- 1College of Environment and Civil Engineering, Chengdu University of Technology, Chengdu, Sichuan, China
- 2State Key Laboratory of Geological Disaster Prevention and Geological Environment Protection, Chengdu University of Technology, Chengdu, Sichuan, China
Many studies are currently focusing on the mechanical properties of granite from macroscopic and microscopic perspectives, but only several studies on the influence of rock mineral composition on the mechanical properties using a combined macro-micro assessment. The biotite granite in the Gaoligong Mountains was selected as the research object, and the variation strength characteristics at 20–130°C were obtained through uniaxial compression experiments. The rock’s uniaxial compressive strength and elastic modulus reached peak values at 40°C. The main mineral components of the rock determined by X-ray powder diffraction were quartz, biotite, albite, and potash feldspar. With Material Studio software, molecular dynamics simulations were carried out on the crystal models of quartz, biotite, albite, and potash feldspar at 20–130°C, and relevant mechanical parameters were calculated. The macroscopic and microscopic experimental data were compared and analyzed. The results show that: 1) due to the thermal expansion of minerals in the rock, the original cracks between particles are partially closed, and the sample strength is the largest at 40°C; 2) temperature mostly affects quartz and biotite phases, and the rock strength increases first and then decreases with the temperature increase. The mechanical properties of albite and potash feldspar are less affected by temperature; 3) macroscopic and microscopic experimental results show that the rock strength reaches the peak value in a certain temperature range. The obtained critical temperature is different because the rock sample also contains a small amount of argillaceous rock, siliceous rock, phyllite, and some siliceous and calcareous cement beside the main mineral composition; 4) The structural changes of quartz and biotite are affected by temperature. The bond length of the quartz crystal decreases while that of the biotite crystal first increases and then decreases with the temperature. The mechanism of the temperature influence on the increase of the rock strength is different for these two crystal phases, and it requires further exploration.
1 Introduction
Granite widely exists in crustal strata and is mainly composed of feldspar and quartz. It exhibits a high strength and stable structure, but under temperature influence, granite undergoes certain thermal damage, which changes the pore size, volume, rock composition, and microstructure of the original material, significantly affecting its mechanical properties. Exploring the impact of temperature-related microstructural changes of rocks on their macroscopic mechanical properties from a microscopic perspective and exploring the relationship between the microscopic mechanical properties and macroscopic mechanical properties can provide a theoretical foundation for predicting and preventing disasters such as rock bursts. Such studies have an important practical significance for solving rock engineering problems at high temperatures.
Chinese and foreign scholars have conducted studies on the mechanical properties of granite under different temperatures. In 1964, Lebedev and Khitaror studied the thermal physical properties of granite, including the determination of basic physical and mechanical parameters (Heuze, 1983; Lau et al., 1995), thermal cracking (Johnson et al., 1978), deformation mechanism (Vander Molen, 1981; Simpson, 1985), failure criterion, and constitutive equation (Heueckel et al., 1994). O Alm (Alm et al., 1985) analyzed the mechanical properties of granite after heat treatment at different temperatures and explored the micro-fracture process. Lin Muzeng (Lin, 1991) discovered that the Young’s modulus of gradesite, quartz trachyte, and other rocks sharply decreases below 300°C as the temperature increases. Du et al. (Du et al., 2004) studied the mechanical properties of granite treated at different temperatures and obtained the variation law of mechanical parameters, such as peak stress, peak strain, elastic modulus, and Poisson’s ratio of rocks with temperature. The results showed that a high temperature of up to 400°C subtly influenced the mechanical properties of granite. However, when the temperature exceeded 400°C, the mechanical properties of granite rapidly deteriorated, exhibiting a sharp decrease in peak stress (or strength) and elastic modulus and a rapid increase in peak strain. Xu (Xu, 2008) found that the mechanical properties of rock samples changed continuously under in-situ high-temperature treatment and showed a sudden change after heating and subsequent cooling, which was closely related to structural phase transformations. With the temperature increase, the brittleness of granite weakened, ductility increased, peak strength decreased, and the post-peak increased significantly. The mechanical properties of granite under high-temperature and low-temperature conditions are distinct. Tang Mingming (Tang et al., 2010) assessed the mechanical properties under low-temperature conditions. In uniaxial and triaxial tests, the compressive strength of slightly weathered granite increased as the temperature decreased in the low-temperature range, representing a nonlinear growth trend, a temperature limit value upon which the compressive strength tended to be stable was identified. The cohesion of micro-weathered granite increased with the temperature decrease under both dry and saturated conditions. The internal friction angle did not change significantly when the temperature decreased under dry conditions but increased with the temperature decrease under saturated conditions.
