- 1Department of Forest Ecology and Management, Swedish University of Agricultural Sciences, Umeå, Sweden
- 2Unit for Field-based Forest Research, Swedish University of Agricultural Sciences, Umeå, Sweden
- 3Department of Ecology and Environmental Science, Umeå University, Umeå, Sweden
- 4Department of Environmental Systems Science D-USYS, ETH Zurich, Zurich, Switzerland
- 5Department of Aquatic Sciences and Assessment, Swedish University of Agricultural Sciences, Uppsala, Sweden
Boreal peatlands represent a biogeochemically unique and diverse environment in high-latitude landscape. They represent a long-term globally significant sink for carbon dioxide and a source of methane, hence playing an important role in regulating the global climate. There is an increasing interest in deciphering peatland biogeochemical processes to improve our understanding of how anthropogenic and climate change effects regulate the peatland biogeochemistry and greenhouse gas balances. At present, most studies investigating land-atmosphere exchanges of peatland ecosystems are commonly based on single-tower setups, which require the assumption of homogeneous conditions during upscaling to the landscape. However, the spatial organization of peatland complexes might feature large heterogeneity due to its varying underlying topography and vegetation composition. Little is known about how well single site studies represent the spatial variations of biogeochemical processes across entire peatland complexes. The recently established Kulbäcksliden Research Infrastructure (KRI) includes five peatland study sites located less than 3 km apart, thus providing a unique opportunity to explore the spatial variation in ecosystem-scale processes across a typical boreal peatland complex. All KRI sites are equipped with eddy covariance flux towers combined with installations for detailed monitoring of biotic and abiotic variables, as well as catchment-scale hydrology and hydrochemistry. Here, we review studies that were conducted in the Kulbäcksliden area and provide a description of the site characteristics as well as the instrumentation available at the KRI. We highlight the value of long-term infrastructures with ecosystem-scale and replicated experimental sites to advance our understanding of peatland biogeochemistry, hydrology, ecology, and its feedbacks on the environment and climate system.
1 Introduction
Boreal peatlands represent a biogeochemically unique and diverse environment providing a long-term globally significant carbon (C) sink and an important methane (CH4) source (Frolking and Roulet, 2007). Specifically, they are the most C dense terrestrial ecosystem in the world (Joosten and Couwenberg, 2008; Dunn and Freeman, 2014). Covering less than 3% of land area (Limpens et al., 2008; Frolking et al., 2011), boreal peatlands store C in excess of all the fossil fuels burnt since 1870 (Le Quéré et al., 2018; Ratcliffe et al., 2020). Peatlands are characterized by a high water table which strongly regulates their ecology and biogeochemistry (Mulqueen, 1986), including the production and consumption of greenhouse gases (GHG) such as carbon dioxide (CO2) and CH4 (Moore and Dalva, 1993; Shen et al., 2022). The current C and GHG balances of peatlands are however susceptible to ongoing climate change and our knowledge on their responses to these perturbations remains limited.
Peatlands are natural examples of complex adaptive systems (Rydin and Jeglum, 2013). Peatland types show both a latitudinal (zonal) and longitudinal (azonal) differentiation (Ruuhijärvi, 1960; Rydin et al., 1999; Masing et al., 2010) indicating that they respond to thermic and hygric changes in the climate. However, the relative importance of the various components combined with the role of local topographical conditions has demonstrated that responses to climate or nutrient forcing may be elusive (Belyea and Baird, 2006). Peatlands have spatially variable feedbacks which contribute to their iconic ‘surface patterning’ but importantly also results in a spatial heterogeneity of response to climate and nutrients changes (Eppinga et al., 2007), potentially confounding up-scaling of studies performed at only plot-scales. The response of peatlands to external forcing can also be slow, potentially over the course of centuries (Swindles et al., 2012), as seen in peatlands which have undergone spontaneous recovery after mining or drainage many centuries earlier (Swindles et al., 2016). The considerable complexity of the peatland response to external perturbations necessitates both a long-term and spatially inclusive approach to research.
One common feature of most peatland research settings is that they are focused either on the small-scale variation between different microforms, i.e., hummocks, lawns and hollows (Bubier et al., 1993; Granberg et al., 1997; Korrensalo et al., 2018), or on large-scale variations between clearly distinct peatland systems (Turetsky et al., 2014; Abdalla et al., 2016; Peltola et al., 2019). However, northern peatlands often occur as an ensemble of patches which form heterogeneous peatland complexes, with distinct and yet hydrologically connected units (Pakarinen, 1995; Laitinen et al., 2007). These complexes represent an intermediate spatial scale which is almost completely overlooked in current peatland research. Instead, peatlands are often implicitly studied as homogeneous entities, i.e., assuming that a small area is representative of the entire peatland complex. Specifically, micrometeorological studies of land-atmosphere exchanges (e.g., from eddy covariance, or EC, towers) are often limited to a single site assuming that the footprint of a single flux tower represents large areas surrounding the tower, with little or no validation (Desai et al., 2022). However, upscaling of flux measurements should be done with footprint awareness, i.e., considering the local conditions such as vegetation type and microtopography, that affect the representativeness of EC measurements at the surrounding areas (Chu et al., 2021). Across an entire peatland complex, local conditions on vegetation type, microtopography and hydrology are prone to variation and thus can be expected to affect biogeochemical processes.
At present, only few studies have explored the effects from varying local conditions across peatland complexes. For instance, research in the Siikaneva mire complex in Finland revealed that its fen and bog sections had similar net ecosystem exchange (NEE), but the fen section exhibited higher gross primary production (GPP) and ecosystem respiration (Reco), as well as higher CH4 emissions compared to the bog section (Alekseychik et al., 2021). A study in a freshwater wetland in California United States combining a stationary and a roving EC tower to compare CH4 fluxes at three sites within the same wetland system, found substantial heterogeneity in CH4 fluxes due to spatial variations in air temperature (Ta), friction velocity, and vegetation composition (Matthes et al., 2014). The spatial variability of CH4 emissions in an agricultural peatland was also observed through the use of EC towers installed at different heights in the Netherlands, which integrated different local conditions as the footprint increased from short towers (6 m height) to taller towers (20 and 60 m height) (Peltola et al., 2015). Different non-coordinated EC towers are in operation in the sub-arctic Stordalen Palsa bog in Abisko, Sweden, with more focus on C fluxes in link with permafrost thawing (Jammet et al., 2017; Łakomiec et al., 2021). Other efforts exist, but mostly limited to short-term infrastructures and other land uses, such as the CHEESEHEAD19 cluster of 20 EC towers investigating the variability of a forested landscape in the upper Midwest United States during 2019 (Desai et al., 2022).
To our knowledge, no coordinated research infrastructures with the aim of investigating ecosystem-scale processes at multiple sites within the same peatland complex in the boreal biome exist. Despite the need for establishing replication, the complex nature and limited accessibility of peatlands encourages the concentration of research within relatively small, dedicated areas, resulting in confined peatland research hubs around the globe. One of these peatland research hubs with long-term records is Degerö Stormyr, Northern Sweden (64° 10′ 55.3″ N, 19° 33′ 23.5″ E) where research on peatland biogeochemistry has been carried out since 1909, with intense contemporary research since 1995 including ongoing EC measurements since 2001. Building on this experimental site, the Kulbäcksliden Research Infrastructure (KRI) was established, including today five peatland study sites located less than 3 km apart within the same peatland complex, to facilitate replicated studies of ecosystem-scale processes. Here, we first present a review of the research history at Degerö Stormyr starting in the early 1900s followed by a comprehensive description of the site characteristics and instrumentation at KRI. We further emphasize the relevance of such replicated ecosystem-scale infrastructures for a better understanding of the biogeochemistry of peatland systems and how they interact with the environment and climate.
2 Review of the infrastructure research history
The research history at Kulbäcksliden is more than a century old, starting in the beginning of the 19th century with the overall aim to provide science-based knowledge on how to manage forests in Sweden. At that time, forest products were one of the fundaments of the Swedish economy. One of the believed major threats to forest growth was paludification, i.e., vertical expansion of mires with the peat mosses transforming soils to conditions that are unsuitable for tree growth. To investigate if this assumption had any scientific support, a study on general mire hydroecology was started in 1909 at Degerö Stormyr, Kulbäcksliden. This study resulted in one of the very first doctoral thesis on general mire ecology and concluded that paludification has halted and that the peat-forming vegetation was mostly connected to sites with shallow groundwater during most of the year (Malmström, 1923). In 1923, Kulbäcksliden was incorporated as part of the Vindeln Experimental Forests, where research has continued until today (Grip, 2015; Laudon et al., 2021).
Most of the historic research was carried out at Degerö Stormyr, the primary hub of the present KRI. After a temporary halt in research activities following these initial investigations during the early 20th century, active research resumed in the late 1990s and has steadily grown until today. A keyword search (“Degerö”, “Degerö Stormyr”, “Kulbäcksliden”, “Krycklan+C18”) both on the Web of Knowledge and Google Scholar (on 2 February 2022) generated a database of 142 peer-reviewed articles based on research at Degerö Stormyr either as a single-site study, regional projects or within global synthesis studies. These research articles can be broadly classified into six research topics: peat stratigraphy; mercury; hydromorphology and microclimate; stream C export; global change (mainly temperature, nitrogen, N, and sulphur, S) impact on vegetation development, peat stoichiometry and microbiology; greenhouse gases and energy exchange (Figure 1). A list of all papers included in this review as well as their categorization can be found at https://slughg.github.io/Kulbacksliden-Publications.
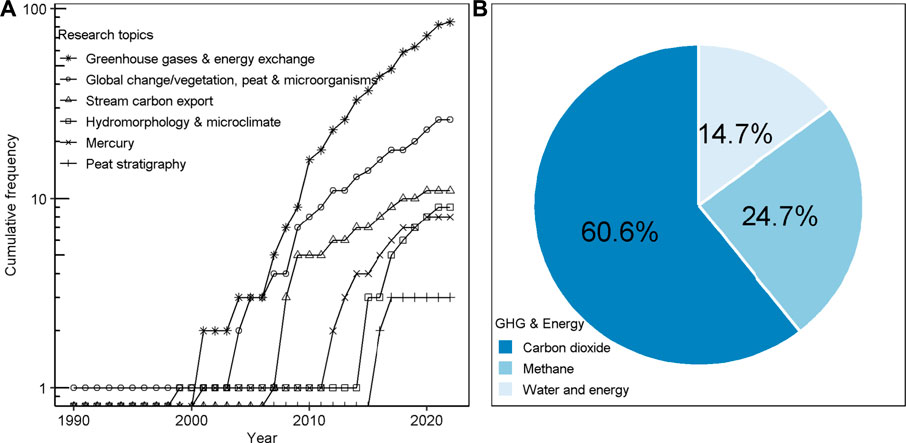
FIGURE 1. Research topics covered at Degerö Stormyr from 1990 to present (A) Cumulative frequencies per research topic, note the logarithmic Y-axis; and (B) relative share in the research topic “greenhouse gases and energy exchange”.
Since 2001, research in the area is dominated by studies of the C and greenhouse gas balances (Figure 1A). The number of investigations of the impact of global change on vegetation development, peat stoichiometry and microorganisms has been growing as well, while peat stratigraphy has, to date, been limited to three studies. Within the topic “greenhouse gases and energy exchange”, CO2 exchange was the most studied gas species (61%), followed by CH4 emissions (25%) (Figure 1B).
2.1 Peat stratigraphy
An early description of peat stratigraphy at Degerö Stormyr was made through multiple cores from a 1,200 m long transect in the direction North-South (Malmström, 1923; Kulczyński, 1949) which were dated using the spruce pollen horizon. However, it was only in 2009 that a high-resolution peat composition and age were investigated with the collection and analysis of a 314 cm long peat core for elemental composition and 14C-dating (Larsson et al., 2017). It was found that organic matter started accumulating prior to 8,300 calendar years BP (i.e. 8300 years before 1950 AD), which was in agreement with the earlier conclusions of Malmström (1923) and Kulczyński (1949). The long-term accumulation rates for C and N were 13.7 ± 5.5 and 0.28 ± 0.14 g m-2 yr-1, respectively. 13C content increased with depth and the content in 15N was higher at lower C:N ratios, likely due to preferential utilization of lighter isotope compounds during decomposition (12C) and plant nutrient uptake (14N). It was also apparent that changes in vegetation species composition over time were reflected in the peat C and N content and had a major impact on the degree of organic matter decomposition, thereby also affecting peat growth and decay (Larsson et al., 2017).
2.2 Mercury
Peatlands are considered as hotspots of methylmercury (MeHg) production due to their low redox status and high C content (St. Louis et al., 1996; Bishop and Lee, 1997; Hu et al., 2020). Highly toxic MeHg can be transported to lakes that are hydrologically connected to peatlands (Bergman et al., 2012) and bioaccumulate in its foodwebs (Åkerblom et al., 2014). Given that sulphate-reducing bacteria are one of the primary methylators of reactive mercury (HgII), investigations of the effect of long-term S deposition on the methylation of HgII revealed a linear relationship between MeHg content in pore water and sulphate additions (Branfireun et al., 2001; Åkerblom et al., 2020). However, the effect of increased S loading on MeHg net production was counteracted by increased temperature, most likely due to increased loss of S at higher temperature (Åkerblom et al., 2013). The availability of HgII in peat that potentially transforms to MeHg also depends on the input of atmospherically deposited Hg by rain (HgII) and vegetation uptake (Hg0) as well as the output of Hg via re-emission (Hg0) (Bishop et al., 2020). Full-year land-atmosphere emission of Hg0 from Degerö Stormyr was 2.4 times greater than inputs by rain and 7.2 times greater than output with runoff (Osterwalder et al., 2017). This finding indicates that Hg0 re-emissions from peatlands prolongs the cycling of anthropogenic Hg in the environment, but will decrease Hg contamination of downstream aquatic ecosystems locally. In another study, dynamic flux chambers measurements helped investigate the effect of S and N deposition as well as greenhouse treatments on the net Hg0 flux (Fritsche et al., 2014). The data revealed that net Hg0 re-emission was significantly reduced by long-term sulphate additions. These recent investigations on Hg cycling at Degerö Stormyr have important implications for future human consumption of freshwater fish in the region.
2.3 Hydromorphology and microclimate
When research started at Degerö Stormyr in order to investigate the possible paludification of northern Sweden, it consisted of soil sampling for groundwater particle flow (tracer experiment), porosity, hydraulic conductivity, water permeability in undisturbed soil cores, mechanical and chemical analysis. In addition, dissolved compounds (including oxygen) were analysed on groundwater samples (Malmström, 1923). During the last two decades, hydromorphology studies mainly investigated topics such as hydraulic conductivity (Nijp et al., 2017a), peat volume change in relation to microtopography (Nijp et al., 2017b; 2019), and N cycling from mires to surface waters (Sponseller et al., 2018). Efforts have been made also towards modelling (Nijp et al., 2017b), gapfilling and upscaling meteorological variables like water table level (WTL) (Granberg et al., 1999) using remote sensing (Bechtold et al., 2019; Burdun et al., 2020) but also soil temperature (Ts) (Granberg et al., 1999; Vuichard and Papale, 2015; Lembrechts et al., 2021).
