Corrigendum: Influence of the Yucatan earthquake event
- Department of Earth and Space Sciences, Southern University of Science and Technology, Shenzhen, China
In recent years, seismic wave effects caused by meteorite impacts have been widely observed. The meteorite impact event that occurred 66 million years ago is one of the most famous impact events in Earth’s history. The influences of the seismic wave field generated by this collision event on the solid Earth itself is worth exploring. Therefore, this study initially estimated the seismic source parameter information based on the multiring structure of the meteorite crater, and then simulates the seismic event. The results of this study provide a possible explanation for the formation of the Earth’s tectonic plates. The findings of this study suggest that the seismic wave field generated by the meteorite impact event 66 million years ago may have caused the destruction of the Solid Earth, leading to the formation of the boundary between the Indian Ocean and the Pacific Plate. Simultaneously, this study has important significance for inspiring the development of new geoscientific methods.
1 Introduction
Collisions between objects, such as those that occur in nuclear reactions and due to the generation of gravitational waves, widely occur in nature. Moreover, collisions between meteorites and planets are common in solar systems (Bierhaus et al., 2022; Mark et al., 2022; Kimi and Vijayan, 2023). Among the many previous celestial collision events, the Chicxulub impact event that occurred 66 million years ago has been widely studied (Alvarez et al., 1980; Goderis Steven et al., 2021; Ross et al., 2022; Nixon et al., 2022). The present results show that the main reason for the extinction of dinosaurs is that a change in the Earth’s environment was triggered 66 years ago when a meteorite impacted the Earth (Kring, 1997; Schulte et al., 2010; Kunio et al., 2016; Morgan et al., 2022). In addition to causing volcanic eruptions, tsunamis (Hull Pincelli et al., 2020), worldwide wildfires (Venkatesan and Dahl, 1989) and other secondary disasters, this meteorite impact also directly produced strong seismic effects (Kim et al., 2022).
A weak seismic wave field can be used to study the internal structure of a planet (Stahler et al., 2021), while a strong seismic wave field usually results in intense damage (Caglar et al., 2023). The seismic wave field is primarily affected by the physical parameters of the source and the underground medium. For seismic sources, the seismic energy, source type, source direction, depth and source time function are important components to consider.
Regarding the source energy of the Chicxulub event, previous studies have shown that the source energy released by the impact was approximately 5×1023 joules (Morgan et al., 1997). Regarding the source direction, numerical experiments show that the meteorite impacted the Yucatan Peninsula at an angle of approximately 45–60 degrees, approaching from the northeast (Collins et al., 2020). However, the source depth and source time function are still unclear.
Nevertheless, the source depth and source time function can be estimated. The method of estimating the seismic time function using near-field seismic waveform information is widely used in the field of seismology (Isadora et al., 2020). For most meteorite impact events, the source time function can normally be approximated as a delta function (Holsapple and Schmidt, 1987). However, due to the seismic wave absorption and attenuation by the existing underground medium, the seismic source time function can generally be approximated as a Ricker wavelet or a triangular function (Ricker, 1977). The dominant frequency of the function is the parameter to be determined. The frequency of large impact events is usually concentrated in low-frequency components (Teanby and Wookey, 2011). To obtain this parameter, we analyzed the multiring structure of the crater and found that this structure reflects a regular ground wave phenomenon due to the presence of excessive energy during the seismic body wave propagation. In this study, two adjacent rings are considered to be the density extremum caused by the same peak frequency. Usually, the corresponding seismic parameters can be determined by solving the body wave equation in the frequency space domain, then setting different parameters, and conducting comparative tests. Basic equations in the field of natural science, such as the acoustic wave equation, light wave equation and seismic body wave equations, they usually have the same form. Li and Chen., 2021 efficiently solved this equation.
After obtaining the above approximate source parameter information, we can conduct research on global seismology to quantitatively explore the influence of the seismic effect generated by this event on both the interior and exterior of the Earth.
2 Data acquisition
An important difficulty in the study of events 66 million years ago is the acquisition of actual data. The direct evidence of meteorite impact mainly comes from meteorite craters.
Since the Chicxulub event occurred, 66 million years have elapsed, and there exists no current seismic data available that can be used to extract the source information from that event. However, geophysical exploration shows that the area retained a multiring structure with a diameter of approximately 180 km (Campos et al., 1997, Morgan et al., 2011; Gulick et al., 2013; Sweeney, 1978). The diameter of the inner ring of the multiring structure is approximately 70 km, and the diameter of the second ring is approximately 100 km at its widest point (Hildebrand et al., 1991). The multiring structure is mainly attributed to variations in the existing medium density caused by the propagation of the compressional wave. The two adjacent peak loops can be regarded as a wave cycle. The exact amplitude of the multiring structure will have changed over 66 million years due to gravity and other effects, but the wavelength information of the multiring structure can be used to estimate the frequency of the seismic source time function of the collision event.
