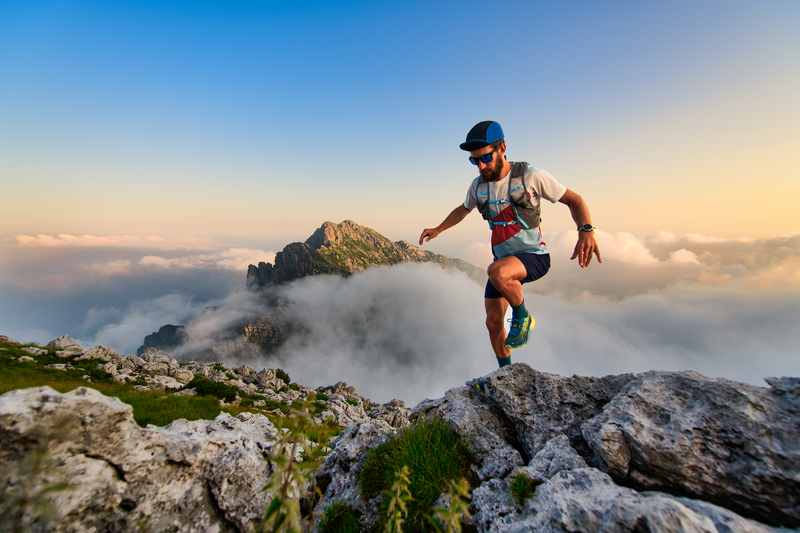
95% of researchers rate our articles as excellent or good
Learn more about the work of our research integrity team to safeguard the quality of each article we publish.
Find out more
ORIGINAL RESEARCH article
Front. Earth Sci. , 26 September 2023
Sec. Solid Earth Geophysics
Volume 11 - 2023 | https://doi.org/10.3389/feart.2023.1260559
This article is part of the Research Topic From Preparation to Faulting: Multidisciplinary Investigations on Earthquake Processes, volume II View all 16 articles
Geochemical composition changes in groundwater related to earthquakes have been documented in previous studies, and most such studies focused on the changes in major ions, hydrogen, oxygen isotopes, and geochemical gases. Changes in trace elements were suggested to be more sensitive to small earthquakes than the commonly used chemical constituents such as major ions, yet they received less attention. Beijing is located in the Zhangjiakou-Bohai seismic belt and experiences frequent occurrences of small earthquakes. In this paper, we collected groundwater samples from a hot spring in Yanqing district of Beijing weekly from August 2021 to August 2022. Each water sample contained 41 trace chemical compositions. During the sampling, an earthquake with a magnitude of ML3.3 occurred in the Chaoyang district of Beijing on 3 February 2022, so these trace elements changes were systematically monitored before and after the earthquake: Li, Sc, Ti, and Pb elements had upward changes before the earthquake, while Cu, Nb, Th, Zn, Tl, and U elements had downward changes before the earthquake. Eu (rare earth elements) had upward changes after the earthquake. At the same time, the earthquake caused no significant changes in the groundwater level in the seismic monitoring well near the Songshan spring. Such responses indicate that trace elements are likely to be more sensitive to crustal strain than groundwater level. We considered that the earthquake-induced rock cracks before or after the earthquake caused enhancing water-rock interaction and led to the migration of trace elements between the water column and rocks, which is the mechanism to explain the trace elemental changes. This study probably provides a comprehensive assessment of the sensitivity of trace element constituents to the earthquake. Furthermore, we suggest that more long-term continuous monitoring and research of trace elements in Beijing and Zhangjiakou-Bohai Fault Zone should be considered to explore the response mechanism of groundwater geochemistry to earthquakes in the future.
As part of the earthquake precursor detection program, changes in groundwater levels, temperatures, and chemical composition related to earthquakes have been documented for decades (Toutain et al., 1997; Roeloffs, 1998; Montgomery and Manga, 2003; Wang and Manga, 2010; Skelton et al., 2014; Manga and Wang, 2015; De Luca et al., 2018; Martinelli, 2020). Because of the feasibility of using automated monitoring systems, earthquake-related changes in water levels, temperatures, and radon concentrations are the most commonly reported responses (King, 1981; Kitagawa et al., 1996; Manga and Wang, 2015; Martinelli, 2020). Hence, these monitoring methods of groundwater also play key roles in precursor detection in China, and a nationwide network of monitoring wells located along strain-sensitive locations has been constructed for this purpose.
In contrast, groundwater geochemical changes induced by earthquakes have been documented relatively rarely (Martinelli, 2020; Shi et al., 2020) because manual sampling and expensive, time-consuming laboratory analyses are required (Wang and Manga, 2010). However, such studies are highly important because earthquake-related chemical changes are considered to be sensitive to crustal stress and to be beneficial for earthquake prediction (Thomas, 1988; Wakita, 1996; Poitrasson et al., 1999; Manga and Wang, 2015; Martinelli and Dadomo, 2017; Shi et al., 2020; Kopylova et al., 2022). For instance, based in part on hundreds of hydrological anomalies including geochemical changes, an imminent prediction was made before the 1975 M 7.3 Haicheng earthquake in China (Wang et al., 2006). Following the 1995 M7.2 Kobe earthquake, several papers reported precursory changes in the concentrations of radon, chlorine, and sulfate ions in groundwater (Igarashi et al., 1995; Igarashi and Wakita, 1995; Tsunogai and Wakita, 1995). Claesson et al. (2004) observed simultaneous changes in trace elements, major elements, and isotopes before and after major earthquakes. Barberio et al. (2017) and Rosen et al. (2018) found that some trace elements exhibited responses to the seismic sequence in central Italy. Skelton et al. (2019) found that changes in major ions and hydrogen and oxygen isotopes were associated with three different earthquakes in Iceland. Shi et al. (2020) found that trace element concentrations showed coseismic decreases following the Tonghai earthquake in the Yunnan Province of China, but no significant changes occurred in major ions and hydrogen and oxygen isotope concentrations.