2.4 Stream carbon export
Part of the large C stock in mires (Yu, 2012) is continuously transported in different aquatic forms (e.g., dissolved organic or inorganic C) by streams to nearby lakes and other freshwaters. The extent of this phenomenon and its controls have been investigated at Degerö Stormyr and its main draining stream (Ågren et al., 2008; Berggren et al., 2009; Giesler et al., 2009; Campeau et al., 2017), suggesting total C exports in the range from 6 to 18 g C m-2 yr-1 with a 12 years (2003–2014) average of 12.2 g C m-2 yr-1 (Leach et al., 2016). Stream and freshwater C support for bacterial growth has also been studied (Berggren et al., 2007; 2009).
2.5 Global change impacts on vegetation development, peat stoichiometry and microorganisms
Response data from plot scale N and S amendments and temperature treatments (Granberg et al., 2001) have been used in several studies which were unanimous on the substantial change in growth, production and competition of plants in response to changes in environmental conditions. One such change is the progressive replacement of the moss carpet by vascular plants with increased N deposition and warming (Gunnarsson et al., 2004; Wiedermann et al., 2007; 2009b; 2009a; Limpens et al., 2011; 2012; Wiedermann and Nilsson, 2020). In the long-term, C input and accumulation was increased by N addition, reduced by S addition, and significant interactions were found between the different factors, i.e., N, S and temperature (Nilsson and Eriksson, 2011; Olid et al., 2014).
The water regime of different mires was found to regulate nutrient allocation, with more nutrients being available in hollows than in hummocks in discharge-dominated mires, compared to evapotranspiration-dominated mires (Eppinga et al., 2010). Increasing Ta and N and S deposition was found to alter the peat geochemistry with an increased retention of Ca, Fe, P, and Zn in the surface peat (Olid et al., 2017).
Microclimate and microtopography control plant (Wiedermann and Nilsson, 2020) and microbial community composition (Økland, 1990; Nilsson et al., 2011; Robroek et al., 2014). Consequently, changes in the local conditions could induce substantial changes in the abundance and activity of microorganisms as observed for the altered activity of methanogens with increased temperature, N and S (Martí et al., 2015; 2019) or the dominance of fungal or bacterial activity depending on the management regime of peatlands (Groß-Schmölders et al., 2020). Increased vascular plant disease occurrence due to parasitic fungi has been observed with increased N deposition which may have important implications for CO2 and CH4 exchange between mires and the atmosphere (Wiedermann et al., 2007).
2.6 Greenhouse gas and energy land-atmosphere exchanges
The long-term EC measurements at Degerö Stormyr since 2001 show that the peatland is an annual CO2 sink with a 12-year mean of 58 ± 21 g C m-2 yr-1 (Peichl et al., 2014). However, during the unusual drought year of 2018, the mire acted as a net C source (15.2 g C m-2 yr-1) for the first time in the measurement record (Rinne et al., 2020). Accounting also for C fluxes via CH4, total organic C in precipitation and stream C export to estimate the net ecosystem C balance (NECB), suggested a net C sink of 24 ± 4.9 g C m-2 yr-1 during 2004 and 2005 (Nilsson et al., 2008). Early studies suggested that among the abiotic variables, Ta and WTL explain most of the variance in NEE (Yi et al., 2010), GPP and Reco (Lindroth et al., 2007; Yurova et al., 2007). The length of the growing season (Lund et al., 2010) and light availability (Nijp et al., 2015) are important variables driving summertime GPP and Reco.
Several studies demonstrated that plant development is the dominant control of C fluxes (Järveoja et al., 2018; Peichl et al., 2018; Koebsch et al., 2020). Some cross-seasonal biotic effects have been observed as well, as reflected for instance by strong correlations between growing season GPP and subsequent winter CH4 emissions (Zhao et al., 2016b). Continuous automated chamber measurements revealed a divergent diel temperature sensitivity of Reco (Järveoja et al., 2020). A synthesis study found that higher heterotrophic respiration will occur with increased temperature, decreased precipitation and lower WTL as observed during a severe drought in 2018 (Fu et al., 2020). Another global synthesis also reported that their CO2 sink strength shows a seasonal divergence to increasing temperature, with warmer early summers causing an increased net CO2 uptake and warmer late summers connected to decreased net CO2 uptake (Helbig et al., 2022).
Lab incubations have revealed that microbial activity in the peat proceed also when the peat is frozen (Segura et al., 2019). The photosynthesizing biomass of different plant functional types was also important to consider in NEE (Laine et al., 2012). This motivated the use of different satellite-derived vegetation indices (Schubert et al., 2010; Wißkirchen et al., 2013; Zhou S. et al., 2016) or digital repeat photography based chromatic coordinates (Peichl et al., 2015) to explain phenology effects on C fluxes. Remote sensing land surface temperature products were also used to estimate Reco (Ai et al., 2018). Some modelling exercises simulated the sensitivity of Degerö Stormyr and other mires to global change (Wania et al., 2009; Wu et al., 2012; Wu and Roulet, 2014; Metzger et al., 2016).
CH4 fluxes across global peatlands (including Degerö Stormyr) have been reported to be mainly controlled by WTL, peat temperature and atmospheric pressure (Knox et al., 2021). Investigations of the short- and long-term effects of plot-scale N addition and increased temperature on CH4 emission at Degerö Stormyr revealed a positive effect of temperature and a negative effect of N addition in the short-term with the opposite effects in the long-term (Granberg et al., 2001; Eriksson et al., 2010a). S pollution was found to reduce CH4 emissions in peatlands (Gauci et al., 2004). In fact, S treatment seemingly altered the vertical distribution of methanogens and sulfate-reducing bacteria, hence inhibiting CH4 production only close to the surface while no effect was observed at the depth of maximum production (Eriksson et al., 2010b). CH4 oxidation has been measured with two new in situ approaches at Degerö Stormyr, one using a two-source isotope mixing model (Nielsen et al., 2019), the other based on passive 13CH4-pulse labelling through diffuse chambers (Dorodnikov et al., 2022). Several studies modelled CH4 emissions (Zhu et al., 2014; Zhou Y. et al., 2016; Peltola et al., 2019; Ueyama et al., 2023), making use of global flux datasets (Delwiche et al., 2021).
Studies on the exchanges of water and energy revealed that plant functional type composition, precipitation, radiation amount and timing were the major drivers of the partitioning of mire energy and water budgets at Degerö Stormyr (Peichl et al., 2013). Peatlands have a great climate warming mitigation potential due to some of their biophysical properties such as their relatively higher growing season albedo compared to forests (Helbig et al., 2020a). It has also been reported that the increased sensitivity of peatland evapotranspiration and vapour pressure deficit, relative to boreal forests, puts them at particular risk to increasing air dryness (Helbig et al., 2020b).
3 Description of the Kulbäcksliden Research Infrastructure (KRI) sites
3.1 Geographical situation
In 2018, Degerö Stormyr was extended with 3 more mire sites and a drained peatland forest site to form the KRI. The KRI is located near the municipality of Vindeln, northern Sweden (Figure 2A), on an elevation between two major rivers: Umeälven and Vindelälven (Figure 2B). The research infrastructure at the peatland complex includes four natural mire catchments (Degerö Stormyr, Stortjärn, Hålmyran and Hälsingfors) and a drained peatland forest site (Hälsingfors-forest), the latter divided into two sub-catchments encompassing an open and a dense tree canopy section (Figure 2C). To limit the EC footprint to the mires, the natural mire sites were selected based on the size of the open areas in the mire complex. The few large open areas were examined to verify that they belonged to the same fen system, and that there were no apparent disparities in vegetation type or local conditions. Wind direction was considered while determining the tower location at each site, ensuring that flux measurements originate only from the mire and not the neighbouring forests. The footprints of the EC towers altogether cover comprehensively any heterogeneity in the mire complex.
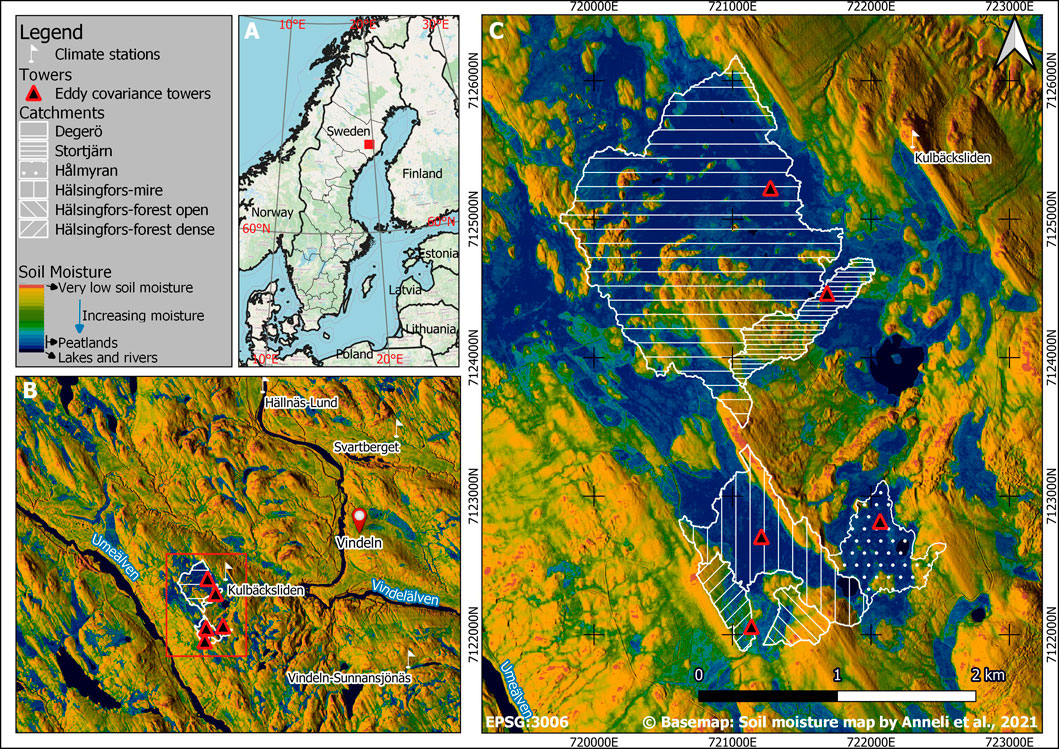
FIGURE 2. Kulbäcksliden Research Infrastructure sites location and its different catchments (A) Geographic location within Sweden (B) Situation of the catchments between two major rivers, i.e., Umeälven and Vindelälven, with a view of the nearest climate stations (white flags); black and red triangles indicate eddy covariance installations (C) Catchments with a soil moisture map (Ågren et al., 2021) background blended with a hillshade derived from a 2 m resolution digital elevation model.
The Degerö Stormyr and Stortjärn sites are located at slightly lower altitudes (265 and 268 m a.s.l., respectively) compared to Hålmyran, Hälsingfors-mire and Hälsingfors-forest (290, 291 and 287 m a.s.l., respectively). The catchment areas range from 25 ha for Hälsingfors-forest to 273 ha for Degerö Stormyr (Table 1). The prevalent winds originate from the north-west, the south and south-southeast except at Hålmyran, where the predominant wind direction is from the south, reflecting the north-south orientation of the open area created by the forested ridges at the east and west (Figure 3).

TABLE 1. Coordinates and altitudes of the EC towers and catchment areas for the Kulbäcksliden Research Infrastructure sites.
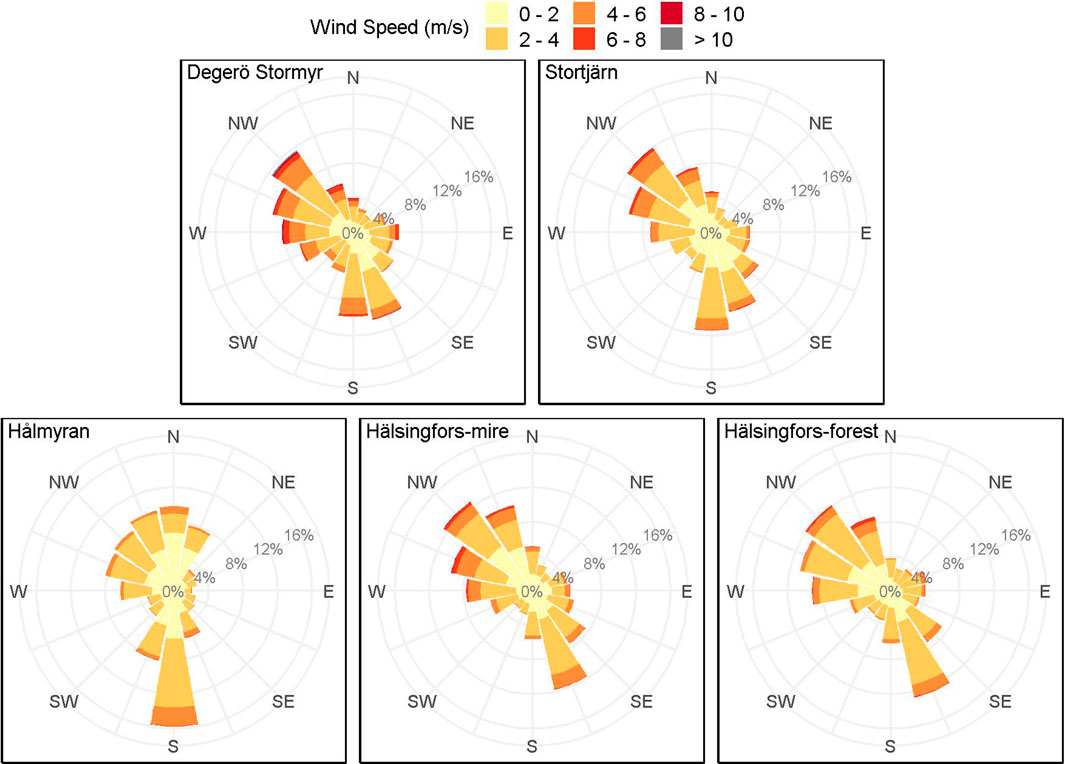
FIGURE 3. Windrose at the different peatland sites, i.e., Degerö Stormyr, Stortjärn, Hålmyran, Hälsingfors-mire and Hälsingfors-forest. The windrose is based on one year wind speed and direction data from May 2020 to April 2021.
3.2 Geology, soils and historic land use
The bedrock consists of paragneiss resulting from the Svecokarelian orogeny (1.92–1.87 billion years ago) (SGU, 1963; Ladenberger et al., 2013). Soils formed from this parent material are often among the most nutrient poor in Fennoscandia (Ivarsson and Bjarnason, 2009; Ruuhola et al., 2016). The peatland complex is situated above the highest postglacial coastline in the area, ∼257–259 m above current sea level (Laudon et al., 2021) (Figure 4) and has therefore never been inundated by the sea. However, the highest coastline is located near the edge of the peatland complex and it has been hypothesised that sea level retreat, associated with isostatic rebound, influenced the hydrology of the peatland in its early development phase, possibly resulting in erosion (Kulczyński, 1949). The quaternary deposits are dominated by till forming ridges of moraine, on which peat has formed for significant areas of the different catchments filling up many of the small depressions (SGU, 1990).