The extraction of data on the multiring structure of meteorite craters in this study are based on previous measurements of gravity data (Hildebrand et al., 1991). The gravity data results mainly reflect changes in the density of the medium. To obtain relatively large amounts of reliable data, this study mined corresponding multiring structures and waveform diagrams from previous articles. Here, the ginput function of MATLAB is Primarily used for data extraction.
The multiring structure and waveform of the crater are shown in Figures 1, 2.
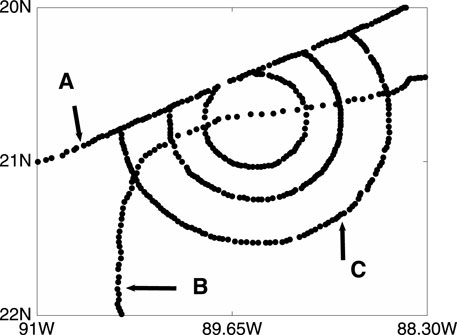
FIGURE 1. Schematic diagram of the crater multi-ring structure of the crater (Hildebrand et al., 1991), in which (A) represents the waveform data acquisition system, (B) represents the fracture caused by meteorite impact, and (C) represents the crater multi-ring structure, there are three multiring structures here, the first ring has a diameter of approximately 70 km, and the second ring has a diameter of approximately 100 km. These two circles represent wave peaks and can be considered as a waveform cycle.
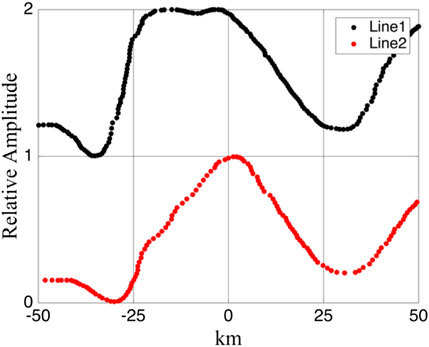
FIGURE 2. Waveform maps of the same region extracted from the survey lines obtained in different articles (Sweeney, 1978; Hildebrand et al., 1991), the wavelength values of Line1 and Line2 are basically the same.
In addition to the multiring structure of the crater, the waveform results of the crater can also be used as an additional source of information in order to constrain the source information. The waveform results obtained from previous data are as shown in Figure 2.
Based on the above information, we can perform wave field simulations to obtain the source information.
3 Source parameter estimation
Generally, we can use the waveform inversion method to invert the source parameters (Simute et al., 2023). However, due to the limitations of the actual data that is available, and the risk of multiple solutions for parameters with limited data constraints, we use the data fitting method to study this problem. During the data fitting process, the wavelength information of the multiring structure of the meteorite crater is mainly affected by the source depth, dominant frequency, and of the medium wave velocity. The simulation of the multi-ring structure of a meteorite crater is shown below.
Firstly, we can approximate the corresponding wavelength range based on the relationship between wavelength, wave velocity, and frequency as follows.
Where
In order to further estimate the relatively accurate source information, we can discuss the results. The rock structure of shallow surface craters is complex (Riller et al., 2018), but this study mainly focuses on low-frequency large-scale structure. To obtain the corresponding source depth. Here, we set the medium velocity to 1,500 m/s and the frequency to 0.1 Hz. Then, set different source depths for calculation. The source depth changes from 0 km to 30 km. The calculation result (Figure 3) shows that when the source is set at the surface, the first ring appears at the source, and as the depth increases, the diameter of the first ring gradually increases. Therefore, we can use the first ring diameter information to constrain the source depth information.
Next, we consider the influence of frequency on the multi-ring structure. At this time, we set the medium velocity to 5,000 m/s, change the frequency from 0.1 Hz to 0.25 Hz, and set the source depth to 20 km. The calculation result (Figure 4) shows that as the calculation frequency gradually increases, the multi-ring structure becomes increasingly dense, which manifests as a shorter wavelength. This information can be used to extract the approximate source dominant frequency information more accurately from the waveform information shown in Figure 2.
