- 1Istituto Nazionale di Geofisica e Vulcanologia, Sezione di Palermo via Ugo La Malfa, Palermo, Italy
- 2Istituto Nazionale di Geofisica e Vulcanologia, Sezione di Bologna Viale Berti Pichat, Bologna, Italy
- 3Dipartimento Scienze della Terra Università di Firenze, Firenze, Italy
- 4Instituto de Geofísica, Universidad Autónoma de México, Mexico City, Mexico
- 5Department of Earth, Atmospheric and Planetary Sciences, Massachusetts Institute of Technology Massachusetts Avenue, Cambridge, MA, United States
- 6Institute of Earth Sciences, University of Iceland, Reykjavík, Iceland
A sharp increase in volatiles, especially SO2 fluxes from the solfataric plume and diffuse CO2 from the soils of the La Fossa crater area, started in June 2021, and subsequently from the Levante Bay area, suggests renewed unrest at Vulcano Island, Italy. This event has encouraged monitoring activities and stimulated new research activities aimed at understanding the recent evolution of the volcanic system. In this study, the chemical and isotopic composition of fumaroles, thermal waters, and soil gases from the main degassing areas of Vulcano Island with a special focus on sulfur isotopes, are used to investigate the fluid transfer mechanism inside the volcano. Sulfur is one of the most abundant volatile elements present in magmas and volcanic fluids from the La Fossa crater, where it mostly occurs as SO2 and H2S at variable relative concentrations depending on oxygen fugacity and temperature. The isotope composition and the chemical ratio of sulfur species depict a complex hydrothermal-magmatic system. In addition, we utilize the installed SO2 monitoring network that measures the total outgassing of SO2 with the UV-scanning DOAS technique. The SO2 fluxes from the La Fossa crater fumaroles, coupled with the SO2/CO2 and SO2/H2O ratios, were measured to evaluate the total mass of fluids emitted by the shallow plumbing system and its relationship with the status of volcanic activity. Combining the whole chemical composition of fumaroles analyzed with a discrete, direct sampling of high-temperature fumaroles located on the crater summit, the output of discharged water vapor has been estimated (5,768 t·d−1). On the basis of the water output, we estimated the total thermal energy dissipated by the crater during the last enhanced degassing activity (167 MW). This strong and sharp increase in energy observed during the current crisis confirms the long-growing trend in terms of mass and energy recorded in recent decades, which has brought the surface system of Vulcano Island to a critical level that has never been recorded since the last eruptive event of 1888–91.
1 Introduction
The outgassing of volatiles from active volcanic systems is the only visible manifestation of volcanic activity in both eruptive and quiescent phases and represents a useful tool for assessing the level of volcanic activity. The degassing of volatiles in open conduit systems is mainly localized in the summit part of the volcanic edifices and normally represents approximately 90% of the total degassing of the volcanic system (Carapezza et al., 2011; Chiodini et al., 1998; Delgado-Granados et al., 1998; Di Martino et al., 2022; Inguaggiato et al., 2005; Inguaggiato et al., 2013; Inguaggiato et al., 2018). The two main sulfur gas species, SO2 and H2S, released from fumaroles and volcanic plumes only reveal part of the story of the trajectory volcanic gases are subjected to during their rise from the magma to the surface. It is widely accepted that the relative proportion of SO2 and H2S in volcanic gases is highly sensitive to the temperature and redox state of the magmatic-hydrothermal system the gases flush through (Giggenbach, 1987). SO2 derives from magmatic degassing at a high temperature under oxidizing conditions, whereas H2S, produced by the reduction of SO2, is released from hydrothermal systems at a lower temperature under reducing conditions.
A recent study by Henley and Fischer (2021) disputes the pioneering study by Giggenbach (1987) based on the fact that gas redox equilibria in the volcanic-hydrothermal rock realm are not only dictated by Fe minerals but also by the role of Ca minerals during gas–rock interaction processes. These authors state that the redox state of volcanic gases, expressed as RH = log(fH2/fH2O), is controlled by reactions involving a mineralogical paragenesis consisting of anorthite-pyroxene-anhydrite-sulfide. Such fluid–rock interactions buffer redox conditions controlling the SO2/H2S ratios of gases eventually released at the surface. Sulfur involved in these gas–mineral interactions will hence be partially sequestered as solid sinks, evidenced by porphyry-type ore deposits (Cu–Mo–Au) and their related alteration mineralogy.
Moreover, at “wet volcanoes,” hosting a hydrothermal system or even a crater lake, sulfur gases can be scrubbed as a solute (i.e., as sulfate, bisulfate, thiosulfates, or polythionates; Takano, 1987; Symonds et al., 2001; Delmelle and Bernard, 2015; Tamburello et al., 2015), thus creating another, this time, solute sulfur sink. Only at hyper-acidic systems (pH near 0), sulfur scrubbing can be reversed or is incomplete, manifested as gas release from a water body (Vaselli et al., 2009; Shinohara et al., 2015; Tamburello et al., 2015). It can be concluded that complex gas–water–mineral interactions inside the volcanic-hydrothermal system will create a deficit in the sulfur budget, which consequently leads to an underestimate of the total sulfur measurable in gases at fumaroles or volcanic plumes.
The role of elemental sulfur and sulfate minerals (alunite, jarosite, anhydrite, and gypsum) as sealers in volcanic-hydrothermal systems has been a key focus during the quest for precursory signals for phreatic eruptions (Takano et al., 1994; Christenson et al., 2010, Christenson et al., 2017; Montanaro et al., 2017, Montanaro et al., 2021, Montanaro et al., 2023; Rouwet et al., 2017; Rouwet, 2021; Pappaterra et al., 2022). Variations in gas chemistry often turned out to be indicative of the source and mechanism of phreatic eruptions at several volcanoes. Indeed, several temporal variations in gaseous emissions with a “more hydrothermal” (H2S-dominant) or “more magmatic” (SO2-dominant) input in sealed or unsealed systems have been provided (de Moor et al., 2016a, de Moor et al., 2016b, de Moor et al., 2019; Stix and de Moor, 2018; Battaglia et al., 2019). A full picture of the sulfur budget from the magma to the surface, as a gas, solute, or solid, is still lacking for any volcanic system on Earth, setting a target for future research.
In active volcanoes, sulfur isotopes can provide useful information to model the extent of fractionation due to magma degassing (Sakai et al., 1982; Yongfei, 1990; Mandeville et al., 1998; Marini et al., 2011; Liotta et al., 2012) and to identify mantle, crustal, and hydrothermal contributions to volcanic fluid discharges (Marini et al., 2011; Oppenheimer et al., 2011). Marini et al. (2011) clearly showed how sulfur fractionation strongly depends on the redox conditions being reduced species usually isotopically depleted. At Vulcano Island, Southern Italy, Cortecci et al. (1996) analyzed fumarole samples, elemental sulfur, hydrothermal pyrite and anhydrite, and whole rock samples for their elemental and sulfur isotope composition. The authors collected fumarole samples over the period of 1978–1995 and observed nearly constant δ34S values of +3.0‰ ±0.3‰ for total sulfur (SO2+H2S) from fumaroles at the crater rim during the period of 1979–1984. Since then, they observed depletion to approximately 0‰, followed by short-term variations from −2.7‰ to 2.2‰ vs. CDT. Based on the whole dataset, the authors suggested that during the period 1979–1983, fumarolic sulfur was related to degassing of enriched latitic-rhyolitic magma, and since then, additional sulfur sources, such as due to hydrothermal leaching of pyrite and perhaps degassing of primary basalt at depth, could have played a major role. The authors also reported δ34S values for the fumaroles from the Vulcano beach area. They measured values between −3 and 0‰ for the period of 1978–1984 and between 0 and +5‰ during the period of 1985–1995, suggesting that the beach fumarolic system could be fed by crater-type fluids modified during the interaction processes occurring in the thermal aquifer. Cortecci et al. (2001) investigated the isotope composition of SO42− in cold and thermal waters. The authors interpreted dissolved sulfate as deriving mainly from the oxidation of fumarolic SO2 in deep and shallow aquifers with a minor contribution of H2S.
Despite the high-frequency monitoring of the Vulcano fumaroles (monthly to bimonthly over the past four decades) (Carapezza et al., 1981; Capasso et al., 1997; Chiodini et al., 1998; Capasso et al., 1999; Paonita et al., 2002, Paonita et al., 2013; Paonita et al., 2002), neither SO2/H2S nor d34S is routinely reported.
The present study aims at laying another piece in the puzzle of sulfur dynamics at Vulcano Island. Furthermore, the last anomalous degassing event, which began in 2021 in the summit area of the volcano (La Fossa crater) and spread throughout the volcanic edifice, is analyzed in terms of mass and energy variations.