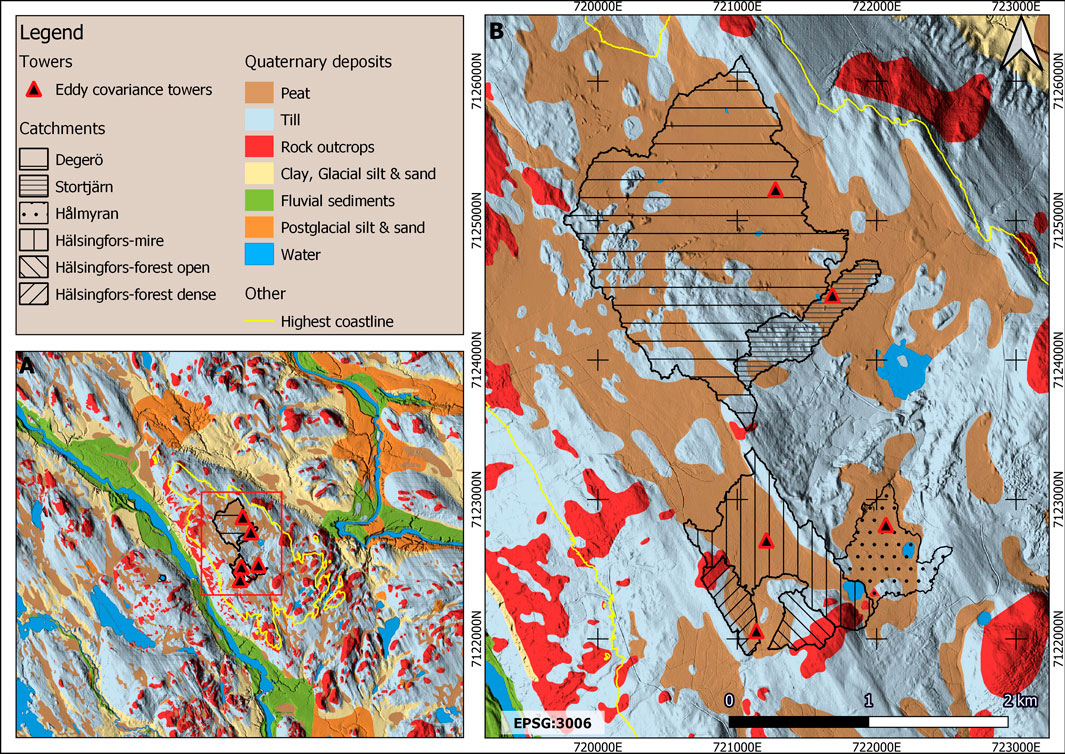
FIGURE 4. Quaternary deposits map of KRI blended with a hillshade derived from a 2 m resolution digital elevation model (A) Zoomed-out view of the quaternary deposits map showing the catchments and the highest postglacial coastline (B) zoomed-in view of the catchments with their relative share of different quaternary deposits.
Soil types are dominated by histosols and podzols (depending on the hydrology) on the till deposits. Forests cover between 47% and 72% of each mire catchment (Figure 5; Table 2). All open lands in the catchments are peatlands, whereas forests have developed on the till and to some extent on the peat covered areas (e.g., at Hälsingfors-forest) (Figure 5). Detailed fractional covers of the quaternary deposits and land use classes for the different catchments are presented in Table 2.
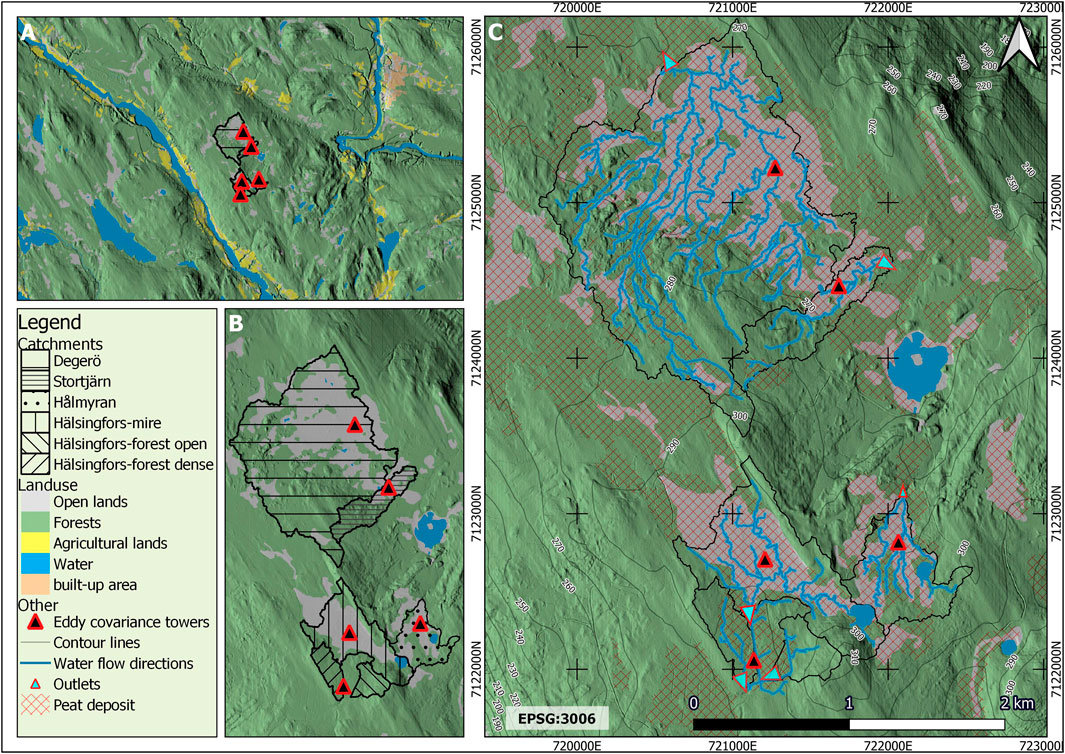
FIGURE 5. Land use and hydrology of the Kulbäcksliden Research Infrastructure (A) Zoomed-out view of the land use map (B) zoomed-in view showing the different catchments (C) zoomed-in view of the land use map indicating in blue the modelled flow direction in the different catchments.
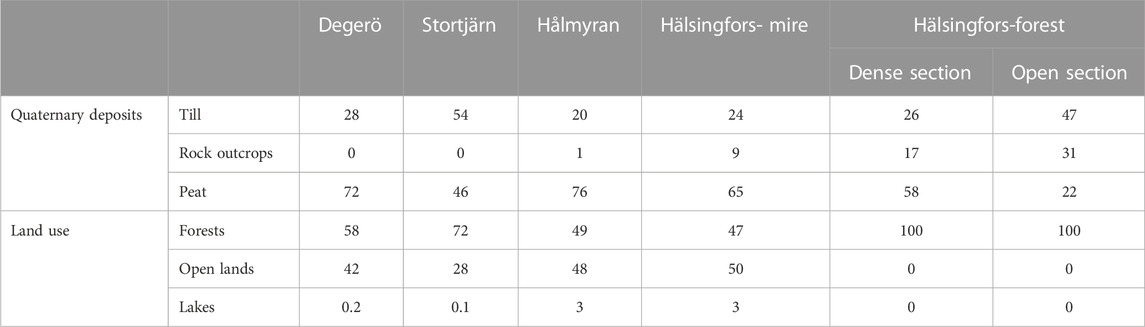
TABLE 2. Fractional cover (%) of the quaternary deposits and land uses at the different catchments. Areal covers of the different combinations of quaternary deposits and land use are available in Supplementary Table S1, APPENDIX A.
Drainage history at the peatland complex is limited to spatially restricted attempts at the edge of Degerö Stormyr back in the end of the 19th century (Malmström, 1923), in addition to the Hälsingfors-forest site which was drained by a network of ditches (still visible today) estimated to ∼ 130 years ago based on unpublished tree ring analysis data.
3.3 Hydrology
Flow accumulation modelling based on a digital elevation model (DEM) provided detailed output information on modelled flow directions at KRI (Figure 5C). More details on the flow accumulation modelling can be found in APPENDIX B of Supplementary Materials. Apart from a few ditches and small streams, most of the water movements in the catchments are surficial groundwater movements in the upper parts of the soil where the peat is less decomposed or where the till is less compacted (Ågren et al., 2008). As the peatland complex is situated at the apex of a wide and shallow ridge between two rivers, the different parts drain in different directions; Degerö and Hålmyran drain to the north, Stortjärn to the north-east, while Hälsingfors (mire and forest) drains south/south-west.
3.4 Climate
Several meteorological stations exist in the vicinity of the study area (Supplementary Table S2, Supplementary Materials APPENDIX C). A selection of the four closest stations (Figure 2B) give a representation of the span of temperature and precipitations (Table 3) in the surroundings of the study area (SLU, 2021; SMHI, 2021). Locally, topography can have a strong influence on microclimate with large differences between south and north slopes and cooler heavier air draining into the valleys and hollows.
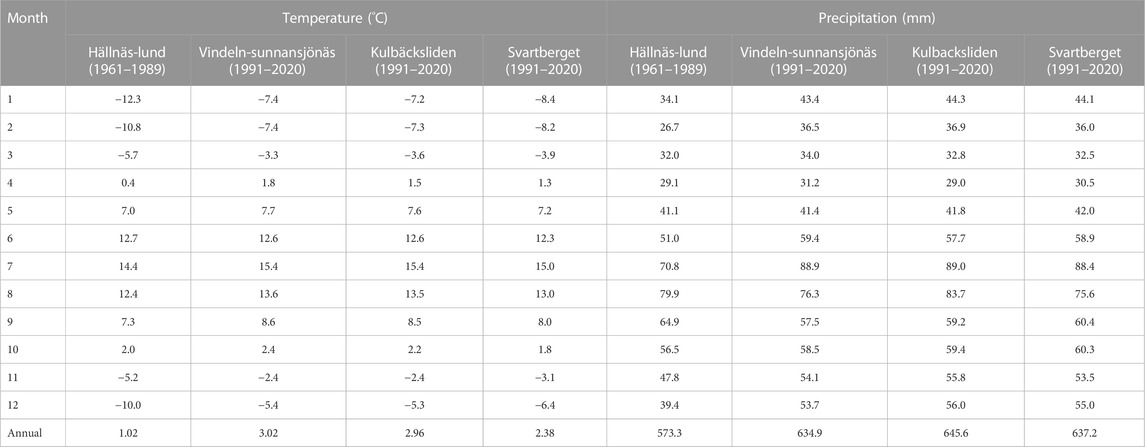
TABLE 3. Thirty years average temperature and precipitation sums for two selected stations of the Swedish Meteorological and Hydrological Institute (Vindeln-Sunnansjönäs station and Hällnäs-Lund station, 11 and 11.3 km away from the study sites respectively) and two reference stations of the Swedish University of Agricultural Sciences (Kulbäcksliden and Svartberget, 1.8 and 13.1 km away from the study sites respectively).
The climate in the area can be defined as subarctic (Dfc) after Köppen-Geiger (Peel et al., 2007), or more specifically a cold temperate humid climate with mean annual precipitation and temperature of 645 mm and +3°C, respectively, with the mean temperatures in July and January being +15.4°C and −7.4°C, respectively (Figure 6). These climate descriptors are 30 years average (1991–2020) from the SLU reference climate station Kulbäcksliden.
3.5 Vegetation classification
The vegetation at the mire sites can be grouped into six broad categories (group I to VI) depending on the microtopography (hummocks, lawns, carpets, loose-bottoms and pools) and vegetation types (Table 4). Detailed information on vegetation types as well as the classification methodology and results are available in Supplementary Materials APPENDIX D.
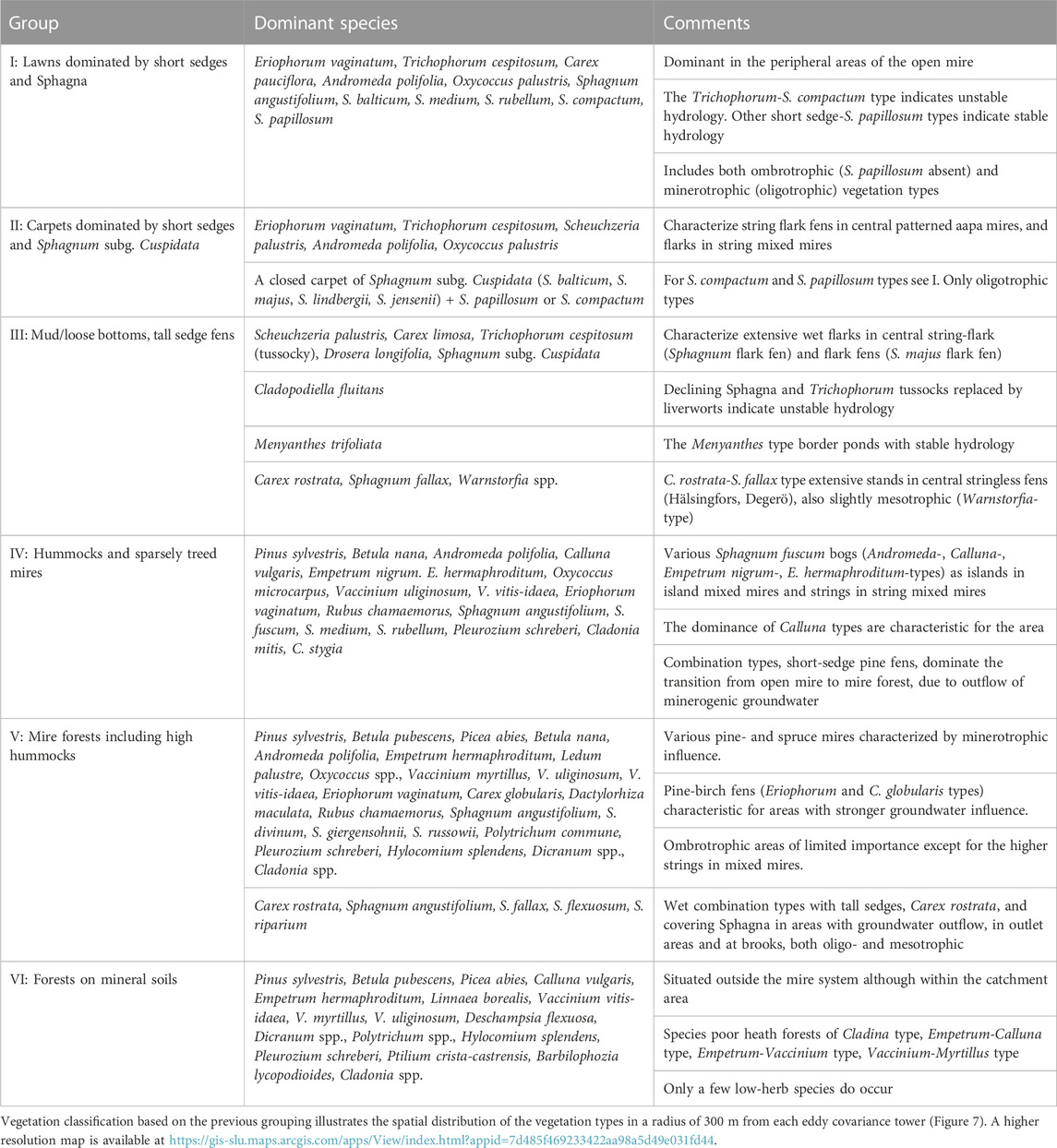
TABLE 4. Vegetation groups (visualized in Figure 7), vegetation types, important plant species, and some comments on ecology (see APPENDIX D for details).
