and the contents were lower (0.5%–6%). The insoluble Al2O3 and Fe2O3 contents were relatively high, with average values of 17.9% and 25.5%, respectively, indicating that these two oxides are gradually accumulated when basaltic is weathered into residual soil. The higher SiO2 content (mean of 37%) may be related to the higher contents of kaolin and illite (the sum of both accounts for more than 75% of the fine granule masses of ZB and DH). Table 6 shows the content of free metal oxides. The main component of the free oxides in the ZB and DH basaltic residual soils was free iron oxide, free alumina and a small amount of free silicon oxide, indicating that the main cementing material was free iron oxide. Rich free iron oxide causes the fine granules to form aggregates in the soil, and the structure is stable after long-term consolidation (Rahman et al., 2010; Zhang et al., 2017). Compared with granite residual soil and hornblende residual soil (Zhao et al., 2022; Amphibolite, 1985), after structural damage, basaltic residual soil is difficult to compact (e.g., ZB, Opt = 19.2%, ρmax = 1.78 g/cm3) and thus a higher optimum moisture content and lower maximum dry density will lead to the need for a higher compaction energy when it is used for subgrade filling (Gidigasu, 2012).
In addition, most of the basalt residual soils in Leizhou Peninsula shown in Table 2 are weakly acidic (PH=5.0–5.8), which may be due to the large amount of iron and aluminum in the residual soils. Due to perennial climatic transport and weathering, iron ions will be transformed from low to high prices. Percolation can also lead to the seepage of bivalent iron ions in the soil, and oxidation reaction under acidic conditions to form iron hydroxide solid, resulting in hydrogen ions. The hydrolysis of aluminum ions will increase the hydrogen ions in the aqueous solution and increase the acidity of the soil.
4.4 Effects of cement content on workability, curing characteristics, and bearing capacity
It can be seen from Figure 10A that the CBR values of ZH, ZB, and DH before improvement were 6.8%, 2.1%, and 1.8%, respectively, which are less than the minimum CBR value of 8% for roadbed soil filling (Communications Second Highway Survey and Design Research Institute Co Ltd, 2015). These values can be improved by the addition of cement. After adding 1.5%, 3%, 4.5%, 6%, and 9% cement, it was found that the CBR values of ZH, ZB, and DH increased significantly as the cement content increased, and the maximum value was 6–15 times that of the soil samples before improvement. Since the fine granule content of ZH was only 60%–70% those of the ZB and DH, the coarse granule content of the improved ZH was higher than those of ZB and DH after reaction with the same quantity of cement, and its bearing capacity was stronger. The CBR of the ZH soil with a cement content of 6% was 57.2% and 43.2% higher than those of ZB and DH, respectively. As shown in Figure 10B, as the cement content increased, the expansion rate of each soil sample decreased continuously. Before the cement content reached 6%, the expansion rate decreased significantly, indicating that a low cement content has a good effect on improving the water stability of the soil’s structure. Through analysis of the microstructure of the improved soils (Figure 11), it was found that there was a needle-like crystal connection structure in the microstructure of the sample after cement improvement, and the arrangement of the granules was dense. In the process of strengthening the soil sample, the complexity of the microstructure had a significant relationship with the strength of the soil sample, and the needle-like crystals improved the strength of the soil sample (Sides and Barden, 1970).
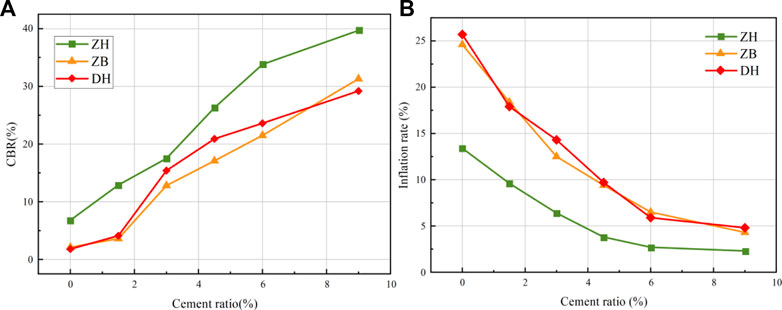
FIGURE 10. Influences of cement content on CBR and inflation rate. (A) The relationship between CBR and cement content. (B) The relationship between inflation and cement content.
4.5 Effect of moisture content on cement improvement
It can be seen from Figure 12 that before reaching the inflection point of the moisture content, the CBR values of ZH, ZB, and DH increased with increasing moisture content, while they decreased with increasing moisture content after exceeding the inflection point of the moisture content. The corresponding expansion rate decreased with increasing water content, tending to be stable after reaching the inflection point. In addition, the experiments revealed that the moisture content corresponding to the highest CBR values for ZH and ZB was Opt+2%. The CBR values corresponding to the optimal moisture contents of the three soil samples were not necessarily the highest. A more in-depth analysis of these phenomena is presented below.