Overall, according to previous geochemical research, most documented studies investigated the major elements (Tsunogai and Wakita, 1995; Toutain et al., 1997; Kingsley et al., 2001; Woith et al., 2013) and hydrogen and oxygen isotopes related to destructive great earthquakes (Taran et al., 2005; Skelton et al., 2019), and only a few studies focused on trace elements’ responses to the earthquake (Rosen et al., 2018; Shi et al., 2020) despite changes in trace elements being more sensitive to small earthquakes than the commonly used chemical constituents. In this paper, we continuously collected 36 water samples of the hot spring from August 2021 to August 2022, and each water sample contained 41 trace elements which contributed to the comparison of the different responses to earthquakes and the discussion on the possible mechanisms and their implications for future seismic monitoring.
North China has experienced a period of strong earthquakes frequently over the last few decades. From 1966 to 1998, a series of strong earthquakes occurred, such as the Xingtai M7.2 earthquake in 1966, the Bohai M7.4 earthquake in 1969, the Haicheng M7.3 earthquake in 1975, and the Tangshan M7.8 earthquake in 1976. Since the Zhangbei M6.2 earthquake in 1998, there has been no earthquake above M6 for 20 years. The seismic activity in North China has been relatively quiet, and there would be a potential danger of damaging earthquakes in the next few years.
Zhangjiakou-Bohai Fault Zone is a group of NW-W orderly active fault zones with high seismic activity in North China; the fault zone starting from the northern margin of Taihang Mountain in the west is distributed along the junction area of Yanshan Mountain and the North China Basin and enters the Bohai Sea in the east. In this zone, many violent earthquakes above M6 have occurred (Yang et al., 2022), so it is an important fault zone for earthquake monitoring and research.
Beijing is the capital of China with a large population, and it is located at the intersection of the North China Plain and Zhangjiakou-Bohai seismic belt, where active faults have developed. These faults mainly included the NW orderly Nankou-Sunhe Fault Zone and two groups of NE orderly active fault zones named Huangzhuang-Gaoliying Fault Zone and Shunyi-Liangxiang Fault Zone. All earthquakes occurred along these fault zones or where they intersect (Figure 1), and all these earthquakes were less than M6. Therefore, it is of great scientific and practical significance to carry out small earthquake monitoring and forecasting in the Beijing area.
FIGURE 1. Earthquake distribution of the Beijing area from August 2021 to August 2022 and the time sequence of earthquakes above M1.0. The biggest yellow circle is the Chaoyang ML3.3 earthquake, and the yellow and green triangles are the locations of the Songshan hot spring and Wuliying monitoring well, respectively.
To better monitor the seismic activity in the capital area, the Chinese Earthquake Administration (CEA) started to deploy the Beijing Metropolitan Digital Seismic Network (BSN) in the late 90s. Currently, the network consists of broadband, borehole, and surface short-period stations that cover Beijing and the surrounding areas densely and uniformly (Jiang et al., 2008; Li and Huang, 2014). Since 2001, 107 stations have been installed in the Chinese capital region, which is the most advanced and densest digital seismic network in Mainland China. A large number of high-quality waveforms have been recorded, which provides an opportunity to improve the location accuracy of small earthquakes in the region (Jiang et al., 2008).
Beijing hosted the 2022 Winter Olympic Games from February 4th to 20th. During this time, continuous monitoring of possible earthquake areas was strengthened. On 3 February 2022, an earthquake with a magnitude of ML3.3 occurred in the Chaoyang district of Beijing (Figure 1). In this study, we conducted the geochemical monitoring study of the Songshan hot spring located in the Yanqing district of Beijing; the spring had an epicentral distance of ∼75 km from the Chaoyang earthquake (Figure 1). Geographically, this spring is exposed to the valley of Dahaituo Mountain which is composed of granite with fissure development (Yang et al., 2001), and fault activities were good channels for deep groundwater migration. The groundwater of Songshan spring comes from the granite of Dahaituo Mountain; there is no lake, river, or surface reservoir in the area.
Songshan Spring is an artesian type of rising spring, and the water is from the granite rock body. The spring has 41°C–42°C water temperature, high fluoride content (15.93 mg/L), and high silica content (54 mg/L); the water type is Na-SO4 (Yang et al., 2001). The main observation items of Songshan Spring were water mercury and there were high correspondences between mercury anomalies and earthquakes (Yang et al., 2001).
In addition, a seismic groundwater monitoring well named Wuliying is ∼10 km near the Songshan spring. The Wuliying well is located on the subsidence transition zone at the northern edge of the Yanqing Basin, with compressive faults developing around the well area (Gao and Xing, 2011). The groundwater level observation is one of the items of the Wuliying well and is usually recorded at 1-min intervals by a SWY-2 digital instrument developed and produced by the Institute of Crustal Dynamics, China Earthquake Administration. The water-level sensor has a range of 0–50 m, resolution of 1 mm, sampling interval of 1 s, precision of 0.02 percent, and accuracy of 0.05 percent.