Vulcano Island is located in the Aeolian Archipelago (southern Italy) and is characterized by solfataric activity after the last explosive eruption activity that occurred in 1888–1891. The main shallow expression of this solfataric activity is represented by a wide and strong high-temperature fumarolic field (around 450°C) located on the summit area in the crater of La Fossa (Figure 1), and by the hydrothermal fluids degassing at a boiling temperature in the area of Levante Bay in the northern-west side of the island. Moreover, many thermal wells with temperatures up to 70°C occur in the Vulcano Village, and anomalously high soil CO2 diffuse degassing from the soil is recognized in the areas of the summit, Palizzi, and Baia Levante areas (Inguaggiato et al., 2012a) (Figure 1). After the last paroxysmal event that occurred in 1888–1891, several “geochemical crises” characterized by anomalous and sudden increases in volcanic outgassing and by strong increases in the maximum temperatures of the summit fumaroles have been observed and studied. In particular, the major geochemical crises occurred in 1978–1980 (Carapezza et al., 1981), 1988–1991 (Barberi et al., 1987; Cioni and D’Amore, 1984; Chiodini et al., 1992; Badalamenti et al., 1991), 1996 (Capasso et al., 1999), 2004–2007 (Capasso et al., 2014; Diliberto, 2017), 2009–2010 (Inguaggiato et al., 2012b; Vita et al., 2012), and 2021 (Inguaggiato et al., 2022a, Inguaggiato et al., 2022b; Vita et al., 2023; Aiuppa et al., 2022; Di Martino et al., 2022; Federico et al., 2023).
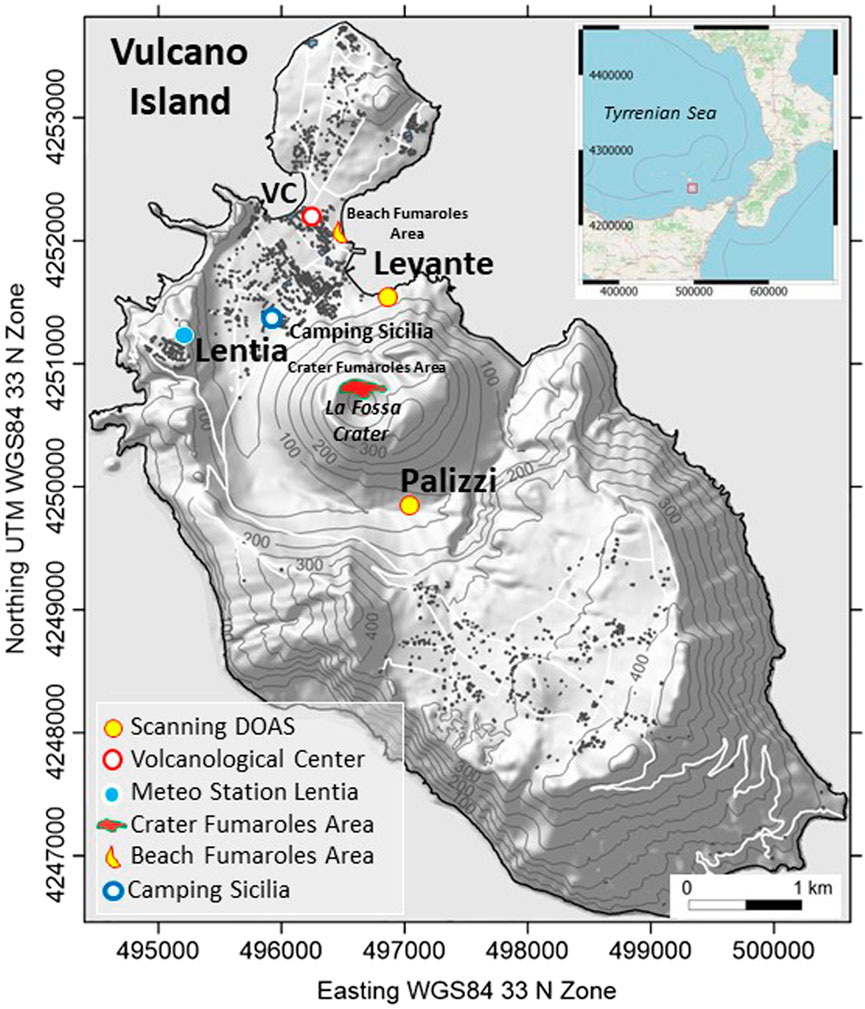
FIGURE 1. Vulcano Map: location of the SO2 UV Scanning DOAS network; the yellow circle indicates the UV scanning-DOAS stations (Palizzi and Levante); the blue circle indicates the environmental station; the white–red circle indicates the Volcanologic Center. The fumaroles of the summit and Levante Bay are indicated, respectively, by red and yellow areas. The blue–white circle indicates the thermal water of Camping Sicilia. The other two thermal water areas (Pozzo well and Vasca) are located inside the beach fumarole area.
Furthermore, these geochemical crises have highlighted increases in the gas/steam ratio (Paonita et al., 2002, Paonita et al., 2013),high-temperature fumaroles (Diliberto, 2017), significant changes toward magmatic pristine values of He and CO2 isotopic composition (Federico et al., 2023), and changes in compositional ratios (CO2/SO2 and SO2/H2S) toward more oxidizing conditions characteristic of volcanic fluids (Aiuppa et al., 2022).
Recent studies have demonstrated that phreatic eruptions—an eruption type that resulted fatally elsewhere in recent years (e.g., Ontake 2014; White Island 2019)—have occurred in Vulcano’s past (Rosi et al., 2018; Selva et al., 2020).
As such, there is a need to hypothesize how the volcanic-hydrothermal system would react from a stage of quiescence (2009) into a potentially more hazardous stage (since 2021) (Inguaggiato et al., 2022a; Aiuppa et al., 2022; Di Martino et al., 2022; Federico et al., 2023), based on variations in fumarole chemistry (SO2/H2S ratios) and in mass and energy increases (Inguaggiato et al., 2022b).
2 Materials and methods
To characterize the chemical and isotope composition of fluids, a geochemical survey to sample the main fluids discharged from Vulcano Island was carried out in 2009, including 1) three types of thermal water, 2) three high-temperature fumaroles located in the summit fumarolic area of the La Fossa crater, and 3) one boiling fumarole in the Levante Bay area (Figure 1). Moreover, other five surveys were carried out in the period of 2013–2022 to collect high-temperature fumaroles at the La Fossa crater.
Here, we present the chemical and isotopic composition (S, He, C, and N2) of the high-temperature fumaroles of the La Fossa crater and low-temperature fumaroles of the Baia di Levante hydrothermal system in the long term, from 2009 to 2021 (Supplementary Table S1). Moreover, the chemical and isotopic composition of thermal wells sampled in 2009 is reported in Supplementary Table S2.
Samples of high-temperature fumaroles located on the summit crater (Figure 1) have been collected for the determination of the whole chemical composition of emitted fluids. From 2009 to 2022, six surveys that focused on high-temperature crater fumaroles have been performed (Supplementary Tables S1, S2).
The modified Giggenbach (1975) method (Montegrossi et al., 2001), consisting of a pre-evacuated flask filled with 4 M NaOH and a flask filled with Cd(OH)2 solution, has been utilized to collect the fumarole samples.
Near-continuous flux measurements of the SO2 plume have been carried out using a network system installed in the framework of the NOVAC project, i.e., the Network for Observation of Volcanic and Atmospheric Change; Galle et al., 2010. Two UV-scanning DOAS stations were located, respectively, at NE and SW of the La Fossa cone (Figure Figure1) (Vita et al., 2012, Vita et al., 2020; Inguaggiato et al., 2022a). This configuration allowed us to track over 80% of plume emissions during the solar year.
NOVAC is a network created for the permanent monitoring of volcanic gas, which emerged in 2005 from a European project to create and install automated prototype instruments capable of monitoring different species of gases emitted by volcanic plumes around the world (Arellano et al., 2021). The remote sensing technique of passive Differential Optical Absorption Spectroscopy (DOAS) (Platt, 1994; Platt and Stulz, 2008) allows quantifying different volcanic gases within the columns emitted from active volcanoes, by collecting the spectra in the ultraviolet region (UV), in order to supply indirect measurements of magmatic volatiles (Edmonds et al., 2003; Vita et al., 2014). The DOAS method is based on the principles of absorption spectroscopy (the Bouger–Beer–Lambert law) and is used for the quantification of different gases in plumes (e.g., SO2, NO2, and BrO).
The estimation of the mass and energy output from the La Fossa crater has been carried out by coupling the extensive (SO2 fluxes) and intensive (SO2/CO2 and SO2/H2O ratios) parameters.
In particular, we utilize the SO2 monitoring networks installed on Vulcano Island that measures the total outgassing of SO2 and the chemical composition of the high-temperature fumaroles located on the volcano summit (Figure 1).
3 Results
3.1 Crater fumaroles
The crater area is characterized by a wide fumarolic field with outlet temperatures ranging from 100°C to 450°C (Figure 1B). The chemical composition of fumaroles shows variable concentrations and compositional ratios on the basis of the solfataric activity level with gas/steam and CO2/SO2 molar ratios of 4–11 and 30–67, respectively (Capasso et al., 1997; Paonita et al., 2002; Aiuppa et al., 2005; McGonigle et al., 2008; Inguaggiato et al., 2012a). The chemical composition of the fumaroles shows variable concentrations and compositional ratios with gas/steam and CO2/SO2 molar ratios of 7.3–17.23 and 14–41, respectively (Table 1). It should be noted that the chemical composition of the highest temperature fumarole showed marked changes in the years 2021–2022, with an abrupt and high increase in the gas/steam ratio starting in June 2021.
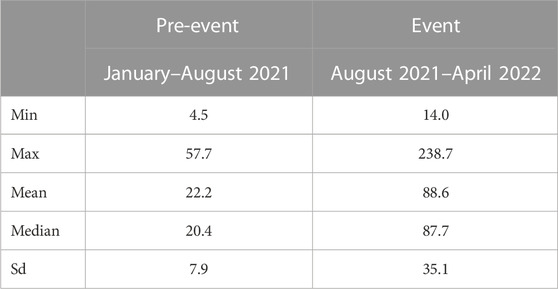
TABLE 1. Statistical data analysis of SO2 fluxes (t d−1) for the period January 2021–April 2022. The data are classified as pre-event (normal solfataric activity) from 1 January 2021 to 16 August 2021 and event (abnormal increase in outgassing) from 17 August 2021 to 28 April 2022.