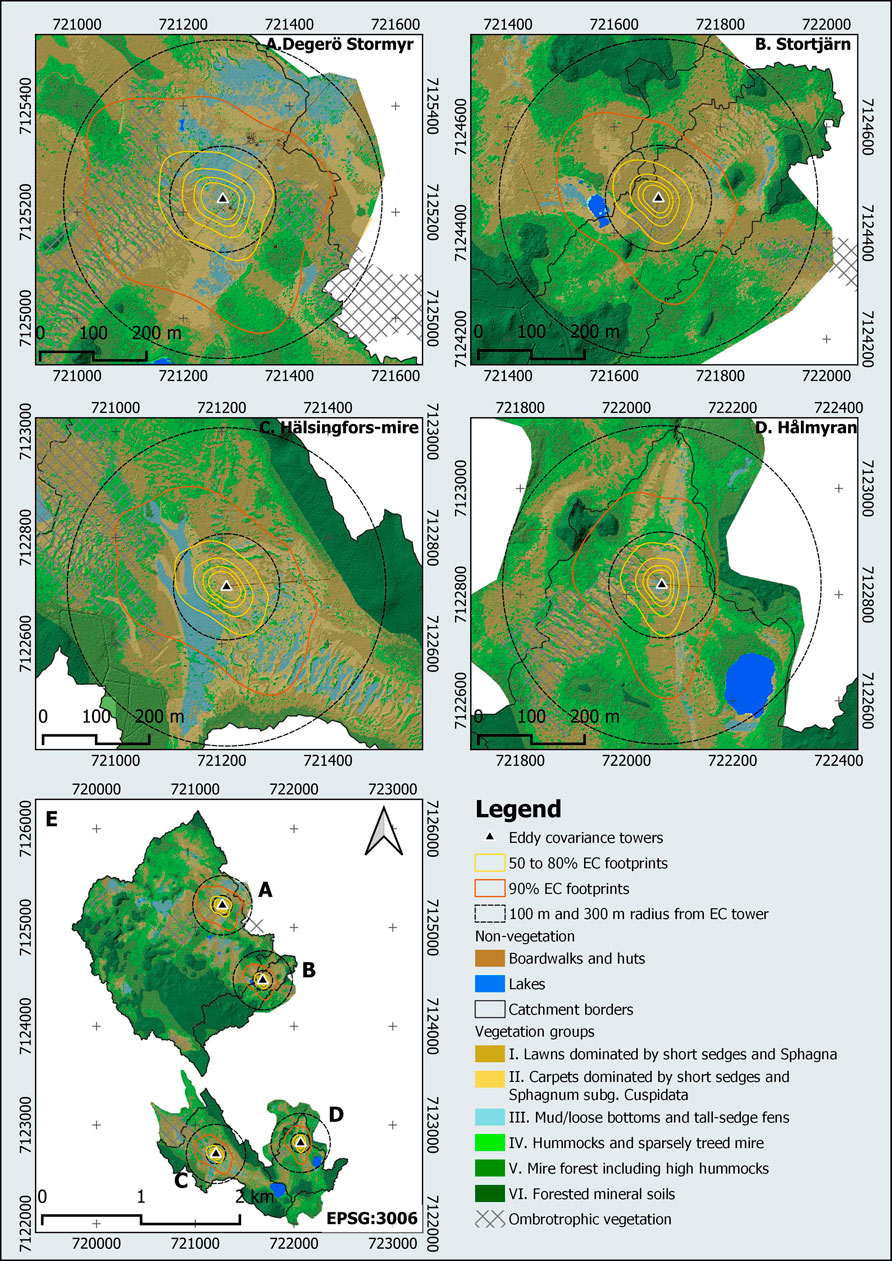
FIGURE 7. Vegetation classification map of the mire sites of the Kulbäcksliden peatland research infrastructure with a focus on a 300 m radius from EC towers at (A) Degerö Stormyr, (B) Stortjärn, (C) Hälsingfors-mire, (D) Hålmyran, and (E) an overview of all four catchments. The footprint climatologies were calculated from 1-year data wind speed and wind direction (May 2020 to April 2021) based on Kljun et al. (2015). The vegetation groups layer is blended with a hillshade derived from a 0.5 m resolution digital elevation model.
The percentage share for each of the different groups per catchment and footprint area (Table 5) shows that their cover vary considerably from one catchment to the other. Within the 90% footprint area, there is almost no forest on mineral soils (group VI) at any of the sites. It is also noticeable that the wettest vegetation groups (groups III and II) represent the dominant vegetation at Degerö Stormyr and Hälsingfors in the 90% footprint area whereas the drier lawn communities (group I) prevail at Stortjärn and Hålmyran.
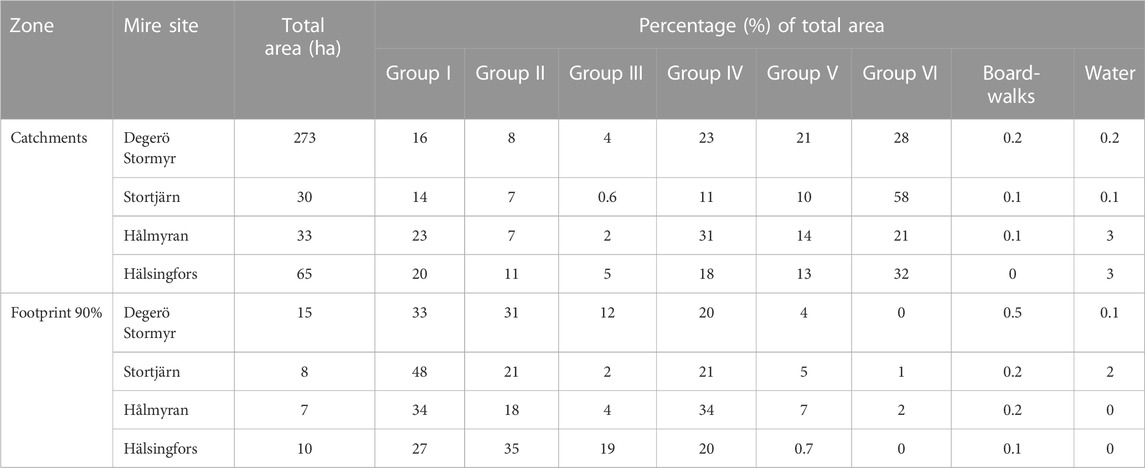
TABLE 5. Percentage share of the different groups of vegetation per catchment, 80% and 90% footprint climatology area. The same information for 50%, 60% and 70% footprint climatology areas is available in Supplementary Table S3 of Supplementary Materials APPENDIX D.
At the forested site (Hälsingfors-forest), Pinus sylvestris is dominant (90%) in the open forest section, while Picea abies and Betula pubescens are dominant (37% and 56%, respectively) in the dense forest section. The understorey in the open section is composed of dwarf-shrub species such as Andromeda polifolia, Calluna vulgaris, Empetrum hermaphroditum, Oxycoccus microcarpus, the graminoid Eriophorum vaginatum and Sphagnum spp. (Sphagnum angustifolium, Sphagnum fuscum) together with Pleurozium schreberi. The understorey in the dense forest section, a mesic-moist dwarf-shrub type, is dominated by Vaccinium myrtillus and Vaccinium vitis-idaea together with Rubus chamaemorus and forest mosses Hylocomium splendens, P. schreberi and Dicranum spp.
4 Instrumentation and experiments
The main instrument installations at KRI (Supplementary Table S4, Supplementary Materials APPENDIX E) are almost all located within the 90% footprint of the EC towers (Figure 8).
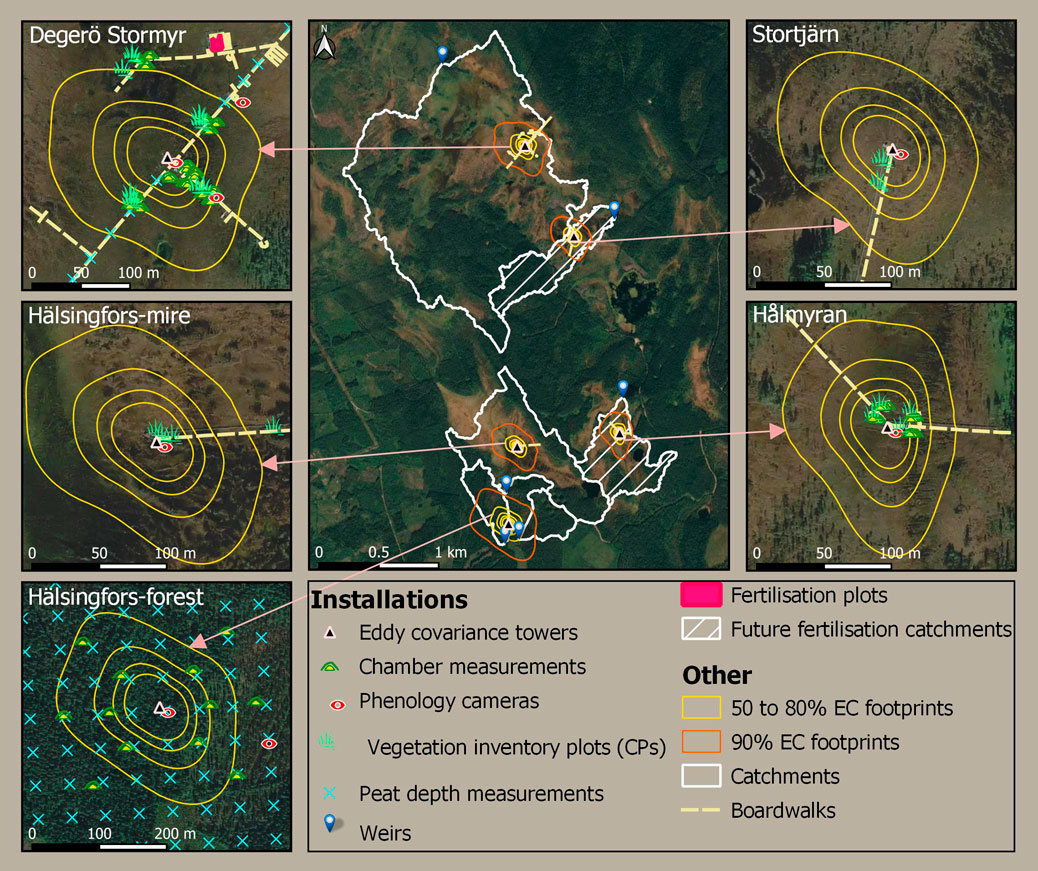
FIGURE 8. Location of the main installations and experiments at the Kulbäcksliden Research Infrastructure (KRI). The footprint climatologies were calculated from one year data wind speed and wind direction (May 2020 to April 2021) based on Kljun et al. (2015).
4.1 Eddy covariance flux towers for monitoring land-atmosphere exchanges of CO2, CH4, water vapour, energy and Hg0
An EC flux tower is located in the centre of each of the sites within KRI (Figure 8). The EC tower at Degerö Stormyr was established in 2001, and has since then provided near-continuous measurements of CO2 and water vapour (H2O) (Nilsson et al., 2022). The EC towers at the remaining sites were established during 2019 and are in operation since 2020. The instrumental setup is consistent at the new sites, whereas the need for standardisation across the Integrated Carbon Observation System (ICOS) was considered at the ICOS Degerö station. Specifically, the EC setup at Degerö initially included a LI-6262 gas analyser which was replaced by a LI-7200 gas analyser in 2014. Since 2013 an additional combined CO2/H2O and CH4 analyser (Los Gatos Research, LGR FGGA 911-0010) was added to the EC system at Degerö Stormyr. The three new mire stations Stortjärn, Hålmyran and Hälsingfors-mire are equipped with a CO2-CH4-H2O Picarro G2311-f analyser. At the Hälsingfors-forest site, the EC system includes a LGR CO2-CH4-H2O analyser (LGR FGGA 908-0010). As part of the above described EC systems, a 3D ultrasonic anemometer has been installed at each site to measure wind speed and wind direction. Current installations consist of a Gill HS-50 anemometer installed at a height of 3.07 m at Degerö Stormyr in conformity with ICOS standards, and a Metek uSonic-3 Class A anemometer was mounted at the other KRI sites, at 2.75 m height at the other three mire sites and at 20.2 m height at the Hälsingfors-forest. The current installations are closed path systems so the air inlet is mounted at the same height as the anemometer at each site.
The land-atmosphere exchange of gaseous elemental mercury (Hg0) is measured using the EC technique at Degerö Stormyr and Hälsingfors-forest sites with two new Eddy Mercury 2.0 systems installed in October 2021. The systems were developed based on the first Eddy Mercury system that measured grassland-atmosphere exchange of Hg0 at the Swiss FluxNet site Chamau (CH-CHA) in 2018 (Osterwalder et al., 2020). In short, the Eddy Mercury 2.0 consists of a Metek uSonic-3 Class A MP anemometer to record wind data and of a Lumex RA-915 a.m. mercury monitor (Lumex Ltd.) to measure ambient Hg0 concentration. The uSonics and air inlets for both closed-path systems were mounted at 2.75 m height at Degerö Stormyr and at 20.2 m height at Hälsingfors-forest. Specific software was implemented to measure the Hg0 concentrations at a frequency of 16 Hz and to achieve real-time merging of high resolution data from both the uSonic and the RA-915 am. The Hg0 fluxes are calculated into 30 min averages and flux data quality control procedures are established based on data quality control information from the concurrently running CO2-CH4-H2O EC system to retain or reject Hg0 fluxes. In addition, long-term ambient Hg0 concentration measurements using Mercury Passive Air Sampler (MerPAS, Tekran Instruments Corporation) were initiated at Degerö Stormyr in 2022. At Degerö Stormyr, ambient Hg0 concentration and peatland-atmosphere exchange of Hg0 have been measured intermittently with diverse methods since 2009 (Fritsche et al., 2014; Osterwalder et al., 2016; 2017; 2018).
4.2 Ancillary meteorological and soil environmental measurements
Each flux tower is equipped with a standard suite of instrumentation for measuring meteorological variables to support the EC data. At the Hälsingfors-forest site, meteorological sensors are installed on the flux tower as well as on a separate flagpole to monitor the dense and open forest parts of the study site, respectively. At each site, the current instrumentation includes.
• Ta and humidity sensors (Rotronic MP102H-331000 at Degerö Stormyr and HC2S3 at the other sites),
• Rain gauges (Lambrecht Rain [e]H3 at Degerö Stormyr and ARG100 at the other sites except Hälsingfors-forest which has no precipitation sensor),
• Global radiation sensors (CMP21, Kipp and Zonen only at Degerö Stormyr),
• Incoming and outgoing shortwave/longwave radiation sensors (CNR4, Kipp and Zonen at Degerö Stormyr and NR01 Campbell Scientific (CS) at the other sites),
• Photosynthetically active radiation (PAR) sensors (LI-190 at all sites).
