- 1Sanya Offshore Oil & Gas Research Institute, Northeast Petroleum University, Sanya, China
- 2College of Geosciences, Northeast Petroleum University, Daqing, China
- 3Bohai-Rim Energy Research Institute, Northeast Petroleum University, Qinhuangdao, China
Mechanical stratigraphy plays an important role in controlling the nucleation, propagation, and development of fractures. The fracture development pattern and development mode were defined in this study via a detailed description. Fracture growth and evolution in bedded rocks were numerically simulated. The results show that fracture growth and propagation are controlled by the mechanical layer. Fractures are divided into bed-confined fractures and throughgoing fractures based on their spatial configuration with the mechanical layer. Fractures preferentially nucleate and expand in the mechanical layer and terminate at mechanical contact. After that, new fractures are generated between two adjacent fractures. No new fractures are further developed when stress reaches a certain value, indicating a saturation state. Finally, throughgoing fractures are developed. Under the same stress field, the bed-confined fracture density is positively correlated with Young’s modulus of the mechanical layer and is negatively correlated with its thickness. Bed-confined fractures can extend to another mechanical layer to develop throughgoing fractures only under significant stress fields or at mechanical contact with small thickness or minor difference in mechanical properties. Exploring the impact of mechanical stratigraphy on fracture development and propagation in bedded rocks is of great significance to investigate the fracture distribution in hydrocarbon reservoirs.
1 Introduction
Natural fractures are primary seepage channels and effective reservoirs, playing an important role in hydrocarbon migration and accumulation (Zeng et al., 2012; Amjad et al., 2023; Fu et al., 2023). In the Midland Basin in the United States, the Outer Moray Firth Basin in the United Kingdom, and the Llanos Basin in South America, etc., it has been shown that natural fractures play a decisive role in the accumulation and migration of oil and gas in oil and gas reservoirs (Aydin et al., 2005; Laubach et al., 2016; Ullah et al., 2023). The occurrence of natural fractures can exert great impacts on reservoir quality, oil and gas enrichment, drilling and fracturing, reservoir development, and single-well productivity (Laubach et al., 2018; Gong et al., 2019a; Mi et al., 2023a). Hence, investigating the natural fracture distribution has a high application value for guiding oil and gas exploration and development (Gong et al., 2015; Lyu et al., 2021; Gong et al., 2023). Therefore, the study of natural fractures in reservoirs has become a hot target globally.
Bedded rocks are characterized by obvious differences in mechanical properties among adjacent layers (Gong et al., 2018; Zhu et al., 2019; Zuo et al., 2019), which are popular in nature under various geological events, for example, sedimentation and tectonic movements. A large number of fractures are developed close to or through lithology contact in bedded rocks, where lithology can exert significant impact on fracture growth and propagation, such as in the Barnett shale gas reservoir of the Fort Worth Basin (Helgeson et al., 1991; Cooke et al., 2001; Fu et al., 2022). Some fractures are terminated at lithology contacts, with no propagation in the vertical direction. Fractures propagating multiple rocks with different lithology provide channels for vertical migration (Gong et al., 2017a). Bedded rocks are composed of multiple lithology layers, which can be divided into different mechanical units based on their mechanical properties (Chang et al., 2017; Zeng et al., 2020).
As early as the 19th century, Willis (1894) proposed the concept of the rock mechanical layer. The rock mechanics layer refers to a rock layer with a similar or consistent rock mechanic behavior, which has a certain control effect on the development and expansion of fractures (Gong et al., 2018). Outcrop and core observations as well as log interpretation show that mechanical properties and bed thickness govern fracture development, resulting in different fracture development intensities and propagation modes in bedded rocks (Wang et al., 2012; Zhao et al., 2013). The fracture distribution in vertical and geometric relationship between fractures and rocks play a key role in underground oil and gas distribution (Gong et al., 2019b; Zeng et al., 2022).
The difference in mechanical properties of fractured reservoirs is the main factor controlling fracture development (Chang et al., 2017; Zhao et al., 2018; Gao et al., 2019). Rocks can be divided into various mechanical layers based on their mechanical properties, which is one of the most important factors controlling the nucleation, expansion, geometric morphology, and distribution of natural fractures (Gong et al., 2021; Wang et al., 2021; Mi et al., 2023b). The fracturing in actual production is commonly controlled by mechanical stratigraphy, which is prone to be deflected at mechanical contact to shorten the vertical extension of fractures and induce interlayer sliding, resulting in casing damage and borehole scrapping (Chen et al., 2014; Zhang et al., 2020; Zhang et al., 2022).
Therefore, mechanical layer models with different mechanical parameters and thickness are established in this study based on the mechanical layer structure and fracture distribution on outcrops to simulate the development and propagation mode of fractures, explore fracture formation mechanisms in bedded rocks, and identify controllers of various fracture propagation patterns. It can provide insight into fracture prediction and hydraulic fracturing in bedded rocks.
2 Materials and methods and techniques
We first characterized the phenomenon of outcrop profiles in the field, truly reflecting the influence of layered rocks on fracture development. Four outcrops were mainly described, including the Shahejie Formation in the Bohai Bay Basin, the Chang7 Formation in the Ordos Basin, and the Qingshankou Formation in the Songliao Basin, totaling four different stratigraphic profiles from three basins (Figure 1). It also includes different lithological sections, such as sandstone and shale, which are used to divide rock mechanical layers and characterize different types of natural fractures. We took high-pixel image shots of each described profile for later data proofreading. The description of fractures mainly focuses on the contact relationship between fractures and lithological layers. This includes fractures that pass through multiple sets of lithological layers, fractures that come into contact with the core interface, and fractures inside the rock layer. Subsequently, based on the division of mechanical layers, the relationship between fractures and mechanical layers will be rechecked.
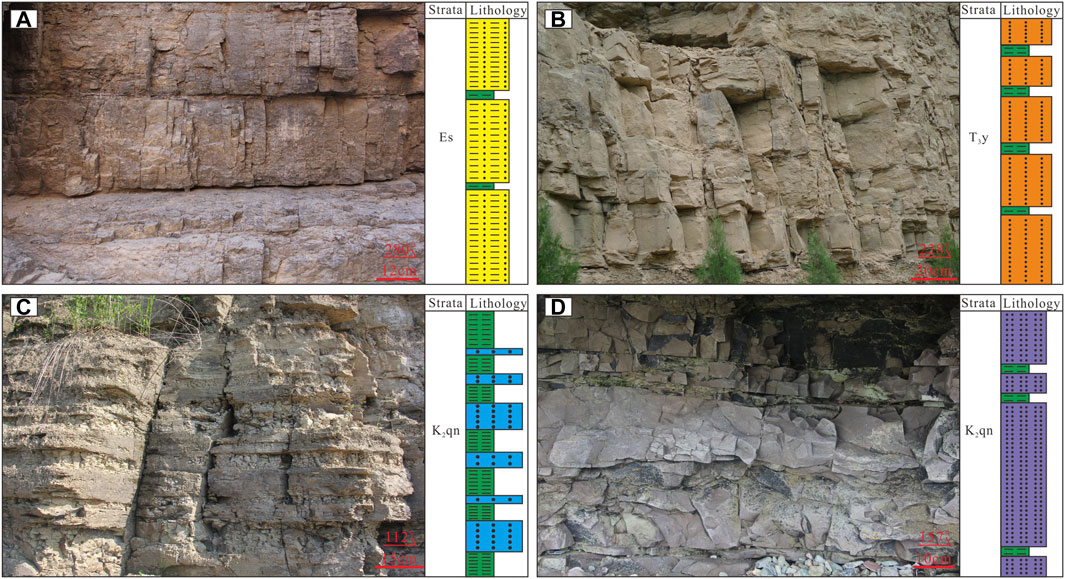
FIGURE 1. (A) Stratigraphic profile of the Shahejie Formation in Nanbao Sag; (B) stratigraphic profile of the Yanchang Formation in the Ordos Basin; (C–D) stratigraphic profile of the Qingshankou Formation in the Songliao Basin.