The most prominent clues of magmatic fluid contribution to crater fumaroles sampled in 2009 are the isotopic compositions of helium R/Ra = 5.73 and δ13CCO2 = −1.29‰ vs. V-PDB, while the positive nitrogen isotopic composition (δ15Ncorr = 4.5‰) indicates mixing between magmatic fluids (δ15N = −5‰) with a prevalent percentage contribution of fluids of sedimentary origin (δ15N = +7‰) for this gas (Supplementary Table S2).
3.2 Fumarolic plume
The SO2 flux of the plume produced by the strong fumarolic discharge from the crater was measured using the continuous UV-scanning DOAS system positioned on the flank of the La Fossa cone (Figure 2). The SO2 fluxes showed values ranging from 4.5 to 239 t·d−1 with a mean value of 22 t·d−1 in the first period of 2021 up to August. Then, in the second period up to the end of April 2022, SO2 outgassing shows a mean value of 88 t·d−1 with a maximum of 239 t·d−1 in November 2021 (Table 1).
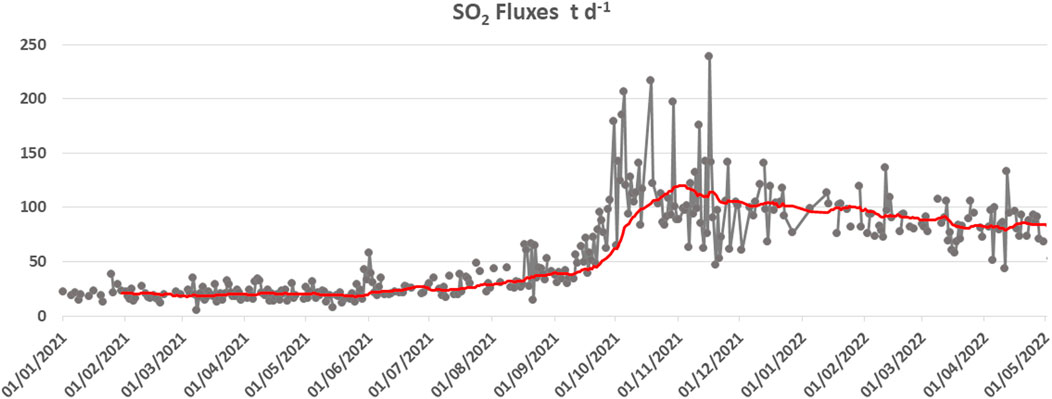
FIGURE 2. SO2 fluxes of the La Fossa crater are expressed in t d−1. A clear, strong, and abrupt increase in SO2 plume degassing has been recorded from August 2021.
3.3 Sulfur isotopes
In this study, we dedicated special attention to investigating the processes regulating the isotopic composition of sulfur from fumarolic gases, sublimate elemental sulfur, and thermal water. Analytical results, where the isotope ratios were expressed in the δ34S notation relative to Canyon Diablo Troilite (CDT), are given in Supplementary Table S3 and plotted in Figure 3.
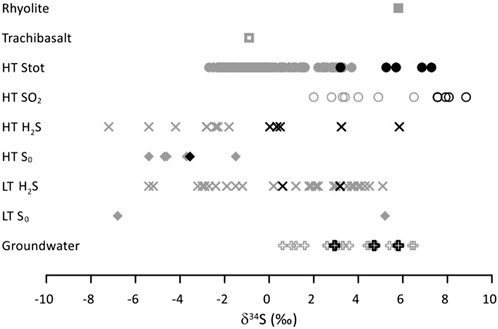
FIGURE 3. Isotope composition of sulfur at Volcano Island. The gray symbols are data from Cortecci et al. (1996) and Cortecci et al. (2001). The black symbols are this study’s data. HT, high temperature fumaroles; LT, low temperature fumaroles. Data relative to total sulfur in HT fumaroles are computed based on the isotope composition of H2S and SO2 (see text for details).
The whole δ34S dataset covers a wide range of values, from −3.6‰ vs. CDT, measured for elemental sulfur up to 8.8‰ vs. CDT, for SO2 from high-temperature fumaroles. With respect to the data on Cortecci et al. (1996) and Cortecci et al. (2001), our results relative to H2S and SO2 are enriched in 34S. On the contrary, data relative to elemental sulfur from high-temperature fumaroles and to H2S from low-temperature fumaroles (Levante Bay), as well as total sulfur dissolved in thermal water, fall in the ranges previously defined by Cortecci et al. (1996) and Cortecci et al. (2001).
3.4 Mass and energy output
Extensive parameters (mass and energy outputs), such as the fluxes of CO2, H2O, and SO2, and heat released in volcanic areas are paramount in providing information about the extent of the ongoing volcanic phenomena and the amount of the involved magma (Harris et al., 2012; Harris and Maciejewski, 2000; Inguaggiato et al., 2012a; Inguaggiato et al., 2018; Harris, 2009; Meza Maldonado et al., 2017). Moreover, coupling the intensive and extensive parameters yields basic information that is useful when formulating models of volcanic fluid degassing (Inguaggiato et al., 2011; Inguaggiato et al., 2012b; Inguaggiato et al., 2013; Inguaggiato et al., 2018).
3.4.1 Crater area mass release
The estimation of the mass flux emitted from the solfataric area was carried out according to the indirect method proposed by Inguaggiato et al. (2011) and Inguaggiato et al. (2012a), which is based on the plume SO2 flux and the SO2/CO2 and SO2/H2O ratios, applying the following equation:
ΦniFarea is the ni flux of a single volatile evaluated for the crater plume; ΦSO2Plume is the SO2 flux of the plume; and (ni/SO2)fumarole is the weight ratio of each volatile in the fumarole composition.
A wide range of CO2 and H2O fluxes have been estimated in the 2021–2022 period (Table 2) with increases of, respectively, 5 and 6 times with respect to the values of 2007 (Inguaggiato et al., 2012a).
We estimated an increase in plume CO2 fluxes from 362 t·d−1 (September 2021; Inguaggiato et al., 2012a) to 2,209 t·d−1 (February 2022). A similar increase was observed in the discharged water vapor from 1,330 t·d−1 to 5,768 t·d−1 in February 2022 (Table 2).
The soil summit CO2 diffuse output, from the no-fumarolized areas, estimated with degassing surveys reflects the deep input indicated by the increasing plume flux, showing a maximum value of 248 t·d−1 measured in September 2021 (Inguaggiato et al., 2022b).
Eventually, based on the output of estimated discharged water (5,768 t·d−1) and the only latent heat of water evaporation (2,500 kJ/kg), we estimated an energy value, dissipated by the crater solfataric area, ranging from 3.33 × 109 kJ·d−1 (Inguaggiato et al., 2012a) to 1.44 × 1010 kJ·d−1 (Table 3), corresponding to 167 MW.
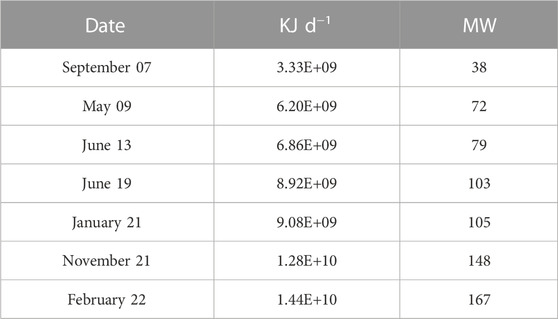
TABLE 3. Energy output (KJ d−1) of the La Fossa crater fumarole field expressed in MW. The data for 2007 are from Inguaggiato et al. (2012b).
3.4.2 Levante Bay area mass release
The peripheral area of Levante Bay is characterized by solfataric emissions and diffuses soil CO2 degassing onshore and offshore. The soil CO2 output in the onshore area showed a strong increase in an order of magnitude (like that observed in the summit area) ranging from 1.2 t·d−1 in May 2011 to 16.4 t·d−1 in May 2022 (Inguaggiato et al., 2022b). The visible effects of this strong increase in degassing were seen in the sea stretch of Levante Bay, in front of the mud pool, which turned white due to the acidification of the seawater from pH = 8.2 to around pH = 5.3 and the presence of sulfurous material flocculating on the sea (Figure 4B).