However, only several studies on the microstructural changes of granite under temperature influence are available in the literature. Gao (Gao et al., 2018) showed in the microscopic study of granite at different temperatures that the number of ink bottle-like pores in granite decreased while the number of parallel plate-like pores increased with the increase in temperature. The percentage of mesopores as a whole in the total pore volume showed an increasing trend. The total pore volume enlarged, and the connectivity was enhanced. Ji (Ji, 2008) determined the mechanical parameters, such as elastic modulus and hardness, of quartz and feldspar contained in the composition of Dagangshan granite minerals using micro- and nano-indentation tests, demonstrating that the elastic moduli of quartz and feldspar basically determine the overall elastic modulus of granite. Gao (Gao et al., 2021) analyzed the composition and micro-fracture characteristics of granite samples using X-ray diffraction and SEM tests, and the micro-fracture mechanism was correlated with the main mineral morphology.
The results of previous studies have largely promoted the research on the mechanical properties of granite rocks at different temperatures. However, the research mainly focused on the rock composition and strength changes, and less attention was paid to the microstructural changes of rock mineral components under temperature variation. Therefore, this paper investigates biotite granite at the Gaoligongshan tunnel site as the research object and addresses the relationship between the changes in the mineral composition structure of granite and mechanical properties at the micro-scale under high-temperature conditions.
2 Uniaxial compression experiments
Rock samples investigated in this study were prepared from biotite granite, and the uniaxial compression tests were performed using an MTS815Teststar program-controlled servo rigid testing machine. The whole stress-strain curves of rock samples were obtained.
The test temperature was set to 20, 40, 60, 90, and 130°C. The investigated sample was heated at a constant temperature in s DHG-9203A electric constant-temperature blast dryer. The sample was retained 2 h at the target temperature. At the beginning of the test, the axial displacement was controlled at a value of 0.1 mm/min. When deformation reached the yield stage, the loading rate was controlled by lateral deformation, and the value was 0.03 mm/min until the specimen was destroyed.
The mechanical and deformation parameters, such as compressive strength and elastic modulus, were determined through the stress-strain curve of the rock samples at different temperatures, and the failure mode and properties after reaching the peak were investigated.
2.1 The whole stress-strain process curve
From Figure 1 can be seen that the rock samples at 20°C and 60°C exhibit type II failure, while the rock samples at 40, 90, and 130°C show type I failure. Due to the inhomogeneity and discontinuity of the samples, brittle failure characteristics appear in the overall temperature range of 20°C–60°C. The test also shows that the same lithology exhibits different strength characteristics and failure modes at different temperatures, which is related to the influence factors, such as temperature, on the sample’s storage capacity of elastic strain energy and stress environment.
2.2 Elastic modulus variation with temperature
Figure 2A shows that the elastic modulus first increases and then decreases with temperature. The elastic modulus increases by 17.4 GPa at 40°C relative to the value at 20°C, then decreases by 17.6 GPa at 60°C compared to the value at 40°C; further, the elastic modulus at 90°C is 4.8 GPa higher than at 60°C, and 5.0 GPa higher at 130°C than at 90°C. Thus, the elastic modulus of rock samples slowly increases with the temperature increase, while the structural stiffness gradually increases. The elastic modulus reaches the peak at 40°C.
2.3 Strength characteristics
The uniaxial compression test at different temperatures allowed for the determination of the temperature influence on the uniaxial compressive strength of rock samples, as shown in Figure 2B. The uniaxial compressive strength first increases and then decreases with temperature. At 40°C, the uniaxial compressive strength of rock increases by 15.54% compared with that at 20 °C; at 60, 90, and 130°C, it decreases by 4.67, 3.74, and 10.28% compared to that at 40°C, i.e., the rate of decrease gradually increases. These results imply that crystalline minerals inside rocks become softer as the temperature rises. At 40°C, the uniaxial compressive strength reaches the maximum. The comprehensive analysis shows that the thermal expansion of the rock’s internal minerals at a certain temperature leads to the partial closure of original cracks between particles, practically densifying the rock and increasing the stiffness and strength compared to non-heated samples. These results need to be further verified via micro-mechanical assessment.