We used a rebound instrument to measure the mechanical parameters of layered rocks. The rebound values measured using the Schmidt hammer are good indicators of rock hardness, which can quantitatively characterize rock strength. The rebound value measurement in the field has certain requirements: test points should be far away from bedding boundaries, and the rock surface should be regular and even with no obvious fractures in the rocks. Under these conditions, no bias can be brought to rebound values. The Schmidt hammer should be perpendicular to the rock surface (Katz et al., 2000; Aydin et al., 2005). The ZC3-A Schmidt hammer was used to measure rebound values at measuring points (every 0.5 m) based on the lithology division of bedded rocks in outcrops. Each measuring point was tested at least seven times to eliminate abnormal values. Finally, we calculated the rebound value range of each lithology unit.
To accurately determine the impact of mechanical layers on facture propagation, we first accurately divided the rock mechanical layers of each section. First, the mechanical layer of bedded rocks was divided based on rebound values and lithology data, and the average height and length of each mechanical layer were measured and calculated using a meter ruler, which could be used to characterize the influence of mechanical stratigraphy on fracture propagation. Whether fractures pass through or terminate at the mechanical contact was determined based on the relationship between fractures and mechanical layer. In addition, fracture shapes, types, and numbers were recorded. Fracture height and spacing were measured based on the one-dimensional scanning line method. In the same rock mechanics layer, there is a negative correlation between fracture density and fracture spacing. The fracture spacing index (FSI) is the ratio of fracture spacing to the thickness of the mechanical layer where fractures are located. The fracture spacing index can display the degree of fracture development, and the smaller the fracture spacing index, the higher the fracture density will be (Chang et al., 2015; Gong et al., 2018).
The simulation technology software mainly used in this article is “real failure process analysis” (RFPA), which was employed to simulate the fracture propagation mode in bedded rocks with different mechanical properties and thickness. RFPA is a two-dimensional finite element code, which can simulate fracturing and failure of quasi-brittle materials (e.g., rocks) (Tang et al., 2002). It considers heterogeneity in rocks and simulates discontinuous behaviors of deformation and failure by weakening elements. The fracture development mode in bedded rocks was simulated based on the two-end loading mode, exhibiting the process from fracture initiation to propagation and development. It can be used to analyze fracture propagation paths and variation patterns and investigate fracture spacing, fracture saturation mechanisms, and fracture propagation under strain conditions (Bai et al., 2000; Bao et al., 2014).
3 Results
3.1 Mechanical stratigraphy division
Mechanical stratigraphy refers to rocks with similar mechanical behaviors or same mechanical properties. It can be composed of one or more lithology units (Su et al., 2023). The mechanical stratigraphy controls fracture initiation and termination in bedded rocks as well as fracture development and expansion (Kou et al., 2022; Gong et al., 2017b). Mechanical layers in bedded rocks from outcrops are divided based on lithology, rebound value, and other information, and fracture development in each mechanical layer was analyzed and described in this study (Figures 2A,B). Fracture development is positively correlated with the rebound value, indicating that fractures can be well developed in rocks with strong strength (Figure 2C). The thickness of the mechanical layer can also exert certain impact on fracture spacing; for example, large thickness can result in large fracture spacing and low fracture density (Figure 2D). Fracture propagation can be hindered by mechanical contact, whereas mechanical contact in our studied area can be divided into three levels, namely, stratigraphic boundary, stratigraphic group boundary, and mudstone layer (Larsen et al., 2010a) (Figures 2A,B). It also varies with the mechanical layer; particularly, the mudstone layer can hinder fracture development greatly, with fractures that terminate in mudstone layers accounting for 90.21%. Fractures terminating at the stratigraphic group boundary account for 78.47%, whereas fracture propagation limited by the stratigraphic boundary only accounts for 40.62%.
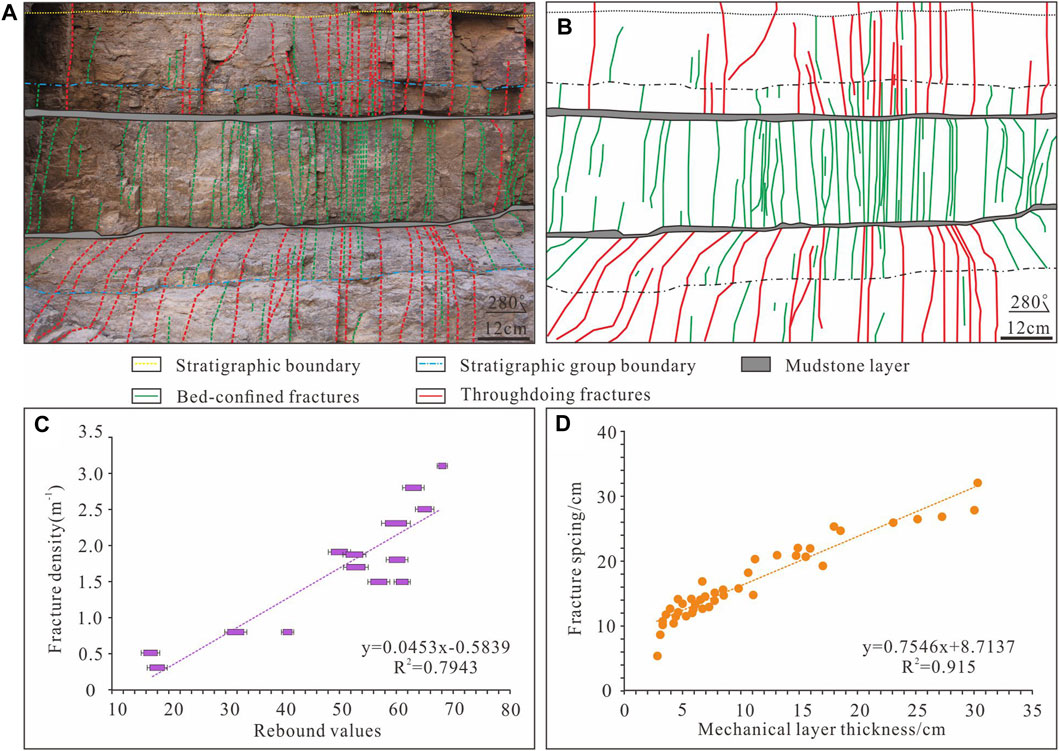
FIGURE 2. (A) Fracture development patterns in bedded rocks. (B) Sketch of fracture developments in bedded rocks. (C) Relationship between rebound values of rocks and fracture development. (D) Relationship between rock thickness and fracture spacing.