Since the Songshan spring belongs to the Yanqing District Earthquake Agency, water samples from the mouth of the fresh spring were collected by the staff working at this station every 7–12 days; the sample collection was from August 2021 to August 2022, and a total of 36 water samples were collected over the monitored period. Each sample was stored in a 200 mL polyethylene bottle. All samples were sent monthly to the Beijing Research Institute of Uranium Geology for 41 trace element constituents analysis, including Li, Ti, Pb, Sc, Ni, Cu, Nb, Th, Zn, Tl, U, Ga, Mo, Ba, Rb, Cs, Sr, Be, Mn, Cr, Co, Y, and Bi as well as rare earth elements (La, Ce, Pr, Nd, Sm, Eu, Gd, Tb, Dy, Y, Ho, Er, Tm, Yb, and Lu) (Supplementary Material,). These trace elements’ concentrations were analyzed with the same instrument (An ELEMENT XR mass spectrometer) and the same staff for accuracy. The analytical precision is better than ±2%.
We use 41 trace elemental composition data to calculate a Pearson correlation matrix (r values) that can quantitatively indicate the correlation between each element. The correlation matrix was a visual network by R program to check geochemical elemental clustering and grouping (Figure 4). In the network, each geochemical element is a node and each correlation is an edge in which the width of the edges according to the magnitude of the correlation and the placement of the nodes is a function of the pattern of correlations (Epskamp et al., 2012). This means that stronger correlations have shorter and wider edges and closer placement of nodes (Chen et al., 2021).
Data smoothing methods include LOWESS, rLOWESS, LOESS, and rLOESS using Matlab (Chen, 2023). The LOWESS (Locally Weighted Scatterplot Smoothing) means local regression using weighted linear least squares and a first-degree polynomial model. The rLOWESS means a robust version of LOWESS that assigns lower weight to outliers in the regression. The LOESS means local regression using weighted linear least squares and a second-degree polynomial model. The rLOESS means a robust version of LOESS.
Songshan spring has no water level monitoring; we checked water level changes in the Wuliying well near the spring for hydrological responses to earthquakes. The groundwater level in the Wuliying well continuously increased from 1.3 to 1.8 m between July 2021 and August 2021 (Figure 2). No abnormal changes in groundwater level were found related to any earthquakes (Figure 2). The water level change also has no relationship to precipitation as there is only large precipitation during summer (from June to August every year).
FIGURE 2. Precipitation (A) and water level changes (B) in the Wuliying well which is ∼10 km away from the Songshan hot spring. The blue vertical line shows the time of the Chaoyang ML3.3 earthquake.
The results of analyses of the 36 water samples are listed in the Supplementary Material, (Concentrations of 41 trace elements of each water sample in the Songshan spring). The PAAS-normalized 15 rare earth elements (REE) display remarkable positive Eu anomalies and flat distribution patterns (Figure 3A). Eu concentrations showed a significant increase from 0.002 to 0.007 mg/L from February 2022 to August 2022 (the end of sampling in August) (Figure 3B). These changes were more evident when data smoothing using the mathematic method (rLOWESS, robust Locally Weighted Scatterplot Smoothing) (Figure 3C). Eu had a marked increase after early February.
FIGURE 3. PAAS shale-normalized REE patterns (A) and variation of Eu element during the study period (B,C). The blue vertical line shows the time of the Chaoyang ML3.3 earthquake.
Apart from REE, the other 26 trace elements show different changes over the monitored period. Based on element correlative clustering, these elements’ constitutes can be broadly classified into four groups (Figure 4). The first group consists of Li, Sc, Ti, and Pb elements which cluster each other in the correlation network (pink circles in Figure 4). The second group consists of Cu, Nb, Th, Zn, Tl, and U elements clustering in grey circles in Figure 4. The third group consists of Ga, Mo, Ba, Rb, Cs, and Sr elements clustering in green circles in Figure 4. The fourth group consists of Be, Mn, Cr, Co, Y, Bi, Cd, Sb, Ni, and V elements dispersing in the correlation network (white circles in Figure 4).
FIGURE 4. Element correlation matrix (A) and Elemental correlation cluster (B). Each circle in (A) represents the strength of element correlation; the larger the circle, the stronger the correlation and the box shows that the correlation has statistical significance. Network in (B) structures based on Pearson correlation matrix of geochemical data. Each geochemical element is a node and each correlation is an edge. Stronger correlations have shorter and wider edges and closer placement of nodes.
The elements of the first group had upward changes (Figure 5): Li, Sc, Ti, and Pb concentrations continued to rise from December 2021 to February 2022 and peak, then remained stable at high values from March to May 2022, and decreased from June to August 2022 (several months after the earthquake). These changes were quite marked when using the smoothing methods (LOWESS, rLOWESS, LOESS, and rLOESS) (Figure 5).
FIGURE 5. Variation of the first group of Li, Sc, Ti, and Pb during the study period (The black line indicates raw data. The green line, blue dotted line, red dashed line, and purple dashed line indicate the LOWESS, rLOWESS, LOESS, and rLOESS fitting methods, respectively). The blue vertical line shows the time of the Chaoyang ML3.3 earthquake.
The elements of the second group had significant downward changes (Figure 6): Cu, Nb, Th, Zn, and Tl contents continued to decline from August 2021 to January 2022, then remained stable at minimum values from February to August 2022 (Figure 6). U contents declined from August 2021 to October 2021, then maintained stable values from November 2021 to February 2022, and the last decreased from March to August 2022 (Figure 6).