2.4 X-ray powder diffraction analysis
We selected five rock samples for testing test to determine the mineral composition of the rock using diffractometry. The results show the highest proportion of quartz, followed by plagioclase, mica, potash feldspar, tremolite, chlorite, pyrite, etc. According to the analysis report of rock and mineral identification, the plagioclase in the rock samples is mainly medium-acidic plagioclase, so it is concluded that it is mainly albite.
2.5 Microscopic simulations
The mineral composition of this group of samples was obtained from X-ray diffraction experiments. However, from the micro-perspective, the influence of temperature change on the mechanical properties of minerals needs to be explored. Therefore, we used the Forcite module in Material Studio, a material calculation software of Accelrys, to conduct molecular-scale mechanical numerical simulations of biotite, quartz, potash feldspar, and plagioclase in four main minerals of the tested rock samples.
The corresponding crystal model was first downloaded from the Material Projects crystal library, and the model cif file was imported into Material Studio software. Chemical keys were added while the structure was optimized. Then, the molecular dynamics simulations (Dynamic) of the model were carried out considering 20, 40, 60, 90, 130°C, and then the structure was optimized again. Finally, the mechanical properties of the model were calculated to get the final results.
During the modeling process, the supercell structure of each crystal 5*5*5 (biotite is 3*3*3) is established to clarify the crystal structure change. Figure 3 shows quartz, biotite, potassium feldspar, and albite crystal models. The NPT ensemble and COMPASS force field were used for calculation in the molecular dynamics simulation. The calculation step was 5000. The obtained results are convergent and reliable. Quartz, potassium feldspar, biotite, and albite crystal-related parameters are selected as follows (Table 1).
2.5.1 α-Quartz crystal
Since α-quartz is ubiquitous in granite, the α-quartz crystal was selected as a simulation object. Figures 4, 5 show that the bulk modulus of quartz increases first and then decreases with temperature, reaching its maximum at 90°C (363.15 K). At this time, the volume change of the crystal is the smallest, while the rigidity is the greatest. The shear modulus does not change significantly during the heating process at 20°C–90°C, and the maximum value increases sharply at 90°C (363.15 K), indicating that the crystal adhesion is enhanced. The shear modulus decreases gradually after heating. The Young’s modulus in the y-direction first increases and then decreases with temperature, reaching the peak value at 60°C (333.15 K). Young’s moduli in x- and z-directions first increase and then decrease with temperature, reaching the peak value at 90°C (363.15 K), which indicates that the crystal stiffness is the largest at this temperature point.
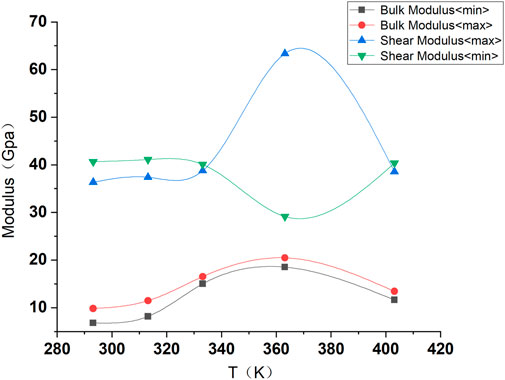
FIGURE 4. Volumetric modulus and Shear modulus change of quartz crystal at 20°C–130°C (293.15–403.15 K).
2.5.2 Potassium feldspar crystal
The related parameters of potassium feldspar are shown in Table 1. As shown in Figures 6, 7, the bulk modulus and Young’s modulus of the potassium feldspar crystal have no obvious change with the increase in temperature. The crystal compressibility decreases in the range of 20°C–40°C (293.15–313.15 K) and increases between 40°C and 60°C (313.15–333.15 K).
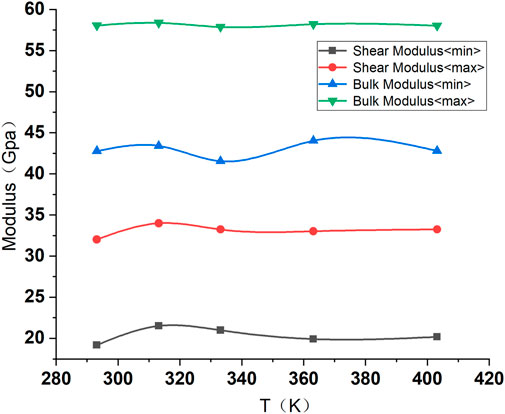
FIGURE 6. Change of volume modulus and Shear modulus of potassium feldspar crystal at 20°C–130°C (293.15–403.15 K).