In addition, Ts at 2, 10, 15, 30, and 50 cm depth and WTL measurements are carried out at replicated plots (six at Degerö Stormyr, two at each of the other sites). The instrumentation includes Ts sensors (Fischer Pt 100 at Degerö Stormyr and TR03 TOJO Skogsteknik at the other sites) and WTL sensors (CS450 at Degerö Stormyr and CS451 at the other sites).
4.3 Phenology observations
To monitor the phenology of the vegetation at each of the peatland sites, digital repeat photography is applied through the installation of phenology cameras (Figure 8). At Degerö Stormyr, a Canon A480 operated from 2011 to 2014, substituted by a Canon PowerShot A810 between 2014 and 2022, itself replaced in 2022 by a Mobotix Mx-M26B-6D. In addition since 2016, a Stardot Netcam SC camera was in operation at Degerö Stormyr as part of ICOS and a Mobotix Mx-M26B-6D061 as part of the Swedish Infrastructure for Ecosystem Sciences (SITES)-Spectral. SITES-Spectral also supports the monitoring of plant vegetation indices (NDVI, SWIR, PRI) as a proxy for vegetation development using fixed spectral sensors (SKYE; SK-1860) mounted to a 20 m flagpole. At each of the other mire sites, a Canon PowerShot A810 camera was in operation since 2020, replaced by Stardot Netcam SC cameras in 2022. At Hälsingfors-forest, two Wingscapes Timelapse cameras were in operation from 2020 to 2022, monitoring each the dense and open areas of the forest. In 2022, they were replaced with Stardot Netcam SC cameras. The phenology cameras record images with a 1-h time resolution and oversee the northern parts of the main EC footprint. In addition to the phenology cameras, NDVI is also monitored using fixed-spectral sensors (Decagon SRS) at the new KRI sites.
4.4 Multispectral UAV images at the mire sites
Since 2021, aerial images were collected over the four mire sites, covering at least the 80% footprint of the EC tower at each site. The campaigns were conducted towards the end of each month, from May to September. In 2021, a MAIA multispectral camera mounted on a DJI matrice UAV was used at a flight height of 60 m for the campaigns except in July when a Parrot Sequoia multispectral camera mounted on a 3DR Solo UAV was used instead at a height of 30 m. In 2022, a DJI Phantom 4 multispectral UAV was flown at ∼55 m height. In either case, the resulting orthomosaics had ∼3 cm pixel resolution on the ground.
4.5 Continuous vegetation inventory plots
In order to assess the growth of vascular plants and mosses, continuous measurement plots (CP) have been installed at each of the mire sites (Figure 8), consisting of 60 × 60 cm squares. In total, 16 CPs were installed at Degerö Stormyr and four CPs at each of the other three mires, spanning both lawn and hummock locations. At each CP, five subplots of 8 × 8 cm are marked in which vegetation height is measured every second week at the CP level, i.e., the average height of the ten tallest individuals in the CP. Green area index and above ground biomass are also estimated at the species level in the subplots using a combination of non-destructive methods within the subplots and destructive methods outside the CP, with specific approaches depending on the plant functional type. More specifically, for mosses a brush wire is inserted into the peat, and moss growth is derived from the height difference of the visible part of the brush at beginning and end of the growing season. In addition, the number of living moss stems (moss stem density) is counted per species in the subplots at the end of the growing season, and samples of the moss carpet that have grown out of a mesh placed at the beginning of the growing season are taken for dry weight calculation. For vascular plants, the number of leaves and stems of individuals is counted inside the CP and the average leaf area and dry weight are calculated on the samples collected outside the CP. All measurements are carried out following the ICOS protocol for mire vegetation monitoring (ICOS, 2020).
4.6 Manual and automated chambers for measuring CH4 and CO2 land-atmosphere exchanges
Fluxes of CH4 and CO2 have been measured using manual chambers (Figure 8) at Degerö Stormyr between 2004 and 2014 at the original six CPs, as well as at experimental N-S-addition, warming and snow exclusion plots (Eriksson et al., 2010a; Zhao et al., 2016a). The CO2 fluxes were quantified in situ with a custom-made portable infrared gas analyzer system (with a PP Systems measurement cell), while repeated air samples drawn with a syringe were analyzed in a gas chromatograph in the lab to determine CH4 fluxes. Between 2014 and 2018, a LGR UGGA analyzer was used to measure both CH4 and CO2 fluxes in the field.
Since 2018, forest floor CO2 and CH4 fluxes have been measured every second week at Hälsingfors-forest with the closed dynamic chamber technique during snow-free seasons. Measurements are taken at 12 spatially equally distributed plots (Figure 8) in the open and dense forest sections, where two measurement frames (48.5 × 48.5 cm) were inserted into the soil, one in a natural setting and the other in an area where all aboveground plant and moss vegetation was clipped and removed within the measurement frame in order to estimate heterotrophic respiration. The fluxes were determined with a LGR UGGA (model 915-0011) in 2018 and with a Picarro GasScouter™ G4301 since 2019.
A custom-made automated chamber system has been in operation at Degerö Stormyr since 2014 (Järveoja et al., 2018; 2020; Nielsen et al., 2019). This system includes 12 chambers connected in a closed loop to originally a CO2-CH4 LGR analyzer (model GGA-24 E P). The chambers are located within the EC footprint (Figure 8) and distributed in four replicate groups over natural and trenching/vegetation removal plots to partition the hourly to 2-hourly resolution NEE into GPP, net primary production, and Reco, i.e., both autotrophic respiration and heterotrophic respiration, using a mass balance approach (Järveoja et al., 2018). In 2017, the LGR analyzer was replaced by a Picarro isotope analyzer (Picarro G1101-I.) that also determines the 12/13C stable isotope ratios for both CO2 and CH4. Combined with the experimental plot setup, this allows for quantifying CH4 oxidation (Nielsen et al., 2019). In 2020, additional moss-only plots were established by clipping all vascular plants to separate the contributions from vascular plants and mosses. At each chamber, headspace Ta and PAR, Ts (2 and 10 cm depth) and WTL are recorded. Additionally, vegetation inventory conducted every second week and seasonal brush wire measurements deliver chamber-specific information on plant and moss production, respectively.
In 2022, a second automated chamber system was installed at the Hålmyran mire within the EC flux footprint. This system also includes 12 chambers (Figure 8) and mirrors the latest experimental set up at Degerö Stormyr to estimate NEE, GPP and Reco at natural and moss-only plots, and Rh at vegetation removal plots. Additionally, the same abiotic (Ta, Ts, PAR and WTL) and vegetation data (vascular plants and moss growth) are collected at each chamber. In contrast to the custom-built Degerö Stormyr system, the Hålmyran system is equipped with Eosense chambers (model eosAC-LT/LO) and the CO2-CH4 concentrations are determined with a LGR UGGA analyzer (model 915-0011).
4.7 Continuous measurements of water discharge and water chemistry
A weir has been installed at the outlet of each catchment and equipped with sensors for recording hourly average water height, partial pressure of CO2 (pCO2), water and Ta (Figure 5). The weir at Degerö Stormyr is located inside a small house set-up on a flume and heated during winter, hence allowing for year-round measurements. The weirs at the other sites are a V-Notch type set-up in open-air, causing discontinuous measurements during winter and so the wintertime data is derived through regressions with the nearby heated and non-heated weirs. In addition to the sensor measurements at the weirs, water samples are collected once to three times a week during the freshet, i.e., spring flood, once a month during winter and every second week the rest of the time. The collected samples are analyzed for pH, total organic C (TOC), dissolved inorganic C (DIC), CH4-C, 18O, nutrients, anions, cations, methylmercury, total mercury and the dissolved organic C absorbance and fluorescence properties (Leach et al., 2016).
4.8 Warming, nitrogen and sulphur addition and snow exclusion plot-scale experiments
In order to investigate the response of mire vegetation to global changes, a 21-plots factorial experiment with warming effect (greenhouse treatment) as well as N (as ammonium nitrate, i.e., NH4NO3) and S (as sodium sulfate, i.e., Na2SO4) additions was set up in 1995, and is still maintained at Degerö Stormyr. The greenhouse enclosure increases the average yearly temperature by 3.6 °C. N and S are added at rates of 30 and 20 kg ha-1 yr-1, respectively as compared to their background levels or controls of 2 and 3 kg ha-1 yr-1 for N and S, respectively. The full experimental setting was described by Granberg et al. (2001).
Snow exclusion plots were set up since 2003 in order to study the effects of an enhanced soil frost regime (which could happen naturally as a consequence of snow reduction induced by the ongoing climate change) on different biogeochemical processes. In total, six plots (3 × 3 m each) separated by a 1.5 m buffer zone were created and snow is excluded via a temporary enclosure every winter from three of the plots, while the other three plots remain uncovered and serve as controls (Zhao et al., 2016a).
4.9 Replicated ecosystem-scale N addition experiment
Starting in 2024, a unique ecosystem-scale N-fertilization experiment will be carried out at the Stortjärn and Hålmyran catchment sites. Specifically, N in the form of pellets will be spread via helicopter over the entire catchment and EC footprint areas once per year before mid-summer, at a rate of ∼10 kg ha-1 yr-1. Dedicated subplots will be covered during the helicopter N application and manually fertilized to ensure consistent N addition rate for experimental plot-scale studies. This experiment will provide an exceptional opportunity to study the ecosystem-scale responses of the water and energy balances to N addition, and how these interact with changes in mire biogeochemistry and land-atmosphere exchanges of GHGs, water and energy.
4.10 LiDAR data
The Kulbäcksliden area has been scanned with LiDAR repeatedly, twice in the national LiDAR scans by Lantmäteriet; in 2010 with a point density of 0.5 points m-2 and in 2019 with a point density of one to two points m-2. In addition, the area has been scanned twice during different research campaigns, allowing for high resolution DEMs down to 0.5 m resolution and providing detailed ground- and tree-level data. The first specific scanning was conducted in 2006 by Blom Geomatics at a flight elevation of 1,100 m with a point density of up to 10 points m-2. In 2019, the area was scanned with a Riegl VQ-1560i-DW 532 nm (green) and 1,064 nm (NIR) with an average point density of 20 points m-2.
4.11 Ground penetrating radar (GPR) data and other peat depth measurements
During winter in 2016, a campaign was conducted to measure peat thickness at Degerö Stormyr using a MALÅ ground-penetrating radar (MALÅ Geosciences, Sweden) with a 100 MHz shielded antenna towed using a snowmobile. Traces (16 stacks each) were spaced 0.1 m and a time window of 790 ns provided a maximum detection depth of 13 m, and a resolution of ∼25 cm. In total, twenty parallel transects (each 500–2,500 m long) with 100 m spacing were surveyed, making it a total length of 25 km. Prior to the GPR campaign, 15 manual peat depth measurement points along the main boardwalk were surveyed as well, and 250 additional control points were manually measured with a probe to validate the GPR data. The peat depth at Degerö Stormyr ranges between 3 and 4 m in average, with some areas having up to 8 m peat thickness. At the Hälsingfors-forest site, peat depth was measured manually with a probe at 81 locations organised in a regular grid of 9 × 9 points with 50 m spacing showing a mean depth of 142 ± 13 cm and 65 ± 7 cm in the open and dense sections of the forest, respectively (C.H.M. Tong, personal communication, 12 April 2022).
4.12 Forest inventory at Hälsingfors-forest
A forest inventory was conducted within a 10 m radius around each of the 12 chamber measurement plots at the Hälsingfors-forest in May 2018. The average stem volume was 52 m3 ha-1 in the open section and 131 m3 ha-1 in the dense section of the forest. In November 2020, increment cores collected from 147 sample trees across the range of tree diameters at Hälsingfors-forest were examined to reconstruct annual series of the individual tree growth using standard dendrochronological methods (Cook and Kairiukstis, 1990). The average biomass production was 55 g C m-2 yr-1 in the open section and 114 g C m-2 yr-1 in the dense section of the forest during the years 2019 and 2020.
5 Global relevance of KRI
Degerö Stormyr is one of the most studied mires in the boreal biome, and its global relevance has grown during the last decade (Figure 9). The increasing importance further results from the labelling of Degerö Stormyr as an ICOS ecosystem station in 2019, and from contributing data to several international flux networks and databases: FLUXNET (Papale et al., 2012), the COSORE soil respiration and other soil-atmosphere fluxes database (Bond-Lamberty et al., 2020), FLUXNET-CH4 (Delwiche et al., 2021), the arctic-boreal CO2-flux (ABC-flux) database (Virkkala et al., 2022).
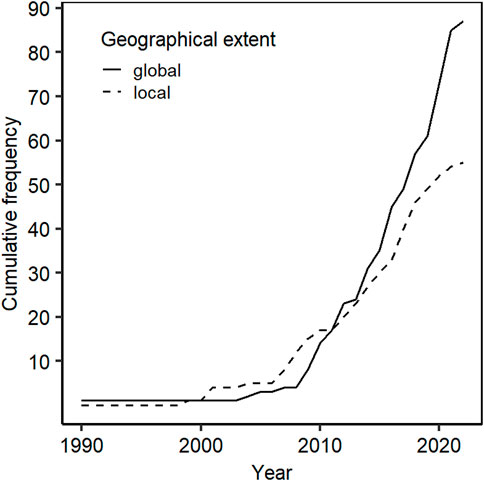
FIGURE 9. Evolution of the cumulative frequency of local vs global studies involving Degerö Stormyr throughout years.
The recently established KRI extends Degerö Stormyr with four additional peatland research sites which creates a unique setting for replicated plot-to ecosystem-scale studies of biogeochemistry and hydrology, including their responses to global changes across northern peatland complexes. Thus, this setup overcomes the traditional spatial limitations in field-based studies, thereby enabling investigations of key drivers regulating the observed spatial differences in processes and refining budget estimates for heterogeneous peatland landscapes.
We hypothesize that the nutrient status of the different sites within the peatland complex would be influenced by their catchment sizes and how much nutrient come from the surrounding mineral soils. Additionally, the orientation of the peatland complex in the landscape and the distinct hydrological pathways around each site would affect water table dynamics and lead to unique vegetation communities, resulting in differences in biogeochemical processes at each site, despite their apparent similarities. Measurements at the research infrastructure will facilitate the investigation of any eventual differences between sites.
The location of KRI, i.e., in the middle boreal zone in a transition area where more southern peatland types are gradually replaced by and co-exist with northern types (Ruuhijärvi, 1983; Laitinen et al., 2007) further provides an attractive platform for northern peatland studies. In fact, within KRI, different mire types (aapa mires, bogs and intermediate types) co-occur in a sort of dynamic equilibrium with the prevailing abiotic conditions (i.e., climate, geology, nutrient levels), and the sites are easily accessible within a limited area. Supported by long-term data records, the KRI therefore offers unique possibilities to study how global changes in, e.g., temperature, precipitation and nutrient availability as well as their interactions may affect species composition and ecosystem processes related to peatland biogeochemistry and hydrology. The KRI is embedded in the national infrastructures of ICOS and SITES which support several of the routine data collection activities as well as the general site maintenance (i.e., physical and technical infrastructure components). Thus, the KRI provides a research hub to support collaborations and interdisciplinary research in order to advance our understanding of northern peatland functioning and feedbacks with the environment and climate.