3.2 Characteristics of fracture development in bedded rocks
Natural fractures are divided into two types (Gross et al., 2007) based on the spatial geometry of fractures and mechanical layer and coupling between fractures and mechanical contacts (Figures 2A,B). Bed-confined fractures are those developing within a single mechanical layer, with contact or no contact between fracture ends and mechanical contact (some fractures contacting mechanical contact at one end and some fractures contacting mechanical contact at both ends). Bed-confined fractures can be divided into two types (Gale et al., 2014) based on contacts between fractures and mechanical layer observed from outcrops: bed-internal fractures and top bed-confined fractures. The former is commonly developed within the mechanical layer, with the fracture height less than the rock thickness and no contact between the fracture ends and mechanical contact. The latter is bounded by adjacent rocks with weak strength, with one or both fracture ends terminating at contacts; as a result, fracture height is less than or equal to rock thickness (Figure 3).
Affected by mechanical stratigraphy, the bed-confined fractures are generally small in length, and lower than or equal to rock thickness. It is mainly distributed over 10–40 cm, following normal distribution patterns. Bed-confined fractures have large dip, nearly vertical to the rock surface. In addition, bed-confined fractures have small apertures, which are generally unfilled ones and regularly distributed in bedded rocks. In most cases, bed-confined fractures in the single layer are parallel or sub-parallel to each other, with nearly equal spacing. Bed-confined fractures in rocks with strong strength are small in distance but high in density, whereas fractures in rocks with weak strength are large in distance but low in density. In addition, a reciprocal relationship can be observed between fracture development and fracture spacing.
Throughgoing fractures are multi-layer structures with large dip angle and extension. They can penetrate two or more mechanical layers vertically (Figure 3). They are generally 10–80 cm in extension, following the normal distribution pattern. Their apertures are obviously larger than those of the bed-confined fractures, with same filling behaviors. Those adjacent throughgoing fractures are different in extension but similar in dip angles. They are nearly parallel with bed-confined fractures on the same horizontal. It indicates that these bed-confined fractures and throughgoing fractures were formed during the same period. Throughgoing fractures are commonly well developed in rocks with strong strength and can extend to the contact of rocks with weak strength. Periodically, they can penetrate rocks with weak strength and continue to expand.
3.3 Numerical simulation of fracture growth and propagation in bedded rocks
Fracture development was numerically simulated using RFPA software based on the elastic damage theory and the Mohr–Coulomb criterion to explore the fracturing mode in bedded rocks. Based on a large number of practical research results, it has been found that the stronger the mechanical layer, the more conducive it is to the development of fractures. Therefore, in order to explore the formation and propagation patterns of fractures and reduce the influence of other factors on fracture formation and propagation, we have set up an idealized model (Figure 4). Among them, there is a significant difference in the mechanical properties between the strong and weak mechanical layers. The simulation shows that in terms of bedded rocks, fractures are preferentially developed in rocks with strong strength, where fracture extension is increased with strain. Defects can occur locally in heterogeneous rocks with strong strength at certain tensile stress (defects are discontinuous and partly opened fractures) (Figures 5A,B). The number and length of defects can be increased with strain, connecting them to form fractures (Figures 5C,D). Fracture extension can be confined to the contact of rocks with weak strength, where new fractures will be gradually developed among existing fractures with equal spacing, which can be defined as a “continuous filling” process (Figure 5E) (Larsen et al., 2010a; Chang et al., 2017). No fractures will be generated even under continuous stress when a certain number of fractures are developed. Some fractures are limited within certain mechanical layers and cannot be expanded, whereas others can penetrate adjacent layers with weak strength (Figure 5F). Fracture development in bedded rocks under the control of mechanical layer and stress can be divided into three stages, namely, nucleation, saturation, and penetration.
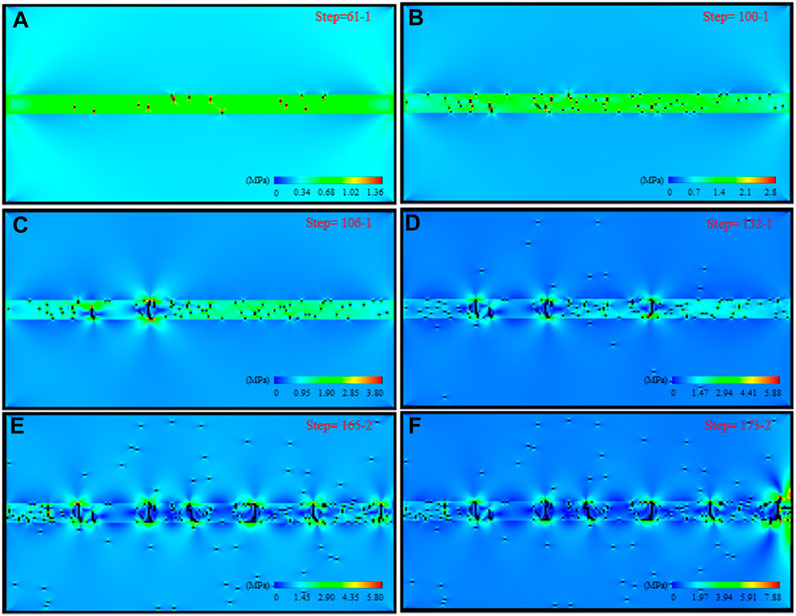
FIGURE 5. Simulation diagram of fracture development in bedded rocks. Mechanics parameters of rocks with strong mechanical layer (middle layers): E = 50 Gpa, υ = 0.2. Mechanics parameters of rocks with weak mechanical layer (layers at two ends): E = 20 Gpa, υ = 0.3. (A) Step = 61−1; (B) Step = 100−1; (C) Step = 106−1; (D) Step = 132−1; (E) Step = 165−2; (F) Step = 173−2. (Displacement: 0.02/m in each step).
4 Discussions
4.1 Fracture development mechanism in bedded rocks
4.1.1 Fracture development mechanism
It has been a challenge to determine the fracture development mechanism for a long time. Scholars have proposed many fracturing criteria via actual observation and experiments based on the rock strength hypothesis, of which the Mohr–Coulomb classic shear fracture criterion describing macro-fracturing and the Griffith generalized maximum tensile stress criterion based on micro fracturing mechanism are the most widely used (Ding et al., 2012). To explore the mechanism of fracture formation in bedded rocks, we use RFPA software to simulate fracture development in bedded rocks and record stress state around two adjacent fractures. The simulation shows the following:
Once fractures are developed in bedded rocks, the tensile stress at fractures will be reduced to zero, and then interfacial shear stress will be developed between two adjacent fractures. However, the tensile stress near the middle of the two adjacent fractures is always at the peak value, resulting in new fractures at these positions with equal fracture spacing. The tensile stress in the middle of two fractures is not only related to the stress on rocks but also to the distance between two fractures. The distribution of the vertical stress between two adjacent fractures is extracted by applying the same strain to these two fractures with different spacing (Figure 6) (compressive stress: positive; tensile stress: negative). A threshold FSI value occurs under the same strain condition. In addition, a compressive stress zone occurs between fractures when the simulated FSI is less than the threshold value, where decreasing the FSI value can increase the compressive stress zone. Tensile stress occurs between these two fractures when the simulated FSI is higher than the threshold value.