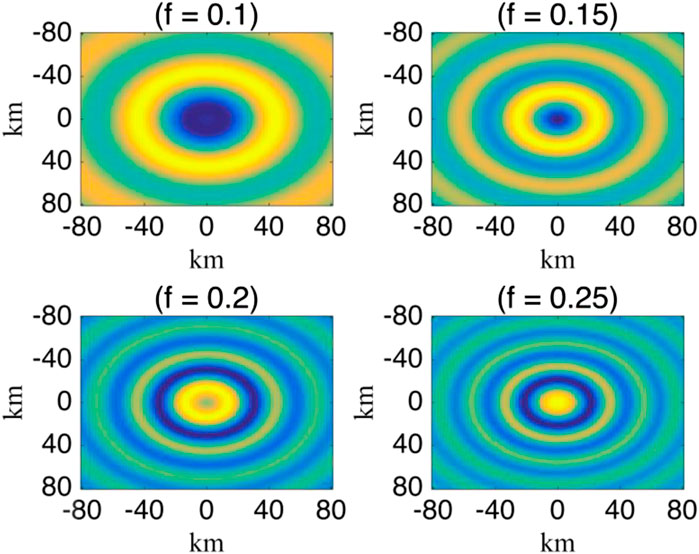
FIGURE 4. The impact of different frequencies on the structure of the multiple circles of the crater.
Finally, considering the impact of the wave velocity on the circular structure, the dominant frequency was set to 0.1 Hz, and the wave velocity was changed from 1,500 m/s to 3,000 m/s, and the source depth was set to 20 km. The calculation result is shown in Figure 5.
After discussing the influence of different source parameters on the structure of the crater, we compared the crater multiring structure, actual waveform information, and theoretical simulation results. In the actual simulation, the determined medium velocity of the formation was 5 km/s, based on the actual crustal velocity model (Dziewonski and Anderson, 1981). The comparison results are shown in Figure 6. The research results show that when the source depth is 20 km and the calculated frequency is 0.15 Hz, the simulated theoretical wavelength results are closest reconstruction of the actual results. At the same time, some scholars believe that this density change is due to the different thicknesses of the layers of deposited caused by fluctuations in the seismic shear wave, which resulted in a change in density (Morgan et al., 2022). For seismic shear waves, the main difference is that the wave velocity can vary to some extent, but for the simulated global impact of seismic fluctuations caused by meteorite impacts, such as those that are generated from a purely natural phenomena, this source parameter has little influence on the results.
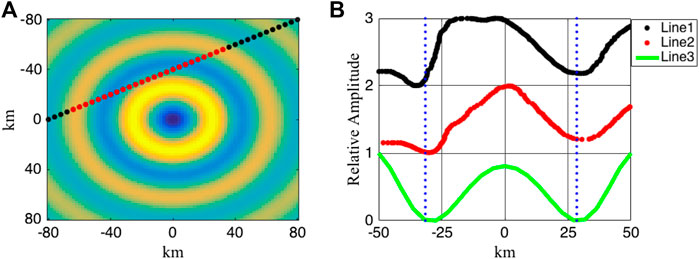
FIGURE 6. (A) Simulated crater multiring structure, (B) Simulated waveform, noted as Line3 and the actual waveform comparison results.
Then, the corresponding source time function can be calculated.
4 Seismic wave effects on the solid earth
To estimate the harm caused by this event, relevant research was conducted on the source. For the meteorite impact process, previous theoretical analysis shows that the process is an external force impact problem (Yang and Chen., 2022). Therefore, the process is discussed first. For a force source, it can be expressed as
For the impact external force impact problem, we can use the impulse theorem to solve it. During the impact process, meteorites are mainly affected by the formation reaction force and the gravitational force of the Earth. The product of the gravitational force and time are negligible relative to the product of the impact velocity and mass of the meteorite, so it is approximately written as follows:
If the Rake wavelet is approximated to a triangular function, the external force is approximately
In the process of using seismological methods to explore the influence of a meteorite impact on solid earth, we accidentally found that the area located beneath the impact of the Hiksurub meteorite would break due to the action of seismic waves, and the break showed clear similarities with the boundary between the Indian plate and the African plate (Figure 7).
As one of the four fundamental theories in the field of natural science in the 20th century, plate tectonics are as important to earth science as the theory of relativity, quantum mechanics, and molecular biology are to the fields of physics and biology (Zheng, 2018). Its development is of great significance to the further development of geology, geochemistry, seismology, geodynamics, rock physics, and other disciplines (Holder et al., 2019). Exploring its initiation mechanism and the timing period of events have always been one of the most innivative of questions to investigate in the field of earth science (Wan et al., 2020).