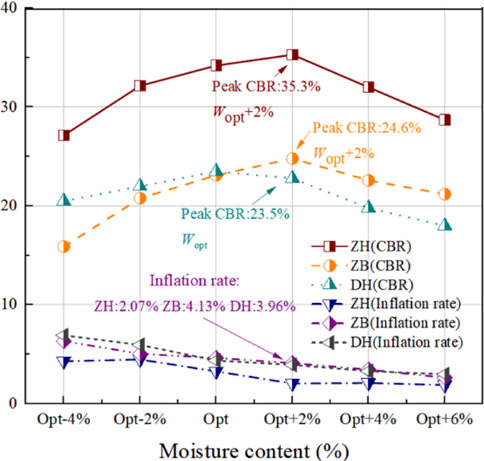
FIGURE 12. Comparison of outdoor CBR values and inflation rates under different moisture contents (6% cement).
The changes in the water content before and after immersion for ZH, ZB, and DH are shown in Figure 13. As the water content increased before immersion, the water content after immersion initially decreased and then increased slightly (the inflection point of the water content were Opt+1% ∼ Opt+2%), which are basically consistent with the water content corresponding to the CBR peak in Figure 12. When the moisture content was less than the inflection-point moisture content, the more water there was in the pores of the soil sample, the higher the efficiency of the hydration reaction with the cement was (red area in Figure 13). Moreover, the smaller the expansion was after soaking, the greater the CBR value of the soil sample was. At this time, the CBR value was affected by both the compactness and moisture content, and the compactness was positively correlated with the hydration reaction rate of the cement. When the moisture content before immersion exceeded the inflection point moisture content because the moisture content before immersion was still increasing, the degree of compaction of the soil samples gradually decreased, the expansion rate was slightly higher than before, and the hydration reaction efficiency decreased (blue area in Figure 13). At this time, the CBR value was mainly controlled by the moisture content before immersion.
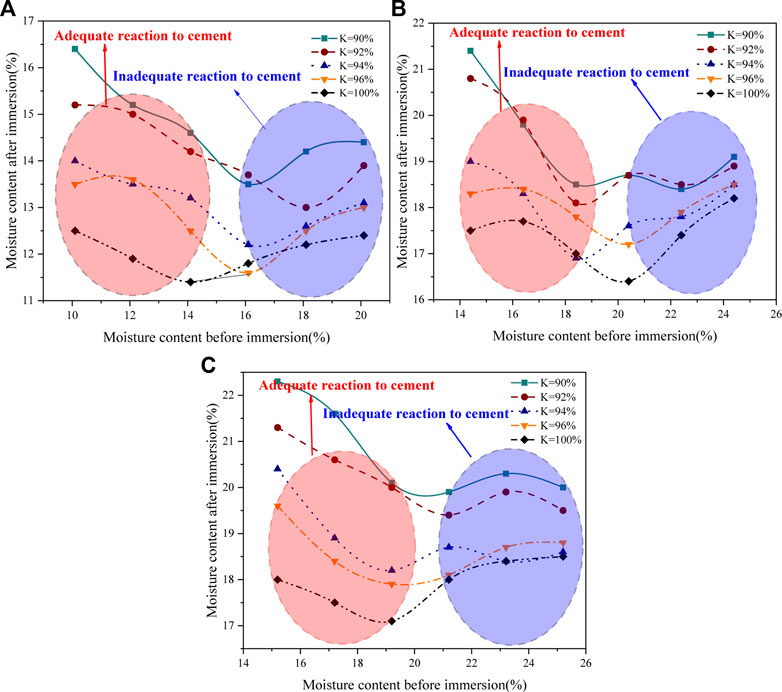
FIGURE 13. Changes in the moisture content of ZH, ZB, and DH (6% cement) before and after immersion under different compaction degrees in the CBR experiments. (A) ZH, (B) ZB, (C) DH.
Since CBR experiments required that the basaltic soil samples be immersed for 96 h when the moisture content of the samples before immersion exceeded the inflection point moisture content, the density of soil samples decreased, their compactness was insufficient, the number of coarse granules formed by the hydration reaction between the soil and cement decreased, and the strength of the skeletons was weakened, resulting in a slight increase in the moisture content after immersion. Therefore, an excessive water content before immersion will hinder the full contact between the cement and fine granules in the soil, and the efficiency of the hydration reaction will decrease. However, a low water content will also lead to an insufficient hydration reaction with the cement and insufficient coarse granules. These two situations are not conducive to improving the compactness of basaltic residual soil. As seen from Figure 13, the maximum moisture content of the reactions for the three soil samples containing cement should be at most Opt+6%, or the efficiency will decrease significantly.
4.6 Relationship between cement content and drying rate
In order to explore the relationship between the hydration reaction of the cement and the water loss in the soil, the water evolution of the ZH and ZB sample groups under different cement contents was compared through curing experiments. In view of the tropical monsoon climate in Leizhou Peninsula, the annual average temperature is 23.5°C, and the subgrade construction time is basically during the day and the temperature is slightly higher than that at night, the temperature is set at 27°C in the experiment. Figure 14 shows that the water loss from ZH and ZB was the fastest in the first 50 min of cement incorporation. The drying rate tended to become stable at 50–250 min, and the drying rate tended to be consistent with that of the soil samples without cement after 250 min. As can be seen from Figures 14A, B, the water content decreased by 2%–4% in the first 50 min after adding 6% cement, while the water contents of ZH and ZB decreased by only 1%–2% in the same time when cement was not added. This phenomenon indicates that adding cement increased the soil’s drying rate, and the cement ratio was positively correlated with the drying rate.