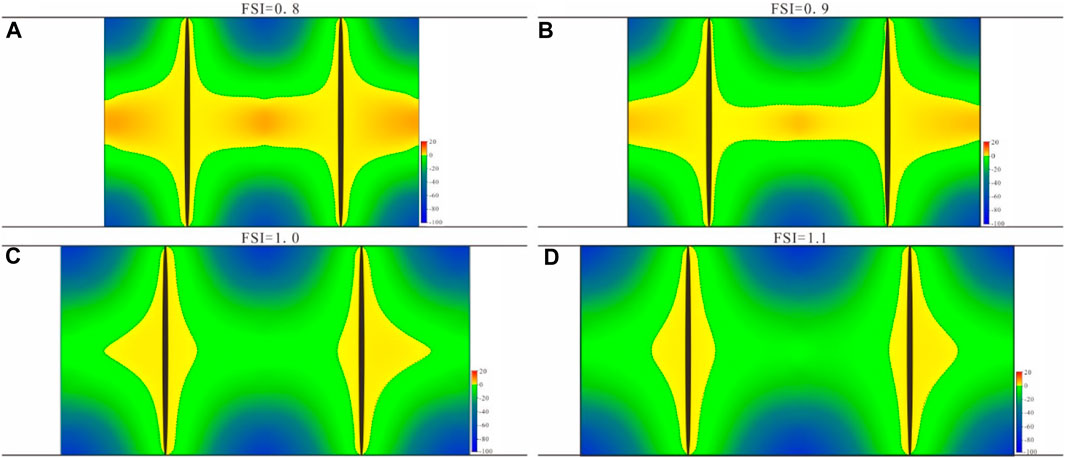
FIGURE 6. Map of vertical stress contours among adjacent fractures under different FSIs (compressive stress: positive, tensile stress: negative). (A) FSI = 0.8; (B) FSI = 0.9; (C) FSI = 1.0; (D) FSI = 1.1.
4.1.2 Fracture saturation mechanism
Increasing strain can continually generate fractures in the middle of the existing fractures during fracture development, decreasing fracture spacing obviously. However, fracture spacing is not changed at certain strain, and no fractures will be generated in rocks. This phenomenon is called fracture saturation (Chang et al., 2017; Gong et al., 2018). Two adjacent fractures in bedded rocks are selected via multiple simulations using RFPA software to track their stress states to better understand the fracture saturation mechanism. The distance between these two fractures is assumed as AA’, where the middle point is represented by symbol “O.” The fracture saturation is simulated by changing AA’, exploring variation in stress under the same strain. A fracture spacing index (FSI) threshold can be identified from the simulation (Figure 7). Tensile stress occurs at the middle point (O) when the simulated FSI value is higher than 1, and compressive stress can be observed at the middle point (O) when the simulated FSI value is less than 1. This is because fractures developing in rocks with strong strength generally stop expanding to spread laterally at the contact of rocks with weak strength, resulting in shear sliding along contacts. The transformation in the stress state causes no new tensile fracture generation. The compressive stress at point O decreases with the decreasing FSI value; thereby, shear fractures are difficult to be generated under a small stress state. Hence, even if strain continues to increase, fracture spacing will not decrease correspondingly, which can well explain fracture saturation.
Fractures are generally developed under tensile stress perpendicular to the fracture plane and/or internal fluid pressure. Fractures will not be developed between two fractures when the FSI value is less than the threshold value unless locally disturbed tensile stress fields occur between these two fractures (e.g., at the fracture ends) or the compressive stress is overcome by some mechanisms (e.g., internal fluid pressure). Stress state transition is an important driver for fracture saturation in bedded rocks (Bai et al., 2000).
4.2 Impact of mechanical stratigraphy on fracture propagation
The development mode and propagation mode of fractures in bedded rocks are mainly controlled by mechanical properties and thickness of mechanical layers. Mechanical stratigraphy can be defined as the resistance of rocks to external forces, which can be described by the deformation behavior of rocks. Fracture development is generally derived from brittle deformation under tectonic stress (Ju et al., 2023). The difference in mechanical properties and thickness of adjacent two rocks can result in different fracture behaviors even under the same tectonic stress. Young’s modulus and Poisson’s ratio are two primary mechanical parameters describing the elastic deformation of rocks. The influence of mechanical properties and thickness of the mechanical layer on fracture development is investigated by considering a single variable.
4.2.1 Impact of mechanical stratigraphy on bed-confined fracture development
The same stress is applied at both ends of the model to simulate the influence of thickness, Young’s modulus E, and Poisson’s ratio (υ) of bedded rocks on bed-confined fracture development. The model and parameters are shown in Figure 8, and the results in Table 1 show the following:
(a) Under the same mechanical parameters and rock thickness (Figure 9), Young’s modulus of rocks with strong strength can exert great impact on fracture development. Bed-confined fractures are preferentially developed in rocks with strong strength under small strain when their Young’s modulus is significantly different from that of adjacent rocks with weak strength. Under the same strain, obvious difference in Young’s modulus between layers with strong strength and weak strength can result in high fracture density and small fracture spacing within layers with strong strength (Figure 10A).
(b) Under the same mechanical parameters and bed thickness (Figure 11), differences in Poisson’s ratio between layers with strong strength and weak strength have no obvious influence on bed-confined fracture development. In terms of layers with strong strength, bed-confined fracture development does not vary with Poisson’s ratio (Figure 10B).
(c) The thickness of the mechanical layer also has significant influence on bed-confined fracture development (Figure 12). Fractures will be preferentially developed in a thin mechanical layer and then extend to thick ones. The bed-confined fractures are well developed in the thin mechanical layer compared with the thick ones, with small fracture spacing (Figure 10C).
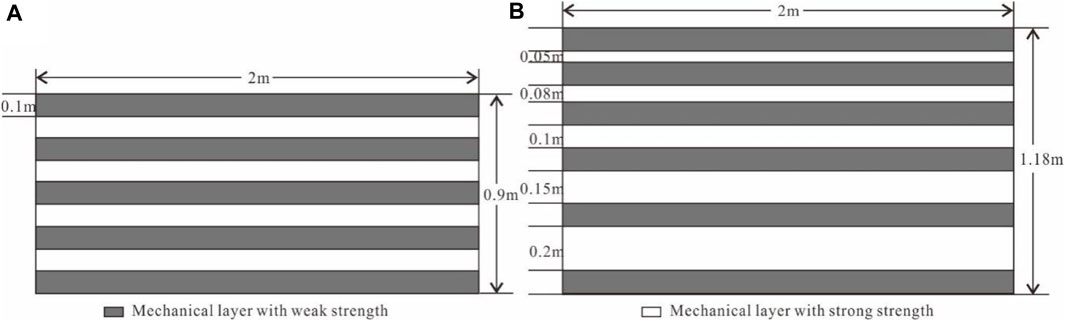
FIGURE 8. (A) Model diagram showing the influence of Young’s modulus and Poisson’s ratio on fracture development in rocks. (B) Model diagram exhibiting the influence of bed thickness on fracture development.