The theory of plate tectonics originated from the hypothesis of continental drift and the theory of seafloor spreading (Cwojdzinski, 2017). According to the theory of plate tectonics, the lithosphere is rigid relative to the asthenosphere, beneath which lies the low viscosity asthenosphere (Conrad and Lithgow-Bertelloni, 2002). The lithosphere is not a uniform entity, but contains directionally heterogeneous characteristics and is divided into large and small blocks by many active zones, such as mid-ocean ridges, trenches, transform faults, Earth sutures, and continental rifts. The interior of the plates are stable, while the edges and joint zones of the plates are active zones on the Earth’s surface, with strong tectonic movement (Ouchi, 1985), magmatic activity (Pilger, 1984), volcanic activity (Canon and Walker, 2004), metamorphic rock (Holder et al., 2019), and seismic activity (Maouche et al., 2019). These active zones are also very favorable mineralization zones (Yang et al., 2022). Second, the lithospheric plates are themselves active. During continental drift, some plates move away from each other, and some collide with each other to form various geological structures, such as the Mid-Ocean Ridge (Macdonald et al., 1988) and the Alpine Himalayan Fold Belt (Ali et al., 2019). Major volcanic activity zones and major deep seismic zones around the world are also mainly distributed in these plate fractures zones (Barrier et al., 2021).
Plate tectonics are important for the field of seismology. The vast majority of earthquakes in the world are mainly concentrated in the seismic zones. Seismic zones refer to regular banded areas where earthquakes are concentrated, and these seismic zones principally coincide with the tectonic plate edges (Dewey, 1972). There are three main seismic zones on the Earth, namely, the Pacific Rim seismic zone (Yin et al., 2019), the Eurasian seismic zone (Tang et al., 2020), and the oceanic ridge seismic zone (Kagan, 2003). The Pacific Rim seismic belt (Wu et al., 2019) is mainly distributed on the continental margin of the Pacific Ocean. This seismic zone is the primary earthquake area of occurrence area on Earth, where most catastrophic earthquakes with magnitude 8 or above and global earthquakes are concentrated (Yin et al., 2019). In addition, seismic zones are also the main volcanic activity zones in the world. The second largest seismic zone in the world is the Eurasian seismic zone (Wu et al., 2019). There is also much volcanic activity in this area. Historically, many large earthquakes have occurred in this area. This seismic zone is widely distributed and basically covers the Eurasian and African continents. Ocean ridge seismic zones (Wu et al., 2019) are mainly distributed in the Pacific, Atlantic, and Indian oceans, including almost all of the ocean ridge tectonic regions.
Therefore, the theory of plate tectonics involves almost all topics that exist in the field of solid earth science. The plate structure is a sign that the Earth is different from other planets. Due to plate tectonics, materials within the Earth can interact and circulate between circles. This process is beneficial to Earth becoming the current blue livable planet (Stern and Leybourne, 2016). Exploring the initiation mechanisms and timing of events are of great significance to the development of the geosciences.
Regarding the starting time of plate tectonics, based on geological rocks, the possibility that plate tectonics began 4.44 billion to 1 billion years ago has been analyzed (Stern and Leybourne, 2016; Johnson et al., 2017; Weller and Stonge, 2017; Stern, 2018). According to the paleomagnetic evidence, this time period of initiation was advanced to having occurred 800 to 600 million years ago (Piper, 2013). Therefore, it can be considered that the of the global movement plate tectonics do not necessarily start at the same time, and varying start times of different plate tectonic may accompany different time periods of the Earth’s evolution. However, there is currently no detailed study on what forces caused the initiation of plate tectonics.
Currently, it is generally believed that there are two possible mechanisms for the initiation of plate tectonics, one is spontaneous generation, and the other is that they were induced by external factors (Zhang, 2022). Regarding spontaneous generation, the possibility of this situation was discussed through numerical simulation experiments (Gerya, 2014). However, this situation needs to reflect the existence of different densities that exist between different plates, and there are also weak zones between plates, which gradually evolve into subduction boundaries. Regarding the theory that tectonics were induced by external factors, some scientists have considered the possibility of early meteorite impacts on the Earth leading to meteorite impacts inducing plate tectonics. By explaining the origin of the Tarsi uplift on Mars, they have speculated on the possibility of meteorite impacts leading to the origin of the Earth’s plate tectonics (Yin, 2012; Maruyama et al., 2018). However, the above theory lacks quantitative constraints and corresponding direct evidence.
Figure 8 shows that due to the destructive effect of the strong seismic wave field energy on the solid earth, an obvious fault anomaly occurs in the Indian Ocean region, and the location of this fault anomaly is very consistent with the boundary of the earth plate. Therefore, we think that the meteorite impact event 66 million years ago may be the cause of this plate formation. To demonstrate that this fracture affects the entire lithosphere, we calculated seismic displacement results at different depths (Figure 8).
The research results show that this kind of fault has almost spread to the entire lithosphere, which may induce the formation of plates in the region. Of course, in addition to being affected by the seismic source, this calculation result can also be influenced by the Earth model. Here, the S362ANI model is used. Sixty-five million years ago, except for the Atlantic region, the Earth model was basically consistent with the current model (Merdith et al., 2021).