The above results show that when the water content of the basaltic residual soil in the construction site is high, the incorporation of a small amount of cement accelerates the evaporation of the water in the soil, so the optimum water content can be reached faster, which is conducive to the compaction of the soil. However, suppose the moisture content of the field soil is close to the optimum moisture content. In that case, compaction should be accomplished within 50 min of cement incorporation to avoid the accelerated drying rate caused by cement incorporation (i.e., the loss of a large amount of water before mechanical rolling) and lead to insufficient cement hydration reaction. In addition, the construction plan also needs to consider the influence of the field temperature on the soil moisture. Excessive temperature accelerates the evaporation of moisture, which can lead to the moisture in the soil sample being lower than the optimal moisture content. This is inconsistent with the design results.
Therefore, the water content corresponding to the highest CBR value of the soil is generally larger than the optimal water content in field measurement. It is suggested that cement mixing should be carried out when the water content of the soil sample is Opt+2% to obtain the optimum CBR.
4.7 Effect of cement content on failure mode of soil
Figure 15A shows the unconfined compressive strength of immersed and unimmersed DH samples with different cement contents. Where Un-S indicates unsoaked, and S-24 h indicates soaked for 24 h. The samples with 0% and 1.5% cement contents disintegrated after immersion, so they are not plotted in the figure. The results show that the cement treatment significantly improved the unconfined compressive strength of the basaltic residual soil, and the unconfined compressive strength of the soaked sample was slightly larger than that of the unsoaked sample by about 10%, indicating that the improved soil needs at least 1 day of sprinkler maintenance after construction. In addition, when the cement content was increased from 0% to 9%, the peak axial stress-strain range decreased from 4% to 0.5%, indicating that the increasing cement will gradually enhance the brittleness of the soil.
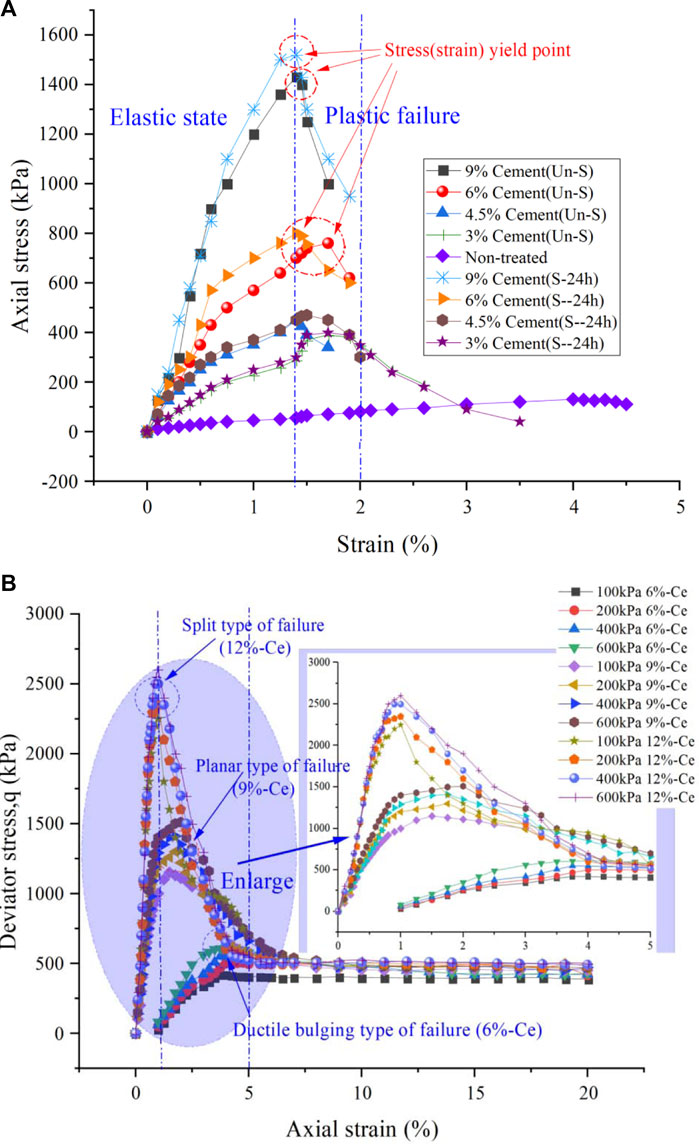
FIGURE 15. Effect of cement treatment on the strength properties. (A) Effect of cement treatment on the unconfined stress-strain behavior of DH. (B) Undrained triaxial behaviour of DH with the addition of 6%, 9%, and 12% cement.