FIGURE 6. Variation of the second group of Cu, Nb, Th, Zn, Tl, and U during the study period. The blue vertical line shows the time of the Chaoyang ML3.3 earthquake.
The elements of the third group had upward and then downward changes (up-convex shape) (Figure 7): elemental concentrations continued to rise from August 2021 to January 2022 and peaked, then remained stable at high values from February to April 2022, and the last decreased from May to August 2022 (Figure 7).
The elements of the fourth group had no regular changes, and the relationship with earthquakes was not clear.
Chaoyang ML3.3 earthquake was an epicentral distance of ∼75 km from Songshan hot spring (Figure 1). The seismic energy density produced by this earthquake at the Songshan hot spring was ∼0.0019 J/m3 (calculation according to the method by Wang, 2007), which is the same order of magnitude with the minimum energy density (10–4 J/m3) that was required to trigger hydrological responses based on global observations (Wang, 2007). However, there were no changes in the groundwater level of the Wuliying well near the Songshan spring groundwater level to the Chaoyang earthquake, which means that the Chaoyang ML3.3 earthquake might have been too small to cause the hydrological responses of Songshan spring, and no additional water into the wellbore could be expected.
According to the above results, the changes in trace elements have a strong temporal correlation with earthquakes, which means that trace elements were probably sensitive to the small earthquake, but different kinds of trace elements have particular responses to the Chaoyang earthquake, for instance, the Eu element of rare earth elements had obvious upward changes after the Chaoyang earthquake. The first group elements’ (Li, Sc, Ti, and Pb) concentrations had upward changes 2 months before the Chaoyang earthquake, then returned to their initial concentration a few months after the earthquake. The second group elements’ (Cu, Nb, Th, Zn, Tl, and U) concentrations had downward changes a few months before the Chaoyang earthquake, then maintained stable minimum values until the end of sampling.
What mechanism could explain our observation? The trace elements were not commonly measured in the previous geochemical studies, and only a very limited number of cases could be found (Shi et al., 2020). Many previous studies have documented co-seismic groundwater level changes following earthquakes and have proposed several mechanisms to explain the responses, such as co-seismic static strain-induced dilation and compaction (Ge and Stover, 2000), liquefication or consolidation of sediments (Wang et al., 2001), and permeability changes caused by shaking (Brodsky et al., 2003). However, mechanism explanations for geochemical anomalies are relatively rare and based on co-seismic geochemical changes following the earthquake (Claesson et al., 2007; Shi et al., 2020). For instance, a great part of the geochemical variations in the chemical composition of groundwaters was attributed to aquifer mixing processes (Thomas, 1988). Geochemical gases involved in the Earth’s degassing activity are considered responsible for water-gas-rock interaction processes able to induce chemical variations in groundwater composition (King, 1986; Martinelli, 2015). About all geochemical anomalies have been directly or indirectly attributable to deep fluid pressure variations induced by crustal deformative processes since fluid pressure is proportional to stress and volumetric strain (Petrini et al., 2012). In this study, we have examined many trace element constituents’ responses to the earthquake in relatively high sample rates and found different constituents obviously increased or decreased changes before or after the Chaoyang earthquake. All trace elements’ changes were not co-seismic, so previous mechanisms' explanations might not have been applied to changes in trace elements of our observation.
We considered the previously proposed mechanisms for changes in chemical elements (Claesson et al., 2007; Rosen et al., 2018; Shi et al., 2020). Favara et al. (2001) collected geochemical compositions (Ca, Cl, and SO4) at three thermal springs in the Umbria region of the Central Apennines (Italy) seismic swarm from 1997 to 1998. The authors suggested that the recorded variations actually seem to have been induced by permeability variations related to crustal deformation in the absence of elastic energy release. According to Claesson et al. (2007), the increase in reactive surface area would lead to more extensive water-rock interaction, and increases in concentrations of some dissolved cations would be expected. Rosen et al. (2018) proposed that the changes in trace elements were likely related to the release of groundwater of different residence times from pore spaces or fractures. Shi et al. (2020) suggested that the changes in trace elements were the combined result of the addition of small amounts of water from the reservoirs isolated by the hydraulic barrier and the changes in the physical-chemical environment following the earthquake.
In our study, the Wuliying well near Songshan spring had no groundwater level changes in our case, which indicates that there were no large volumes of chemically distinct groundwater entering the spring and mixing with the original water, so the mechanism of co-seismic strain and permeability changes were excluded. The mechanism of extensive water-rock interaction most likely explained the seismic responses in the Songshan spring as follows (Figure 8).
FIGURE 8. Concept model of earthquake-induced trace elements changes in Songshan spring. Microcracks led to intensive water-rock interaction before the earthquake, and Li, Sc, Ti, and Pb were released into water, and Cu, Nb, Th, Zn, Tl, and U adsorbed on the surface of rocks. After the earthquake, Eu (REE) was released into the water.
The Eu element of rare earth elements had obvious upward changes after the Chaoyang earthquake, and the upward pattern of changes was consistent with previous examples. For instance, following the Loma Prieta earthquake in 1989, major ion chemistries (Na, K, Ca, Mg, Cl, SO4, and HCO3) in stream water showed a marked increase in ionic strength, together with the changes in the excess stream discharge (Rojstaczer and Wolf, 1992). Rojstaczer and Wolf (1992) suggested that the additional stream discharge following the Loma Prieta earthquake was derived from groundwater from the surrounding highlands of the drainage basin.