5 Conclution and discussion
Based on seismological methods, this study quantitatively explored the impact of seismic wave effects that were generated by meteorite impacts over 66 million years ago on the solid Earth itself. The research results show that this event promoted the formation of the Indian Ocean plate. This result is of great significance for improving our understanding of spatial distribution patterns of the globe disaster risk from outer space, as well as for the development of plate tectonics theory.
This study has also highlighted some unresolved issues in the field of earth science.
1. In addition to the formation of plate tectonics, meteorite impacts can also form some special internal and external anomalies of the Earth, but existing theories cannot well describe the dynamic formation process of these phenomena. In theory, the best mathematical and physical description method for this study should be the application of geodynamics, which describe the process of meteorites impacting the Earth from the perspective of earthquake sources and geodynamics, as well as their infulence on the progression of future tectonic changes on Earth. However, the existing geodynamic methods mainly describe the Earth’s change process on a large time scale. For the rapid external force effect of meteorite impact, further research may be needed. This method will have an important role in studying the past and present, and predicting the future of the Earth, and can also help us accelerate the evolution of exoplanets to make them more suitable for human beings.
2. The direct evidence for meteorite impacts primarily comes from the multi-ring structure of the crater. This study is generally aimed at extracting source parameter information from the multi-ring structure of a meteorite crater to conduct global seismic wave field simulation, without involving the dynamic formation process of the meteorite crater itself. The further development of elastoplastic wave theory in the future can better help us better understand the formation process of meteorite craters.
3. Among the numerous tectonic plate divisions, the most obvious is the boundary that exists between the American plate and the African plate. The continental drift hypothesis suggests that the two plates originally belonged to the same plate. Based on the comparison of similarities and differences between strata and rocks, it is theoretically possible to predict when the plate split in the past. For the development of the fracture mechanism, concurrent meteorite impacts may further verify this view.
Data availability statement
The original contributions presented in the study are included in the article/Supplementary Material, further inquiries can be directed to the corresponding author.
Author contributions
The author confirms being the sole contributor of this work and has approved it for publication.
Funding
This research was supported by the National Key Research and Development Program of China (No. 2021YFC3000702), and the National Natural Science Foundation of China (Grant Nos. U1901602 and 41790465).
Acknowledgments
We appreciate the invitation from the journal and Huang Fuqiong. We thank the editor and reviewers for their valuable opinions and suggestions during the revision of this article.
Conflict of interest
The author declares that the research was conducted in the absence of any commercial or financial relationships that could be construed as a potential conflict of interest.
Publisher’s note
All claims expressed in this article are solely those of the authors and do not necessarily represent those of their affiliated organizations, or those of the publisher, the editors and the reviewers. Any product that may be evaluated in this article, or claim that may be made by its manufacturer, is not guaranteed or endorsed by the publisher.
References
Ali, S. A., Nutman, A. P., Aswad, K. J., and Jones, B. G. (2019). Overview of the tectonic evolution of the Iraqi zagros thrust zone: sixty million years of neotethyan ocean subduction. J. Geodyn. 129, 162–177. doi:10.1016/j.jog.2019.03.007