The relationship between the deviatoric stress and axial strain for DH with cement contents of 6%, 9%, and 12% was studied. In Figure 15B, 6%–Ce denotes a cement content of 6%. The stress and strain of the soil samples with cement contents of 6% exhibited strain softening. The deviatoric stress increased with increasing confining pressure, and the peak value occurred when the strain was 4%. When the cement content increased from 6% to 9%, the strain of the soil peak value was 1.8%. (It should be noted that a cement content of 12% was tested in the experiment because the simulation of adding 9% cement to improve the DH basaltic residual soil had a 3% mechanical error for the maximum value, as shown in Table 7). When the cement content was 12%, the deviatoric stress of DH increased rapidly before the strain increment reached 1%, and it peaked at about 1% of the strain increment. The softening interval was about 0.1%, and then it decreased rapidly. The shear strength decreased by 70% when the strain increment was 1%–4%, which indicates that DH experienced significant splitting failure. This type of severe failure of the specimen under a deficient strain will instantly offset the effect of the cement treatment. Therefore, serious caution is needed when using a high proportion of cement to improve basaltic residual soil.

TABLE 7. Measurement of cement dosage in cement stabilized DH soil by using the EDTA method (6% cement).
4.8 Results of field mixing and rolling tests
According to the indoor test results, the CBR values of the modified ZH, ZB, and DH samples with cement contents of 6% were 33.8%, 21.5%, and 23.6%, respectively. In contrast, the CBR values of the modified ZH, ZB, and DH with cement contents of 3% were 17.5%, 12.8%, and 15.4%, respectively. Adding 3% cement is more economical and meets the requirements for subgrade filling.
EDTA titration, a method for rapidly determining the cement dosage in cement-stabilized soils in a site, is used to verify the uniformity of the mixing (Unit Highway Research Institute of the Ministry of Communic ations, 2009). As is shown in Table. 7, it was found in the field that the uniformity of the soil and cement mixing during cold recycling machine improvement is quite different from that in the laboratory. There is a ±3% error, which may occur after 3% cement is added (some soil cement contents were 0% and 6%) or after 6% cement is added (some of the soil contents were 3% and 9%). According to the CBR test results, the higher the cement ratio, the greater the strength of the improved soil. However, according to the results of the unconfined compressive strength tests and triaxial compression tests on the DH soil after a high proportion of cement improvement, a cement content of greater than 9% makes it easy for the instantaneous splitting failure of the soil to occur under a large load, which offsets the effect of the cement improvement (the maximum error is 12% after the addition of 9% cement to the soil). In summary, a cement content of 6% is recommended for improvement because the maximum cement content of 9% does not lead to large brittle soil failure.
The compaction of soils refers to the process of soil bulk density increasing and soil porosity decreasing under the action of external forces. The rolling equipment is a 12-ton vibrating single steel wheel roller (steel wheel diameter is 1400 mm). The compaction degree corresponding to the rolling times of the four soil samples in different moisture content is shown in Figure 17. The gradation of TH is good, and the maximum degree of compaction corresponding to the rolling times of plain soil at 3,6,9 are 91.1%, 94.3%, and 96.3%, respectively, and the recommended water content is Opt. ZH is improved by 3% cement, and the maximum degree of compaction corresponding to the rolling times of 3, 5, and 7 are 92.2%, 94.7%, and 96.1%, respectively, and the recommended water content is Opt+2%. The maximum high-pressure firmness of ZB and DH after 7 times rolling is 95.0% and 94.8%, and the recommended water content is Opt+1%.
According to the technical specifications for highway subgrade construction in China (Communications Second Highway Survey and Design Research Institute Co Ltd, 2015), the roads are classified as high-grade highways, secondary roads, and below-secondary roads. The lower the road grade, the lower the compactness required for each subgrade structure. The minimum compactness and strength scheme for subgrade fillers at different depths for each grade highway is shown in Figure 16. As shown in Figure 17, TH and ZH can use as high-grade roadbed filling materials, ZB and DH are only suitable for the part below the high-grade road bed or low-grade subgrade filling because their compaction degree cannot reach 96%.
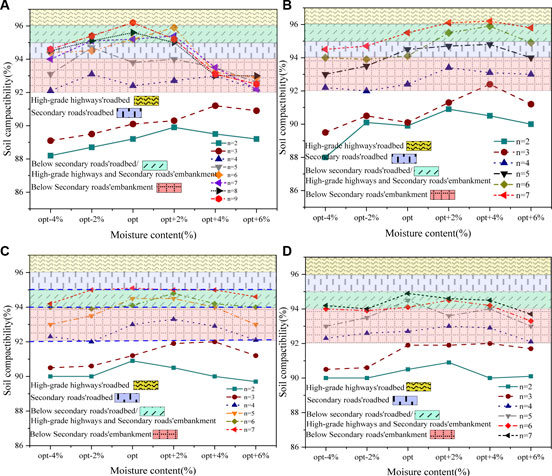
FIGURE 17. Application conditions for basaltic residual soil with different grain size compositions. (A) TH, (B) ZH, (C) ZB, (D) DH.
5 Discussion
Compared with soft soils, the physical properties of the highly sensitive basaltic residual soils (ZB and DH) (Yong and Silvestri, 1979) studied in this paper are similar, and they also have adverse engineering characteristics (such as a high fine granule content, weak bearing capacity, and high compressibility), a large void ratio, and high natural water content (the maximum natural water content found in the field is close to 40%) (Tavenas and Leroueil, 1980). Using cementitious material to improve soft soils is a mature technology with good effects and can be introduced into the research object of this paper. Since the basaltic residual soil in the Leizhou Peninsula is generally profoundly buried and the quasi preconsolidation pressure is higher than the adequate overlying pressure, its mechanical properties in its natural state are similar to those of super-compacted soil (with high shear strength). Soderman and Quigley (1965); Quigley (1966) also proved high shear strength characteristics through a large number of triaxial tests and microscopic observations. However, when the structure is destroyed, these mechanical properties weaken or completely disappear. This characteristic differs from the overpressure dense soil caused by unloading (Zhang, 1983).