2.5.3 Biotite crystals
The composition of biotite is complex, and it may easily undergo extensive isomorphic transformations. Therefore, the chemical composition of biotite produced in different rocks greatly varies. Most acidic and alkaline rocks contain biotite; generally, biotite in acidic and alkaline magmatic rocks has a high content of FeO and a low content of Mg. Since this group of rocks belongs to granite, and the content of Fe in rocks is relatively high, KMg2AlFeSi3 (HO6) 2 is selected as a research model. As shown in Figures 8, 9, the bulk modulus of the biotite crystal increases first and then decreases during the heating process, reaching the maximum at 60°C (333.15 K). Under such conditions, the crystal volume change is the smallest, while the rigidity is the highest. The shear modulus first increases, then decreases between 60°C and 90°C (333.15–363.15 K), and remains constant between 90°C and 130°C (333.15–403.15 K), demonstrating that the crystal bonding force rises initially, then falls, and rises again. The bonding force decreases at 60°C–90°C (333.15–363.15 K), exhibiting the lowest strength. In the x-direction, the Young’s modulus first increases and then decreases, reaching the peak at 40°C–60°C (313.15–333.15 K). In the y-direction, the Young’s modulus shows repetitive increasing-decreasing sequences and peaks at 90°C–130°C (363.15–403.15 K). In the z-direction, the Young’s modulus increases slowly, and the change rate is not significant.
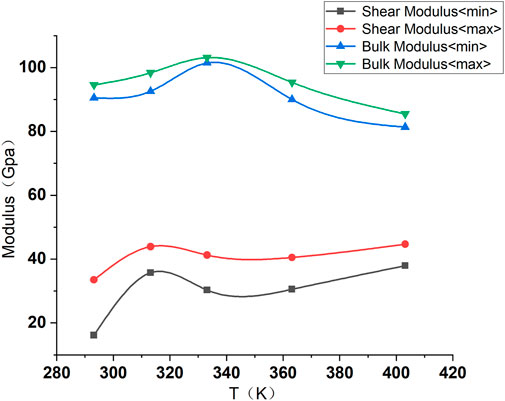
FIGURE 8. Change of volume modulus and Shear modulus of biotite crystal at 20°C–130°C (293.15–403.15 K).
2.5.4 Albite crystal
According to the rock and mineral identification report, the granite in this group contains acidic and medium-acidic plagioclase, so it is preliminarily assessed as albite. As shown in Figures 10, 11, the volume modulus of the albite crystal remains stable after increasing during heating, being in the range of 50–52 GPa. There is no significant change in shear modulus. In the x-direction, the Young’s modulus initially decreases and then remains stable. In the y-direction, the Young’s modulus increases in the beginning and then remains stable. In the z-direction, the Young’s modulus exhibits no obvious change.
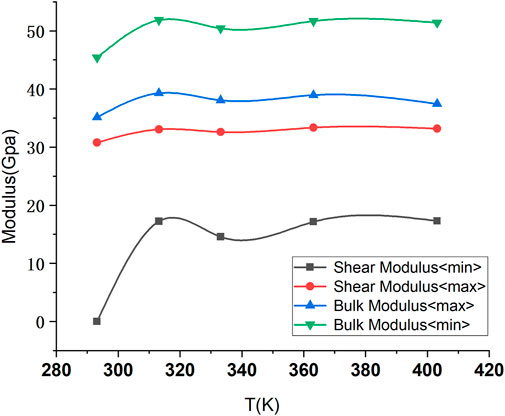
FIGURE 10. Change of Bulk modulus and Shear modulus of albite crystal at 20°C–130°C (293.15–403.15 K).