In our case, Songshan spring displayed remarkable positive Eu anomalies of REE (3A), which coincided with petrological evidence because the bedrock of Songshan spring is granite containing a large amount of feldspar minerals, which commonly became Eu-enriched during crystallization owing to the ready substitution of Eu2+ for Ca2+ (Taylor and McLennan, 1985; Chen et al., 2021). Eu concentrations showed obvious upward changes before the Chaoyang earthquake. The most likely mechanism was intensive water-rock interaction. The probable scenario was that rock ruptures after the earthquake increased the reactive surface area between rocks and groundwater, exposing more feldspar minerals to water, and leading to increased Eu concentrations in the water column (Figure 8).
The first group of Li, Sc, Ti, and Pb elements increased before the earthquake then decreased significantly over a period of several months after the earthquake until it approached the pre-earthquake conditions. The type of changes were consistent with the previous few cases. For instance, before the 1995 M 7.2 Kobe earthquake, chloride concentration gradually increased (Tsunogai and Wakita, 1995). The chloride changes were considered to be consistent with a ‘drastic’ increase in strain (Tsunogai and Wakita, 1995). The mechanism of extensive water-rock interaction can also explain the response that the first group elements rise rapidly before the earthquake.
Songshan spring came from granite, which is rich in these elements, for instance, Li is a typical lithophile and often enriched in mica and other silicate minerals and easy to hydrolyze. Sc has a very high partition coefficient in granites, Ti is high content in quartz, and Pb can replace K+ and Ca2+ in rock-forming minerals with isomorphism (Mou, 1999). Hence, the most likely scenario was that local stresses might create granite microcracks before the earthquake, exposing more fresh minerals to water, enhancing water-rock interaction, releasing more elements into the water column, and causing the rise response (Figure 8). Following the earthquake, microcracks may merge to form large-scale ruptures (Lockner and Beeler, 2002), the elements would accelerate into the water column, and the element concentrations would further rise until saturation. Several months after the earthquake, the stress would be redistributed, the fractures would no longer develop or partially fault-reseal (Claesson et al., 2007), and the elemental contents in the water column would decline.
The second group of Cu, Nb, Th, Zn, Tl, and U elements concentrations had downward changes during a few months before the Chaoyang earthquake, then maintained stable minimum values until the end of sampling, and the pattern of changes was similar to the previous few examples. Claesson et al. (2004) reported pre-seismic rapid decreasing anomalies in the concentrations of Cr and Fe before the Mw 5.8 earthquake on 16 September 2002. The authors interpreted that the earthquake ruptured a hydrological barrier, permitting a rapid influx of water from other aquifers.
In our case, the second group elements declined before the earthquake with no addition of distinct additional water. Thus, the most likely mechanism was also intensive water-rock interaction. The pattern of falling changes in the second group elements was completely contrary to the pattern of rising changes in the first group elements. Because second group elements have large proton numbers and mass, and the elements with large mass mostly exist in minerals by the mechanism of adsorption (Mou, 1999), enhanced rock ruptures would result in the increase of the mineral surface area, and the increase of these elements adsorbed on the surface of minerals, leading to the decrease of the concentration of these elements in the water column (Figure 8). Due to the different adsorption and desorption capacity of different elements, the decline rate was inconsistent (Figure 6). These elements’ concentrations decreased in groundwater further until the element contents were saturated on the rock surface.
The third group of Ga, Mo, Ba, Rb, Cs, and Sr elements had upward and then downward changes (up-convex shape), and these elements were likely to be related to the season but not to the earthquake. These elements are closely located in the periodic table near the Ca element, indicating these elements have very similar chemical properties to Ca. According to petrology, elements of Ba, Rb, Cs, and Sr often replace Ca in the mineral with isomorphism (Mou, 1999). The solubility of Ca in water decreases with the increase in temperature; when the temperature is high in summer, these elements’ concentrations decrease in the water, and when the temperature is low in winter, these elements’ concentrations increase in the water.
Besides the mechanism of intensive water-rock interaction, slight changes in the physical-chemical or oxidation-deoxidation environment would cause changes in trace element concentrations. Thus, the changes in trace elements in the Songshan spring were mainly caused by the result of the water-rock interaction combined with the changes in the physical-chemical environment caused by the earthquake.
There were approximately 400 earthquakes occurring in the Beijing area during sampling (from August 2021 to August 2022) (Figure 1); only 22 of them were above M1.0, and the Chaoyang ML3.3 earthquake was the biggest one, which means that seismic activities in Beijing were dominated by frequent small earthquakes. In terms of the spatial distribution of earthquakes, these earthquakes mainly occurred in two groups of NE orderly active fault zones and one NW orderly Nankou-Sunhe Fault Zone which was controlled by the large-scale Zhangjiakou-Bohai Fault Zones in the northwest direction. The Chaoyang ML3.3 earthquake and Songshan hot spring were located in the northwest direction and were consistent with the distribution direction of Zhangjiakou-Bohai Fault Zones, so they had a strong spatial correlation.