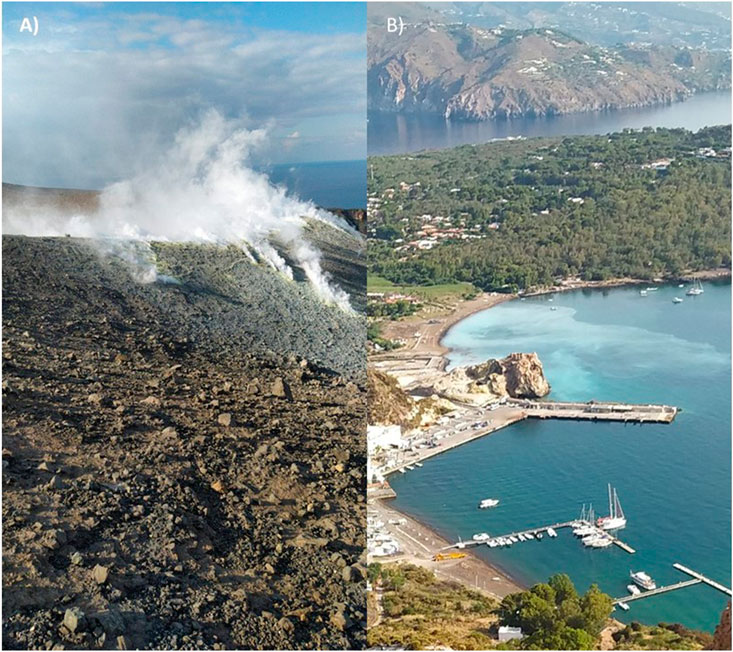
FIGURE 4. Pictures of the summit and Levante Bay areas. (A) Picture of La Fossa crater solfataric emissions (October 2021) highlighting the strong degassing fumaroles with an extension of the fumarolic field over the crater rim; (B) picture of the Levante Bay area (May 2021) where the white color of the sea can be observed due to the presence of suspended sulfurous material.
4 Discussion
4.1 Sulfur isotopes
In order to compare our results with those previously reported in the literature, we computed the isotopic composition of total sulfur from high-temperature fumaroles based on the isotope ratios of H2S and SO2 and their relative fractions (H2S/SO2 ratios). The calculated d34S values of the total sulfur ranging from 3.6‰ to 8.8‰ are plotted in Figure 3 and cover the d34S value of local rhyolite of 6.0‰. The isotopic composition of SO2, H2S, and total sulfur differs from the data by Cortecci et al. (1996) and Cortecci et al. (2001), with our data more enriched in 34S. Since temporal variation was also reported by Cortecci et al. (1996), our data could reflect such variability. Cortecci et al. (1996) explained data recorded in fumaroles from June 1980 to July 1984 (δ34S values of +3.0‰ ± 0.3‰) discussing several hypotheses and concluded that the major source of sulfur in crater fumaroles prior to 1984 was supplied by degassing of a latitic-rhyolitic melt. They also explained subsequent temporal variations assuming stream-driven leaching of secondary pyrite in the hydrothermal zone. However, the isotopic fractionation of sulfur species strongly depends on redox conditions and temperature. Although the attempt of retrieving information on the deep sulfur source is a desirable perspective, we cannot neglect that temporal variations in temperature and redox conditions in the Vulcano fumaroles are well-documented (Paonita et al., 2013; Federico et al., 2023). In light of this, the temporal variations observed by Cortecci et al. (1996), as well as our enriched values, could be the result of a hydrothermal-magmatic system much more complex with respect to that depicted by Cortecci et al. (1996). Even if our computed total sulfur isotope composition from high-temperature fumaroles fits the rhyolite isotope composition, we have to take into account that the compositions of emitted fluids depend on different relative contributions of magma degassing and hydrothermal fluids (Di Liberto et al., 2002). Concerning the groundwater, we highlight that our results fit those previously reported. This could reflect almost steady-state conditions of the system where the sulfur input of deep magmatic gases, the contribution of hydrothermal fluids, and the occurrence of interaction processes between fluids and mineral phases do not produce large variations over time. Taking into account that the water in the hydrothermal system exhibits a high content of sulfur, possible changes due to some input of magmatic gases to this shallow system could be masked.
4.2 La Fossa crater CO2 fumarole output
We compared Vulcano Island’s “new” 2021–2022 increased CO2 summit fluxes with other volcanic systems of Italy (Figure 5). In particular, we observed that Vulcano Island in 2007 showed CO2 degassing fluxes in the same order as other quiescent volcanoes, like Pantelleria and Ischia (around 1,000 t·d−1), and the open conduit Stromboli volcano (500–700 t·d−1). The CO2 outgassing values recorded during the 2021–2022 crisis show a sharp increase (2,200 t·d−1) and classify Vulcano as the second highest CO2 emitter of Italian volcanoes, only after Etna (approximately 40,000 t·d−1).
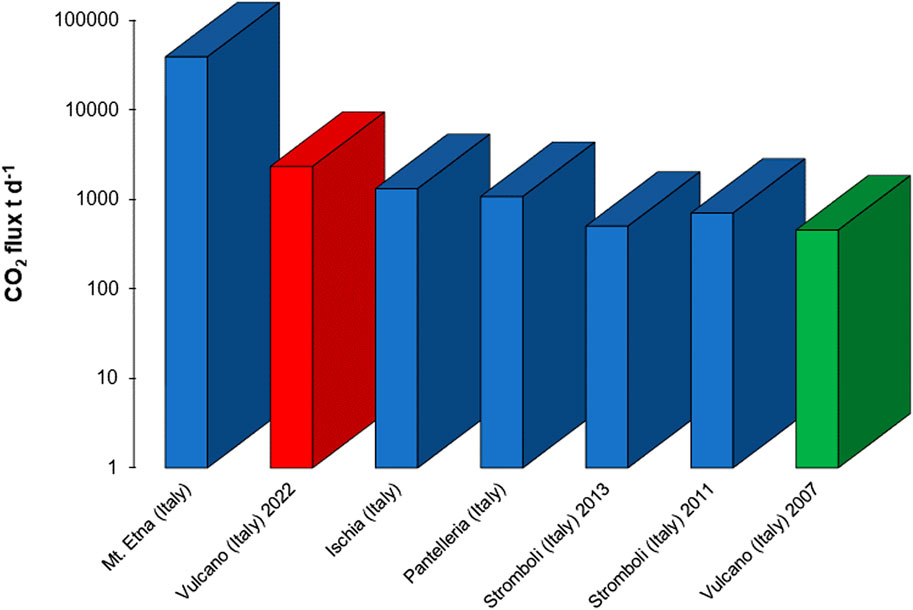
FIGURE 5. Total CO2 output discharged from Vulcano Island in volcanic unrest in 2022 (red bar), compared to Vulcano Island in volcanic quiescence in 2007 (green bar), and to other Italian volcanic systems.
Previous studies (Favara et al., 2001; Pecoraino et al., 2005) have shown that the proportion of degassing from the summit crater (S) and that from the peripheral (P) area (R=S/P) at Ischia and Pantelleria is close to 0.1, reflecting degassing styles in closed-conduit systems with solfataric degassing activity, such as Ischia and Pantelleria (Favara et al., 2001; Pecoraino et al., 2005). On the other hand, open-conduit volcanic degassing systems, such as Stromboli and Etna (D’Alessandro et al., 1997; Inguaggiato et al., 2005, Inguaggiato et al., 2011, Inguaggiato et al., 2013), show the opposite behavior with the CO2 summit degassing with an order of magnitude higher than peripheral degassing (R = 10) (Figure 6).
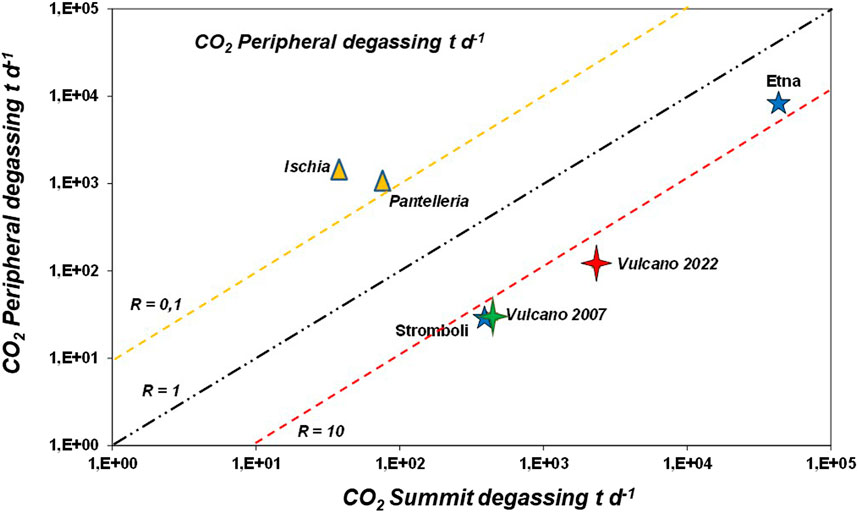
FIGURE 6. Summit vs. peripheral CO2 degassing of Vulcano Island in 2022 (red star) compared with Vulcano Island in 2007 (green big star), and other closed- (yellow triangles) and open-conduit (blue star) volcanic systems in Italy. The dashed lines (R) represent the proportion of degassing from the summit (S) and peripheral (P) areas, R=S/P.
Although Vulcano is a closed-conduit system with solfataric activity, it has shown similar behavior to that of open-conduit systems, being the CO2 solfataric summit degassing with an order of magnitude higher than peripheral degassing (Inguaggiato et al., 2012a, Inguaggiato et al., 2013). Moreover, as shown in Figure 5, the total outgassing increased in 2022 (red star) by about an order of magnitude with respect to 2007 (green bar) but continued to maintain the same outgassing ratios between the summit and the peripheral systems (Figure 6). This indicates that the deep degassing input is affecting the entire volcanic edifice.
The sharp and significant recorded increase in the harmful volatile fluxes, from the soil and fumarole degassing toward the atmosphere, observed during this ongoing geochemical crisis suggests continuing monitoring and investigating for better quantification of the hazard posed by SO2 and other volcanic gas species (i.e., CO2 and H2S).