Apart from the advantages offered by the KRI, its main limitation is the considerable effort in terms of funding and labour that are required to coordinate and maintain such a research infrastructure. The general support from national infrastructures (SITES, ICOS) helps to overcome this challenge. In addition, while the KRI settings are typical for boreal nutrient-poor fen systems, the spatial variability within the KRI peatland complex system could still be specific and thus difficult to generalize across other forms of peatland complexes within the boreal biome. This highlights the need for more peatland complex infrastructures, which will reduce the uncertainties related to upscaling of fluxes from single towers or plot level measurements.
Author contributions
KN, JR, AÅ, MÖ, MN, and MP conceived and designed the study. KN, AÅ, MN, LE, CT, JJ, WZ, SO, HP, JR, MÖ, and MP contributed to gathering required information for drafting the manuscript. KN synthesized the information and drafted the manuscript. All authors contributed to the article and approved the submitted version.
Funding
Research and instrumentation at the Kulbäcksliden Research Infrastructure sites have been funded by project grants from the Swedish Research Council (VR, grant no. 2018-03966) and the Swedish Research Council for Spatial Planning (FORMAS, grant no. 2016-01289), as well as by infrastructure grants from the Kempe Foundation (grant no. JCK-1712) and the Swedish University of Agricultural Sciences (SLU). The sites contribute to the Swedish Infrastructure for Ecosystem Science (SITES) and Degerö Stormyr is part of the Swedish Integrated carbon Observation System (ICOS-Sweden) research infrastructure. Financial support from the Swedish Research Council and contributing research institutes to both SITES and ICOS-Sweden are acknowledged.
Acknowledgments
We thank the state-owned forest company Sveaskog for allowing for research to be carried out at Kulbäcksliden. We thank the engineers and technicians from the SLU Unit for Field-based Forest research for maintenance and operation of equipment and experiments at the Kulbäcksliden Research Infrastructure.
Conflict of interest
The co-author JJ is also co-editor of the special issue “Wetland Ecology and Biogeochemistry Under Natural and Human Disturbance-Volume II”.
Publisher’s note
All claims expressed in this article are solely those of the authors and do not necessarily represent those of their affiliated organizations, or those of the publisher, the editors and the reviewers. Any product that may be evaluated in this article, or claim that may be made by its manufacturer, is not guaranteed or endorsed by the publisher.
Supplementary material
The Supplementary Material for this article can be found online at: https://www.frontiersin.org/articles/10.3389/feart.2023.1194749/full#supplementary-material
References
Abdalla, M., Hastings, A., Truu, J., Espenberg, M., Mander, Ü., and Smith, P. (2016). Emissions of methane from northern peatlands: A review of management impacts and implications for future management options. Ecol. Evol. 6 (19), 7080–7102. doi:10.1002/ece3.2469
Ågren, A. M., Buffam, I., Berggren, M., Bishop, K., Jansson, M., Laudon, H., et al. (2008). Dissolved organic carbon characteristics in boreal streams in a forest-wetland gradient during the transition between winter and summer. J. Geophys. Res. Biogeosciences 113 (G3), G03031. doi:10.1029/2007jg000674
Ågren, A. M., Larson, J., Paul, S. S., Laudon, H., and Lidberg, W. (2021). Use of multiple LIDAR-derived digital terrain indices and machine learning for high-resolution national-scale soil moisture mapping of the Swedish forest landscape. Geoderma 404, 115280. doi:10.1016/j.geoderma.2021.115280
Ai, J., Jia, G., Epstein, H. E., Wang, H., Zhang, A., and Hu, Y. (2018). MODIS-based estimates of global terrestrial ecosystem respiration. J. Geophys. Res. Biogeosciences 123 (2), 326–352. doi:10.1002/2017jg004107
Åkerblom, S., Bignert, A., Meili, M., Sonesten, L., and Sundbom, M. (2014). Half a century of changing mercury levels in Swedish freshwater fish. Ambio 43 (1), 91–103. doi:10.1007/s13280-014-0564-1
Åkerblom, S., Bishop, K., Björn, E., Lambertsson, L., Eriksson, T., and Nilsson, M. B. (2013). Significant interaction effects from sulfate deposition and climate on sulfur concentrations constitute major controls on methylmercury production in peatlands. Geochimica Cosmochimica Acta 102, 1–11. doi:10.1016/j.gca.2012.10.025
Åkerblom, S., Nilsson, M. B., Skyllberg, U., Björn, E., Jonsson, S., Ranneby, B., et al. (2020). Formation and mobilization of methylmercury across natural and experimental sulfur deposition gradients. Environ. Pollut. 263, 114398. doi:10.1016/j.envpol.2020.114398
Alekseychik, P., Korrensalo, A., Mammarella, I., Launiainen, S., Tuittila, E. S., Korpela, I., et al. (2021). Carbon balance of a Finnish bog: Temporal variability and limiting factors based on 6 years of eddy-covariance data. Biogeosciences 18 (16), 4681–4704. doi:10.5194/bg-18-4681-2021
Bechtold, M., De Lannoy, G. J. M., Koster, R. D., Reichle, R. H., Mahanama, S. P., Bleuten, W., et al. (2019). PEAT-CLSM: A specific treatment of peatland hydrology in the nasa catchment land surface model. J. Adv. Model. Earth Syst. 11 (7), 2130–2162. doi:10.1029/2018ms001574
Belyea, L. R., and Baird, A. J. (2006). Beyond “the limits to peat bog growth”: Cross-scale feedback in peatland development. Ecol. Monogr. 76 (3), 299–322. doi:10.1890/0012-9615(2006)076[0299:btltpb]2.0.co;2
Berggren, M., Laudon, H., and Jansson, M. (2009). Hydrological control of organic carbon support for bacterial growth in boreal headwater streams. Microb. Ecol. 57 (1), 170–178. doi:10.1007/s00248-008-9423-6
Berggren, M., Laudon, H., and Jansson, M. (2007). Landscape regulation of bacterial growth efficiency in boreal freshwaters. Glob. Biogeochem. Cycles 21 (4). doi:10.1029/2006gb002844
Bergman, I., Bishop, K., Tu, Q., Frech, W., Åkerblom, S., and Nilsson, M. (2012). The influence of sulphate deposition on the seasonal variation of peat pore water methyl Hg in a boreal mire. PLoS ONE 7 (9), e45547. doi:10.1371/journal.pone.0045547
Bishop, H. K., and Lee, Y.-H. (1997). “Catchments as a source of mercury/methylmercury in boreal surface waters,” in Metal ions in biological systems. Editors A. Sigel, and H. Sigel (Basel: Marcel Dekker), 34, 113–130. Retrieved from https://books.google.se/books?hl=sv&lr=&id=VCRxnObbsSIC&oi=fnd&pg=PA113&ots=s1FOo58B2x&sig=ZspCsyNVP-2ON3k9Dsb95_MUy2s&redir_esc=y#v=onepage&q&f=false.
Bishop, K., Shanley, J. B., Riscassi, A., de Wit, H. A., Eklöf, K., Meng, B., et al. (2020). Recent advances in understanding and measurement of mercury in the environment: Terrestrial Hg cycling. Sci. Total Environ. 721, 137647. doi:10.1016/j.scitotenv.2020.137647
Bond-Lamberty, B., Christianson, D. S., Malhotra, A., Pennington, S. C., Sihi, D., AghaKouchak, A., et al. (2020). Cosore: A community database for continuous soil respiration and other soil-atmosphere greenhouse gas flux data. Glob. Change Biol. 26 (12), 7268–7283. doi:10.1111/gcb.15353
Branfireun, B. A., Bishop, K., Roulet, N. T., Granberg, G., and Nilsson, M. (2001). Mercury cycling in boreal ecosystems: The long-term effect of acid rain constituents on peatland pore water methylmercury concentrations. Geophys. Res. Lett. 28 (7), 1227–1230. doi:10.1029/2000gl011867
Bubier, J., Costello, A., Moore, T. R., Roulet, N. T., and Savage, K. (1993). Microtopography and methane flux in boreal peatlands, northern Ontario, Canada. Can. J. Bot. 71 (8), 1056–1063. doi:10.1139/b93-122
Burdun, I., Bechtold, M., Sagris, V., Lohila, A., Humphreys, E., Desai, A. R., et al. (2020). Satellite determination of peatland water table temporal dynamics by localizing representative pixels of A SWIR-based moisture index. Remote Sens. 12 (18), 2936. doi:10.3390/rs12182936
Campeau, A., Bishop, K. H., Billett, M. F., Garnett, M. H., Laudon, H., Leach, J. A., et al. (2017). Aquatic export of young dissolved and gaseous carbon from a pristine boreal fen: Implications for peat carbon stock stability. Glob. Change Biol. 23 (12), 5523–5536. doi:10.1111/gcb.13815
Chu, H., Luo, X., Ouyang, Z., Chan, W. S., Dengel, S., Biraud, S. C., et al. (2021). Representativeness of Eddy-Covariance flux footprints for areas surrounding AmeriFlux sites. Agric. For. Meteorology 301–302, 108350. doi:10.1016/j.agrformet.2021.108350
Delwiche, K. B., Helen Knox, S., Malhotra, A., Fluet-Chouinard, E., McNicol, G., Feron, S., et al. (2021). FLUXNET-CH<sub>4</sub>: A global, multi-ecosystem dataset and analysis of methane seasonality from freshwater wetlands. Earth Syst. Sci. Data 13, 3607–3689. doi:10.5194/essd-13-3607-2021
Desai, A. R., Murphy, B. A., Wiesner, S., Thom, J., Butterworth, B. J., Koupaei-Abyazani, N., et al. (2022). Drivers of decadal carbon fluxes across temperate ecosystems. J. Geophys. Res. Biogeosciences 127 (12). doi:10.1029/2022jg007014
Dorodnikov, M., Knorr, K.-H., Fan, L., Kuzyakov, Y., and Nilsson, M. B. (2022). A novel belowground in-situ gas labeling approach: CH4 oxidation in deep peat using passive diffusion chambers and 13C excess. Sci. Total Environ. 806, 150457. doi:10.1016/j.scitotenv.2021.150457
Dunn, C., and Freeman, C. (2014). Peatlands: Our greatest source of carbon credits? Carbon Manag. 2 (3), 289–301. doi:10.4155/Cmt.11.23
Eppinga, M. B., Rietkerk, M., Belyea, L. R., Nilsson, M. B., De Ruiter, P. C., and Wassen, M. J. (2010). Resource contrast in patterned peatlands increases along a climatic gradient. Ecology 91 (8), 2344–2355. doi:10.1890/09-1313.1
Eppinga, M. B., Rietkerk, M., Wassen, M. J., and De Ruiter, P. C. (2007). Linking habitat modification to catastrophic shifts and vegetation patterns in bogs. Plant Ecol. 200 (1), 53–68. doi:10.1007/s11258-007-9309-6
E. R. Cook, and L. A. Kairiukstis (Eds.). (1990). Methods of dendrochronology. Dordrecht: Springer, Netherlands.
Eriksson, T., Öquist, M. G., and Nilsson, M. B. (2010a). Effects of decadal deposition of nitrogen and sulfur, and increased temperature, on methane emissions from a boreal peatland. J. Geophys. Res. Biogeosciences 115 (G4), G04036. doi:10.1029/2010jg001285
Eriksson, T., Öquist, M. G., and Nilsson, M. B. (2010b). Production and oxidation of methane in a boreal mire after a decade of increased temperature and nitrogen and sulfur deposition. Glob. Change Biol. 16 (7), 2130–2144. doi:10.1111/j.1365-2486.2009.02097.x
Fritsche, J., Osterwalder, S., Nilsson, M. B., Sagerfors, J., Åkerblom, S., Bishop, K., et al. (2014). Evasion of elemental mercury from a boreal peatland suppressed by long-term sulfate addition. Environ. Sci. Technol. Lett. 1 (10), 421–425. doi:10.1021/ez500223a
Frolking, S., and Roulet, N. T. (2007). Holocene radiative forcing impact of northern peatland carbon accumulation and methane emissions. Glob. Change Biol. 13 (5), 1079–1088. doi:10.1111/j.1365-2486.2007.01339.x
Frolking, S., Talbot, J., Jones, M. C., Treat, C. C., Kauffman, J. B., Tuittila, E.-S., et al. (2011). Peatlands in the Earth’s 21st century climate system. Environ. Rev. 19, 371–396. doi:10.1139/a11-014
Fu, Z., Ciais, P., Bastos, A., Stoy, P. C., Yang, H., Green, J. K., et al. (2020). Sensitivity of gross primary productivity to climatic drivers during the summer drought of 2018 in Europe. Philosophical Transactions of the Royal Society B, 375.
Gauci, V., Matthews, E., Dise, N., Walter, B., Koch, D., Granberg, G., et al. (2004). Sulfur pollution suppression of the wetland methane source in the 20th and 21st centuries. Proc. Natl. Acad. Sci. 101 (34), 12583–12587. doi:10.1073/pnas.0404412101
Giesler, R., Björkvald, L., Laudon, H., and Mörth, C. M. (2009). Spatial and seasonal variations in stream water δ34S-dissolved organic matter in Northern Sweden. Environ. Sci. Technol. 43 (2), 447–452. doi:10.1021/es8017946
Granberg, G., Grip, H., Löfvenius, M. O., Sundh, I., Svensson, B. H., and Nilsson, M. B. (1999). A simple model for simulation of water content, soil frost, and soil temperatures in boreal mixed mires. Water Resour. Res. 35 (12), 3771–3782. doi:10.1029/1999wr900216
Granberg, G., Mikkelä, C., Sundh, I., Svensson, B. H., and Nilsson, M. (1997). Sources of spatial variation in methane emission from mires in northern Sweden: A mechanistic approach in statistical modeling. Glob. Biogeochem. Cycles 11 (2), 135–150. doi:10.1029/96gb03352
Granberg, G., Sundh, I., Svensson, B. H., and Nilsson, M. (2001). Effects of temperature, and nitrogen and sulfur deposition, on methane emission from a boreal mire. Ecology 82 (7), 1982–1998. doi:10.1890/0012-9658(2001)082[1982:eotana]2.0.co;2
Grip, H. (2015). Sweden’s first forest hydrology field study 1905–1926: Contemporary relevance of inherited conclusions and data from the rokliden hillslope. Hydrol. Process. 29 (16), 3616–3631. doi:10.1002/hyp.10420
Groß-Schmölders, M., Von Sengbusch, P., Paul Krüger, J., Klein, K., Birkholz, A., Leifeld, J., et al. (2020). Switch of fungal to bacterial degradation in natural, drained and rewetted oligotrophic peatlands reflected in <i&gt;δ</i&gt;<sup&gt;15&lt;/sup&gt;N and fatty acid composition. SOIL 6 (2), 299–313. doi:10.5194/soil-6-299-2020
Gunnarsson, U., Granberg, G., and Nilsson, M. (2004). Growth, production and interspecific competition in Sphagnum: Effects of temperature, nitrogen and sulphur treatments on a boreal mire. New Phytol. 163 (2), 349–359. doi:10.1111/j.1469-8137.2004.01108.x
Helbig, M., Waddington, J. M., Alekseychik, P., Amiro, B. D., Aurela, M., Barr, A. G., et al. (2020a). The biophysical climate mitigation potential of boreal peatlands during the growing season. Environ. Res. Lett. 15 (10), 104004. doi:10.1088/1748-9326/abab34
Helbig, M., Waddington, J. M., Alekseychik, P., Amiro, B. D., Aurela, M., Barr, A. G., et al. (2020b). Increasing contribution of peatlands to boreal evapotranspiration in a warming climate. Nat. Clim. Change 10 (6), 555–560. doi:10.1038/s41558-020-0763-7
Helbig, M., Živković, T., Alekseychik, P., Aurela, M., El-Madany, T. S., Euskirchen, E. S., et al. (2022). Warming response of peatland CO2 sink is sensitive to seasonality in warming trends. Nat. Clim. Change 12 (8), 743–749. doi:10.1038/s41558-022-01428-z
Hu, H., Wang, B., Bravo, A. G., Björn, E., Skyllberg, U., Amouroux, D., et al. (2020). Shifts in mercury methylation across a peatland chronosequence: From sulfate reduction to methanogenesis and syntrophy. J. Hazard. Mater. 387, 121967. doi:10.1016/j.jhazmat.2019.121967
ICOS (2020). Ancillary vegetation measurements in mires. Retrieved from https://fileshare.icos-cp.eu/s/9E3bEdrNtsoQDTH.