According to the test results, the basaltic residual soil in the Leizhou Peninsula contains a lot of kaolinite, illite, and iron-aluminum oxide. Kaolinite easily absorbs water, swells, and shrinks. However, iron-aluminum oxide cement does not easily dissolve in water, and so the soil skeleton limits the above capabilities of kaolinite (Zhang et al., 2017; Zhang et al., 2016; Dexter et al., 1984; Kay and Dexter, 1992) found that repeated dry-wet cycles would promote the continuous generation of microcracks inside the soil, destroy the bond formed by free iron oxide in basaltic residual soils (Tuncer and Lohnes, 1977; Silva et al., 2020), weaken the stability of cement inside the soil, and provide a channel for the discharge of water inside the soil. Therefore, if there are some hard granules of moderate size, mechanical mixing can sufficiently disturb the soil, create a sufficient number of shear surfaces, completely destroy the cementation bond in the soil sample, transform small pores into large-medium pores (Horpibulsuk et al., 2006), improve the cemented soil into a loose state, increase its internal drainage channel, and accelerate the evaporation of water in the soil, which is conducive to the uniform distribution of soil and cement and other modifiers to improve its reaction efficiency.
In addition, it is difficult to realize the idea of using natural climate to impose repeated dry-wet cycles in the construction site to achieve the natural disintegration of the internal fracture of the soil. ZB and DH soil (high sensitivity and high plasticity soil) have a large volume, stable structure, and strong water locking ability, and their water evaporation efficiency is very low. Possible directions for future research include determining how to combine the mechanical (Figure 18A) and coarse-grained materials (Figure 18B) with high friction coefficient to mix them in the soil before sunning, how to completely destroy the bond formed by free iron oxide in the susceptible basaltic residual soil, and how to destroy the mutual cementation state in the soil so that the water can be fully evaporated, which not only reduces the error of cement content caused by machine mixing inhomogeneity but also does not cause the material cost to rise due to environmental destruction caused by adding coarse-grained materials.
6 Conclusion
In this study, the engineering characteristics of four basaltic residual soil with significantly different fine granule contents used in a large subgrade project were investigated, including the microstructure of the soil, chemical, and mineral compositions, workability, compaction characteristics, and curing characteristics when different proportions of cement were added. The changes in the unconfined compressive strength and the failure mode under consolidated undrained triaxial compression were analyzed. Based on the field mixing tests and rolling tests, suggestions for the use of basaltic residual soil with different granule size distributions in engineering projects were provided. The main conclusions of this study are as follows.
1) Fine granule content greatly influences the physical and mechanical properties of Leizhou Peninsula basalt residual soil, and the residual soil with fine granules of less than 55% is a good filling material for highway roadbeds.
2) The Leizhou Peninsula basaltic residual soil with fine granules of more than 85% has mechanical properties of high strength and low compressibility, as well as physical properties of high pore ratio, high liquid limit, and low natural density. These special physical and mechanical properties are related to the vast fine granules in their interior after long-term consolidation and bonding with iron oxide to form stable colloids. This undisturbed residual soil can be directly used as the lower embankment structure of the subgrade, but the disturbance will cause its strength to decrease greatly and require cement to improve.
3) The special colloidal state results in a cement uniformity error of ±3% when mechanical improvement of the Leiqiong residual soil with fine granule composition >85%. In order to ensure that the minimum cement content in the fluctuation range meets the strength requirements of the roadbed and the maximum does not cause severe brittle damage, the recommended cement content is 6%.
4) According to the experimental results of unconfined compressive strength, it is recommended that the residual soil be watered for 1 day after improvement to obtain higher strength.
5) The phenomenon that the moisture content corresponding to the peak CBR of the soil is slightly greater than its optimal water content is related to the error caused by the cement hydration reaction, outdoor temperature, and mechanical mixing time. It is recommended that the soil moisture content before improvement in high-temperature areas should be Opt+2%, and the cement improvement time should be controlled within 50 min.
6) In order to meet the requirements of high-grade road bed compaction of not less than 96%, the Leqiong basalt residual soil with fine granules less than 30% needs to be rolled 7–9 times, while the residual soil with fine granules of 30%∼60% needs to be improved by 3% cement and cycled 6∼7 times. Because the maximum compaction degree of residual soil (with fine granule greater than 85%) is less than 95%, it is only suitable for filling embankment structures below the highway’s roadbed.
Data availability statement
The original contributions presented in the study are included in the article/Supplementary Material, further inquiries can be directed to the corresponding author.