4.3 La Fossa crater H2O fumarole mass and energy output historical dataset
In order to investigate the long-time evolution of the discharged water vapor from the solfataric area of the La Fossa crater of Vulcano, we recompile the historical dataset of H2O steam measured by several authors from 1983 to 2007 (Figure 7).
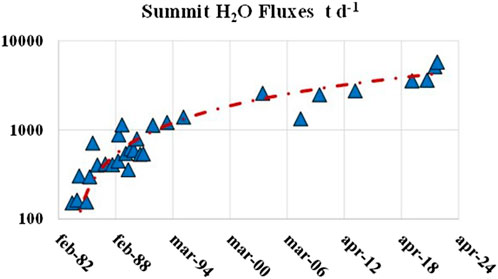
FIGURE 7. Summit water vapor degassing at the La Fossa crater of Vulcano Island from 1983 to February 2022 expressed in t d−1. The data on water fluxes from 1983 to 1995 are from Italiano and Nuccio (1992) and Italiano et al. (1998); for September 2003, from Aiuppa et al. (2005); and for September 2007, from Inguaggiato et al. (2012a). The post-2007 data are from this study.
We can observe that the H2O fluxes, extracted from the scientific literature, range from 157 t·d−1 (February 1983; Italiano and Nuccio, 1992) to 1,330 t·d−1 (September 2007; Inguaggiato et al., 2012a), with a continuous long trend increases of water fluxes. Then, the last new dataset of this work, from September 2009 to February 2022, shows a further continuous long-term increase in water flux up to 3,570 t·d−1 in June 2019. Finally, in 2021–2022, a further abrupt increase in the water vapor flux was estimated, with values up to 5,768 t·d−1 in February 2022 (Figure 7).
The total geothermal potential estimated at Vulcano (179 MW) has to be compared with other geothermal systems, as shown in Figure 8. As we see, the Tacaná hydrothermal system, Mexico–Guatemala, shows a geothermal potential of approximately 98 MW (Collard et al., 2014; Rouwet et al., 2015); El Chichón, Mexico, near 171 MW (Taran and Peiffer, 2009); the Flegrean Fields, Italy, approximately 100 MW (Chiodini et al., 2010); Puracè, Colombia, approximately 360 MW; and Irazú-Turrialba, Costa Rica, near 110 MW (Rouwet et al., 2021), while for Vulcano it is approximately 50 MW (Inguaggiato et al., 2012a). Domuyo volcano, Argentina, shows a geothermal potential of 1,000 MW (Chiodini et al., 2014), whereas the Yellowstone hydrothermal system shows a geothermal potential of 5,300–6,100 MW (Fournier, 1989; Ingebritsen et al., 2001), which are both about one to two orders of magnitude higher than the thermal output of Vulcano Island. Considering the surface extent of each volcanic system, the theoretical lines of heat flux expressed in MW km−2 have been added. Flegrean Fields, El Chichón, and Puracé, approximately 1, 10, and 30 km2, respectively, show the highest heat flux per unit of area (20–100 MW·km−2), the system of Yellowstone (approximately 2,500 km2), and Tacanà (∼25 km2), have lower heat fluxes per unit area (about 2 and 3.9 MW·km−2). The heat flux of Vulcano (about 20 km2) in 2022 approximates 10 MW·km−2.
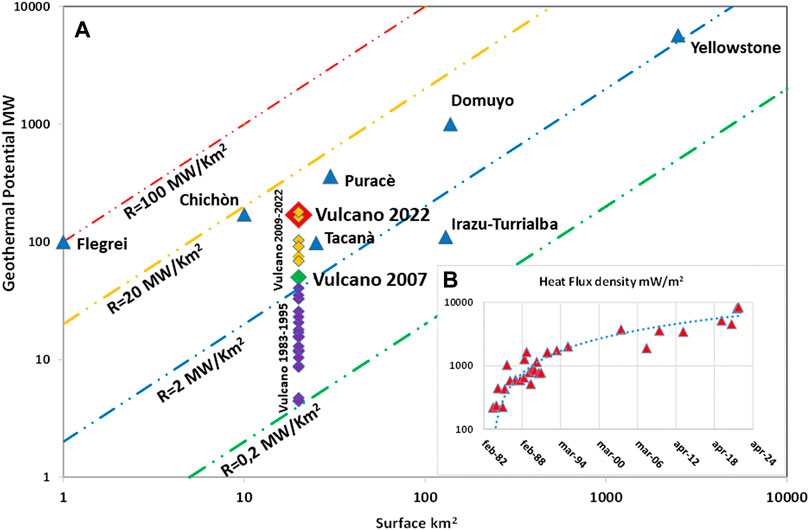
FIGURE 8. (A) Geothermal potential estimated at Vulcano Island in 2022 (169 MW) compared with other geothermal systems of the world and Vulcano Island in 2007. (B) Heat flux density (R= MW km−2) of Vulcano Island has been calculated from 1983 to 2022, showing that the heat flux at Vulcano increases the heat flux density from 220 to 8,450 mW m−2 from 1983 to 1995 (purples rhombus), 2007 (green rhombus), and 2009 to 2013 (yellow rhombus).
To follow the evolution of energy output discharged from Vulcano Island, the estimated energy in MW·m−2 from 1983 to 2022 is plotted in Figure 8B, where the continuous increases in heat fluxes are evident from 220 MW·m−2 in 1983 to 8,450 MW·m−2 in 2022.
The increased outgassing observed in the last few years marks a new degassing crisis, suggesting that Vulcano Island has entered a renewed stage of volcanic unrest. The large increase in volatile mass and energy output of the Vulcano system, both from the summit and in peripheral areas, indicates pressurization of the entire volcanic edifice, taking the degassing activity to a critical level that has never been reached in the last few decades. The high volatile pressure and energy in the shallow plumbing systems can lead to new energetic volcanic events in the future (e.g., phreatic eruptions that could culminate into magmatic eruptions). This new strong degassing framework deserves great attention from the scientific community and from the volcanic observatories to monitor any further variations in the degassing activity.
5 Conclusion
The isotope composition of sulfur from fumarolic gases, sublimate elemental sulfur, and thermal water reflects a complex hydrothermal-magmatic system where the pristine magmatic signature of the emitted magmatic gases is modified by post-magmatic processes occurring in the hydrothermal system.
The latest ongoing geochemical crisis at Vulcano Island, which began in 2021, has shown an increase of over an order of magnitude in the degassing of SO2 from the solfataric plume and from the soils of both the summit and the peripheral anomalous areas, respectively, the La Fossa crater and Levante Bay and Palizzi areas.
Furthermore, a strong increase in the energy dissipated by the volcanic system estimated only from the summit fumarolic area was recorded. The heat flux reached values that were never recorded in the past, reaching up to 8,450 mW/m2 with a total energy of 167 MW.
This degassing increase observed in 2021 does not represent an isolated case in the last four decades but is part of a broader evolution of the degassing activity of the volcanic system, representing a further input that since 1978 to date (seven strong inputs documented) has characterized and confirmed the continuous growth of the degassing activity of the island of Vulcano.
It is, therefore, necessary to continue monitoring the volcano’s outgassing activity to catch any signal of a further input that could irreversibly alter the delicate mass balance between the deep input and the surface output discharged toward the atmosphere, which could trigger volcanic activity.
The sharp increase in the degassing of volatile flows has also led to an environmental implication by producing an increase in the average concentrations of gaseous species harmful to human health in the atmosphere, such as SO2 and H2S (Diliberto. 2017; Vita et al., 2023).
The control of air quality, therefore, deserves attention from the official institutions responsible for the protection of public health.
Data availability statement
The original contributions presented in the study are included in the article/Supplementary Material. Further inquiries can be directed to the corresponding author.
Author contributions
Conceptualization: SI, FT, SK, and FV; methodology: SI, FV, ML, SK, BS, and FT; validation: SI, FV, BS, and SO; investigation: SI, DR, and ML; data curation: FV, SI, FT, BS, and SO; writing—original draft preparation: SI, ML, FT, and DR; and writing—review and editing: SI, DR, ML, FT, and SO. All authors contributed to the article and approved the submitted version.
Funding
This research was funded by the TORS project in the framework of institutional INGV projects “Ricerca Libera” ObFu 9999.549; and Pianeta Dinamico Task V2, ObFu 1020.010.
Acknowledgments
The chemical analysis data on fumarole samples were produced in the geochemical laboratories of Dipartimento Scienze della Terra, University of Firenze, Italy, while the isotopic data on helium, nitrogen, and carbon were produced in the geochemical laboratory of INGV Palermo. Finally, the isotopic analysis of sulfur was performed in the geochemical laboratory of MIT Boston, United States.
Conflict of interest
The authors declare that the research was conducted in the absence of any commercial or financial relationships that could be construed as a potential conflict of interest.
Publisher’s note
All claims expressed in this article are solely those of the authors and do not necessarily represent those of their affiliated organizations, or those of the publisher, the editors, and the reviewers. Any product that may be evaluated in this article, or claim that may be made by its manufacturer, is not guaranteed or endorsed by the publisher.
Supplementary material
The Supplementary Material for this article can be found online at: https://www.frontiersin.org/articles/10.3389/feart.2023.1197796/full#supplementary-material
SUPPLEMENTARY TABLE S1 | Average chemical composition of the La Fossa crater fumaroles sampled from 2009 to 2022 surveys. The data are expressed in μM.
SUPPLEMENTARY TABLE S2 | Isotopic composition of the summit and peripheral fumaroles. 3He/4He ratios expressed as Ra, with Ra the 3He/4He ratio in air being 1.40 x 10−6. Isotopic values are expressed in δ‰.