From a time series perspective, trace elements of different groups had significant upward or downward changes before or after February. Coincidentally, the biggest Chaoyang earthquake occurred on February 3, so they had a strong temporally relationship. In addition to the Chaoyang earthquake, there were 10 earthquakes above M1.0 densely occurring from December 2021 to March 2022 (Figure 1); the swarm of earthquakes might be temporally related to changes in trace elements, but the spatial distribution of these earthquakes was not clustered and spread along a northwest direction which was also consistent with the distribution direction of Zhangjiakou-Bohai Fault Zones. Hence, a group of small earthquakes above M1.0, which is mainly the biggest Chaoyang earthquake, indicates that the Zhangjiakou-Bohai seismic zones were strongly active in February, which probably caused the abnormal changes in trace elements.
On a regional scale, there were three earthquakes above M1.0 during sampling in the Yanqing district, and the three earthquakes occurred near the Mountain Front Fault of Nankou (Figure 1) which was far away from Songshan spring. At the same time, no earthquakes above M1.0 occurred during sampling in the Northern Marginal Fault Zones of the Yanqing basin which is closest to Songshan spring.
Above all, the Chaoyang earthquake had a small magnitude and longer epicenter distance, and trace elements had the potential to be more sensitive in detecting small changes caused by minor crustal strain. Hence, the monitoring of trace elements had temporal-spatial significance in the Beijing area where small earthquakes occurred frequently.
Notably, different geological and hydrogeological settings may show distinct sensitivities to trace element constitutes, thus sensitive trace elements to seismic activities are determined by the aquifer lithology and physical-chemical conditions for specific sites. In the Songshan spring aquifer system, Li, Sc, Ti, Pb, Cu, Nb, Th, Zn, Tl, U, and REEs were sensitive to earthquake stress and would be good candidates for monitoring earthquake activities. Furthermore, we propose to comparatively investigate the sensitivity of trace element composition in the Beijing area even in the Zhangjiakou-Bohai Fault Zone. Additionally, we do not know the changes in major ions and isotopes in Songshan spring, so more comprehensive geochemical composition should be provided in future research, providing additional useful information to deepen the understanding of the mechanism of geochemistry changes to earthquake stress.
This study has the following three conclusions.
1. Although the Chaoyang ML3.3 earthquake had a small magnitude and longer epicenter distance, some trace elements in Songshan spring are likely to be sensitive to crustal minor strain by the earthquake. The Eu element of rare earth elements had obvious upward changes after the earthquake. The first group consisting of Li, Sc, Ti, and Pb elements had significantly upward changes, and the second group consisting of Cu, Nb, Th, Zn, Tl, and U elements had downward changes before the earthquake.
2. The changes in trace elements in the Songshan spring were mainly caused by the result of the water-rock interaction.
3. The monitoring of trace elements had temporal-spatial significance in the Beijing area where small earthquakes occurred frequently. Furthermore, we proposed to comparatively investigate the sensitivity of trace element composition in the Zhangjiakou-Bohai Fault Zone.
The original contributions presented in the study are included in the article/Supplementary Material, further inquiries can be directed to the corresponding author.
YC: Conceptualization, Investigation, Writing–original draft. JL: Writing–review and editing, Conceptualization, Investigation.
This work was supported by grants from the National Natural Science Foundation of China (41972027) and the Project of Monitoring, Forecasting, and Research of China Earthquake Administration (3JH-202201057).
We express our sincere thanks to two reviewers and Dr. Gaetano De Luca for valuable constructive comments on this manuscript that greatly enhanced the clarity and improved this work.
The authors declare that the research was conducted in the absence of any commercial or financial relationships that could be construed as a potential conflict of interest.
All claims expressed in this article are solely those of the authors and do not necessarily represent those of their affiliated organizations, or those of the publisher, the editors and the reviewers. Any product that may be evaluated in this article, or claim that may be made by its manufacturer, is not guaranteed or endorsed by the publisher.
The Supplementary Material for this article can be found online at: https://www.frontiersin.org/articles/10.3389/feart.2023.1260559/full#supplementary-material
Barberio, M. D., Barbieri, M., Billi, A., Doglioni, C., and Petitta, M. (2017). Hydrogeochemical changes before and during the 2016 Amatrice-Norcia seismic sequence (central Italy). Sci. Rep. 7 (1), 11735. doi:10.1038/s41598-017-11990-8