Author contributions
ZX: Conceptualization, Data curation, Formal Analysis, Investigation, Methodology, Software, Visualization, Writing–original draft, Writing–review and editing. LT: Funding acquisition, Project administration, Resources, Supervision, Validation, Writing–review and editing. YH: Data curation, Investigation, Methodology, Software, Writing–review and editing. QZ: Conceptualization, Data curation, Investigation, Methodology, Software, Writing–review and editing. FY: Data curation, Formal Analysis, Software, Writing–review and editing.
Funding
The author(s) declare financial support was received for the research, authorship, and/or publication of this article. This research was funded by the National Natural Science Foundation of China (grant number 42277142) and the Natural Science Foundation in Guangdong Province (grant number 2018B030311066).
Acknowledgments
We thank LetPub (www.letpub.com) for its linguistic assistance during the preparation of this manuscript.
Conflict of interest
Author QZ was employed by the company Guangxi Beitou Highway Construction and Investment Group Co., Ltd.
The remaining authors declare that the research was conducted in the absence of any commercial or financial relationships that could be construed as a potential conflict of interest.
Publisher’s note
All claims expressed in this article are solely those of the authors and do not necessarily represent those of their affiliated organizations, or those of the publisher, the editors and the reviewers. Any product that may be evaluated in this article, or claim that may be made by its manufacturer, is not guaranteed or endorsed by the publisher.
References
Abboud, M. M. (2022). Mechanical properties of cement-treated soils in relation to their use in embankment construction. Appl. Sci.
Abd Karim, N. A., Rashid, A. S. A., Noor, N. M., and Yaacob, H. (2014). Effect of cement stabilized kaolin subgrade on strength properties. J. Appl. Sci. 14 (8), 842–845. doi:10.3923/jas.2014.842.845
Amphibolite, D. U. N. E. (1985). Variability in the shear strength characteristics of an amphibolite derived laterite soil. Bull. Int. Assoc. Eng. Geol. - Bull. de l'Association Int. de Géologie de l'Ingénieur 32, 111–115. doi:10.1007/bf02594773
ASTM International (2011b). Astm D4767-11: standard test method for consolidated undrained triaxial compression test for cohesive soils. ASTM D4767-11.
ASTM International (2011c). Astm F1647-11: standard test methods for organic matter content of athletic field rootzone mixes. ASTM F1647-11.
ASTM International (2011a). D2435/D2435M-11: standard test methods for one-dimensional consolidation properties of soils using incremental loading. ASTM D2435/D2435M-11.
ASTM International (2017). D4318 - 17: standards,for liquid limit, plastic limit, and plasticity index of soils this c of soils. ASTM D 4318-17, 1–14.
Bhattacharja, S., and Bhatty, J. I. (2003). Comparative performance of portland cement and lime stabilization of moderate to high plasticity clay soils. Portl. Cem. Assoc. 125, 26. Available: www.cement.org.
Bishop and, W. A. (2015). The influence of progressive failure on the choice of the method of stability analysis. Géotechnique 21 (2), 168–172. doi:10.1680/geot.1971.21.2.168
Caner, L., Radtke, L. M., Vignol-Lelarge, M. L., Inda, A. V., Bortoluzzi, E. C., and Mexias, A. S. (2014). Basalt and rhyo-dacite weathering and soil clay formation under subtropical climate in southern Brazil. Geoderma 235–236, 100–112. doi:10.1016/j.geoderma.2014.06.024
Chen, D., Liao, Y., Jiang, C., and Feng, X. (2013). The mechanical properties of coastal soil treated with cement. J. Wuhan. Univ. Technol. Mater. Sci. Ed. 28 (6), 1155–1160. doi:10.1007/s11595-013-0836-9
Chen, H., and Wang, Q. (2006). The behaviour of organic matter in the process of soft soil stabilization using cement. Bull. Eng. Geol. Environ. 65 (4), 445–448. doi:10.1007/s10064-005-0030-1
Chen, Y., Tang, L. S., Ye, Y. H., Cheng, Z. H., and Zhou, Z. X. (2023). Effects of different chloride salts on granite residual soil: properties and water–soil chemical interaction mechanisms. J. Soils Sediments 23 (4), 1844–1856. doi:10.1007/s11368-023-03445-7
Communications Second Highway Survey and Design Research Institute Co Ltd (2015). JTG D30-2015, Wuhan, China. China: Specifications for Design of Highway Subgrades.
Consoli, N. C., da Fonseca, A. V., Cruz, R. C., and Silva, S. R. (2011). Voids/cement ratio controlling tensile strength of cement-treated soils. J. Geotech. Geoenvironmental Eng. 137 (11), 1126–1131. doi:10.1061/(asce)gt.1943-5606.0000524
Dexter, A. R., Kroesbergen, B., and Kuipers, H. (1984). Some mechanical properties of aggregates of top soils from the IJsselmeer polders. 2. Remoulded soil aggregates and the effects of wetting and drying cycles. Neth. J. Agric. Sci. 32 (3), 215–227. doi:10.18174/njas.v32i3.16898
Eze-Uzomaka, O. J., and Agbo, D. (2010). Suitability of quarry dust as improvement to cement stabilized-laterite for road bases. Electron. J. Geotech. Eng. 15, 1053–1066.