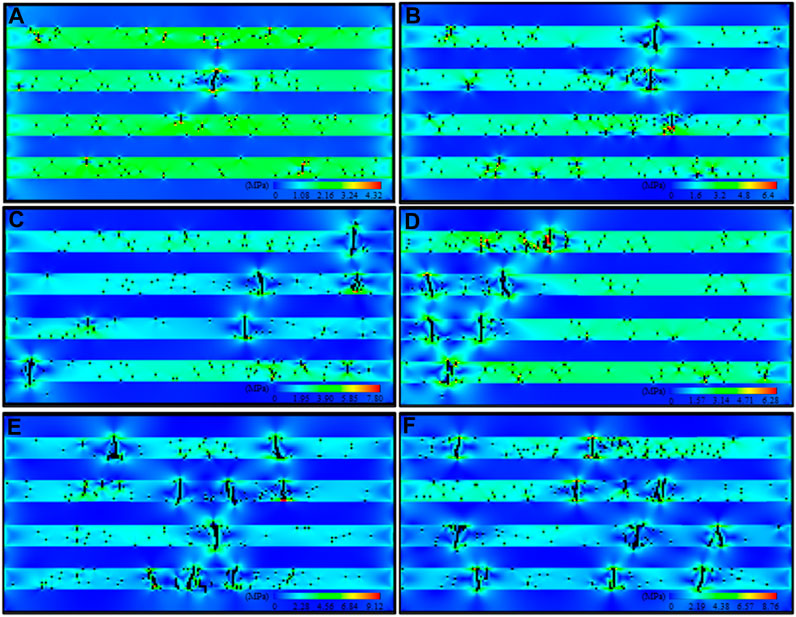
FIGURE 9. Simulated bed-confined fracture development under different Young’s moduli (E). (A) Rocks with strong strength with E = 20 GPa. (B) Rocks with strong strength with E = 30 GPa. (C) Rocks with strong strength with E = 35 GPa. (D) Rocks with strong strength with E = 40 GPa. (E) Rocks with strong strength with E = 45 GPa. (F) Rocks with strong strength with E = 50 GPa.
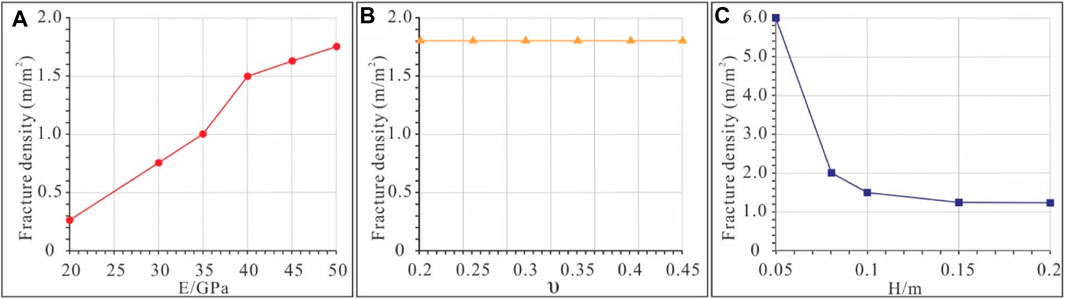
FIGURE 10. (A) Relationship between Young’s modulus (The E in the Figure represents the Young’s modulus) and bed-confined fracture density. (B) Relationship between Poisson’s ratio (The υ in the Figure represents the Poisson’s ratio) and bed-confined fracture density. (C) Relationship between rock thickness (The H in the Figure represents the rock thickness ) and bed-confined fracture density.
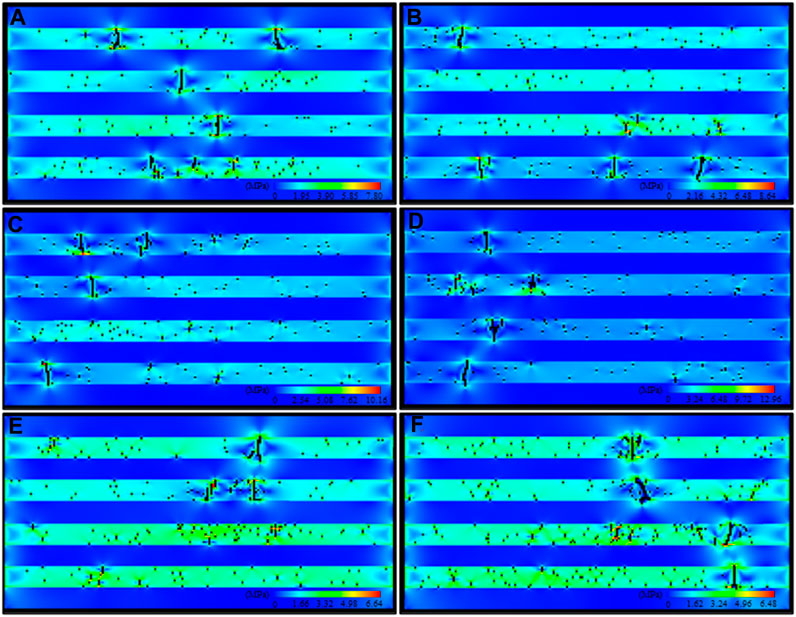
FIGURE 11. Simulated bed-confined fracture development under different Poisson’s ratios (υ). (A) Rocks with strong strength with υ = 0.45. (B) Rocks with strong strength with υ = 0.4. (C) Rocks with strong strength with υ= 0.35. (D) Rocks with strong strength with υ = 0.3. (E) Rocks with strong strength with υ = 0.25. (F) Rocks with strong strength with υ = 0.2.
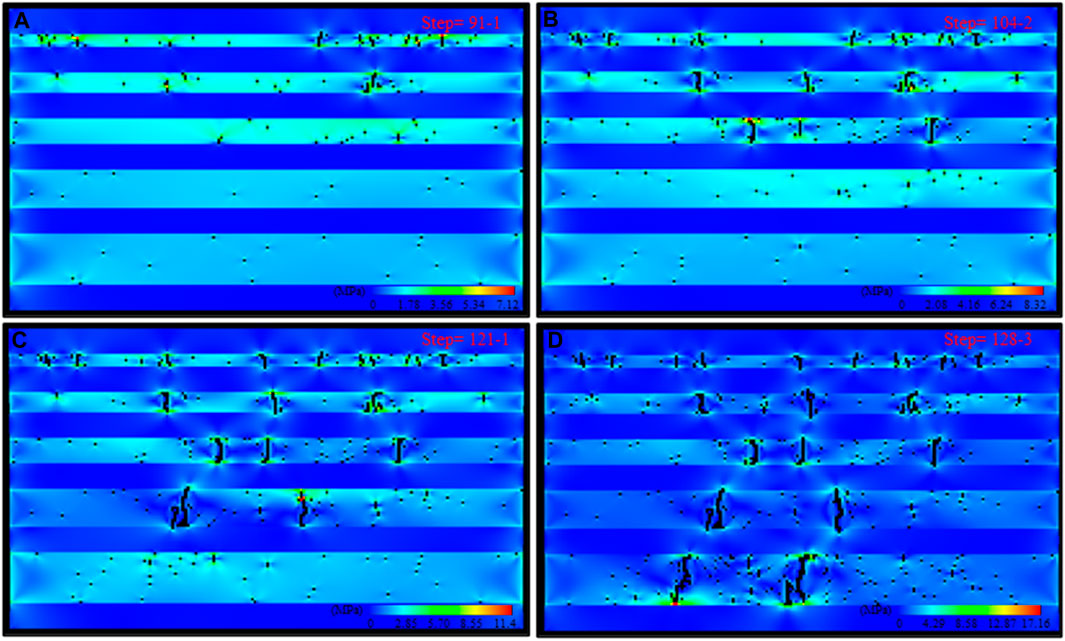
FIGURE 12. Simulated bed-confined fracture development under different thickness of rocks with strong strength. (A) Step = 91−1; (B) Step = 104−2; (C) Step = 121−1; (D) Step = 128−3 (displacement: 0.02/m at each step).