SUPPLEMENTARY TABLE S3 | Isotopic composition of SO2 and H2S in the fumaroles (2009 survey), waters, and native S.
References
Aiuppa, A., Bitetto, M., Calabrese, S., Delle Donne, D., Lages, J., La Monica, F. P., et al. (2022). Volcanic gas SO2 flux data from La Fossa volcano and Volcanic gas compositions of La Fossa volcano fumaroles (Vulcano island, Italy), Version 1.0. Interdiscip. Earth Data Alliance (IEDA). doi:10.26022/IEDA/112522
Aiuppa, A., Inguaggiato, S., McGonigle, A. J. S., O'Dwyer, M., Oppenheimer, C., Padgett, M. J., et al. (2005). H2S fluxes from Mt. Etna, Stromboli, and Vulcano (Italy) and implications for the sulfur budget at volcanoes. Geoch.et Cosmoch.Acta 69, 1861–1871. doi:10.1016/j.gca.2004.09.018
Arellano, S., Galle, B., Apaza, F., Avard, G., Barrington, C., Bobrowski, N., et al. (2021). Synoptic analysis of a decade of daily measurements of SO2 emission in the troposphere from volcanoes of the global ground-based Network for Observation of Volcanic and Atmospheric Change. Earth Syst. Sci. Data 13 (13), 1167–1188. doi:10.5194/essd-13-1167-2021
Badalamenti, B., Chiodini, G., Cioni, R., Favara, R., Francofonte, S., Gurrieri, S., et al. (1991). Special field workshop at Vulcano (Aeolian Islands) during summer 1988. Geochemical results. Acta Vulcanol. 1, 223–227.
Barberi, F., Neri, G., Valenza, M., and Villari, L. (1991). 1987–1990 unrest at Vulcano. Acta Vulcanol. 1, 95–106.
Battaglia, A., de Moor, M. J., Aiuppa, A., Avard, G., Bakkar, H., Bitetto, M., et al. (2019). Insights into the mechanisms of phreatic eruptions from continuous high frequency volcanic gas monitoring: Rincon de la Vieja Volcano, Costa Rica. Front. Earth Sci. 6. doi:10.3389/feart.2018.00247
Capasso, G., Favara, R., Francofonte, S., and Inguaggiato, S. (1999). Chemical and isotopic variations in fumarolic discharge and thermal waters at vulcano island (aeolian islands, Italy) during 1996: Evidence of resumed volcanic activity. J. Volcanol. Geotherm. Res. 88, 167–175. doi:10.1016/s0377-0273(98)00111-5
Capasso, G., Favara, R., and Inguaggiato, S. (1997). Chemical features and isotopic composition of gaseous manifestations on vulcano island (aeolian islands, Italy): An interpretative model of fluid circulation. Geoch.et Cosmoch.Acta 61, 3425–3440. doi:10.1016/s0016-7037(97)00163-4
Capasso, G., Federico, C., Madonia, P., and Paonita, A. (2014). Response of the shallow aquifer of the volcano-hydrothermal system during the recent crises at Vulcano Island (Aeolian Archipelago, Italy). J. Volcanol. Geotherm. Res. 273, 70–80. doi:10.1016/j.jvolgeores.2014.01.005
Carapezza, M. L., Barberi, F., Rinaldi, M., Ricci, T., Tarchini, L., Barrancos, J., et al. (2011). Diffuse CO2 soil degassing and CO2 and H2S concentrations in air and related hazards at Vulcano Island (Aeolian arc Italy). J. Volcanol. Geotherm. Res. 207 (3–4), 130–144. doi:10.1016/j.jvolgeores.2011.06.010
Carapezza, M., Nuccio, P. M., and Valenza, M. (1981). Genesis and evolution of the fumaroles of vulcano (aeolian islands, Italy): A geochemical model. Bull. Volcanol. 44, 547–563. doi:10.1007/bf02600585
Chiodini, G., Caliro, S., Cardellini, C., Granieri, D., Avino, R., Baldini, A., et al. (2010). Long-term variations of the Campi Flegrei, Italy, volcanic system as revealed by the monitoring of hydrothermal activity. J. Geophys Res. 115, B03205. doi:10.1029/2008JB006258
Chiodini, G., Cioni, R., Falsaperla, S., Guidi, M., Marini, L., and Montalto, A. (1992). Geochemical and seismological investigations at vulcano (aeolian islands) during 1978–1989. J. Geophys. Res. 97, 11025–11032. doi:10.1029/92jb00518
Chiodini, G., Cioni, R., Guidi, M., Marini, L., and Raco, B. (1998). Soil CO2 flux measurements in volcanic and geothermal areas. Appl. Geochem. 13 (5), 543–552. doi:10.1016/S0883-2927(97)00076-0
Chiodini, G., Liccioli, C., Vaselli, O., Calabrese, S., Tassi, F., Caliro, S., et al. (2014). The Domuyo volcanic system: An enormous geothermal resource in Argentine Patagonia. J. Volcanol. Geotherm. Res. 274, 71–77. doi:10.1016/j.jvolgeores.2014.02.006
Christenson, B. W., Reyes, A. G., Young, R., Moebis, A., Sherburn, S., Cole-Baker, J., et al. (2010). Cyclic processes and factors leading to phreatic eruption events: Insights from the 25 september 2007 eruption through ruapehu crater lake, New Zealand. J. Volcanol. Geotherm. Res. 191, 15–32. doi:10.1016/j.jvolgeores.2010.01.008
Christenson, B. W., White, S., Britten, K., and Scott, B. J. (2017). Hydrological evolution and chemical structure of a hyper-acidic spring-lake system on Whakaari/White Island, NZ. NZ. J. Volcanol. Geotherm. Res. 346, 180–211. doi:10.1016/j.jvolgeores.2017.06.017
Cioni, R., and D’Amore, F. (1984). A genetic model for the crater fumaroles of Vulcano island (Sicily, Italy). Geothermics 13, 375–384. doi:10.1016/0375-6505(84)90051-8
Collard, N., Taran, Y., Peiffer, L., Campion, R., and Paz, M. P. J. (2014). Solute fluxes and geothermal potential of Tacaná volcano-hydrothermal system, Mexico–Guatemala. J. Volcanol. Geotherm. Res. 288, 123–131. doi:10.1016/j.jvolgeores.2014.10.012
Cortecci, G., Dinelli, E., Bolognesi, L., Boschetti, T., and Ferrara, G. (2001). Chemical and isotopic compositions of water and dissolved sulfate from shallow wells on Vulcano Island, Aeolian Archipelago, Italy. Aeolian Archipel. Italy. Geothermics 30, 69–91. doi:10.1016/S0375-6505(00)00037-7
Cortecci, G., Ferrara, G., and Dinelli, E. (1996). Isotopic time-variations and variety of sources for sulfur in fumaroles at Vulcano island, Aeolian archipelago, Italy. Acta Vulcanol. 8 (2), 147–160.
D’Alessandro, W., Giammanco, S., Parello, F., and Valenza, M. (1997). CO2 output and δ13C(CO2) from Mount Etna as indicators of degassing of shallow asthenosphere. Bull. Volcanol. 58, 455–458. doi:10.1007/s004450050154
de Moor, J. M., Aiuppa, A., Pacheco, J., Avard, G., Kern, C., Liuzzo, M., et al. (2016b). Short-period volcanic gas precursors to phreatic eruptions: Insights from poás volcano, Costa Rica. Earth Planet Sci. Lett. 442, 218–227. doi:10.1016/j.epsl.2016.02.056
de Moor, J. M., Stix, J., Avard, G., Muller, C., Corrales, E., Diaz, J. A., et al. (2019). Insights on hydrothermal-magmatic interactions and eruptive processes at Poa´s volcano (Costa Rica) from high-frequency gas monitoring and drone measurements. Geophys. Res. Lett. 46, 1293–1302. doi:10.1029/2018GL080301
de Moor., J. M., Aiuppa, A., Avard, G., Wehrmann, H., Dunbar, N., Muller, C., et al. (2016a). Turmoil at turrialba volcano (Costa Rica): Degassing and eruptive processes inferred from high-frequency gas monitoring: Turmoil at turrialba volcano. J. Geophys Res. Solid Earth 121, 5761–5775. doi:10.1002/2016JB013150
Delgado-Granados, H., Piedad Sanchez, N., Galvan, L., Julio, P., Alvarez, J. M., and Cardenas, L. (1998). CO2 flux measurements at popocatepetl volcano: II. Magnitude of emissions and significance (abstract). EOS Trans. AGU 79 (45), 926.