Ivarsson, K., and Bjarnason, S. (2009). The long-term soil fertility experiments in southern Sweden. Acta Agric. Scand. 38 (2), 137–143. doi:10.1080/00015128809438477
Jammet, M., Dengel, S., Kettner, E., Parmentier, F. J. W., Wik, M., Crill, P., et al. (2017). Year-round CH&lt;sub&gt;4&lt;/sub&gt; and CO&lt;sub&gt;2&lt;/sub&gt; flux dynamics in two contrasting freshwater ecosystems of the subarctic. Biogeosciences 14 (22), 5189–5216. doi:10.5194/bg-14-5189-2017
Järveoja, J., Nilsson, M. B., Crill, P. M., and Peichl, M. (2020). Bimodal diel pattern in peatland ecosystem respiration rebuts uniform temperature response. Nat. Commun. 11 (1), 4255–4259. doi:10.1038/s41467-020-18027-1
Järveoja, J., Nilsson, M. B., Gažovič, M., Crill, P. M., and Peichl, M. (2018). Partitioning of the net CO2 exchange using an automated chamber system reveals plant phenology as key control of production and respiration fluxes in a boreal peatland. Glob. Change Biol. 24 (8), 3436–3451. doi:10.1111/gcb.14292
Joosten, H., and Couwenberg, J. (2008). “Peatlands and carbon,” in Assessment on peatlands, biodiversity and climate change: Main report. Editors F. Parish, A. Sirin, D. Charman, H. Joosten, T. Minayeva, M. Silviuset al. (Wageningen: Global Environment Centre, Kuala Lumpur and Wetlands International), 99–117. Retrieved from www.peat-portal.net.
Kljun, N., Calanca, P., Rotach, M. W., and Schmid, H. P. (2015). A simple two-dimensional parameterisation for Flux Footprint Prediction (FFP). Geosci. Model Dev. 8 (11), 3695–3713. doi:10.5194/gmd-8-3695-2015
Knox, S. H., Bansal, S., McNicol, G., Schafer, K., Sturtevant, C., Ueyama, M., et al. (2021). Identifying dominant environmental predictors of freshwater wetland methane fluxes across diurnal to seasonal time scales. Glob. Change Biol. 27 (15), 3582–3604. doi:10.1111/gcb.15661
Koebsch, F., Sonnentag, O., Järveoja, J., Peltoniemi, M., Alekseychik, P., Aurela, M., et al. (2020). Refining the role of phenology in regulating gross ecosystem productivity across European peatlands. Glob. Change Biol. 26 (2), 876–887. doi:10.1111/gcb.14905
Korrensalo, A., Männistö, E., Alekseychik, P., Mammarella, I., Rinne, J., Vesala, T., et al. (2018). Small spatial variability in methane emission measured from a wet patterned boreal bog. Biogeosciences 15 (6), 1749–1761. doi:10.5194/bg-15-1749-2018
Ladenberger, A., Andersson, M., Reimann, C., Tarvainen, T., Filzmoser, P., Uhlbäck, J., et al. (2013). Geochemical mapping of agricultural soils and grazing land (GEMAS) in Norway, Finland and Sweden-regional report. Uppsala. Retrieved from http://resource.sgu.se/produkter/sgurapp/s1217-rapport.pdf.
Laine, A. M., Bubier, J., Riutta, T., Nilsson, M. B., Moore, T. R., Vasander, H., et al. (2012). Abundance and composition of plant biomass as potential controls for mire net ecosytem CO2 exchange. Botany 90 (1), 63–74. doi:10.1139/b11-068
Laitinen, J., Rehell, S., Huttunen, A., Tahvanainen, T., Heikkilä, R., and Lindholm, T. (2007). Mire systems in Finland-special view to aapa mires and their water-flow pattern. Suo 58 (1), 1–26.
Łakomiec, P., Holst, J., Friborg, T., Crill, P., Rakos, N., Kljun, N., et al. (2021). Field-scale CH&lt;sub&gt;4&lt;/sub&gt; emission at a subarctic mire with heterogeneous permafrost thaw status. Biogeosciences 18 (20), 5811–5830. doi:10.5194/bg-18-5811-2021
Larsson, A., Segerström, U., Laudon, H., and Nilsson, M. B. (2017). Holocene carbon and nitrogen accumulation rates in a boreal oligotrophic fen. Holocene 27 (6), 811–821. doi:10.1177/0959683616675936
Laudon, H., Hasselquist, E. M., Peichl, M., Lindgren, K., Sponseller, R. A., Lidman, F., et al. (2021). Northern landscapes in transition: Evidence, approach and ways forward using the Krycklan Catchment Study. Hydrol. Process. 35 (4), e14170. doi:10.1002/hyp.14170
Le Quéré, C., Andrew, R., Friedlingstein, P., Sitch, S., Hauck, J., Pongratz, J., et al. (2018). Global carbon budget 2018. Earth Syst. Sci. Data 10 (4), 2141–2194. doi:10.5194/essd-10-2141-2018
Leach, J. A., Larsson, A., Wallin, M. B., Nilsson, M. B., and Laudon, H. (2016). Twelve year interannual and seasonal variability of stream carbon export from a boreal peatland catchment. J. Geophys. Res. Biogeosciences 121 (7), 1851–1866. doi:10.1002/2016jg003357
Lembrechts, J. J., Hoogen, J. V. D., Aalto, J., Ashcroft, M. B., Frenne, P. D., Kemppinen, J., et al. (2021). Global maps of soil temperature. Glob. Change Biol. 28, 3110–3144. doi:10.1111/gcb.16060
Limpens, J., Berendse, F., Blodau, C., Canadell, J. G., Freeman, C., Holden, J., et al. (2008). Peatlands and the carbon cycle: From local processes to global implications – a synthesis. Biogeosciences 5 (5), 1475–1491. doi:10.5194/bg-5-1475-2008
Limpens, J., Granath, G., Aerts, R., Heijmans, M. M. P. D., Sheppard, L. J., Bragazza, L., et al. (2012). Glasshouse vs field experiments: Do they yield ecologically similar results for assessing N impacts on peat mosses? New Phytol. 195 (2), 408–418. doi:10.1111/j.1469-8137.2012.04157.x
Limpens, J., Granath, G., Gunnarsson, U., Aerts, R., Bayley, S., Bragazza, L., et al. (2011). Climatic modifiers of the response to nitrogen deposition in peat-forming Sphagnum mosses: A meta-analysis. New Phytol. 191 (2), 496–507. doi:10.1111/j.1469-8137.2011.03680.x
Lindroth, A., Lund, M., Nilsson, M., Aurela, M., Christensen, T. R., Laurila, T., et al. (2007). Environmental controls on the CO 2 exchange in north European mires. Tellus B Chem. Phys. Meteorology 59 (5), 812–825. doi:10.3402/tellusb.v59i5.17061
Lund, M., Lafleur, P. M., Roulet, N. T., Lindroth, A., Christensen, T. R., Aurela, M., et al. (2010). Variability in exchange of CO2 across 12 northern peatland and tundra sites. Glob. Change Biol. 16 (9), 2436–2448.
Malmström, C. (1923). Degerö Stormyr. En botanisk, hydrologisk och utvecklingshistorisk undersökning över ett nordsvenskt myrkomplex (Meddelanden från Statens Skogsförsöksanstalt No. 20). Stockholm. Retrieved from https://pub.epsilon.slu.se/10091/1/medd_statens_skogsforskningsanst_020_01.pdf.
Martí, M., Juottonen, H., Robroek, B. J. M., Yrjälä, K., Danielsson, Å., Lindgren, P. E., et al. (2015). Nitrogen and methanogen community composition within and among three Sphagnum dominated peatlands in Scandinavia. Soil Biol. Biochem. 81, 204–211. doi:10.1016/j.soilbio.2014.11.016
Martí, M., Nilsson, M. B., Danielsson, Å., Lindgren, P.-E., and Svensson, B. H. (2019). Strong long-term interactive effects of warming and enhanced nitrogen and sulphur deposition on the abundance of active methanogens in a boreal oligotrophic mire. Mires Peat 24, 1–14.
Masing, V., Botch, M., and Läänelaid, A. (2010). Mires of the former soviet union. Wetl. Ecol. Manag. 18 (4), 397–433. doi:10.1007/s11273-008-9130-6
Matthes, J. H., Sturtevant, C., Verfaillie, J., Knox, S., and Baldocchi, D. (2014). Parsing the variability in CH4 flux at a spatially heterogeneous wetland: Integrating multiple eddy covariance towers with high-resolution flux footprint analysis. J. Geophys. Res. Biogeosciences 119 (7), 1322–1339. doi:10.1002/2014jg002642
Metzger, C., Nilsson, M. B., Peichl, M., and Jansson, P. E. (2016). Parameter interactions and sensitivity analysis for modelling carbon heat and water fluxes in a natural peatland, using CoupModel v5. Geosci. Model Dev. 9 (12), 4313–4338. doi:10.5194/gmd-9-4313-2016
Moore, T. R., and Dalva, M. (1993). The influence of temperature and water table position on carbon dioxide and methane emissions from laboratory columns of peatland soils. J. Soil Sci. 44 (4), 651–664. doi:10.1111/j.1365-2389.1993.tb02330.x
Mulqueen, J. (1986). Hydrology and drainage of peatland. Environ. Geol. Water Sci. 9 (1), 15–22. doi:10.1007/bf02439882
Nielsen, C. S., Hasselquist, N. J., Nilsson, M. B., Öquist, M., Järveoja, J., and Peichl, M. (2019). A novel approach for high-frequency in-situ quantification of methane oxidation in peatlands. Soil Syst. 3 (1), 4. doi:10.3390/soilsystems3010004
Nijp, J. J., Limpens, J., Metselaar, K., Peichl, M., Nilsson, M. B., van der Zee, S. E. A. T. M., et al. (2015). Rain events decrease boreal peatland net CO2 uptake through reduced light availability. Glob. Change Biol. 21 (6), 2309–2320. doi:10.1111/gcb.12864
Nijp, J. J., Metselaar, K., Limpens, J., Bartholomeus, H. M., Nilsson, M. B., Berendse, F., et al. (2019). High-resolution peat volume change in a northern peatland: Spatial variability, main drivers, and impact on ecohydrology. Ecohydrology 12 (6), e2114. doi:10.1002/eco.2114
Nijp, J. J., Metselaar, K., Limpens, J., Gooren, H. P. A., and van der Zee, S. E. A. T. M. (2017a). A modification of the constant-head permeameter to measure saturated hydraulic conductivity of highly permeable media. MethodsX 4, 134–142. doi:10.1016/j.mex.2017.02.002
Nijp, J. J., Metselaar, K., Limpens, J., Teutschbein, C., Peichl, M., Nilsson, M. B., et al. (2017b). Including hydrological self-regulating processes in peatland models: Effects on peatmoss drought projections. Sci. Total Environ. 580, 1389–1400. doi:10.1016/j.scitotenv.2016.12.104
Nilsson, B. M., Ottosson Lofvenius, M., Peichl, M., and Holst, J.ICOS Ecosystem Thematic Center (2022). Warm winter 2020 ecosystem eddy covariance flux product from Degerö. (Version 1.0) [dataset].
Nilsson, M. B., Baath, E., and Soderstrom, B. (2011). The microfungal communities of a mixed mire in northern Sweden. Can. J. Bot. 70 (2), 272–276. doi:10.1139/b92-037
Nilsson, M., and Eriksson, T. (2011). Boreal mire carbon exchange-long term effects of climate change and nitrogen and sulphur additions. Geophys. Res. Abstr. 13, 2011–8602.
Nilsson, M., Sagerfors, J., Buffam, I., Laudon, H., Eriksson, T., Grelle, A., et al. (2008). Contemporary carbon accumulation in a boreal oligotrophic minerogenic mire – A significant sink after accounting for all C-fluxes. Glob. Change Biol. 14 (10), 2317–2332. doi:10.1111/j.1365-2486.2008.01654.x
Økland, R. (1990). Regional variation in SE Fennoscandian mire vegetation. Nordic J. Bot. 10 (3), 285–310. doi:10.1111/j.1756-1051.1990.tb01774.x
Olid, C., Bindler, R., Nilsson, M. B., Eriksson, T., and Klaminder, J. (2017). Effects of warming and increased nitrogen and sulfur deposition on boreal mire geochemistry. Appl. Geochem. 78, 149–157. doi:10.1016/j.apgeochem.2016.12.015
Olid, C., Nilsson, M. B., Eriksson, T., and Klaminder, J. (2014). The effects of temperature and nitrogen and sulfur additions on carbon accumulation in a nutrient-poor boreal mire: Decadal effects assessed using 210Pb peat chronologies. J. Geophys. Res. Biogeosciences 119 (3), 392–403. doi:10.1002/2013jg002365
Osterwalder, S., Bishop, K., Alewell, C., Fritsche, J., Laudon, H., Åkerblom, S., et al. (2017). Mercury evasion from a boreal peatland shortens the timeline for recovery from legacy pollution. Sci. Rep. 7 (1), 16022–16029. doi:10.1038/s41598-017-16141-7
Osterwalder, S., Eugster, W., Feigenwinter, I., and Jiskra, M. (2020). Eddy covariance flux measurements of gaseous elemental mercury over a grassland. Atmos. Meas. Tech. 13 (4), 2057–2074. doi:10.5194/amt-13-2057-2020
Osterwalder, S., Fritsche, J., Alewell, C., Schmutz, M., Nilsson, M. B., Jocher, G., et al. (2016). A dual-inlet, single detector relaxed eddy accumulation system for long-term measurement of mercury flux. Atmos. Meas. Tech. 9 (2), 509–524. doi:10.5194/amt-9-509-2016
Osterwalder, S., Sommar, J., Åkerblom, S., Jocher, G., Fritsche, J., Nilsson, M. B., et al. (2018). Comparative study of elemental mercury flux measurement techniques over a Fennoscandian boreal peatland. Atmos. Environ. 172, 16–25. doi:10.1016/j.atmosenv.2017.10.025
Pakarinen, P. (1995). “Classification of boreal mires in Finland and scandinavia: A review,” in Classification and inventory of the world’s wetlands. Advances in vegetation science. Editors C. M. Finlayson, and A. G. van der Valk (Springer Netherlands), 16, 29–38.