4.2.2 Impact of mechanical stratigraphy on throughgoing fracture development
Mechanical stratigraphy not only controls fracture growth and development but also governs fracture expansion. Under the same geological setting, mechanical properties and thickness of mechanical layers are primary factors determining whether bed-confined fractures can pass through the mechanical contact to develop throughgoing fractures. Fracture growth and evolution are simulated based on the fracture mechanics model using RFPA software to establish the relationship between fracture propagation and mechanical properties, thickness, and tectonic stress. The mechanical layer model is composed of interbedded rocks with strong strength and weak strength. The influence of the mechanical contact on fracture propagation is also investigated by changing mechanical parameters, thickness, and boundary stress of rocks with various strengths. The results show the following (Figure 13):
(a) Under certain boundary stress, the ratio of the thickness of rocks with weak strength to that of rocks with strong strength (H weak/H strong) is positively correlated with the corresponding ratio of Young’s modulus (E weak/E strong). It suggests that under the same tectonic stress, a small difference in mechanical properties between rocks with weak strength and rocks with strong strength requires a large thickness difference between them to confine bed-confined fractures to be developed into throughgoing fractures.
(b) Under certain E weak/E strong, H weak/H strong follows an increasing trend with the boundary stress. It indicates that under fixed mechanical properties of the mechanical layer, the large tectonic stress requires the small thickness difference between rocks with weak strength and rocks with strong strength to hinder bed-confined fractures to be extended into throughgoing fractures.
(c) Under certain H weak/H strong, E weak/E strong is negatively correlated with the boundary stress. It also represents that with the fixed thickness of the mechanical layer, a small thickness difference between rocks with weak strength and rocks with strong strength is needed to limit bed-confined fractures to grow into throughgoing fractures under large tectonic stress.
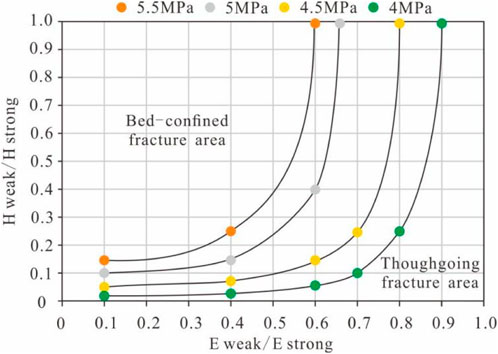
FIGURE 13. Impact of mechanical properties and thickness of the mechanical layer and stress on fracture propagation (curves are the stress threshold for developing throughgoing fractures).
The simulation suggests that whether bed-confined fractures can develop into throughgoing fractures depends not only on mechanical properties and the thickness of the adjacent mechanical layer but also on tectonic stress. Bed-confined fractures can grow in thin mechanical layers or in rocks with strong strength, whose extension can be limited by minor mechanical property variations or thin mechanical contact. However, large tectonic stress made them to pass through thick mechanical contact with various mechanical properties, developing throughgoing fractures. Only large differences in mechanical properties between adjacent mechanical layers and thick mechanical contact can limit the propagation of bed-confined fractures.
5 Conclusion
The nucleation and propagation of fractures are controlled by mechanical stratigraphy. Fractures are divided into two types based on the spatial configuration between fractures and the mechanical layer, namely, bed-confined fractures and throughgoing fractures. Controlled by mechanical stratigraphy, fracture development in bedded rocks can be divided into three stages: nucleation, saturation, and propagation. Fractures will be first nucleated and extended in rocks with strong strength under the control of stress distribution, and then new fractures will be generated among these existing fractures until reaching the saturation state. High Young’s modulus and small thickness can result in high bed-confined fracture density. Variation in Poisson’s ratio has little impact on the fracture intensity. The mechanical contact plays a certain role in hindering throughgoing fracture propagation. Small tectonic stress can generate bed-confined fractures in rocks with strong strength or small thickness, whereas large tectonic stress can make those bed-confined fractures pass through thick mechanical contact with various mechanical properties to develop throughgoing fractures.
Data availability statement
The original contributions presented in the study are included in the article/Supplementary Material; further inquiries can be directed to the corresponding author.
Author contributions
XS: Conceptualization, Writing –original draft, Writing –review and editing. LG: Conceptualization, Writing –original draft, Writing –review and editing. XF: Data curation, Writing –review and editing. YW: Formal Analysis, Writing –review and editing. SG: Writing –review and editing, Data curation. JW: Formal Analysis, Writing –review and editing. XQ: Investigation, Writing –review and editing. HL: Methodology, Writing –review and editing. TB: Writing –review and editing.
Funding
This research was funded by the National Natural Science Foundation of China (Grant Nos. 42072155 and U20A2093); the Natural Science Foundation of Heilongjiang Province (Grant No. YQ 2022D006); the Specific Research Fund of the Innovation Platform for Academicians of Hainan Province (Grant No. YSPTZX202301); the Young Innovative Talents Training Program for Universities in Heilongjiang Province (Grant No. UNPYSCT-2020147); and the Postdoctoral Scientific Research Developmental Fund of Heilongjiang Province (Grant No. LBH-Q21001).
Conflict of interest
The authors declare that the research was conducted in the absence of any commercial or financial relationships that could be construed as a potential conflict of interest.
Publisher’s note
All claims expressed in this article are solely those of the authors and do not necessarily represent those of their affiliated organizations, or those of the publisher, the editors, and the reviewers. Any product that may be evaluated in this article, or claim that may be made by its manufacturer, is not guaranteed or endorsed by the publisher.