3 Discussion
1) According to the results of the uniaxial compression experiments and mineral microscopic numerical simulations of the investigated rock samples, it can be seen that the elastic modulus first increases and then decreases with the increase of temperature, reaching the peak value at about 40°C (313.15 K). Similarly, the uniaxial compressive strength first increases and then decreases as the temperature rises, having the largest value at 40°C (313.15 K). The X-ray powder diffraction analysis indicated four main mineral components in the sample, namely, quartz, biotite, potash feldspar, and albite. In the microscopic numerical simulations of minerals, the mechanical properties of quartz and biotite change greatly under the influence of temperature. The strength reaches the peak value at 60°C–90°C. The albite and potash feldspar phases are less affected by temperature.
2) The crystal compressibility decreases to the minimum value when the temperature is 60°C–90°C (333.15–363.15 K), while the material stiffness has the maximum value (Figure 12). Therefore, the compressive strength and strength of quartz crystals are at their highest between 60°C and 90°C, and temperature fluctuations significantly affect the mechanical properties of quartz minerals. The compressibility of potassium feldspar, biotite, and albite is less affected by temperature.
3) Macroscopically, due to the temperature increase, the original cracks between particles are partially closed, which increases the overall stiffness and strength of the rock, with the mechanical strength peaking at 40°C. Microscopically, the two mineral components, i.e., quartz and biotite, are greatly affected by temperature, and the mechanical strength reaches the peak value at about 60°C. The reason for the difference between the two sets of experimental results is that the rock samples contain a small amount of argillaceous rock, siliceous rock, phyllite, and some siliceous and calcareous types of cement beside the main mineral components. The mechanical response mechanism of the entire rock and single minerals under temperature still differs, which needs further exploration.
4) The mechanism behind the influence of heating on the mechanical properties of granite is mainly related to the thermal expansion and different thermo-elastic properties of anisotropic particles in different crystallization directions, which causes uncoordinated thermal expansion across the particle boundaries, resulting in tensile or compressive stress between particles or within particles. As the temperature increases, the stress between particles or within particles increases further, resulting in microcrack formation or expansion and widening and connection of primary cracks in granite, which macroscopically shows the deterioration of the mechanical properties of granite.
5) Since quartz and biotite are greatly affected by temperature, according to the results of numerical simulation experiments, the Si-O bond length in quartz minerals decreases with temperature, as shown in Figure 13. The Si-O bond length in biotite first increases and then decreases, as shown in Figure 14.
It shows that the bond length of quartz minerals decreases with temperature, and the spatial structure is changed. We conclude that the mineral crystal may be in a compression state during the heating process. The strength gradually grows because the quartz crystal is reasonably stable and the change in mechanical properties. With the temperature increase, the bond length of the biotite crystal first increases and then decreases, indicating that the spatial structure also changes. The difference is that the biotite crystal may undergo thermal expansion. During the heating process, the expansion of the interlayer structure favors densification. Macroscopically, it leads to a dense structure and increased rock strength.
4 Conclusion
1) According to the uniaxial compression test results, the elastic modulus first increases and then decreases with temperature, and the structural stiffness of rock samples gradually becomes higher. At 40°C, the elastic modulus reaches the peak value. Similarly, with the temperature increase, the uniaxial compressive strength first increases and then decreases because the thermal expansion of minerals inside the rock leads to the partial closure of original cracks between particles, increasing the overall stiffness and strength of the rock.
2) According to the numerical simulation results of the MS molecular dynamics module, the samples contain four minerals: quartz, albite, potash feldspar, and biotite. The strength of quartz and biotite is most affected by temperature, which first increases and then decreases with temperature. Albite and potassium feldspar are not affected by temperature.
3) In the uniaxial compression test, the strength reaches the peak temperature between 40°C–60°C; in the MS numerical simulation, the temperature peaks in the 60°C–90°C range. The difference originates from the fact that rock samples also contain other compositions, including a small amount of argillaceous rocks, siliceous rocks, phyllite, and some siliceous and calcareous types of cement.
4) The rock structure changes when quartz and biotite crystals are affected by temperature. The bond length of quartz crystals decreases, while the bond length of biotite crystals first increases and then decreases with temperature. The mechanism behind the influence of these two phenomena on the rock strength increase is different and needs further exploration.
Data availability statement
The raw data supporting the conclusions of this article will be made available by the authors, without undue reservation.
Author contributions
YH: Conceptualization, Methodology, Software, Investigation, Formal Analysis, Writing—Original Draft, TL: Conceptualization, Review and Editing. All authors contributed to the article and approved the submitted version.