Papale, D., Agarwal, D. A., Baldocchi, D., Cook, R. B., Fisher, J. B., and Ingen, C. van. (2012). “Database maintenance, data sharing policy, collaboration,” in Eddy covariance. Editors M. Aubinet, T. Vesala, and D. Papale (Dordrecht: Springer Atmospheric Sciences, Springer), 399–424.
Peel, M. C., Finlayson, B. L., and McMahon, T. A. (2007). Updated world map of the Köppen-Geiger climate classification. Hydrology Earth Syst. Sci. 11 (5), 1633–1644. doi:10.5194/hess-11-1633-2007
Peichl, M., Gažovič, M., Vermeij, I., de Goede, E., Sonnentag, O., Limpens, J., et al. (2018). Peatland vegetation composition and phenology drive the seasonal trajectory of maximum gross primary production. Sci. Rep. 8 (1), 8012. doi:10.1038/s41598-018-26147-4
Peichl, M., Öquist, M., Ottosson Löfvenius, M., Ilstedt, U., Sagerfors, J., Grelle, A., et al. (2014). A 12-year record reveals pre-growing season temperature and water table level threshold effects on the net carbon dioxide exchange in a boreal fen. Environ. Res. Lett. 9 (5), 055006. doi:10.1088/1748-9326/9/5/055006
Peichl, M., Sagerfors, J., Lindroth, A., Buffam, I., Grelle, A., Klemedtsson, L., et al. (2013). Energy exchange and water budget partitioning in a boreal minerogenic mire. J. Geophys. Res. Biogeosciences 118 (1), 1–13. doi:10.1029/2012jg002073
Peichl, M., Sonnentag, O., and Nilsson, M. B. (2015). Bringing color into the picture: Using digital repeat photography to investigate phenology controls of the carbon dioxide exchange in a boreal mire. Ecosystems 18 (1), 115–131. doi:10.1007/s10021-014-9815-z
Peltola, O., Hensen, A., Belelli Marchesini, L., Helfter, C., Bosveld, F. C., van den Bulk, W. C. M., et al. (2015). Studying the spatial variability of methane flux with five eddy covariance towers of varying height. Agric. For. Meteorology 214–215, 456–472. doi:10.1016/j.agrformet.2015.09.007
Peltola, O., Vesala, T., Gao, Y., Räty, O., Alekseychik, P., Aurela, M., et al. (2019). Monthly gridded data product of northern wetland methane emissions based on upscaling eddy covariance observations. Earth Syst. Sci. Data 11 (3), 1263–1289. doi:10.5194/essd-11-1263-2019
Ratcliffe, J. L., Lowe, D. J., Schipper, L. A., Gehrels, M. J., French, A. D., and Campbell, D. I. (2020). Rapid carbon accumulation in a peatland following Late Holocene tephra deposition, New Zealand. Quat. Sci. Rev. 246, 106505. doi:10.1016/j.quascirev.2020.106505
Rinne, J., Tuovinen, J.-P., Klemedtsson, L., Aurela, M., Holst, J., Lohila, A., et al. (2020). Effect of the 2018 European drought on methane and carbon dioxide exchange of northern mire ecosystems. Philosophical Transactions of the Royal Society B, 375.1810
Robroek, B. J. M., Wubs, E. R. J., Martí, M., Zając, K., Andersen, J. P., Andersson, A., et al. (2014). Microclimatological consequences for plant and microbial composition in Sphagnum-dominated peatlands. Boreal Environ. Res. 19 (3), 195–208. Retrieved from https://www.dora.lib4ri.ch/wsl/islandora/object/wsl%3A9545/.
Ruuhijärvi, R. (1960). “Über die regionale Einteilung der nordfinnischen Moore,” in Annales botanici societas zoologica botanica fennica “vanamo, 31. Retrieved from https://books.google.se/books/about/Über_die_regionale_Einteilung_der_nordf.html?id=i7VQAAAAYAAJ&redir_esc=y.1
Ruuhijärvi, R. (1983). “The Finnish mire types and their regional distribution,” in Ecosystems of the world, 4 B. Mires: Swamp, bog, fen and moor. Regional studies. Editor A. G. P. Gore (Amsterdam: Elsevier), 47–49.
Ruuhola, T., Nikula, A., Nivala, V., Nevalainen, S., and Matala, J. (2016). Effects of bedrock and surficial deposit composition on moose damage in young forest stands in Finnish Lapland. Silva Fenn. 50 (3). doi:10.14214/sf.1565
Rydin, H., Sjörs, H., and Löfroth, M. (1999). Mires. Acta Phytogeogr. Suec. 84, 91–112. Retrieved from https://www.researchgate.net/publication/286628326_7_Mires.
Schubert, P., Eklundh, L., Lund, M., and Nilsson, M. (2010). Estimating northern peatland CO2 exchange from MODIS time series data. Remote Sens. Environ. 114 (6), 1178–1189. doi:10.1016/j.rse.2010.01.005
Segura, J. H., Nilsson, M. B., Schleucher, J., Haei, M., Sparrman, T., Székely, A., et al. (2019). Microbial utilization of simple carbon substrates in boreal peat soils at low temperatures. Soil Biol. Biochem. 135, 438–448. doi:10.1016/j.soilbio.2019.06.006
SGU (1963). Geological survey of Sweden, bedrock map, 1:50000. Retrieved from https://apps.sgu.se/kartvisare/kartvisare-berg-50-250-tusen.html.
SGU. (1990). Geological survey of Sweden, quaternary deposits map 1:25000. Retrieved from https://apps.sgu.se/kartvisare/kartvisare-jordarter-25-100.html.
Shen, X., Liu, Y., Zhang, J., Wang, Y., Ma, R., Liu, B., et al. (2022). Asymmetric impacts of diurnal warming on vegetation carbon sequestration of marshes in the qinghai tibet plateau. Glob. Biogeochem. Cycles 36 (7), e2022GB007396. doi:10.1029/2022gb007396
SLU (2021). Reference measurements of the climate at the experimental forests at SLU. Retrieved September 20, 2021, from https://www.slu.se/en/departments/field-based-forest-research/environment/climate-data/referenceclimate/.
SMHI (2021). Swedish Climate data. Retrieved September 20, 2021, from http://www.smhi.se/data/meteorologi.
Sponseller, R. A., Blackburn, M., Nilsson, M. B., and Laudon, H. (2018). Headwater mires constitute a major source of nitrogen (N) to surface waters in the boreal landscape. Ecosystems 21 (1), 31–44. doi:10.1007/s10021-017-0133-0
St. Louis, V. L., Rudd, J. W. M., Kelly, C. A., Beaty, K. G., Flett, R. J., and Roulet, N. T. (1996). Production and loss of methylmercury and loss of total mercury from boreal forest catchments containing different types of wetlands. Environ. Sci. Technol. 30 (9), 2719–2729. doi:10.1021/es950856h
Swindles, G. T., Morris, P. J., Baird, A. J., Blaauw, M., and Plunkett, G. (2012). Ecohydrological feedbacks confound peat-based climate reconstructions. Geophys. Res. Lett. 39 (11), 4. doi:10.1029/2012gl051500
Swindles, G. T., Morris, P. J., Wheeler, J., Smith, M. W., Bacon, K. L., Turner, T. E., et al. (2016). Resilience of peatland ecosystem services over millennial timescales: Evidence from a degraded British bog. J. Ecol. 104 (3), 621–636. doi:10.1111/1365-2745.12565
Turetsky, M. R., Kotowska, A., Bubier, J., Dise, N. B., Crill, P., Hornibrook, E. R. C., et al. (2014). A synthesis of methane emissions from 71 northern, temperate, and subtropical wetlands. Glob. Change Biol. 20 (7), 2183–2197. doi:10.1111/gcb.12580
Ueyama, M., Knox, S. H., Delwiche, K. B., Bansal, S., Riley, W. J., Baldocchi, D., et al. (2023). Modeled production, oxidation, and transport processes of wetland methane emissions in temperate, boreal, and Arctic regions. Glob. Change Biol. 00 (2022), 2313–2334. doi:10.1111/gcb.16594
Virkkala, A.-M., Natali, S. M., Rogers, B. M., Watts, J. D., Savage, K., June Connon, S., et al. (2022). The ABCflux database: Arctic–boreal CO&lt;sub&gt;2&lt;/sub&gt; flux observations and ancillary information aggregated to monthly time steps across terrestrial ecosystems. Earth Syst. Sci. Data 14, 179–208. doi:10.5194/essd-14-179-2022
Vuichard, N., and Papale, D. (2015). Filling the gaps in meteorological continuous data measured at FLUXNET sites with ERA-Interim reanalysis. Earth Syst. Sci. Data 7 (2), 157–171. doi:10.5194/essd-7-157-2015
Wania, R., Ross, I., and Prentice, I. C. (2009). Integrating peatlands and permafrost into a dynamic global vegetation model: 2. Evaluation and sensitivity of vegetation and carbon cycle processes. Glob. Biogeochem. Cycles 23 (3), 15. doi:10.1029/2008gb003413
Wiedermann, M. M., Gunnarsson, U., Ericson, L., and Nordin, A. (2009a). Ecophysiological adjustment of two Sphagnum species in response to anthropogenic nitrogen deposition. New Phytol. 181 (1), 208–217. doi:10.1111/j.1469-8137.2008.02628.x
Wiedermann, M. M., Gunnarsson, U., Nilsson, M. B., Nordin, A., and Ericson, L. (2009b). Can small-scale experiments predict ecosystem responses? An example from peatlands. Oikos 118 (3), 449–456. doi:10.1111/j.1600-0706.2008.17129.x
Wiedermann, M. M., and Nilsson, M. B. (2020). Peatland vegetation patterns in a long term global change experiment find no reflection in belowground extracellular enzyme activities. Wetlands 40 (6), 2321–2335. doi:10.1007/s13157-020-01377-3
Wiedermann, M. M., Nordin, A., Gunnarsson, U., Nilsson, M. B., and Ericson, L. (2007). Global change shifts vegetation and plant–parasite interactions in a boreal mire. Ecology 88 (2), 454–464. doi:10.1890/05-1823
Wißkirchen, K., Tum, M., Günther, K. P., Niklaus, M., Eisfelder, C., and Knorr, W. (2013). Quantifying the carbon uptake by vegetation for Europe on a 1 km2 resolution using a remote sensing driven vegetation model. Geosci. Model Dev. 6, 1623–1640. doi:10.5194/gmd-6-1623-2013
Wu, J., and Roulet, N. T. (2014). Climate change reduces the capacity of northern peatlands to absorb the atmospheric carbon dioxide: The different responses of bogs and fens. Glob. Biogeochem. Cycles 28 (10), 1005–1024. doi:10.1002/2014gb004845
Wu, J., Roulet, N. T., Nilsson, M., Lafleur, P., and Humphreys, E. (2012). Simulating the carbon cycling of northern peatlands using a land surface scheme coupled to a wetland carbon model (CLASS3W-mwm). Atmosphere-Ocean 50 (4), 487–506. doi:10.1080/07055900.2012.730980
Yi, C., Li, R., Wolbeck, J., Xu, X., Nilsson, M., Aires, L., et al. (2010). Climate control of terrestrial carbon exchange across biomes and continents. Environ. Res. Lett. 5 (3), 034007. doi:10.1088/1748-9326/5/3/034007
Yu, Z. C. (2012). Northern peatland carbon stocks and dynamics: A review. Biogeosciences 9 (10), 4071–4085. doi:10.5194/bg-9-4071-2012
Yurova, A., Wolf, A., Sagerfors, J., and Nilsson, M. (2007). Variations in net ecosystem exchange of carbon dioxide in a boreal mire: Modeling mechanisms linked to water table position. J. Geophys. Res. 112 (G2), G02025. doi:10.1029/2006jg000342
Zhao, J., Peichl, M., and Nilsson, M. B. (2016a). Enhanced winter soil frost reduces methane emission during the subsequent growing season in a boreal peatland. Glob. Change Biol. 22 (2), 750–762. doi:10.1111/gcb.13119
Zhao, J., Peichl, M., Öquist, M., and Nilsson, M. B. (2016b). Gross primary production controls the subsequent winter CO2 exchange in a boreal peatland. Glob. Change Biol. 22 (12), 4028–4037. doi:10.1111/gcb.13308
Zhou, S., Zhang, Y., Caylor, K. K., Luo, Y., Xiao, X., Ciais, P., et al. (2016a). Explaining inter-annual variability of gross primary productivity from plant phenology and physiology. Agric. For. Meteorology 226–227, 246–256. doi:10.1016/j.agrformet.2016.06.010
Zhou, Y., Wu, X., Ju, W., Chen, J. M., Wang, S., Wang, H., et al. (2016b). Global parameterization and validation of a two-leaf light use efficiency model for predicting gross primary production across FLUXNET sites. J. Geophys. Res. Biogeosciences 121 (4), 1045–1072. doi:10.1002/2014jg002876
Keywords: greenhouse gas fluxes, global change, manipulation experiments, mercury, stream carbon export, boreal biome, biogeochemistry, wetland
Citation: Noumonvi KD, Ågren AM, Ratcliffe JL, Öquist MG, Ericson L, Tong CHM, Järveoja J, Zhu W, Osterwalder S, Peng H, Erefur C, Bishop K, Laudon H, Nilsson MB and Peichl M (2023) The Kulbäcksliden Research Infrastructure: a unique setting for northern peatland studies. Front. Earth Sci. 11:1194749. doi: 10.3389/feart.2023.1194749
Received: 27 March 2023; Accepted: 05 May 2023;
Published: 31 May 2023.
Edited by:
Dominic E. L. Ong, Griffith University, AustraliaReviewed by:
Mashal Alawi, Dr. Brill + Partner GmbH, GermanyXiangjin Shen, Chinese Academy of Sciences, China
Copyright © 2023 Noumonvi, Ågren, Ratcliffe, Öquist, Ericson, Tong, Järveoja, Zhu, Osterwalder, Peng, Erefur, Bishop, Laudon, Nilsson and Peichl. This is an open-access article distributed under the terms of the Creative Commons Attribution License (CC BY). The use, distribution or reproduction in other forums is permitted, provided the original author(s) and the copyright owner(s) are credited and that the original publication in this journal is cited, in accordance with accepted academic practice. No use, distribution or reproduction is permitted which does not comply with these terms.
*Correspondence: Koffi Dodji Noumonvi, a29mZmkubm91bW9udmlAc2x1LnNl