Alvarez, L. W., Alvarez, W., Asaro, F., and Michel, H. V. (1980). Extraterrestrial cause for the Cretaceous/Tertiary extinction. Science 208, 1095–1108. doi:10.1126/science.208.4448.1095
Barrier, A., Bischoff, A., Nicol, A., Browne, G. H., and Bassett, K. N. (2021). Relationships between volcanism and plate tectonics: A case-study from the canterbury basin, New Zealand. Mar. Geol. 433, 106397. doi:10.1016/j.margeo.2020.106397
Bierhaus, E. B., Trang, D., Daly, R. T., Bennett, C. A., Barnouin, O. S., Walsh, K. J., et al. (2022). Crater population on asteroid (101955) Bennu indicates impact armouring and a young surface. Nat. Geosci. 15, 440–446. doi:10.1038/s41561-022-00914-5
Caglar, N., Vural, I., Kirtel, O., Saribiyik, A., and Sumer, Y. (2023). Structural damages observed in buildings after the January 24, 2020 Elazığ-Sivrice earthquake in Türkiye. Case Stud. Constr. Mater. 18, e01886. doi:10.1016/j.cscm.2023.e01886
Cañón-Tapia, E., and Walker, G. P. (2004). Global aspects of volcanism: the perspectives of “plate tectonics” and “volcanic systems”. Earth-Science Rev. 66 (1-2), 163–182. doi:10.1016/j.earscirev.2003.11.001
Collins, G. S., Patel, N., Davison, T. M., Rae, A. S. P., Morgan, J. V., Gulick, S. P. S., et al. (2020). A steeply-inclined trajectory for the Chicxulub impact. Nat. Commun. 11, 1480. doi:10.1038/s41467-020-15269-x
Conrad, C. P., and Lithgow-Bertelloni, C. (2002). How mantle slabs drive plate tectonics. Science 298 (5591), 207–209. doi:10.1126/science.1074161
Cwojdzinski, S. (2017). History of a discussion: selected aspects of the earth expansion v. plate tectonics theories. Spec. Publ. 442 (1), 93–104. doi:10.1144/sp442.24
Dewey, J. F. (1972). Plate tectonics. Sci. Am. 226 (5), 56–68. doi:10.1038/scientificamerican0572-56
Dziewonski, A. M., and Anderson, D. L. (1981). Preliminary reference Earth model. Phys. earth Planet. interiors 25, 297–356. doi:10.1016/0031-9201(81)90046-7
Gerya, T. (2014). Precambrian geodynamics: concepts and models. Gondwana Res. 25 (2), 442–463. doi:10.1016/j.gr.2012.11.008
Goderis, S., Sato, H., Ferrière, L., Schmitz, B., Burney, D., Kaskes, P., et al. (2021). Globally distributed iridium layer preserved within the Chicxulub impact structure. Sci. Adv. 7, ABE3647. doi:10.1126/sciadv.abe3647
Gulick, S., Christeson, G., Barton, P., Grieve, R., Morgan, J., and Urrutia-Fucugauchi, J. (2013). Geophysical characterization of the Chicxulub impact crater. Rev. Geophys. 51, 31–52. doi:10.1002/rog.20007
Hildebrand, A. R., Penfield, G. T., Kring, D. A., Pilkington, M., Camargo Z., A., Jacobsen, S. B., et al. (1991). Chicxulub Crater: A possible cretaceous/tertiary boundary impact crater on the yucatán Peninsula, Mexico. Mex. Geol. 19, 867–871. doi:10.1130/0091-7613(1991)019<0867:ccapct>2.3.co;2
Holder, R. M., Viete, D. R., Brown, M., and Johnson, T. E. (2019). Metamorphism and the evolution of plate tectonics. Nature 572 (7769), 378–381. doi:10.1038/s41586-019-1462-2
Holsapple, K. A., and Schmidt, R. M. (1987). Point source solutions and coupling parameters in cratering mechanics. J. Geophys. Res. Solid Earth 92 (B7), 6350–6376. doi:10.1029/jb092ib07p06350
Hull Pincelli, M., Bornemann, A., Penman, D. E., Henehan, M. J., Norris, R. D., Wilson, P. A., et al. (2020). On impact and volcanism across the Cretaceous-Paleogene boundary. Science 367, 266–272. doi:10.1126/science.aay5055
Isadorade Macedo, A. S., de Figueiredo, J. J. S., de Sousa, M. C., and Nascimento, M. J. (2020). Estimation of the seismic wavelet through homomorphic deconvolution and well log data: application on well-to-seismic tie procedure. Geophys. Prospect. 68, 1328–1340. doi:10.1111/1365-2478.12908
Johnson, T. E., Brown, M., Gardiner, N. J., Kirkland, C. L., and Smithies, R. H. (2017). Earth’s first stable continents did not form by subduction. Nature 543 (7644), 239–242. doi:10.1038/nature21383
Kagan, Y. Y. (2003). Accuracy of modern global earthquake catalogs. Phys. Earth Planet. Interiors 135 (2-3), 173–209. doi:10.1016/s0031-9201(02)00214-5
Kim, D., Banerdt, W. B., Ceylan, S., Giardini, D., Lekić, V., Lognonné, P., et al. (2022). Surface waves and crustal structure on Mars. Science 378 (6618), 417–421. doi:10.1126/science.abq7157