Conflict of interest
The authors declare that the research was conducted in the absence of any commercial or financial relationships that could be construed as a potential conflict of interest.
Publisher’s note
All claims expressed in this article are solely those of the authors and do not necessarily represent those of their affiliated organizations, or those of the publisher, the editors and the reviewers. Any product that may be evaluated in this article, or claim that may be made by its manufacturer, is not guaranteed or endorsed by the publisher.
References
Alm, O., Jaktlund, L. L., and Shaoquan, K., The influence of microcrack density on the elastic and fracture mechanical properties of Stripa granite. Phys. Earth Planet. Interiors, 1985, 40(3): 161–179. doi:10.1016/0031-9201(85)90127-x
Du, Shouji, Liu, Hua, and Zhi, Hongtao, Experimental study on mechanical properties of granite after high temperatur. J. Rock Mech. Eng., 2004, 23 (14): 2359–2359. doi:10.3321/j.issn:1000-6915.2004.14.010
Gao, M., Li, T., Zhu, J., Yin, H., and Yang, Y., An analysis of relationship between the microfracture features and mineral morphology of granite. Adv. Civ. Eng., 2021, 2021(5): 1–6.doi:10.1155/2021/4765731
Gao, Hongmei, Meng, Liyan, and Yang, yue., Microstructure and pore size distribution characteristics of granite at different temperatures. J. Heilongjiang Univ. Sci. Technol., 2018, 028 (002): 185–188. doi:10.3969/j.issn.2095-7262.2018.02.012
Heueckel, T., Peano, A., and Pellegrint, R., A constitutive law for ther-mo-plastic behavior of rocks: An analogy with clays. Surv. Geophys, 1994, 15: 643–671. doi:10.1007/BF00690178
Heuze, F. E., High-temperature mechanical, physical and thermalproperties of granitic rocks-a review. Int. J. Rock Mech. Min. Sciand Geomech. Abstr., 1983, 20(1): 3–10.doi:10.1016/0148-9062(83)91609-1
Ji, Jingchen. 2008, Physical and mechanical properties and macroscopic and microscopic mechanical properties of granite. Anhui University of Technology. Ma'anshan, China
Johnson, B., Gangi, A. F., and Handin, J., Thermal cracking of rock sub-ject to slow, uniform temperature changes. Proc. 19th U. S. SympRock Mech., 123 (3), 1978. 259–267.
Lau, J. S. O., Gorski, B., and Jackson, R., The effects of temperature andwater saturation on mechanical properties of Lac du Bonnet pinkgranite. 8th International congress on Rock Mech, Tokyo, Japan, 1995.
Lin, Muzeng. Rock thermophysics and its engineering applications. Chongqing University Press, Chongqing, China, 1991.
Simpson, C., Deformation of granitic rocks across the brittle-duc-tile transition. J. Struct. Geol., 1985, 7: 503–511.doi:10.1016/0191-8141(85)90023-9
Tang, Mingming, Wang, Zhiyin, and Sun, Yili, Experimental study on mechanical properties of granite under low temperature. Rock Mech. Eng., 2010, 29 (004): 787–794.
Vander Molen, I., The shift of the α-β transition temperature of quartz associated with the thermal expansion of granite at high pressure. Tectonophysics, 1981, 73: 323–342.doi:10.1016/0040-1951(81)90221-3
Keywords: rock mechanics, granite, microstructure, numerical simulation, temperature
Citation: Huang Y and Li T (2023) On the relationship between the mechanical strength and mineral microstructure of biotite granite under temperature influence. Front. Earth Sci. 11:1209433. doi: 10.3389/feart.2023.1209433
Received: 20 April 2023; Accepted: 30 May 2023;
Published: 09 June 2023.
Edited by:
Lidong Dai, Chinese Academy of Sciences, ChinaReviewed by:
Hao Rao, China University of Geosciences Wuhan, ChinaHongrui Xu, Southwest Jiaotong University, China
Copyright © 2023 Huang and Li. This is an open-access article distributed under the terms of the Creative Commons Attribution License (CC BY). The use, distribution or reproduction in other forums is permitted, provided the original author(s) and the copyright owner(s) are credited and that the original publication in this journal is cited, in accordance with accepted academic practice. No use, distribution or reproduction is permitted which does not comply with these terms.
*Correspondence: Tianbin Li, bHRiQGNkdXQuZWR1LmNu