Supplementary material
The Supplementary Material for this article can be found online at: https://www.frontiersin.org/articles/10.3389/feart.2023.1253787/full#supplementary-material
References
Amjad, M. R., Shakir, U., Hussain, M., Rasul, A., Mehmood, S., and Ehsan, M. (2023). Sembar Formation as an unconventional prospect: New insights in evaluating shale gas potential combined with deep learning. New York, N.Y. Natural resources research. doi:10.1007/s11053-023-10244-x
Aydin, A., and Basu, A. (2005). The Schmidt hammer in rock material characterization. Eng. Geol. 81 (1), 1–14. doi:10.1016/j.enggeo.2005.06.006
Bai, T., and Pollard, D. D. (2000). Fracture spacing in layered rocks: A new explanation based on the stress transition. J. Struct. Geol. 22 (1), 43–57. doi:10.1016/S0191-8141(99)00137-6
Bai, T., Pollard, D. D., and Gao, H. (2000). Explanation for fracture spacing in layered materials. Nature 403 (6771), 753–756. doi:10.1038/35001550
Bao, C. Y., Tang, C. A., Tang, S. N., and Ci, M. (2014). Numerical simulation and mechanism research of surface fracture equal-spacing subjected to cooling shrinking. Chin. J. Rock Mech. Eng. 33 (03), 549–557. doi:10.3969/j.issn.1000-6915.2014.03.013
Chang, X., Lu, J. Y., Wang, S. Y., Wang, S. R., and Liu, X. L. (2017). Formation of cracks in layered rock considering layer thickness variations. Geophys. J. Int. 210 (3), 1623–1640. doi:10.1093/gji/ggx270
Chang, X., Shan, Y. F., Zhang, Z. H., Tang, C. N., and Ru, Z. L. (2015). Behavior of propagating fracture at bedding interface in layered rocks. Eng. Geol. 197, 33–41. doi:10.1016/j.enggeo.2015.08.010
Chen, B., Chen, M., Li, Z. M., Wang, Y. H., and Diao, C. (2014). Propagation area evaluation of hydraulic fracture networks in shale gas reservoirs. Petroleum Explor. Dev. (06), 763–768. doi:10.1016/S1876-3804(14)60101-4
Cooke, M. L., and Underwood, C. A. (2001). Fracture termination and step-over at bedding interfaces due to frictional slip and interface opening. J. Struct. Geol. 23 (2), 223–238. doi:10.1016/S0191-8141(00)00092-4
Ding, W. L., Li, C., Li, C. Y., Xu, C. C., Jiu, K., Zeng, W. T., et al. (2012). Fracture development in shale and its relationship to gas accumulation. Earth Sci. Front. 19 (02), 97–105. doi:10.1016/j.gsf.2011.10.001
Fu, X. F., Gong, L., Su, X. C., Liu, B., Gao, S., Yang, J. G., et al. (2022). Characteristics and controlling factors of natural fractures in continental tight-oil shale reservoir. Minerals 12 (12), 1616. doi:10.3390/min12121616
Fu, X. F., Su, X. C., Gong, L., Wang, Q. Q., Gao, S., and Xie, Z. H. (2023). Control of faults and fractures on shale oil enrichment. Geoenergy Sci. Eng. 228, 212080. doi:10.1016/j.geoen.2023.212080
Gale, J. F. W., Laubach, S. E., Olson, J. E., Eichhubl, P., and Fall, A. (2014). Natural fractures in shale, a review and new observations. AAPG Bull. 98 (11), 2165–2216. doi:10.1306/08121413151
Gao, F. (2019). Use of numerical modeling for analyzing rock mechanic problems in underground coal mine practices. J. Min. Strata Control Eng. 1 (1), 013004. doi:10.13532/j.jmsce.cn10-1638/td.2019.02.009
Gong, L., Fu, X. F., Wang, Z. S., Gao, S., Jabbari, H., Yue, W. T., et al. (2019a). A new approach for characterization and prediction of natural fracture occurrence in tight oil sandstones with intense anisotropy. AAPG Bull. 103 (6), 1383–1400. doi:10.1306/12131818054
Gong, L., Gao, M. Z., Zeng, L. B., Fu, X. F., Gao, Z. Y., Gao, A., et al. (2017a). Controlling factors on fracture development in the tight sandstone reservoirs: A case study of. Jurassic-neogene in the kuqa foreland basin. Nat. Gas. Geosci. 28 (2), 199–208. doi:10.11764/j.issn.1672-1926.2016.12.003
Gong, L., Gao, S., Fu, X. F., Chen, S. M., Lyu, B. Y., and Yao, J. Q. (2017b). Fracture characteristics and their effects on hydrocarbon migration and accumulation in tight volcanic reservoirs: A case study of the xujiaweizi fault depression, Songliao Basin, China. Songliao Basin, China. Interpret. 5 (4), 57–70. doi:10.1190/INT-2016-0227.1
Gong, L., Gao, S., Liu, B., Yang, J. G., Fu, X. F., Xiao, F., et al. (2021). Quantitative prediction of natural fractures in shale oil reservoirs. Geofluids 2021, 1–15. doi:10.1155/2021/5571855
Gong, L., Liu, K., and Ju, W. (2023). Editorial: advances in the study of natural fractures in deep and unconventional reservoirs. Front. earth Sci. 10. doi:10.3389/feart.2022.1096643
Gong, L., Su, X. C., Gao, S., Fu, X. F., Jabbari, H., Wang, X. X., et al. (2019b). Characteristics and formation mechanism of natural fractures in the tight gas sandstones of Jiulongshan gas field, China. J. Petroleum Sci. Eng. 175, 1112–1121. doi:10.1016/j.petrol.2019.01.021
Gong, L., Yao, J. Q., Gao, S., Wei, B., Zeng, L. B., Fu, X. F., et al. (2018). The control of rock mechanical stratigraphy on tectonic fracture spacing. Geotect. Metallogenia 42 (06), 965–973. doi:10.16539/j.ddgzyckx.2018.06.002
Gong, L., Zeng, L. B., Du, J. Y., and Han, Z. R. (2015). Influences of structural diagenesis on fracture effectiveness: A case study of the cretaceous tight sandstone reservoirs of kuqa foreland basin. J. China Univ. Min. Technol. 44 (3), 514–519. doi:10.13247/j.cnki.jcumt.000263
Gross, M. R., and Eyal, Y. (2007). Throughgoing fractures in layered carbonate rocks. GSA Bull. 119 (11-12), 1387–1404. doi:10.1130/0016-7606(2007)119[1387:tfilcr]2.0.co;2
Helgeson, D. E., and Aydin, A. (1991). Characteristics of joint propagation across layer interfaces in sedimentary rocks. J. Struct. Geol. 13 (8), 897–911. doi:10.1016/0191-8141(91)90085-W
Ju, W., Zhong, Y., Liang, Y., Gong, L., Yin, S., and Huang, P. M. (2023). Factors influencing fault-propagation folding in the kuqa depression: insights from geomechanical models. J. Struct. Geol. 168, 104826. doi:10.1016/j.jsg.2023.104826
Katz, O., Reches, Z., and Roegiers, J. C. (2000). Evaluation of mechanical rock properties using a Schmidt Hammer. Int. J. Rock Mech. Min. Sci. 37 (4), 723–728. doi:10.1016/S1365-1609(00)00004-6
Kou, Z. L., Wen, L., Liu, Y. F., Shen, J. L., and Wang, K. H. (2022). Study on the failure characteristics of sandstone during uniaxial compression. Nonferrous Metals (Mining Section) 74 (1), 89–96. doi:10.3969/j.issn.1671-4172.2022.01.013