Delmelle, P., and Bernard, A. (2015). “The remarkable chemistry of sulfur in hyper-acid crater lakes: A scientific tribute to bokuichiro Takano and minoru kusakabe,” in Book chapter in volcanic lakes. Editors D. Rouwet, B. Christenson, F. Tassi, and J. Vandemeulebrouck (Heidelberg, Germany: Springer), 239–260. doi:10.1007/978-3-642-36833-2_10
Di Liberto, V., Nuccio, P. M., and Paonita, A. (2002). Genesis of chlorine and sulfur in fumarolic emissions at vulcano island Italy): Assessment of pH and redox conditions in the hydrothermal system. J. Volcanol. Geotherm. Res. 116. doi:10.1016/S0377-0273(02)00215-9
Di Martino, R. M. R., Gurrieri, S., Camarda, M., Capasso, G., and Prano, V. (2022). Hazardous changes in soil CO2 emissions at Vulcano, Italy, in 2021. J. Geophys. Res. Solid Earth 127 (11), e2022JB024516. doi:10.1029/2022JB024516
Diliberto, I. S. (2017). Long-term monitoring on a closed-conduit volcano: A 25 year long time-series of temperatures recorded at La Fossa cone (vulcano island, Italy), ranging from 250 °C to 520 °C. J. Volcanol. Geotherm. Res. 346, 151–160. doi:10.1016/j.jvolgeores.2017.03.005
Edmonds, M., Herd, H., Galle, B., and Oppenheimer, C. (2003). Automated, high time-resolution measurements of SO2 flux at Soufri-re Hills Volcano, Montserrat. Volcanol 65, 578–586. doi:10.1007/s00445-003-0286-x
Favara, R., Giammanco, S., Inguaggiato, S., and Pecoraino, G. (2001). Preliminary estimate of CO2 output from Pantelleria Island volcano (Sicily, Italy): Evidence of active mantle degassing. Appl. Geochem. 16, 883–894. doi:10.1016/S0883-2927(00)00055-X
Federico, C., Cocina, O., Gambino, S., Paonita, A., Branca, S., Coltelli, M., et al. (2023). Inferences on the 2021 ongoing volcanic unrest at vulcano island (Italy) through a comprehensive multidisciplinary surveillance network. Remote Sens. 15 (5), 1405. doi:10.3390/rs15051405
Fournier, R. O. (1989). Geochemistry and dynamics of the Yellowstone national park hydrothermal system. Annu. Rev. Earth Planet Sci. 17, 13–53. doi:10.1146/annurev.ea.17.050189.000305
Galle, B., Johansson, M., Rivera, C., Zhang, Y., Kihlman, M., Kern, C., et al. (2010). Network for observation of volcanic and atmospheric change (NOVAC): A global network for volcanic gas monitoring-network layout and instrument description. J. Geophys. Res. 115, D05304. doi:10.1029/2009JD011823
Giggenbach, W. F. (1975). A simple method for the collection and analysis of volcanic gas samples. Bull. Volcanol. 39, 132–145. doi:10.1007/bf02596953
Giggenbach, W. F. (1987). Redox processes governing the chemistry of fumarolic gas discharges from White Island, New Zealand. Appl. Geochem 2, 143–161. doi:10.1016/0883-2927(87)90030-8
Harris, A., Alparone, S., Bonforte, A., Dehn, J., Gambino, S., Lodato, L., et al. (2012). Vent temperature trends at the vulcano Fossa fumarole field: The role of permeability. Bull. Volcanol. 74, 1293–1311. doi:10.1007/s00445-012-0593-1
Harris, , Lodato, L., Dehn, J., and Spampinato, L. (2009). Thermal characterization of the Vulcano fumarole field. Bull. Volcanol. 71, 441–458. doi:10.1007/s00445-008-0236-8
Harris, , and Maciejewski, (2000). Thermal surveys of the vulcano Fossa fumarole field 1994–1999: Evidence for fumarole migration and sealing. J. Volcanol. Geotherm. Res. 102, 119–147. doi:10.1016/S0377-0273(00)00184-0
Henley, R. W., and Fischer, T. P. (2021). Sulfur sequestration and redox equilibria in volcanic gases. J. Volcanol. Geotherm. Res. 414, 107181. doi:10.1016/j.jvolgeores.2021.107181
Ingebritsen, S. E., Galloway, D. L., Colvard, E. M., Sorey, M. L., and Mariner, R. H. (2001). Time variation of hydrothermal discharge at selected sites in the Western United States: Implications for monitoring. J. Volcanol. Geotherm. Res. 111, 1–23. doi:10.1016/s0377-0273(01)00207-4
Inguaggiato, S., Vita, F., Rouwet, D., Bobrowski, N., Morici, S., and Sollami, A. (2011). Geochemical evidence of the renewal of volcanic activity inferred from CO2 soil and SO2 plume fluxes: The 2007. Stromboli Erupt. (Italy) Bullettin Volcanol. 73 (4), 443–456. doi:10.1007/s00445-010-0442-z
Inguaggiato, S., Calderone, L., Inguaggiato, C., Mazot, A., Morici, S., and Vita, F. (2012b). Long-time variation of soil CO2 fluxes at the summit of Vulcano Island (Italy). Bull. Volcanol. 74, 1859–1863. doi:10.1007/s00445-012-0637-6
Inguaggiato, S., Diliberto, I. S., Cinzia, Federico, Paonita, Antonio, and Vita, Fabio (2018). Review of the evolution of geochemical monitoring, networks and methodologies applied to the volcanoes of the. Aeolian Arc (Italy) Earth-Science Rev. 176, 241–276. doi:10.1016/j.earscirev.2017.09.006
Inguaggiato, S., Jacome Paz, M. P., Mazot, A., Delgado Granados, H., Inguaggiato, C., and Vita, F. (2013). CO2 output discharged from Stromboli Island (Italy). Chem. Geol. 339, 52–60. doi:10.1016/j.chemgeo.2012.10.008
Inguaggiato, S., Martin-Del Pozzo, A. L., Aguayo, A., Capasso, G., and Favara, R. (2005). Isotopic, chemical and dissolved gas constraints on spring water from popocatepetl volcano (Mexico): Evidence of gas–water interaction between magmatic component and shallow fluids. J. Volcanol. Geoth. Res. 141, 91–108. doi:10.1016/j.jvolgeores.2004.09.006
Inguaggiato, S., Mazot, A., Diliberto, I. S., Inguaggiato, C., Madonia, P., Rouwet, D., et al. (2012a). Total CO2 output from vulcano island (aeolian islands, Italy) geochemistry. Geophys. Geosystems 13, Q02012. doi:10.1029/2011GC003920
Inguaggiato, S., Vita, F., Diliberto, I. S., Inguaggiato, C., Mazot, A., Cangemi, M., et al. (2022b). The volcanic activity changes occurred in the 2021–2022 at Vulcano island (Italy), inferred by the abrupt variations of soil CO2 output. Sci. Rep. 12, 21166. doi:10.1038/s41598-022-25435-4
Inguaggiato, S., Vita, F., Diliberto, I. S., Mazot, A., Calderone, L., Mastrolia, A., et al. (2022a). The extensive parameters as a tool to monitoring the volcanic activity: The case study of Vulcano Island (Italy). Remote Sens. 14 (5), 1283. doi:10.3390/rs14051283
Italiano, F., and Nuccio, P. M. (1992). Volcanic steam output directly measured in fumaroles: The observed variations at vulcano island, Italy, between 1983 and 1987. Bull. Volcanol. 54, 623–630. doi:10.1007/BF00430775
Italiano, F., Pecoraino, G., and Nuccio, P. M. (1998). Steam output from fumaroles of an active volcano: Tectonic and magmatic-hydrothermal controls on the degassing system at Vulcano (Aeolian arc). J. Geophys. Res. Solid Earth 103 (B12), 29829–29842. doi:10.1029/98JB02237
Liotta, M., Rizzo, A., Paonita, A., Caracausi, A., and Martelli, M. (2012). Sulfur isotopic compositions of fumarolic and plume gases at Mount Etna (Italy) and inferences on their magmatic source. Geochem. Geophys. Geosystems 13, Q05015. doi:10.1029/2012GC004118
Mandeville, C. W., Sasaki, A., Saito, G., Faure, K., King, R., and Hauri, E. (1998). Open-system degassing of sulfur from Krakatau 1883 magma. Earth Planet. Sci. Lett. 160, 709–722. doi:10.1016/S0012-821X(98)00122-8
Marini, L., Moretti, R., and Accornero, M. (2011). Sulfur isotopes in magmatic-hydrothermal systems, melts, and magmas. Rev. Mineral. Geochem. 73, 423–492. doi:10.2138/RMG.2011.73.14
McGonigle, A. J. S., Aiuppa, A., Giudice, G., Tamburello, G., Hodson, A. J., and Gurrieri, S. (2008). Unmanned aerial vehicle measurements of volcanic carbon dioxide fluxes. Geophys. Res. Lett. 35, L06303. doi:10.1029/2007GL032508
Meza Maldonado, L. F., Inguaggiato, S., Tulio Jaramillo, M., Garzón Valencia, G., and Mazot, A. (2017). Volatiles and energy released by Puracé volcano. Bull. Volcanol. 79 (12), 84. doi:10.1007/s00445-017-1168-y
Montanaro, C., Mayer, K., Isaia, R., Gresse, M., Scheu, B., Ylmaz, T. I., et al. (2017). Hydrothermal activity and subsoil complexity: Implication for degassing processes at Solfatara crater, Campi Flegrei caldera. Bull. Volcanol. 79, 83. doi:10.1007/s00445-017-1167-z
Montanaro, C., Mortensen, K. A., Weisenberger, T. B., Dingwell, D. B., and Scheu, B. (2021). Stratigraphic reconstruction of the víti breccia at krafla volcano (Iceland): Insights into pre-eruptive conditions priming explosive eruptions in geothermal areas. Bull. Volcanol. 83, 81–27. doi:10.1007/s00445-021-01502-y