Brodsky, E. E., Roeloffs, E., Woodcock, D., Gall, I., and Manga, M. (2003). A mechanism for sustained groundwater pressure changes induced by distant earthquakes. J. Geophys. Res. 108 (B8), 2390. doi:10.1029/2002JB002321
Chen, Y. (2023). Time-frequency characteristics of groundwater in Wuliying monitoring well, Yanqing, Beijing and its relationship with regional seismic activity[J]. North China Earthquake Sci. 41 (2), 1–12. doi:10.3969/j.issn.1003–1375.2023.02.000
Chen, Y., Liu, J., Shen, B., Wang, Y., Wu, R., and Zhan, R. (2021). Geochemistry of lower ordovician microbialites on the yangtze platform, south China: implications for oceanic oxygenation at the onset of the GOBE. Palaeogeogr. Palaeoclimatol. Palaeoecol. 578, 110564. doi:10.1016/j.palaeo.2021.110564
Claesson, L., Skelton, A., Graham, C., Dietl, C., Mörth, M., Torssander, P., et al. (2004). Hydrogeochemical changes before and after a major earthquake. Geology 32 (8), 641–644. doi:10.1130/g20542.1
Claesson, L., Skelton, A., Graham, C., and Mörth, C. M. (2007). The timescale and mechanisms of fault sealing and water-rock interaction after an earthquake. Geofluids 7 (4), 427–440. doi:10.1111/j.1468-8123.2007.00197.x
De Luca, G., Di Carlo, G., and Tallini, M. (2018). A record of changes in the Gran Sasso groundwater before, during and after the 2016 Amatrice earthquake, central Italy. Sci. Rep. 8, 15982. doi:10.1038/s41598-018-34444-1
Epskamp, S., Cramer, A., Waldorp, L., Schmittmann, V., and Borsboom, D. (2012). Qgraph: network visualizations of relationships in psychometric data. J. Stat. Softw. 48, 1–18. doi:10.18637/jss.v048.i04
Favara, R., Italiano, F., and Martinelli, G. (2001). Earthquake-induced chemical changes in the thermal waters of the Umbria region during the 1997-1998 seismic swarm. Terra nova. 13 (3), 227–233. doi:10.1046/j.1365-3121.2001.00347.x
Gao, L., and Xing, C. Q. (2011). Two abnormalities in fluid precursor observation in beijing wuliying well and related discussion (in Chinese). Northwest. Seismol. J. 33 (3), 644–652. doi:10.3969/j.issn.0253-4967.2011.03.013
Ge, S., and Stover, S. C. (2000). Hydrodynamic response to strike-and dip-slip faulting in a halfspace. J. Geophys. Res. 105 (B11), 25513–25524. doi:10.1029/2000JB900233
Igarashi, G., Saeki, S., Takahata, N., Sumikawa, K., Tasaka, S., Sasaki, Y., et al. (1995). Groundwater radon anomaly before the Kobe earthquake in Japan. Science 269, 60–61. doi:10.1126/science.269.5220.60
Igarashi, G., and Wakita, H. (1995). Geochemical and hydrological observations for earthquake prediction in Japan. J. Phys. Earth 43, 585–598. doi:10.4294/jpe1952.43.585
Jiang, C. S., Wu, Z. L., and Li, Y. T. (2008). Estimating the location accuracy of the Beijing Capital Digital Seismograph Network using repeating events. Chin. J. Geophys. 51 (3), 817–827.
King, C. Y. (1986). Gas geochemistry applied to earthquake prediction: an overview. J. Geophys. Res. Solid Earth 91 (B12), 12269–12281. doi:10.1029/jb091ib12p12269
Kingsley, S., Biagi, P., Piccolo, R., Capozzi, V., Ermini, A., Khatkevich, Y., et al. (2001). Hydrogeochemical precursors of strong earthquakes: A realistic possibility in kamchatka. Part C. Sol. Terr. Planet. Sci. 26, 769–774. doi:10.1016/s1464-1917(01)95023-8
Kitagawa, Y., Koizumi, N., and Tsukuda, T. (1996). Comparison of postseismic groundwater temperature changes with earthquake-induced volumetric strain release: yudani Hot Spring, Japan. Jpn. Geophys Res. Lett. 23 (22), 3147–3150. doi:10.1029/96gl02517
Kopylova, G. N., Boldina, S. V., and Serafimova, Y. K. (2022). Earthquake precursors in the ionic and gas composition of groundwater: A review of world data. Geochem. Int. 60 (10), 928–946. doi:10.1134/s0016702922100056
Li, Z. C., and Huang, Q. H. (2014). Assessment of detectability of the Capital circle Seismic Network by using the probability-based magnitude of completeness (PMC) method. Chin. J. Geophys. 57 (8), 2584–2593. doi:10.6038/cjg20140818
Lockner, D. A., and Beeler, N. M. (2002). Rock failure and earthquakes, in International handbook of earthquake and engineering seismology. Editor W. H. K. Leeet al. (San Diego: Academic Press), 505–537.
Manga, M., and Wang, C.-Y. (2015). Earthquake hydrology, treatise on geophysics. Amsterdam: Elsevier.
Martinelli, G., and Dadomo, A. (2017). Factors constraining the geographic distribution of earthquake geochemical and fluid-related precursors. Chem. Geol. 469, 176–184. doi:10.1016/j.chemgeo.2017.01.006
Martinelli, G. (2015). Hydrogeologic and geochemical precursors of earthquakes: an assessment for possible applications. Boll. Geofis. Teor. Appl. 56, 83–94. doi:10.4430/bgta0146
Martinelli, G. (2020). Previous, current, and future trends in research into earthquake precursors in geofluids. Geosciences 10, 189. doi:10.3390/geosciences10050189
Montgomery, D. R., and Manga, M. (2003). Streamflow and water well responses to earthquakes. Science 300, 2047–2049. doi:10.1126/science.1082980
Petrini, R., Italiano, F., Riggio, A., Slejko, F. F., Santulin, M., Buccianti, A., et al. (2012). Coupling geochemical and geophysical signatures to constrain strain changes along thrust faults. Boll. Geof. Teor. Appl. 53, 113–134. doi:10.4430/bgta0017