Larsen, B., Gudmundsson, A., Grunnaleite, I., Sælen, G., Talbot, M. R., and Buckley, S. J. (2010b). Effects of sedimentary interfaces on fracture pattern, linkage, and cluster formation in peritidal carbonate rocks. Mar. Petroleum Geol. 27 (7), 1531–1550. doi:10.1016/j.marpetgeo.2010.03.011
Larsen, B., and Gudmundsson, A. (2010a). Linking of fractures in layered rocks: implications for permeability. Tectonophysics 492 (1-4), 108–120. doi:10.1016/j.tecto.2010.05.022
Laubach, S. E., Fall, A., Copley, L. K., Marrett, R., and Wilkins, S. (2016). Fracture porosity creation and persistence in a basement-involved laramide fold, upper cretaceous frontier formation, green river basin, USA. U.S.A. Geol. Mag. 153 (5/6), 887–910. doi:10.1017/S0016756816000157
Laubach, S. E., Lamarche, J., Gauthier, B. D. M., Dunne, W. M., and Sanderson, D. J. (2018). Spatial arrangement of faults and opening-mode fractures. J. Struct. Geol. 108, 2–15. doi:10.1016/J.JSG.2017.08.008
Lyu, W. Y., Zeng, L. B., Cheng, S. Q., Lyu, P., Dong, S. Q., Hui, C., et al. (2021). Characterization methods of multi-scale natural fractures in tight and low-permeability sandstone reservoirs. Geol. Rev. 67 (02), 543–556. doi:10.16509/j.georeview.2021.02.020
Mi, L. J., Fan, H. J., Fan, T. G., Gong, L., Niu, T., Su, X. C., et al. (2023a). Development characteristics of multi-scale fracture network systems in metamorphic buried hills. Front. Earth Sci. 10. doi:10.3389/feart.2022.1108032
Mi, L. J., Fan, T. G., Fan, H. J., Niu, T., Gong, L., Su, X. C., et al. (2023b). Fracture development and controlling factors at metamorphic buried-hill reservoirs of Bozhong 19-6 gas field in Bohai Bay, East China. Front. Earth Sci. 10. doi:10.3389/feart.2022.1082439
Su, X. C., Gong, L., Fu, X. F., Gao, F., Zhou, X. P., Lu, Q., et al. (2023). Fracture distribution characteristics and effectiveness evaluation of tight sandstone reservoir of Chang 7 Member in Sanbian area, Ordos Basin. Earth Sci. 48 (7), 1–13. doi:10.3799/dqkx.2022.116
Tang, C. A., Tham, L. G., Lee, P. K. K., Yang, T. H., and Li, L. C. (2002). Coupled analysis of flow, stress and damage (FSD) in rock failure. Int. J. Rock Mech. Min. Sci. 39 (4), 477–489. doi:10.1016/S1365-1609(02)00023-0
Ullah, J., Li, H., Ashraf, U., Heping, P., Ali, M., Ehsan, M., et al. (2023). Knowledge-based machine learning for mineral classification in a complex tectonic regime of Yingxiu-Beichuan fault zone, Sichuan basin. Geoenergy Sci. Eng. 229, 212077. doi:10.1016/j.geoen.2023.212077
Wang, B., Lu, C., Huang, Z., and Hu, S. (2021). Experimental study on damage evolution characteristics of rock under triaxial rheological disturbance. J. Min. Strata Control Eng. 3 (4), 043028. doi:10.13532/j.jmsce.cn10-1638/td.20210525.001
Wang, R. F., Lyu, X. H., Guo, D. B., Su, H., and Huang, X. W. (2012). Fracture characteristics and main control factors of triassic sand reservoir in dongpu Sag. J. Jilin Univ. (Earth Sci. Ed. 42 (4), 1003–1010. doi:10.13278/j.cnki.jjuese.2012.04.014
Willis, B. (1894). The mechanics of appalachian struc-ture. Washington: US Government Printing Office, 222–224.
Zeng, L. B., Gong, L., Guan, C., Zhang, B. J., Wang, Q. Q., Zeng, Q., et al. (2022). Natural fractures and their contribution to tight gas conglomerate reservoirs: A case study in the northwestern sichuan basin, China. J. Petroleum Sci. Eng. 210, 110028. doi:10.1016/j.petrol.2021.110028
Zeng, L. B., Gong, L., Zu, K. W., Tang, X. M., Wang, T. C., Wang, C. G., et al. (2012). Influence factors on fracture validity of the paleogene reservoir, western oaidam basin. Acta Geol. Sin. 86 (11), 1809–1814. doi:10.3969/j.issn.0001-5717.2012.11.010
Zeng, L. B., Lyu, P., Qu, X. F., and Fan, J. M. (2020). Multi-scale fractures in tight sandstone reservoirs with low permeability and geological conditions of their development. Oil Gas Geol. 41 (03), 449–454. doi:10.11743/ogg20200301
Zhang, J. C., and Qi, Y. C. (2020). Impact of in-situ stresses on shale reservoir development and its countermeasures. Oil Gas Geol. 41 (04), 776–783. doi:10.11743/ogg20200411
Zhang, P., Xu, D., Fu, X., Xie, J., Dong, Y., and Zhang, X. (2022). Evaluation of hydraulic conductivity based on fault confinement studies. J. Min. Strata Control Eng. 4 (2), 023033. doi:10.13532/j.jmsce.cn10-1638/td.20211215.001
Zhao, L. Q., and Feng, J. W. (2018). Interrelationship study between rock mechanicalstratigraphy and structural fracture development. J. Shandong Univ. Sci. Technol. Nat. Sci. 37 (01), 35–46. doi:10.16542/j.cnki.sdkjzk.2018.01.004
Zhao, W. T., Hou, G. T., Sun, X. W., Ju, W., Shen, Y. M., Ren, K. X., et al. (2013). Influence of layer thickness and lithology on the fracture growth of clastic rock in East Kuqa. Geotect. Metallogenia 37 (4), 603–610. doi:10.16539/j.ddgzyckx.2013.04.003
Zhu, W., Niu, L., Li, S., and Li, S. (2019). Creep-impact test of rock:Status-of-the-art and prospec. J. Min. StrataControl Eng. 1 (1), 013003. doi:10.13532/j.jmsce.cn10-1638/td.2019.02.007
Keywords: mechanical stratigraphy, bedded rocks, fractures, development behavior, controlling factors
Citation: Su X, Gong L, Fu X, Wang Y, Gao S, Wang J, Qin X, Luo H and Bao T (2023) Impact of mechanical stratigraphy on fracture growth and propagation. Front. Earth Sci. 11:1253787. doi: 10.3389/feart.2023.1253787
Received: 06 July 2023; Accepted: 31 August 2023;
Published: 15 September 2023.
Edited by:
Guiting Hou, Peking University, ChinaReviewed by:
Muhsan Ehsan, Bahria University, PakistanHu Li, Southwest Petroleum University, China
Zhonghu Wu, Guizhou University, China
Copyright © 2023 Su, Gong, Fu, Wang, Gao, Wang, Qin, Luo and Bao. This is an open-access article distributed under the terms of the Creative Commons Attribution License (CC BY). The use, distribution or reproduction in other forums is permitted, provided the original author(s) and the copyright owner(s) are credited and that the original publication in this journal is cited, in accordance with accepted academic practice. No use, distribution or reproduction is permitted which does not comply with these terms.
*Correspondence: Lei Gong, a2Nnb25nbGVpQGZveG1haWwuY29t