Montanaro, C., Ray, L., Cronin, S. J., Calibugan, A., Rott, S., Bardsley, C., et al. (2023). Linking top and subsoil types, alteration and degassing processes at Rotokawa geothermal field, New Zealand. Front. Earth Sci. 10, 1067012. doi:10.3389/feart.2022.1067012
Montegrossi, G., Tassi, F., Vaselli, O., Buccianti, A., and Garofalo, K. (2001). Sulfur species in volcanic gases. Anal. Chem. 73, 3709–3715. doi:10.1021/ac001429b
Oppenheimer, C., Scaillet, B., and Martin, R. S. (2011). Sulfur degassing from volcanoes: Source conditions, surveillance, plume chemistry and Earth system impacts. Rev. Mineralogy Geochem. 73 (1), 363–421. doi:10.2138/rmg.2011.73.13
Paonita, A., Favara, R., Nuccio, P. M., and Sortino, F. (2002). Genesis of fumarolic emissions as inferred by isotope mass balances: CO2 and water at vulcano island, Italy. Geochim. Cosmochim. Acta 66, 759–772. doi:10.1016/S0016-7037(01)00814-6
Paonita, A., Federico, C., Bonfanti, P., Capasso, G., Inguaggiato, S., Italiano, F., et al. (2013). The episodic and abrupt geochemical changes at La Fossa fumaroles (Vulcano Island, Italy) and related constraints on the dynamics, structure, and compositions of the magmatic system. Geochimica Cosmochimica Acta 120, 158–178. doi:10.1016/J.GCA.2013.06.015
Pappaterra, S., Inguaggiato, C., Rouwet, D., Mora-Amador, R., Ramírez-Umaña, C., González, G., et al. (2022). Rare earth elements variations in a hyperacid crater lake and their relations with changes in phreatic activity, physico-chemical parameters, and chemical composition: The case of poás volcano (Costa Rica). Front. Earth Sci. 9, 716970. doi:10.3389/feart.2021.716970
Pecoraino, G., Brusca, L., D’Alessandro, W., Giammanco, S., Inguaggiato, S., and Longo, M. (2005). Total CO2 output from Ischia island volcano (Italy). Geochem. J. 39, 451–458. doi:10.2343/geochemj.39.451
Platt, U. (1994). “Differential optical absorption spectroscopy (DOAS),” in Air monitoring by spectroscopic techniques, chemical analysis series; sigrist, M.W., Editor M. W. Sigrist (Hoboken, New Jersey, United States: John Wiley and Sons Inc.), 27–83. doi:10.5194/essd-13-1167-2021
Platt, U., and Stutz, J. (2008). Differential optical absorption spectroscopy principles and applications, Physics of Earth and Space environments. Berlin, Germany: Springer-Verlag, 597. doi:10.1007/978-3-540-75776-4
Rosi, M., Di Traglia, F., Pistolesi, M., Esposti Ongaro, T., de’Michieli Vitturi, M., and Bonadonna, C. (2018). Dynamics of shallow hydrothermal eruptions: New insights from Vulcano’s breccia di Commenda eruption. Bull. Volcanol. 80, 83–28. doi:10.1007/s00445-018-1252-y
Rouwet, D., Mora-Amador, R., Ramírez, C., González, G., Baldoni, E., Pecoraino, G., et al. (2021). Response of a hydrothermal system to escalating phreatic unrest: The case of turrialba and Irazú in Costa Rica (2007-2012). Earth, Planets Space 73, 142. doi:10.1186/s40623-021-01471-8
Rouwet, D., Mora-Amador, R., Ramírez, C. J., González, G., and Inguaggiato, S. (2017). “Dynamic fluid recycling at laguna caliente (poás, Costa Rica) before and during the 2006—Ongoing phreatic eruption cycle (2005–10),” in Geochemistry and Geophysics of active volcanic lakes. Editors T. Ohba, B. Capaccioni, and C. Caudron (Burlington House, Piccadilly, London: Geol Soc London, Special Publication), 73–96. doi:10.1144/sp437.11
Rouwet, D., and Morrissey, M. M. (2015). “Mechanisms of Crater lake breaching eruptions,” in Volcanic lakes. Advances in Volcanology. Editors D. Rouwet, B. Christenson, F. Tassi, and J. Vandemeulebrouck (Berlin, Germany: Springer). doi:10.1007/978-3-642-36833-2_3
Rouwet, D., Taran, Y., and Inguaggiato, S. (2015). “Fluid geochemistry of Tacaná volcano-hydrothermal system,” in Springer-IAVCEI series, active volcanoes of the world, active volcanoes of chiapas (Mexico): El Chichón and Tacaná. Editors T. Scolamacchia, and J. L. Macías (Berlin, Germany: Springer). doi:10.1007/978-3-642-25890-9_7
Sakai, H., Casadevall, T. J., and Moore, J. G. (1982). Chemistry and isotope ratios of sulfur in basalts and volcanic gases at Kilauea volcano, Hawaii. Geochim. Cosmochim. Acta 46, 729–738. doi:10.1016/0016-7037(82)90024-2
Selva, J., Bonadonna, C., Branca, S., De Astis, G., Gambino, S., Paonita, A., et al. (2020). Multiple hazards and paths to eruptions: A review of the volcanic system of vulcano (aeolian islands, Italy). Earth Sci. Rev. 207, 103186. doi:10.1016/j.earscirev.2020.103186
Shinohara, H., Yoshikawa, S., and Miyabuchi, Y. (2015). Degassing activity of a volcanic crater lake: Volcanic plume measurements at the yudamari crater lake, aso volcano, Japan. Berlin, Germany: Springer. doi:10.1007/978-3-642-36833-2_8
Stix, J., and de Moor, J. M. (2018). Understanding and forecasting phreatic eruptions driven by magmatic degassing. Earth Planets Space 70, 83. doi:10.1186/s40623-018-0855-z
Symonds, R. B., Gerlach, T. M., and Reed, M. H. (2001). Magmatic gas scrubbing: Implications for volcano monitoring. J. Volcanol. Geotherm. Res. 108, 303–341. doi:10.1016/S0377-0273(00)00292-4
Takano, B., Saitoh, H., and Takano, E. (1994). Geochemical implications of subaqueous molten sulfur at Yugama crater lake, Kusatsu-Shirane volcano, Japan. Geochem J. 28, 199–216. doi:10.2343/geochemj.28.199
Takano, B. (1987). Correlation of volcanic activity with sulfur oxy-anion speciation in acrater lake. Science 235, 1633–1635. doi:10.1126/science.235.4796.1633
Tamburello, G., Agusto, M., Caselli, A., Tassi, F., Vaselli, O., Calabrese, S., et al. (2015). Intense magmatic degassing through the lake of Copahue volcano, 2013–2014. J. Geophys Res. 120, 6071–6084. doi:10.1002/2015JB012160
Taran, Y., and Peiffer, L. (2009). Hydrology, hydrochemistry and geothermal potential of El Chichón volcano hydrothermal system, Mexico. Geothermics 38, 370–378. doi:10.1016/j.geothermics.2009.09.002
Vaselli, O., Tassi, F., Duarte, E., Fernndez, E., Poreda, R. J., and Delgado Huertas, A. (2009). Evolution of fluid geochemistry at the Turrialba volcano (Costa Rica) from 1998 to 2008. Bull. Volcanol. 72, 397–410. doi:10.1007/s00445-009-0332-4
Vita, F., Arellano, S., Inguaggiato, S., and Galle, B. (2020). SO2 flux of -VULCANO- volcano from the NOVAC database. NOVAC Database. doi:10.17196/novac.vulcano.001
Vita, F., Inguaggiato, S., Bobrowski, N., Calderone, L., Galle, B., and Parello, F. (2012). Continuous SO2 flux measurements for vulcano island, Italy. Ann. Geophys. 55 (2), 301–308. doi:10.4401/ag-5759
Vita, F., Kern, C., and Inguaggiato, S. (2014). Development of a portable active long-path differential optical absorption spectroscopy system for volcanic gas measurements. J. Sens. Sens. Syst. 3, 355–367. doi:10.5194/jsss-3-355-2014
Vita, F., Schiavo, B., Inguaggiato, C., Inguaggiato, S., and Mazot, A. (2023). Environmental and volcanic implications of volatile output in the atmosphere of vulcano island detected using SO2 plume (2021–23). Remote Sens. 15 (12), 3086. doi:10.3390/rs15123086
Keywords: sulfur isotopes, Vulcano Island, SO2 plume, solfataric activity, mass and energy output
Citation: Inguaggiato S, Liotta M, Rouwet D, Tassi F, Vita F, Schiavo B, Ono S and Keller NS (2023) Sulfur origin and flux variations in fumarolic fluids of Vulcano Island, Italy. Front. Earth Sci. 11:1197796. doi: 10.3389/feart.2023.1197796
Received: 31 March 2023; Accepted: 11 July 2023;
Published: 02 August 2023.
Edited by:
Roberto Sulpizio, University of Bari Aldo Moro, ItalyReviewed by:
Silvia Massaro, Università degli Studi Aldo Moro, ItalyKaroly Nemeth, Institute of Earth Physics and Space Sciences, Hungary
Copyright © 2023 Inguaggiato, Liotta, Rouwet, Tassi, Vita, Schiavo, Ono and Keller. This is an open-access article distributed under the terms of the Creative Commons Attribution License (CC BY). The use, distribution or reproduction in other forums is permitted, provided the original author(s) and the copyright owner(s) are credited and that the original publication in this journal is cited, in accordance with accepted academic practice. No use, distribution or reproduction is permitted which does not comply with these terms.
*Correspondence: Salvatore Inguaggiato, c2FsdmF0b3JlLmluZ3VhZ2dpYXRvQGluZ3YuaXQ=