Garga, V. K. (1988). Effect of sample size on shear strength of basaltic residual soils. Can. Geotech. J. 25 (3), 478–487. doi:10.1139/t88-053
Gidigasu, M. D. (2012). Laterite soil engineering: pedogenesis and engineering. Amsterdam: Elsevier Scientific Publishing Co.,.
Gutierrez, N. H. M., de Nóbrega, M. T., and Vilar, O. M. (2009). Influence of the microstructure in the collapse of a residual clayey tropical soil. Bull. Eng. Geol. Environ. 68 (1), 107–116. doi:10.1007/s10064-008-0180-z
Horpibulsuk, S., Katkan, W., Sirilerdwattana, W., and Rachan, R. (2006). Strength development in cement stabilized low plasticity and coarse grained soils: laboratory and field study. Soils Found. 46 (3), 351–366. doi:10.3208/sandf.46.351
Ikhlef, N. S., Ghem_Ba_Za, M. S., and Dadouch, M. (2014). Effect of cement and compaction on the physicochemical behavior of a material in the region of sidi bel abbes. Eng. Technol. Appl. Sci. Res. 4 (4), 677–680. doi:10.48084/etasr.467
Kasim, A. M. F. (2003).Characterisation of Malaysian residual soils for geotechnical and construction engineering. Report, 283.
Kay, B. D., and Dexter, A. R. (1992). The influence of dispersible clay and wetting/drying cycles on the tensile strength of a red-brown earth. Soil Res. 30 (3), 297–310. doi:10.1071/sr9920297
Ladd, J., Moran, K., Kroon, D., Jarrard, R., Chen, M. P., Palmer-Julson, A., et al. (1993). Porosity variation and consolidation on the northeastern Australian margin. Proc. Sci. results, Odp. Leg. 133, 617–623. doi:10.2973/odp.proc.sr.133.269.1993
Lasisi, F., and Ogunjide, A. M. (1984). Effect of grain size on the strength characteristics of cement-stabilized lateritic soils. Build. Environ. 19 (1), 49–54. doi:10.1016/0360-1323(84)90013-1
Lee, F. H., Lee, Y., Chew, S. H., and Yong, K. Y. (2005). Strength and modulus of marine clay-cement mixes. J. Geotech. Geoenvironmental Eng. 131 (2), 178–186. doi:10.1061/(asce)1090-0241(2005)131:2(178)
Leshchinsky, D., Yu, H. S., Salgado, R., Sloan, S. W., and Kim, J. M. (1999). Limit analysis versus limit equilibrium for slope stability. J. Geotech. Geoenvironmental Eng. 125 (10), 914–918. doi:10.1061/(asce)1090-0241(1999)125:10(914)
Mengue, E., Mroueh, H., Lancelot, L., and Eko, R. M. (2017). Mechanical improvement of a fine-grained lateritic soil treated with cement for use in road construction. J. Mater. Civ. Eng. 29 (11), 4017206. doi:10.1061/(asce)mt.1943-5533.0002059
Oluwatuyi, O. E., Adeola, B. O., Alhassan, E. A., Nnochiri, E. S., Modupe, A. E., Elemile, O. O., et al. (2018). Ameliorating effect of milled eggshell on cement stabilized lateritic soil for highway construction. Case Stud. Constr. Mater. 9, e00191. doi:10.1016/j.cscm.2018.e00191
Oluwatuyi, O. E., Ashaka, E. C., and Ojuri, O. O. (2019). Cement stabilization treatment of lead and naphthalene contaminated lateritic soils. J. Environ. Eng. Landsc. Manag. 27, 41–48. doi:10.3846/jeelm.2019.7778
Oluwatuyi, O. E., Ojuri, O. O., and Khoshghalb, A. (2020). Cement-lime stabilization of crude oil contaminated kaolin clay. J. Rock Mech. Geotechnical Eng. 12 (1), 160–167. doi:10.1016/j.jrmge.2019.07.010
Osula, D. O. A. (1996). A comparative evaluation of cement and lime modification of laterite. Eng. Geol. 42, 71–81. doi:10.1016/0013-7952(95)00067-4
Quigley, R. M. (1966). The fabric of anisotropically consolidated sensitive marine clay. Can. Geotech. J. 3, 242–245. doi:10.1139/t66-029
Rahman, Z. A., Hamzah, U., Taha, M. R., Ithnain, N. S., and Ahmad, N. (2010). Influence of oil contamination on geotechnical properties of basaltic residual soil. Am. J. Appl. Sci. 7 (7), 941–948. doi:10.3844/ajassp.2010.954.961
Raihan Taha, M., Mofiz, S. A., and Kamal Hossain, M. (2000). Stress-strain behaviour of compacted residual soil in direct shear test. ISRM Int. Symp., 2018.
Rigo, M. L., Pinheiro, R. J. B., Bressani, L. A., Bica, A. V. D., and da Silveira, R. M. (2006). The residual shear strength of tropical soils. Can. Geotech. J. 43 (4), 431–447. doi:10.1139/t06-015
Sariosseiri, F., and Muhunthan, B. (2009). Effect of cement treatment on geotechnical properties of some Washington State soils. Eng. Geol. 104 (1–2), 119–125. doi:10.1016/j.enggeo.2008.09.003
Schwertmann, U. (1993). Relations between iron oxides, soil color, and soil formation. Soil Color 31, 51–69.