Kimi, K. B., and Vijayan, S. (2023). Mare filled craters on the Moon. Icarus 390, 115298. doi:10.1016/j.icarus.2022.115298
Komatitsch, J. R., and Tromp, J. (2002). The spectral-element method, Beowulf computing, and global seismology. Science 298 (5599), 1737–1742. doi:10.1126/science.1076024
Kring, D. A. (1997). Air blast produced by the Meteor Crater impact event and a reconstruction of the affected environment. Meteorit. Planet. Sci. 32, 517–530. doi:10.1111/j.1945-5100.1997.tb01297.x
Kunio, K., Oshima, N., Adachi, K., Adachi, Y., Mizukami, T., Fujibayashi, M., et al. (2016). Global climate change driven by soot at the K-Pg boundary as the cause of the mass extinction. Sci. Rep. 6, 28427. doi:10.1038/srep28427
Li, C., and Chen, X. (2021). Efficient solution of large scale matrix of acoustic wave equations in 3D frequency domain. Appl. Geophys. 18, 299–316. doi:10.1007/s11770-020-0844-4
Macdonald, K. C., Fox, P. J., Perram, L. J., Eisen, M. F., Haymon, R. M., Miller, S. P., et al. (1988). A new view of the mid-ocean ridge from the behaviour of ridge-axis discontinuities. Nature 335 (6187), 217–225. doi:10.1038/335217a0
Maouche, S., Bouhadad, Y., Harbi, A., Rouchiche, Y., Ousadou, F., and Ayadi, A. (2019). Active tectonics and seismic hazard in the tell atlas (northern Algeria): A review. Geol. Arab World-An Overv., 381–400. doi:10.1007/978-3-319-96794-3_10
Mark, B., Sholes, S., and Hwang, A. (2022). Impact craters and the observability of ancient martian shorelines[J]. Icarus 387. doi:10.1016/j.icarus.2022.115178
Maruyama, S., Santosh, M., and Azuma, S. (2018). Initiation of plate tectonics in the hadean: eclogitization triggered by the ABEL bombardment. Geosci. Front. 9 (4), 1033–1048. doi:10.1016/j.gsf.2016.11.009
Merdith, A. S., Williams, S. E., Collins, A. S., Tetley, M. G., Mulder, J. A., Blades, M. L., et al. (2021). Extending full-plate tectonic models into deep time: linking the neoproterozoic and the phanerozoic. Earth-Science Rev. 214, 103477. doi:10.1016/j.earscirev.2020.103477
Morgan Joanna, V., Bralower, T. J., Brugger, J., and Wünnemann, K. (2022). The Chicxulub impact and its environmental consequences. Nat. Rev. Earth Environ. 3, 338–354. doi:10.1038/s43017-022-00283-y
Morgan, J. V., Warner, M. R., Collins, G. S., Grieve, R. A. F., Christeson, G. L., Gulick, S. P. S., et al. (2011). Full waveform tomographic images of the peak ring at the Chicxulub impact crater. J. Geophys. Res. 116, B06303. doi:10.1029/2010jb008015
Morgan, J., Warner, M., Brittan, J., Buffler, R., Camargo, A., Trejo, A., et al. Chicxulub Working Group (1997). Size and morphology of the Chicxulub impact crater. Nat. Int. Wkly. J. Sci. 390 (6659), 472–476. doi:10.1038/37291
Nixon, C. G., Schmitt, D. R., Kofman, R., Lofi, J., Gulick, S. P., Saustrup, S., et al. (2022). Borehole seismic observations from the Chicxulub impact drilling: implications for seismic reflectivity and impact damage. Geochem. Geophys. ,Geosystems 23 (3), e2021GC009959. doi:10.1029/2021gc009959
Ouchi, S. (1985). Response of alluvial rivers to slow active tectonic movement. Geol. Soc. Am. Bull. 96 (4), 504–515. doi:10.1130/0016-7606(1985)96<504:roarts>2.0.co;2
Pilger, R. H. (1984). Cenozoic plate kinematics, subduction and magmatism: south American andes. J. Geol. Soc. 141 (5), 793–802. doi:10.1144/gsjgs.141.5.0793
Piper, J. D. A. (2013). A planetary perspective on earth evolution: lid tectonics before plate tectonics. Tectonophysics 589, 44–56. doi:10.1016/j.tecto.2012.12.042
Range Molly, M. (2022). The Chicxulub impact produced a powerful global tsunami[J]. AGU Adv. 3, e2021AV000629. doi:10.1029/2021AV000627
Riller, U., Poelchau, M. H., Rae, A. S., Schulte, F. M., Collins, G. S., Melosh, H. J., et al. (2018). Rock fluidization during peak-ring formation of large impact structures. Nature 562 (7728), 511–518. doi:10.1038/s41586-018-0607-z
Ross, C. H., Stockli, D. F., Rasmussen, C., Gulick, S. P., De Graaff, S. J., Claeys, P., et al. (2022). Evidence of Carboniferous arc magmatism preserved in the Chicxulub impact structure. GSA Bull. 134 (1-2), 241–260. doi:10.1130/b35831.1