Poitrasson, F., Dundas, S. H., Toutain, J.-P., Munoz, M., and Rigo, A. (1999). Earthquake-related elemental and isotopic lead anomaly in a springwater. Earth. Planet. Sci. Lett. 169 (3), 269–276. doi:10.1016/s0012-821x(99)00085-0
Roeloffs, E. A. (1998). Persistent water level changes in a well near Parkfield, California, due to local and distant earthquakes. J. Geophys. Res. 103 (B1), 869–889. doi:10.1029/97JB02335
Rojstaczer, S., and Wolf, S. (1992). Permeability changes associated with large earthquakes:An example from Loma Prieta, California. Geology 20 (3), 211–214. doi:10.1130/0091-7613(1992)020<0211:pcawle>2.3.co;2
Rosen, M. R., Binda, G., Archer, C., Pozzi, A., Michetti, A. M., and Noble, P. J. (2018). Mechanisms of earthquake-induced chemical and fluid transport to carbonate groundwater springs after earthquakes. Water. Resour. Res. 54 (8), 5225–5244. doi:10.1029/2017wr022097
Shi, Z. M., Zhang, H., and Wang, G. C. (2020). Groundwater trace elements change induced by M5.0 earthquake in Yunnan. J. Hydrology 581, 124424. doi:10.1016/j.jhydrol.2019.124424
Skelton, A., Andrén, M., Kristmannsdóttir, H., Stockmann, G., Mörth, C. M., Sveinbjörnsdóttir, Á., et al. (2014). Changes in groundwater chemistry before two consecutive earthquakes in Iceland. Nat. Geosci. 7, 752–756. doi:10.1038/ngeo2250
Skelton, A., Liljedahl-Claesson, L., Wästeby, N., Andrén, M., Stockmann, G., Sturkell, E., et al. (2019). Hydrochemical changes before and after earthquakes based on long-term measurements of multiple parameters at two sites in northern Iceland—a review. J. Geophys. Res. SolidEarth(ja) 124, 2702–2720. doi:10.1029/2018JB016757
Taran, Y. A., Ramirez-Guzman, A., Bernard, R., Cienfuegos, E., and Morales, P. (2005). Seismic-related variations in the chemical and isotopic composition of thermal springs near Acapulco, Guerrero, Mexico. Geophys. Res. Lett. 32 (14). doi:10.1029/2005gl022726
Taylor, S. R., and McLennan, S. M. (1985). The continental crust: Its composition and evolution. Oxford: Blackwell.
Thomas, D. (1988). Geochemical precursors to seismic activity. Pure. Appl. Geophys. 126 (2–4), 241–266. doi:10.1007/bf00878998
Toutain, J., Munoz, M., Poitrasson, F., and Lienard, A. (1997). Springwater chloride ion anomaly prior to a M L = 5.2 Pyrenean earthquake. Earth. Planet. Sci. Lett. 149 (1), 113–119. doi:10.1016/s0012-821x(97)00066-6
Tsunogai, U., and Wakita, H. (1995). Precursory chemical changes in ground water: kobe earthquake, Japan. Jpn. Sci. 269, 61–63. doi:10.1126/science.269.5220.61
Wakita, H. (1996). Geochemical challenge to earthquake prediction. PNAS 93 (9), 3781–3786. doi:10.1073/pnas.93.9.3781
Wang, C-Y. (2007). Liquefaction beyond the near field. Seismol. Res. Lett. 78, 512–517. doi:10.1785/gssrl.78.5.512
Wang, C.-Y., Cheng, L. H., Chin, C. V., and Yu, S. B. (2001). Coseismic hydrologic response of an alluvial fan to the 1999 Chi-Chi earthquake, Taiwan. Taiwan. Geol. 29 (9), 831–834. doi:10.1130/0091-7613(2001)029<0831:chroaa>2.0.co;2
Wang, K., Chen, Q.-F., Sun, S., and Wong, A. (2006). Predicting the 1975 Haicheng earthquake. Bull. Seism. Soc. Am. 96, 757–795. doi:10.1785/0120050191
Woith, H., Wang, R., Maiwald, U., Pekdeger, A., and Zschau, J. (2013). On the origin of geochemical anomalies in groundwaters induced by the Adana 1998 earthquake. Chem. Geol. 339, 177–186. doi:10.1016/j.chemgeo.2012.10.012
Yang, M., Liu, G., Liu, Z., Ma, J., Li, L., Wang, Z., et al. (2022). Geochemical characteristics of geothermal and hot spring gases in Beijing and Zhangjiakou Bohai fault zone. Front. Earth Sci. 10, 933066. doi:10.3389/feart.2022.933066
Keywords: Zhangjiakou-bohai fault zone, earthquake monitor, geochemistry, rare earth elements, small earthquake
Citation: Chen Y and Liu J (2023) Groundwater trace element changes were probably induced by the ML3.3 earthquake in Chaoyang district, Beijing. Front. Earth Sci. 11:1260559. doi: 10.3389/feart.2023.1260559
Received: 18 July 2023; Accepted: 04 September 2023;
Published: 26 September 2023.
Edited by:
Giovanni Martinelli, National Institute of Geophysics and Volcanology, ItalyReviewed by:
Xiaocheng Zhou, China Earthquake Administration, ChinaCopyright © 2023 Chen and Liu. This is an open-access article distributed under the terms of the Creative Commons Attribution License (CC BY). The use, distribution or reproduction in other forums is permitted, provided the original author(s) and the copyright owner(s) are credited and that the original publication in this journal is cited, in accordance with accepted academic practice. No use, distribution or reproduction is permitted which does not comply with these terms.
*Correspondence: Yuxuan Chen, Y3l4NjMwQDE2My5jb20=
Disclaimer: All claims expressed in this article are solely those of the authors and do not necessarily represent those of their affiliated organizations, or those of the publisher, the editors and the reviewers. Any product that may be evaluated in this article or claim that may be made by its manufacturer is not guaranteed or endorsed by the publisher.
Research integrity at Frontiers
Learn more about the work of our research integrity team to safeguard the quality of each article we publish.