Sides, G., and Barden, L. (1970). The microstructure of dispersed and flocculated samples of kaolinite, illite, and montmorillonite. Can. Geotechnical J. 8, 391–399. doi:10.1139/t71-041
Silva, L. S., Marques Júnior, J., Barrón, V., Gomes, R. P., Teixeira, D. D. B., Siqueira, D. S., et al. (2020). Spatial variability of iron oxides in soils from Brazilian sandstone and basalt. Catena 185, 104258. doi:10.1016/j.catena.2019.104258
Soderman, L. G., and Quigley, R. M. (1965). Geotechnical properties of three ontario clays. Can. Geotech. J. 2 (2), 167–189. doi:10.1139/t65-023
Tang, J., Yu, P., Wei, Z., and Meng, Q. (2011). Experimental study on disintegration characteristics of residual basalt soil in Guizhou. J. Eng. Geol. 19 (5), 778–783.
Tavenas, F., and Leroueil, S. (1980). The behavior of embankments on clay foundations. Can. Geotech. J. 17 (2), 236–260. doi:10.1139/t80-025
Tuncer, E. R., and Lohnes, R. A. (1977). An engineering classification for certain basalt-derived lateritic soils. Eng. Geol. 11 (4), 319–339. doi:10.1016/0013-7952(77)90037-0
Uddin, D. T., Balasubramaniam, K., and Bergardo, A. S. (1997). Engineering behavior of cement- treated bangkok so clay. Geotech Eng. 28 (1), 89–119.
Unit Highway Research Institute of the Ministry of Communications (2009). “Inorganic binder stable material test procedures for highway engineering: jtg E51-2009,” in Inorganic binder stable material test procedures for highway engineering (JTG).
Veloso Marques, S. F., Consoli, N. C., and Almeida e Sousa, J. (2014). Testing cement improved residual soil layers. J. Mater. Civ. Eng. 26 (3), 544–550. doi:10.1061/(asce)mt.1943-5533.0000725
Venkatarama Reddy, B. V., and Latha, M. S. (2014). Influence of soil grading on the characteristics of cement stabilised soil compacts. Mater. Struct. Constr. 47 (10), 1633–1645. doi:10.1617/s11527-013-0142-1
Wang, F., Li, K., and Liu, Y. (2022). Optimal water-cement ratio of cement-stabilized soil. Constr. Build. Mater. 320, 126211. doi:10.1016/j.conbuildmat.2021.126211
yan Meng, F., peng Chen, R., and Kang, X. (2018). Effects of tunneling-induced soil disturbance on the post-construction settlement in structured soft soils. Tunn. Undergr. Sp. Technol. 80, 53–63. doi:10.1016/j.tust.2018.06.007
Yang, Y., Wang, G., Xie, S., Tu, X., and Huang, X. (2013). Effect of mechanical property of cemented soil under the different pH value. Appl. Clay Sci. 79, 19–24. doi:10.1016/j.clay.2013.02.014
Yong, R. N., and Silvestri, V. (1979). Anisotropic behaviour of a sensitive clay. Can. Geotech. J. 16 (2), 335–350. doi:10.1139/t79-033
Zhang, C. (1983). The mechanical characteristics of two kinds of structural clay (in Chinese). J. Waterw. Transp. Sci. 04 (65–1).
Zhang, X. W., Kong, L. W., Cui, X. L., and Yin, S. (2016). Occurrence characteristics of free iron oxides in soil microstructure: evidence from XRD, SEM and EDS. Bull. Eng. Geol. Environ. 75 (4), 1493–1503. doi:10.1007/s10064-015-0781-2
Zhang, X. W., Kong, L. W., Yin, S., and Chen, C. (2017). Engineering geology of basaltic residual soil in Leiqiong, southern China. Eng. Geol. 220, 196–207. doi:10.1016/J.ENGGEO.2017.02.002
Keywords: subgrade engineering, basaltic residual soil, iron oxide, cement improvement, moisture content, degree of compaction
Citation: Xie Z, Tang L, He Y, Zhong Q and Yang F (2023) Subgrade engineering characteristics of basaltic residual soil in Leizhou Peninsula, southern China. Front. Earth Sci. 11:1266219. doi: 10.3389/feart.2023.1266219
Received: 24 July 2023; Accepted: 29 August 2023;
Published: 16 October 2023.
Edited by:
Dongri Song, Chinese Academy of Sciences (CAS), ChinaReviewed by:
Opeyemi Emmanuel Oluwatuyi, University of Wyoming, United StatesXianwei Zhang, Chinese Academy of Sciences (CAS), China
Copyright © 2023 Xie, Tang, He, Zhong and Yang. This is an open-access article distributed under the terms of the Creative Commons Attribution License (CC BY). The use, distribution or reproduction in other forums is permitted, provided the original author(s) and the copyright owner(s) are credited and that the original publication in this journal is cited, in accordance with accepted academic practice. No use, distribution or reproduction is permitted which does not comply with these terms.
*Correspondence: Liansheng Tang, ZWVzdGxzQG1haWwuc3lzdS5lZHUuY24=