Schulte, P., Alegret, L., Arenillas, I., Arz, J. A., Barton, P. J., Bown, P. R., et al. (2010). The Chicxulub asteroid impact and mass extinction at the Cretaceous-Paleogene boundary. Science 327, 1214–1218. doi:10.1126/science.1177265
Simutė, S., Boehm, C., Krischer, L., Gokhberg, A., Vallée, M., and Fichtner, A. (2023). Bayesian seismic source inversion with a 3-D Earth model of the Japanese Islands. J. Geophys. Res. Solid Earth 128 (1), e2022JB024231. doi:10.1029/2022jb024231
Stahler, S. C., Khan, A., Banerdt, W. B., Lognonné, P., Giardini, D., Ceylan, S., et al. (2021). Seismic detection of the martian core. Science 373, 443–448. doi:10.1126/science.abi7730
Stern, R. J., Leybourne, M. I., and Tsujimori, T. (2016). Kimberlites and the start of plate tectonics. Geology 44, 799–802. doi:10.1130/g38024.1
Stern, R. J. (2018). The evolution of plate tectonics. Philos. Trans. R. Soc. A 376, 20170406. doi:10.1098/rsta.2017.0406
Sweeney, J. F. (1978). Gravity study of great impact. J. Geophys. Res. Solid Earth 83 (B6), 2809–2815. doi:10.1029/jb083ib06p02809
Tang, Y., Liu, S., Li, X., Fan, Y., Deng, Y., Liu, Y., et al. (2020). Earthquakes spatio–temporal distribution and fractal analysis in the Eurasian seismic belt. Sci. Fis. Nat. 31, 203–209. doi:10.1007/s12210-020-00871-4
Teanby, N. A., and Wookey, J. (2011). Seismic detection of meteorite impacts on Mars. Phys. Earth Planet. Interiors 186 (1–2), 70–80. doi:10.1016/j.pepi.2011.03.004
Venkatesan, M. I., and Dahl, J. (1989). Organic geochemical evidence for global fires at the Cretaceous/Tertiary boundary. Nature 338, 57–60. doi:10.1038/338057a0
Wan, B., Yang, X., Tian, X., Yuan, H., Kirscher, U., and Mitchell, R. N. (2020). Seismological evidence for the earliest global subduction network at 2 Ga ago. Sci. Adv. 6 (32), eabc5491. doi:10.1126/sciadv.abc5491
Weller, O. M., and Stonge, M. R. (2017). Record of modern-style plate tectonics in the Palaeoproterozoic Trans-Hudson orogen. Nat. Geosci. 10 (4), 305–311. doi:10.1038/ngeo2904
Wu, C., Cui, P., and Li, Y. (2019). “Spatial distribution of coseismic landslides around a UNESCO world heritage site: a case of the 2017 jiuzhaigou earthquake,” in Geophysical research abstracts, 21.
Yang, S., Wang, Q., Liu, X., Kan, Z., Santosh, M., and Deng, J. (2022). Global spatio-temporal variations and metallogenic diversity of karst bauxites and their tectonic, paleogeographic and paleoclimatic relationship with the Tethyan realm evolution. Earth-Science Rev. 233, 104184. doi:10.1016/j.earscirev.2022.104184
Yang, Y., and Chen, X. (2022). A seismic meteor strike on Mars. Science 378 (6618), 360–361. doi:10.1126/science.add8574
Yin, A. (2012). An episodic slab-rollback model for the origin of the tharsis rise on Mars: implications for initiation of local plate subduction and final unification of a kinematically linked global plate-tectonic network on earth. Lithosphere 4 (6), 553–593. doi:10.1130/l195.1
Yin, L., Li, X., Zheng, W., Yin, Z., Song, L., Ge, L., et al. (2019). Fractal dimension analysis for seismicity spatial and temporal distribution in the circum-Pacific seismic belt. J. Earth Syst. Sci. 128, 22–27. doi:10.1007/s12040-018-1040-2
Zhang, S. (2022). When and how plate tectonics begun on earth?. Earth Sci. 47 (10), 3806–3815. doi:10.3799/dqkx.2022.817
Keywords: solid earth, seismology, seismic source, meteorite impact, plate tectonics
Citation: Li C (2023) Influence of the Yucatan earthquake event. Front. Earth Sci. 11:1201576. doi: 10.3389/feart.2023.1201576
Received: 06 April 2023; Accepted: 11 August 2023;
Published: 28 August 2023.
Edited by:
Fuqiong Huang, China Earthquake Networks Center, ChinaReviewed by:
Rosalino Fuenzalida, Arturo Prat University, ChileUnger Zoltan, Eötvös Loránd University, Hungary
Copyright © 2023 Li. This is an open-access article distributed under the terms of the Creative Commons Attribution License (CC BY). The use, distribution or reproduction in other forums is permitted, provided the original author(s) and the copyright owner(s) are credited and that the original publication in this journal is cited, in accordance with accepted academic practice. No use, distribution or reproduction is permitted which does not comply with these terms.
*Correspondence: Changcheng Li, MTAyMDE2ODY4MUBxcS5jb20=